- Hildebrand Department of Petroleum and Geosystems Engineering and Center for Subsurface Energy and the Environment, The University of Texas at Austin, Austin, TX, United States
As the important role of enhanced oil recovery (EOR) in meeting the world’s energy requirement is growing, use of nanoparticles in lieu of, or in combination with, the existing EOR agents to expand EOR’s applicable range is receiving significant attention. Two of the most actively investigated applications are: 1) wettability alteration by addition of nanoparticles into the waterflood injection water, and 2) use of nanoparticle-stabilized Pickering foams and emulsions mainly for EOR process mobility control. As comprehensive reviews are recently available on these topics, two other emerging nanoparticle applications are critically reviewed here: 1) nanoparticle addition for enhanced polymer flooding, and 2) use of magnetic nanoparticles for oil displacement control. Three and five proposed mechanisms of these two applications are critically reviewed, respectively. The most recent progresses are covered, and the challenges and possible future works are discussed.
1 Introduction
After primary recovery, reservoirs are often waterflooded in secondary recovery until water cuts of the production wells no longer make it profitable. At the end of a waterflood, normally 60–70% of the original oil in place remains. The remaining oil is categorized as unswept/bypassed oil, due to the poor mobility ratio between water and oil, and residual oil which is capillary trapped due to interfacial forces. Tertiary (or enhanced) oil recovery, EOR, is sometimes employed to produce some of the remaining oil. EOR methods include chemical (e.g., polymers and surfactants), solvent (e.g., miscible CO2), thermal (e.g., steam), and other (e.g., microbial). The choice of whether to pursue EOR and which method depends on economics as well as the reservoir conditions (permeability, heterogeneity, temperature, oil saturation, brine salinity, etc.) at the end of the waterflood.
In chemical EOR, polymers are added to injected water and are used to recover bypassed oil. The polymer solutions are more viscous than water and thus provide mobility control. The viscosity is a function of polymer concentration, molecular weight, brine salinity and hardness, and temperature. Increasing the polymer concentration can be cost ineffective, large molecular weight polymers may not transport through low permeability rock, and the polymer may degrade at high temperature and salinity. Attempts to reduce the cost of polymer flooding and expand the window of conditions that can be exploited by polymer flooding are ongoing.
Nanoparticles have special physical and chemical properties and are being studied as a potential agent for enhanced oil recovery (EOR) (Huh et al., 2019). The advancement of general nanoparticle EOR has been reviewed by a few recent papers (Cheraghian and Hendraningrat, 2016a; Cheraghian and Hendraningrat, 2016b; Agista et al., 2018; Corredor et al., 2019; Kazemzadeh et al., 2019; Ali et al., 2020; Xu et al., 2020; Franco et al., 2021; Hassan et al., 2021; Panchal et al., 2021). In this paper, we focus on two evolving technologies: nanoparticle enhanced polymer flooding and magnetic nanoparticle flooding.
Nanoparticle-enhanced polymer flooding: While polymer flooding has been widely employed, the high-salinity, high-temperature conditions in many oil reservoirs make the use of the commonly employed polymer, partially hydrolyzed polyacrylamide (HPAM), difficult. This is because the large effective size of the HPAM molecule in water is due to the carboxyl anion’s electrostatic repulsion, and when the salinity of water is higher than ∼2 wt%, the effectiveness of the electrostatic repulsion decreases substantially. As a result, HPAM’s hydrodynamic radius decreases sharply along with its viscosifying ability. Another critical limitation of HPAM is that, because it is a very long, single-chain molecule, it is highly susceptible to the chain scission. This usually occurs when an oxidizing radical, such as O or Fe, attacks the oxygen in amide group along the polymer chain. A number of improvements to alleviate the weaknesses of HPAM have recently been made. Probably the most effective way so far developed is to attach 2-acrylamide-2-methylpropane sulfonate (AMPS) group, instead of (or in addition to) the carboxyl group, along the polymer chain (Jouenne et al., 2019). Since AMPS is a much stronger acidic ion, the buffering effect of the salt ions to reduce the electrostatic repulsion between chain elements can be significantly reduced. Figures 1A,B show the molecular structures of the HPAM and AMPS-modified HPAM. Figure 2 shows the dependence of the HPAM viscosity on salinity with and without divalent ions (Levitt and Pope, 2008).
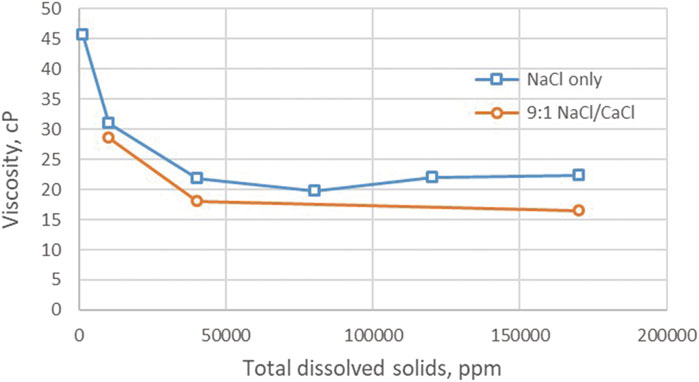
FIGURE 2. Dependence of HPAM polymer viscosity on salinity (based on Levitt and Pope, 2008).
While a significant improvement, these modifications so far do not fully meet the requirements for successful applications to Middle East-type reservoirs. In attempts to overcome such limitations of using HPAM and its modifications, the addition of various nanoparticles to the injection polymer solution has been actively investigated for the following potential benefits: 1) maintaining high polymer viscosity at harsh reservoir conditions, 2) reduction of polymer chemical/thermal degradation, 3) polymer retention reduction by sacrificial adsorption of nanoparticles in porous media, and 4) wettability alteration by nanoparticle adsorption on solid. The most commonly used nanoparticle is made of silica (SiO2) because it is easy to functionalize its surface by hydrogen-bonding various chemicals to its OH sites. Other oxides are also used, such as
Magnetic Nanoparticle Flooding: Magnetic nanoparticles, whose diameter is smaller than 100 nm (Huh et al., 2019), (Rosensweig, 1985), may have only one magnetic domain if its size is smaller than the critical diameter, while there are usually many magnetic domains in bulk materials. A magnetic domain is a region of uniform direction of magnetization in a magnetic material. The critical diameter ranges between 15 and 162 nm in different materials (Caizer and Aliofkhazraei, 2015). Superparamagnetism describes the phenomenon in which a magnetic nanoparticle rotates to align with any external magnetic field like a very small magnet (Wang, 2021), as shown in Figure 3A. Such magnetic properties may help mobilize trapped oil in various ways, e.g., by deforming the oil blob (Saint-Martin de Abreu Soares, 2015) (Figure 3B), as further described below.
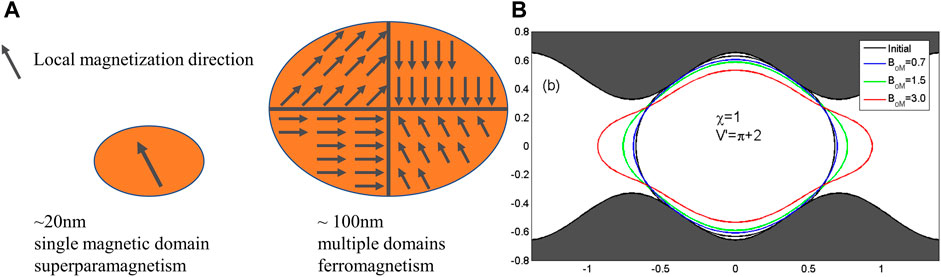
FIGURE 3. (A) Superparamagnetism and ferromagnetism (based on Wang, 2021). (B) Oil blob deformation in a pore in a magnetic field parallel to the flow direction of ferrofluid (Saint-Martin de Abreu Soares, 2015).
Due to their magnetic properties, flooding with magnetic nanoparticle-containing fluids (also known as ferrofluids) and an application of external magnetic field has the potential to achieve additional/higher oil recovery than flooding with general nanoparticles without an external magnetic field.
Over the past decade, several mechanisms have been proposed and studied for the electromagnetic EOR with magnetic nanoparticles. Most of the applications can be categorized by the magnetic field frequency, where low frequency applications tend to take advantage of the magnetic forces and high frequency applications rely more on the induction heat. These mechanisms may contribute together to the oil mobilization, and it is worthwhile to discuss how each method works in specific scenarios compared to others.
2 Nanoparticle enhanced polymer flooding
2.1 Possible benefits of nanoparticle addition to polymer flood
In attempts to overcome the current limitations of using HPAM and its modifications, the addition of various nanoparticles to the injection polymer solution has been actively investigated. Comprehensive recent reviews on the topic (Corredor et al., 2019), (Gbadamosi et al., 2019), (Agi et al., 2018) are available. Some of the earlier works used the vendor-supplied nanoparticles without recognizing the importance of the chemical’s surface coating, thus not reporting their nature. This makes their assessment difficult because the interactions between the polymer and nanoparticles are largely governed by the nature of ligands on the particle surface and along the polymer chain. In this section, some of the notable research efforts on the following possible benefits will be described: 1) polymer viscosity increase, as discussed above, 2) reduction of polymer chemical/thermal degradation, 3) polymer retention reduction by sacrificial adsorption of nanoparticles in porous media, and 4) wettability alteration by nanoparticle adsorption on solid.
2.1.1 Polymer viscosity increase
As described above, the AMPS-modified HPAM has a good salinity tolerance and some resistance to chemical/thermal degradation. Its currently available molecular weight is only 2–4 million (e.g., for SNF’s SAV10), meaning that a high concentration is needed to generate a desired viscosity with a resultant high cost. In order to overcome the limitation, a potential solution is to connect these polymer molecules by adding a low concentration of surface-coated nanoparticles to serve as “linkers” between polymer molecules. As the polymer molecules will be present as “fluffy globules”, connecting two polymer molecules by one molecule’s chain end to another’s chain end, as schematically shown in Figure 4A, will be ideal but generally very difficult (Gbadamosi et al., 2019). As described below, a more practical approach would be to make the nanoparticles serve as linkers between the “fluffy globules”, as conceptually depicted in Figure 4B. Compared with individual polymer globules, a much higher viscosity is expected once the globules are connected by nanoparticles to form long “strings”.
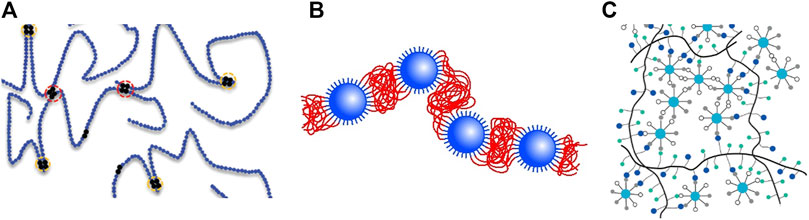
FIGURE 4. (A) Ideal case of NP connecting two polymer molecules by their chain ends (Gbadamosi et al., 2019); (B) Conceptual picture of nanoparticle linking two polymer “fluffy globules”, rather than chain elements; (C) Small nanoparticles serving as inter-molecular linker, but also as intra-molecular associator (Cao et al., 2018a).
On maintaining high polymer viscosity, a common approach is to add nanoparticles to serve as “linkers” between polymer molecules. Available literature (Zhu et al., 2014), (Hu et al., 2017) suggests that small nanoparticles serve as inter-molecular linkers (which is desirable) but also cause intra-molecular association (which is undesirable). This conceptual picture implies that not only nanoparticles but also the chain elements of another polymer molecule can easily penetrate into a polymer molecule’s “fluffy globule” inner domain, as depicted in Figure 4C (Cao et al., 2018a). Literature data however shows that the size of nanoparticles (∼50–200 nm, usually in slightly aggregated state) (Huh et al., 2019), (Bagaria et al., 2013) is not much smaller than polymer’s hydrodynamic radius (∼100–1,000 nm) (Ghosh and Mohanty, 2020), (Silva et al., 2018). Thus, a rational approach would be to make the nanoparticles serve as linkers between the “fluffy globules” of polymer (Figure 4B), so that the viscosity of the “string” becomes significantly higher than that of the individual globules. To make the particle surface have sufficient repulsion so that it would not penetrate into the polymer’s inner domain, but still allow its attachment to the polymer globule, available literature is critically examined with the above improvement objective in mind.
Cao et al. (Cao et al., 2018a), (Cao et al., 2018b) described their nanoparticle surface coating development to obtain the polymer viscosity increase in some detail, which is reviewed here as a good example of similar research efforts. They applied to silica nanoparticle (diameter = 7–40 nm) different amounts of surface coating of 3-aminopropyl-triethoxy-silane (APTES) to study their effect on the rheology of AMPS-modified poly (acrylamide) (PM). Figure 5A shows the nanoparticle size in DI water at pH = 6, and Figure 5B shows the dependence of zeta potential of the particles on pH (Cao et al., 2018b). In the figures, NS is the nanoparticle as received from vendor (presumably without any surface coating) and for ANS-1, -2 and -4, the mole ratio of APTES to–OH on silica nanoparticle surface are 0.2, 0.5 and 1.5, respectively. Figure 5A shows that, while the nanoparticle size increased with more APTES coating (peak values from 134 to 179 nm for ANS-2 to -4), the “bare” nanoparticle size is larger (peak value ∼240 nm) suggesting that minor aggregation occurred (Cao et al., 2018b). Note that the nanoparticle sizes are quite comparable to the hydrodynamic radius of polymer, as described above. Figure 5B shows that with the APTES coating, the nanoparticle’s surface charge is now positive, making them electrostatically attractive to the negatively charged sulfonate ligands along the polymer chain, so that the nanoparticles can be readily attached to the polymer chain (Cao et al., 2018b). Figure 6A shows that, while the addition of the APTES-coated nanoparticles significantly increased the polymer viscosity, its undesirable dependence on the salinity is still significant (Cao et al., 2018b). On the other hand, the dependence of polymer viscosity on the hardness of brine is much less with the nanoparticles with higher APTES surface density (Figure 6B) (Cao et al., 2018b). In an attempt to improve upon the above APTES-coated silica nanoparticles, Cao et al. (Cao et al., 2019) also developed a coating that additionally includes octyl-triethoxyl-silane (OTES) which is more hydrophobic than APTES, thus allowing the nanoparticle to have both electrostatic and hydrophobic interactions with the polymer chain (Israelachvili, 2011), (Meyer et al., 2006).
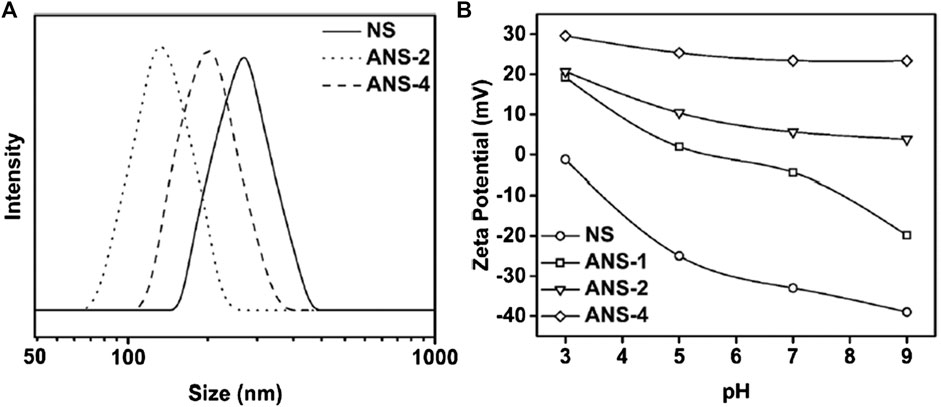
FIGURE 5. (A) Size of untreated (NS) and APTES-treated nanoparticles; (B) dependence of zeta potential on pH for nanoparticles of varying APTES treatment (Cao et al., 2018b).
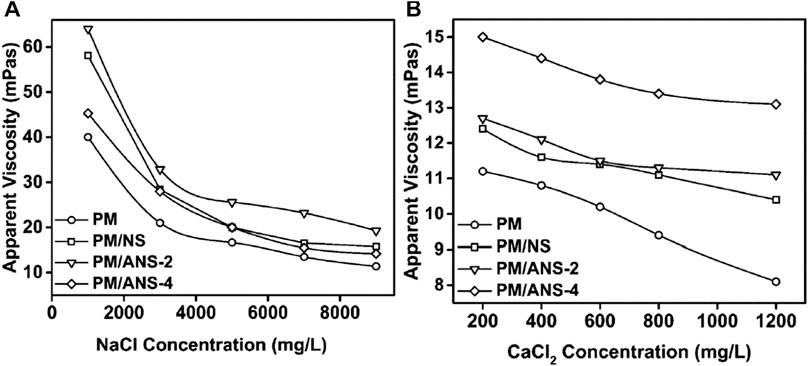
FIGURE 6. (A) Dependence of polymer viscosity on salinity with addition of different APTES-treated nanoparticles; (B) dependence of viscosity on hardness (Cao et al., 2018b).
Zheng et al. (Zheng et al., 2017) applied to the silica nanoparticle surface two different coatings: hexamethyl-disilazane (HMDS) and hexadecyl-trimethoxy-silane (HDTS) at different mole ratios of the coating material to the -OH on the silica surface. Figure 7A shows the schematics of the HMDS-attached silica nanoparticle, and Figure 7B shows the dependence of the polymer viscosity on the nanoparticle concentration for untreated nanoparticle and HMDA- and HDTS-treated nanoparticles (Zheng et al., 2017). With the HMDS coating, the polymer viscosity improved over the untreated nanoparticle but the HDTS coating did not help. This suggests that the hydrophobic end of the silane attached to the particle plays an important role in linking the nanoparticle to the polymer, and the hydrophobic interactions between them need to be better understood. Figure 8 shows the dependence of the HPAM (MW = 20 million) viscosity on the temperature, for different concentrations of untreated nanoparticles (Zheng et al., 2017). While the viscosity of polymer without nanoparticle addition decreased substantially with temperature increase, the nanoparticle addition not only increased the viscosity but also reduced the temperature-dependent reduction of the polymer viscosity. This compares with the dependence of the viscosity on temperature with the similar addition of “as-received, untreated” nanoparticles, which shows a substantial decrease in viscosity with temperature increase, as shown in Figure 9 (Hu et al., 2017). It is noted that, at almost any salinity, the bare silica nanoparticle is unstable and quickly aggregates. This suggests that the “untreated” nanoparticle has some surface coating that needs to be characterized.
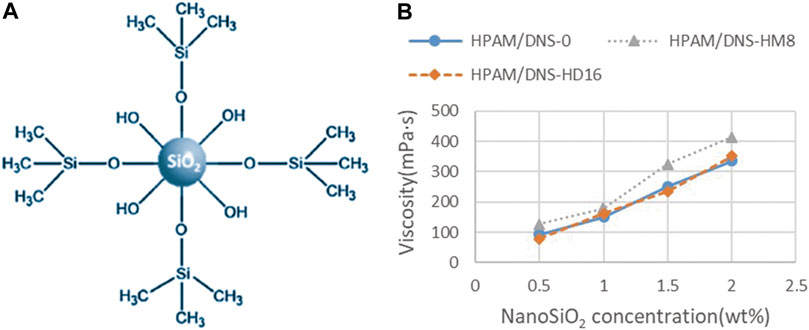
FIGURE 7. (A) Schematic diagram of HMDA-treated silica nanoparticle; (B) dependence of polymer viscosity on salinity, with addition of untreated, and HMDS- and HDTS-treated nanoparticles (based on Zheng et al., 2017).
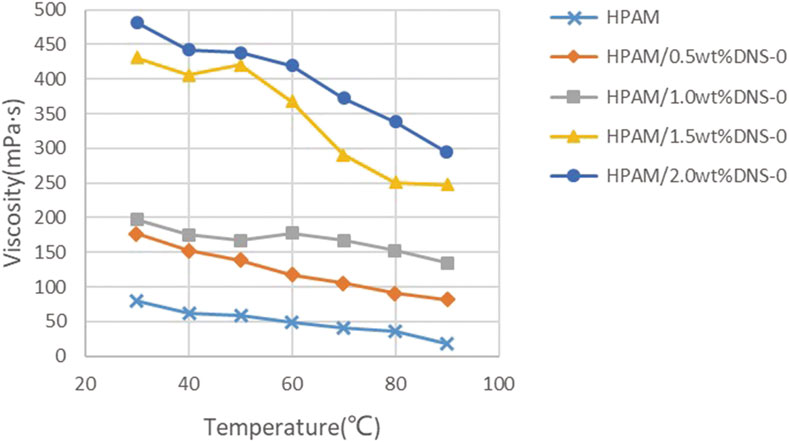
FIGURE 8. Viscosities of HPAM, and with addition of different concentration of silica NPs. Effect of temperature (based on Zheng et al., 2017).
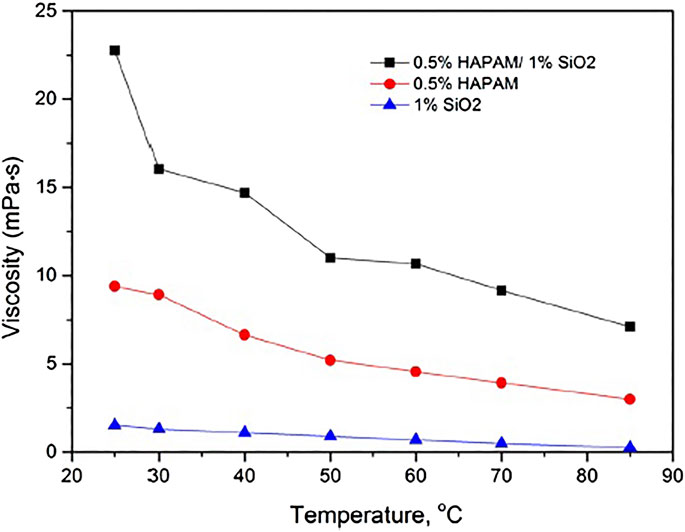
FIGURE 9. Viscosities of HAPAM, silica NP, and their combination. Effect of temperature (Hu et al., 2017).
The quantitative assessment on the effects of nanoparticle addition on polymer viscosity is rather difficult because, for a number of studies, the chemical nature of the nanoparticle surface coating is not provided. Overall, with increase in nanoparticle concentration, the polymer viscosity increase is observed, and the reduction in viscosity due to temperature increase is less severe. As described above, the nanoparticle surface coating has a significant effect on polymer viscosity, but the detailed molecular mechanism for the nanoparticle-polymer linkage and the consequent viscosity increase is yet to be determined.
In addition to the study on the effects of various nanoparticles on polymer viscosity, a number of researchers performed oil recovery core flood experiments. The results showed that, with addition of nanoparticles, not only was the viscosity higher than that of nanoparticle-free polymer but also a higher oil recovery was generally observed (Maghzi et al., 2014). When they increased the nanoparticles concentration, a higher oil recovery was attained (Hu et al., 2017).
2.1.2 Reduction of Polymer chemical degradation
For a long, single-chain polymer molecule such as HPAM, the chemical and mechanical degradation, i.e., the simple scission of the chain, drastically reduces the polymer molecular weight. This is critically important because the dependence of polymer viscosity on the molecular weight is quite large, as revealed by the Mark-Houwink equation that provides the polymer’s intrinsic viscosity,
where a and b are polymer-specific constants. The intrinsic viscosity, which is a measure of the hydrodynamic size of polymer molecules, is the dominant parameter for polymer viscosity (Jouenne et al., 2019). An effective method developed to alleviate the oxidizing agent’s attack of the -c-c- bond of the HPAM molecular chain is to attach N-vinyl pyrrolidone (NVP) group along the chain, but then such modification adds cost. An alternate strategy to reduce the impact of the degradation is to connect the smaller polymer molecules with the nanoparticles as the linker, as depicted in Figure 4B. It is important to note that the effects of degradation are relatively less for smaller polymer molecules. The amide groups in the HPAM molecule can associate with silanol groups at the surface of silica, and the degradation tendency can be reduced as observed by Zhu et al. (Zhu et al., 2014). As described above (Cao et al., 2018a), (Cao et al., 2018b), (Cao et al., 2019), the silica nanoparticles functionalized with amino- and other silanes are found to behave much better in improving the chemical/thermal stability of HPAM in harsh conditions, compared with the cases of polymer only or polymer with untreated nanoparticles.
As the polymer degradation mechanisms even without nanoparticle addition are varied and complex, the detailed mechanism on how the nanoparticle helps reduce the degradation is yet to be determined.
2.1.3 Polymer retention reduction
Although polymer retention in porous media is also caused by trapping at pore throats and pore crevices, adsorption is the main mechanism that removes polymer from the solution and results in a significant viscosity reduction. The adsorption measurement by the bulk static method is generally greater than that by dynamic flow conditions (Lakatos et al., 1981), but is commonly employed to study the effects of different process parameters, such as the addition of nanoparticles.
Polymer solutions containing nanoparticles have been generally found to have less adsorption compared to polymer-only solutions (Sirk et al., 2009), (Phenrat et al., 2010). The results of static adsorption experiments show that nanoparticles play a major role in polymer adsorption on the rock surface (Cheraghian, 2016). Cheraghian et al. (Cheraghian et al., 2014) studied the effect of silica nanoparticle concentration on the retention reduction of HPAM polymer. Static polymer adsorption experiments were conducted at room temperature by adding crushed sandstone or carbonate rock samples to the polymer solution and stirring until adsorption was complete. Figure 10 shows the polymer adsorption data on sandstone samples with the addition of 2.25 wt% (A24) and 1.8 wt% (A41) nanoparticles; and on carbonate samples with similar addition of nanoparticles (B16 and B52, respectively). It shows that the adsorption in sandstone is much smaller than that in carbonate and that polymer solutions containing more silica nanoparticles have less adsorption. These results clearly show that the nanoparticles served the role of sacrificial adsorption agent.
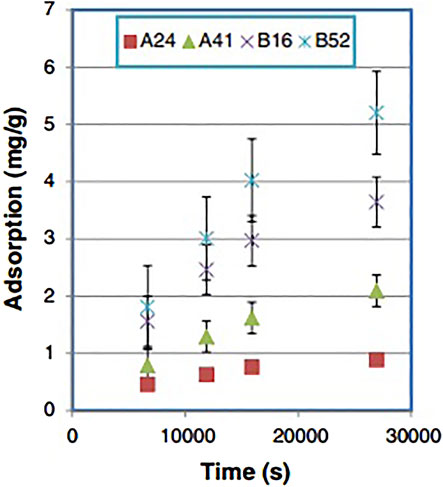
FIGURE 10. Static adsorption under different nanoparticle concentrations based on the weight of stone of polymers onto sandstone and carbonate (Cheraghian et al., 2014).
2.1.4 Wettability alteration
Another factor that may increase the reservoir oil recovery when using nanoparticle-enhanced polymer flooding is that it can change an oil-wet reservoir to water-wet. It is well documented that oil recovery is affected by the wettability of the reservoir with more oil being recovered from water-wet rocks compared to oil-wet rocks. Extensive research efforts have been made to investigate the effects of “nanofluid” injection for improved oil recovery (Cheraghian and Hendraningrat, 2016a), (Cheraghian and Hendraningrat, 2016b), (Hendraningrat and Torsæter, 2015), (Hendraningrat and Torsæter, 2016). Usually, the alteration of wettability is examined by measurements of interfacial tensions and contact angles. Nanoparticles have been found to reduce the contact angle that polymer solution makes on the rock surface. A higher nanoparticle concentration would be expected to better reduce contact angle; however, the increased nanoparticle concentration may also reduce the stability of fluid because of the aggregation and sedimentation of nanoparticles, which results in a specific threshold of nanoparticle concentration (Cheraghian et al., 2014).
Because the wettability is directly affected by the interfacial tension via the Young’s equation (Berg, 2010), the effect of nanoparticles on interfacial tension between HPAM solution and oil has been studied. El-hoshoudy et al. (El-hoshoudy et al., 2016) studied the effect of silica nanoparticle on the interfacial tension of polymer solution and oil recovery. Flooding experiments were performed with a sandstone core using HPAM with different concentrations of silica (0, 1, 2, 3, and 4 g/L). Figures 11A,B show that with the increase of nanoparticle concentration, the crude oil/brine interfacial tension decreases, and the cumulative oil recovery increases. The interfacial tension reduction is not sufficient enough to mobilize the oil by the capillary-number mechanism, indicating that the increased oil recovery is most likely due to the wettability alteration. It is observed that a concentration threshold appears to exist at ∼2 g/L with a maximum oil recovery there; but the mechanism for synergy between polymer and nanoparticle is not yet known. A pore-level study on the synergy mechanism, similar to that between surfactant and nanoparticle (Xu et al., 2017), is warranted.
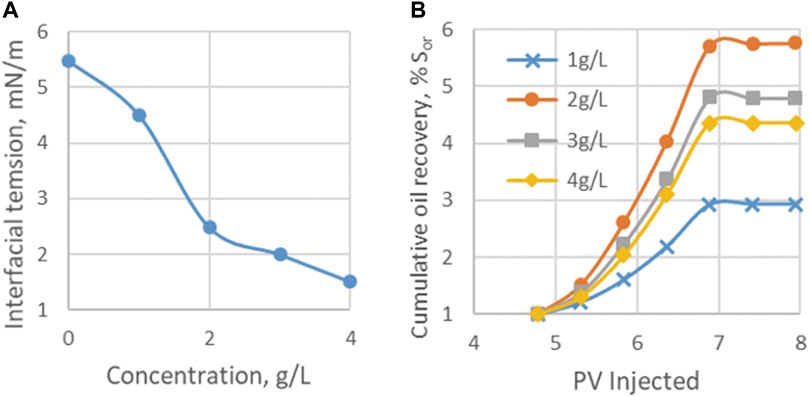
FIGURE 11. (A) Interfacial tension values of HPAM–SiO2; (B) HPAM–SiO2 cumulative oil recovery related to injected pore volume (based on El-hoshoudy et al., 2016).
Sharma et al. (Sharma et al., 2016) studied the effect of nanoparticles on polymer and surfactant-polymer flooding. Coreflood experiments were carried out at both 30 and 90°C with SiO2 nanoparticles at 1 wt%. Figure 12 shows the relative permeability curves for brine and oil before and after the nanoparticle-added polymer flooding at 30°C. The addition of 0.1 wt% SiO2 nanoparticles clearly increased the oil relative permeability while the brine relative permeability was reduced, showing the wettability alteration. They also showed that the nanoparticle addition increased the oil recovery.
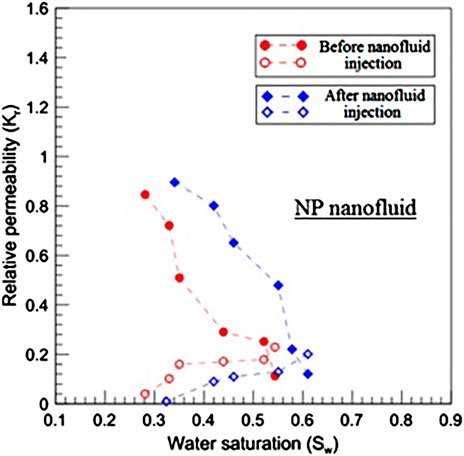
FIGURE 12. Relative permeability curves for brine (empty symbols) and oil (filled symbols) before and after nanoparticle-added polymer flooding (Sharma et al., 2016).
Kumar et al. (Kumar et al., 2022) studied the effect of different types of nanoparticles (SiO2 and GO) and salinities (NaCl and CaCl2) on the interfacial tensions and contact angles. Figure 13A shows that the crude oil/brine interfacial tension decreases linearly with increasing nanoparticle concentration, when HPAM-SiO2 is in NaCl brine. Figure 13B shows that the contact angle, measured on a silica plate with the fluids of (a), also decreased for NP concentration from 0.05 to 0.1%. While the decreases for both were relatively minor but showed positive trends.
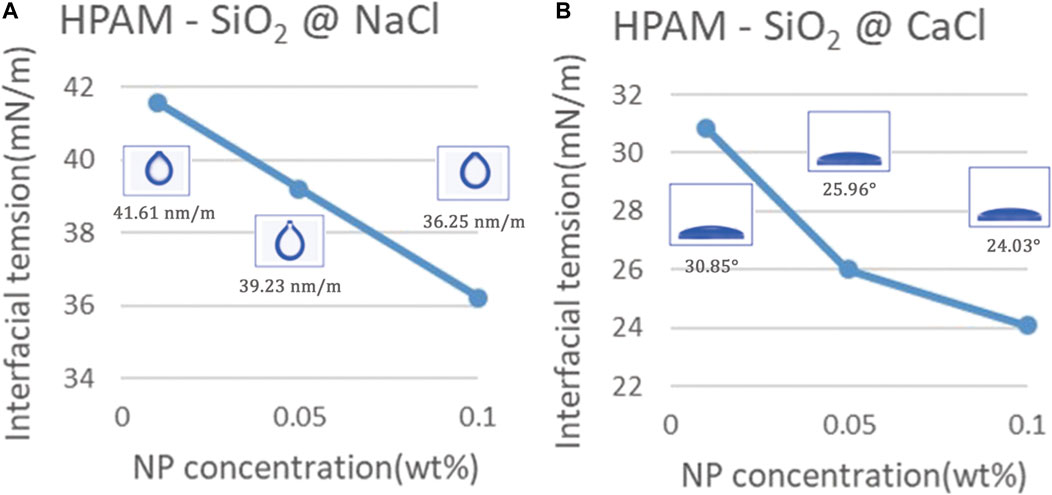
FIGURE 13. (A) Crude oil/brine interfacial tension, and (B) contact angle on silica plates, for HPAM-SiO2@NaCl at different nanoparticle concentrations (based on Kumar et al., 2022).
3 Magnetic nanoparticle flooding
Although the suspension of magnetic nanoparticles and its interaction with an external magnetic field have been intensively studied in the last century (Rosensweig, 1985), the first published preliminary numerical and experimental investigation on the enhanced oil recovery potential was by Prodanović et al., in 2010 (Prodanovic et al., 2010). Since then, several mechanisms of the magnetic nanoparticle flooding have been studied for static, low-frequency (<10 Hz), and high-frequency magnetic fields (>1 kHz). A few review papers in the recent years have briefly touched upon this topic (Agista et al., 2018), (Kazemzadeh et al., 2019), (Xu et al., 2020), (Hassan et al., 2021), (Panchal et al., 2021). However, much remains to be investigated as the mechanisms are complex and we lack a fundamental understanding of how a fluid and a ferrofluid displace each other in porous media under the influence of an external magnetic field. In this section, we review several mechanisms about magnetic nanoparticle flooding published in literature. More specifically, we focus on the mechanisms that require the presence of an external magnetic field. EOR studies using dielectric nanoparticles are included because of their similarity to magnetic nanoparticles in high-frequency magnetic fields. Applications using magnetic nanoparticles without applying an external magnetic field (Betancur et al., 2020) are not discussed in this paper. Most of the published investigations are at core scale or pore scale as well as a few studies using larger lab-scale sand packs (Huang et al., 2017) and rock cores (Esmaeilnezhad et al., 2018a), while reservoir scale applications are only briefly discussed (Wang et al., 2021).
It is also worth mentioning that the magnetic nanoparticles, like other nanoparticles, can alter the fluid viscosity and interfacial properties, and can have stability and adsorption issues. Furthermore, assuming the stability of the suspension, the electromagnetic forces on the ferrofluid could potentially improve oil mobilization due to several mechanisms elaborated in subsequent sections.
3.1 Magnetic nanoparticle flooding with application of low-frequency magnetic field
Magnetic nanoparticles stably suspended in a liquid carrier, when exposed to an external magnetic field, are subject to magnetic forces. Macro-scale motion and deformation can result from the magnetic forces (Rosensweig, 1985). Existing studies on magnetic nanoparticle flooding that apply direct magnetic forces are based on a static magnetic field or slow rotating magnetic fields of sub-Hertz frequency. Magnetic fields of higher frequency are not yet used to apply direct magnetic forces for the purposes of oil recovery in published works.
A static magnetic field can impact the two-phase flow of ferrofluid (the flooding fluid carrying suspended magnetic nanoparticles) and fluid (the oil) via three mechanisms: the pore-scale heterogeneity of the magnetic field, the micron-scale heterogeneity of the ferrofluid, and the reservoir-scale heterogeneity of the magnetic field. We discuss the first two mechanisms together, followed by the third mechanism.
3.1.1 General low-frequency magnetic field
A magnetic field induces an additional pressure
where
Even when a uniform external magnetic field
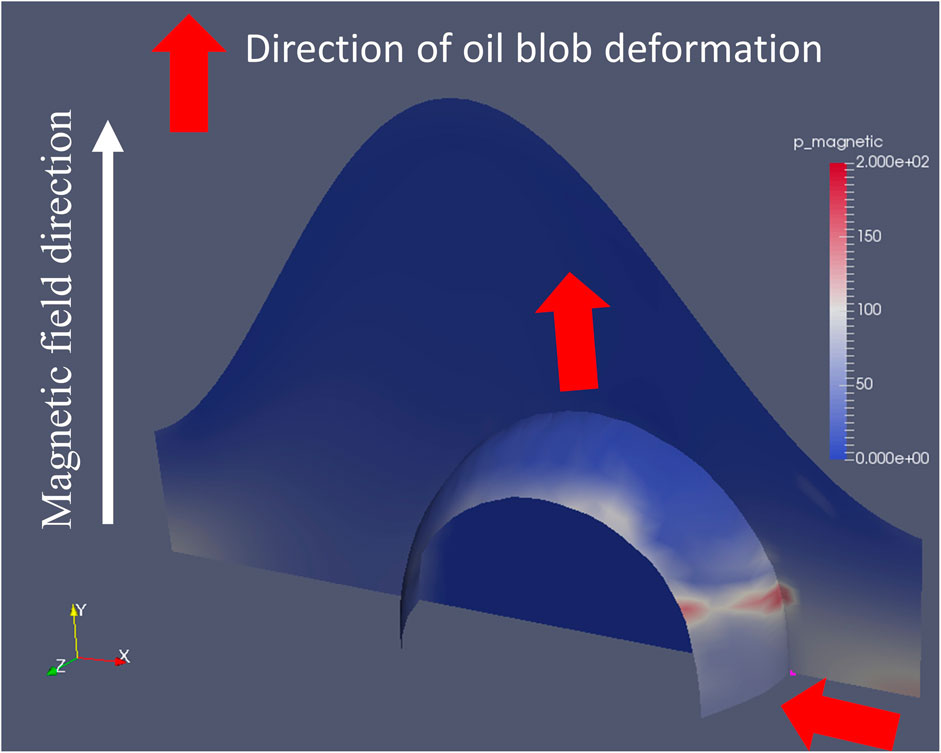
FIGURE 14. Magnetic-induced pressure in the pore near the oil blob in a ferrofluid flooding. The magnetic field is transverse to the flow direction. The pressure is plotted in a cross section (the plane) in the pore and at the oil/ferrofluid interface (the curved surface). The higher magnetic-induced pressure at the throat pushes the oil blob away from the throat, making it harder to mobilize the oil blob (based on Wang et al., 2021).
Magnetic nanoparticles form temporal micro-structures when exposed to external disturbances of either magnetic fields (Robbes et al., 2011), (Tracy and Crawford, 2013) or shears (Ishida et al., 2021), as shown in Figure 15. These micro-structures can be permanentized by solidifying the fluid (Tracy and Crawford, 2013). These micro-structures can either interact with the oil droplets and apply additional propulsion to the oil droplet, or adhere to the oil droplets and provide additional hydrodynamic forces to the oil droplets (Wang, 2021), (Wang et al., 2021). Either way, the oil droplets may have an increased chance of displacement from the pore.
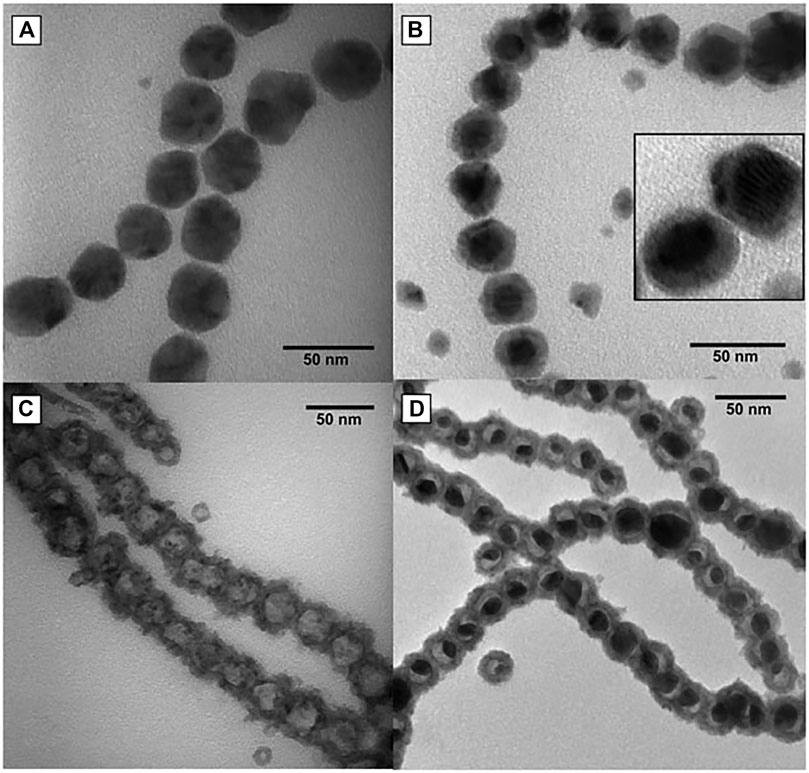
FIGURE 15. TEM images of magnetic nanoparticle chains. (A) Co, (B) Au/Co core-shell, (C) hollow CoO shells, and (D) hollow CoO shells containing smaller Au nanoparticles. (Tracy and Crawford, 2013).
Wang et al. experimentally demonstrated the EOR effect of a 2.6 mT static magnetic field transverse to the flow direction in a ferrofluid flooding despite of unsuccessful approaches by other researchers, as shown in Figure 16 (Wang et al., 2021), (Wang et al., 2020). They measured the oil saturation in a micromodel during a ferrofluid flooding before and after the application of an external magnetic field. In one experiment, the application of the static magnetic field mobilized 86.2% of the oil that was not mobilized by the ferrofluid flooding alone. They also observed the chaining of oil droplets both in the micromodel and in a Hele Shaw cell.
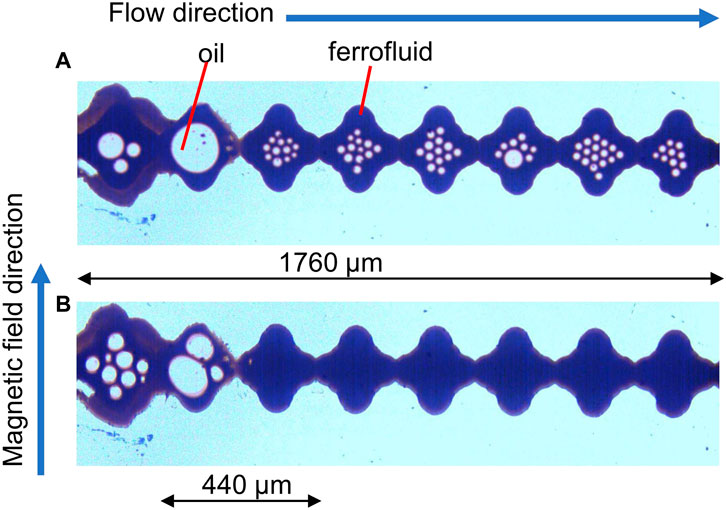
FIGURE 16. Displacement of small oil blobs in a converging/diverging single channel with first ferrofluid only, then ferrofluid and magnetic field: (A) The micromodel was first flooded without magnetic field for 40 h, and then (B) the flooding continued with a static magnetic field for another 26 h and 100% of the small oil blobs in the right six pores were displaced. This is a portion of the entire micromodel and upstream and downstream are not shown (based on Wang et al., 2021).
Whether the mobilization of oil droplets is because of the non-uniform magnetic-induced pressure or the nanoparticle chains is not yet clear. However, presently it appears that the existence of nanoparticle chains could better explain the increased oil recovery when the magnetic field is transverse to the flow direction than the theory where we have uniform suspension of nanoparticles (which was the assumption made in simulation in Figure 14). No matter which mechanism is dominant, the magnetic forces aided mobilization of the trapped oil in a foot-long micromodel when the magnetic field is slowly rotating at a sub-Hertz frequency (Wang et al., 2021).
The increased oil recovery driven by a static magnetic field was also confirmed by Esmaeilnezhad et al. in core flooding (Esmaeilnezhad et al., 2018a). However, they only guessed the oil recovery was caused by an improvement in sweep efficiency without further analysis.
The magnetic field strength around a static magnetic source decreases at the rate of
3.1.2 Non-uniform low-frequency magnetic field
Magnetic materials are attracted to higher magnetic field intensity gradient direction. In a ferrofluid, the body force density
where
The body force term can be plugged into the Darcy’s equation to study the flow of ferrofluid in porous media.
A static magnetic source, e.g., a magnet, induces a large-scale non-uniform magnetic field and attracts magnetic nanoparticles and ferrofluid to the magnetic source. This effect can be used to manipulate the flooding fluid and improve the volume sweep efficiency, as shown in Figure 17 (Huang et al., 2017).
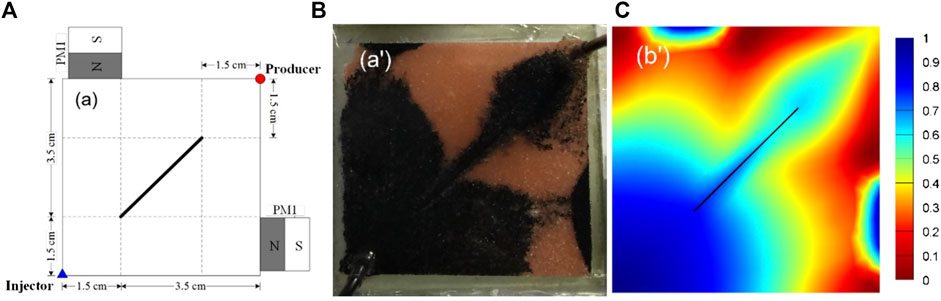
FIGURE 17. (A) Schematic of sand pack with injector, producer, and permanent magnets. (B) Photo of sand pack with ferrofluid (dark color). (C) Simulated ferrofluid saturation in the sand pack (Huang et al., 2017).
However, the corresponding magnetic body force decreases at the rate of
Further, manipulating flooding fluid by magnetic attraction forces requires the magnetic field source (a magnet) being placed in the reservoir. When applied in the field, the magnet needs to be placed in a well that is not flowing. The production planning is thus to be studied.
3.2 Magnetic nanoparticle flooding with induction heat using high-frequency magnetic field
Electromagnetic heating of downhole fluids have been studied a long time ago to heat the heavy oil and reduce its viscosity (Abernethy, 1976). This heating method transports the electromagnetic energy to downhole and convert the energy to heat in the reservoir. It does not require injection of hot materials, and may get slower temperature decline from the wellbore into the reservoir. Electromagnetic heating effectiveness and efficiency depends on many factors. To enhance the electromagnetic heating, one way is to add magnetic nanoparticles.
When the external magnetic field changes direction, the magnetic nanoparticles in a ferrofluid rotate to align with the external magnetic field. The heating power density of the nanoparticles is evaluated by the specific absorption rate (SAR) is the (Chou, 1990), (Jordan et al., 1993)
where
where
Davidson et al. measured the SAR in the lab for oil production applications (Davidson et al., 2012) and the procedure can be applied to EOR related applications. Phenrat et al. proposed using the electromagnetic heating of nanoparticles to enhance the dichlorination of trichloroethylene in contaminated ground water and soil (Phenrat et al., 2016).
The EOR phenomena of many nanoparticles in a high-frequency external magnetic field has been studied in glass-bead packs and sand packs by Yahya et al., including
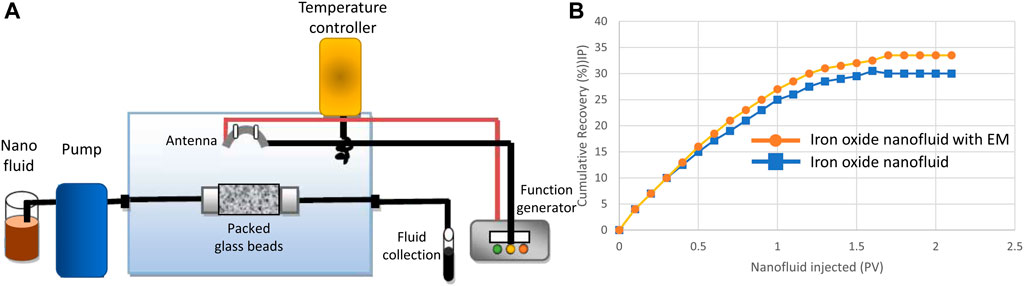
FIGURE 18. (A) Schematic of electromagnetic heating of core sample aided by
A major challenge of EOR using electromagnetic heating using nanoparticles is the limited penetration of the electromagnetic wave. A ground penetrating radar is a device used to detect inside the rock using electromagnetic waves (Utsi, 2017). The typical penetration depth of a modern ground penetrating radar is smaller than 20 m into the rock, limited by the penetration depth of the electromagnetic waves. The application of electromagnetic heating may be limited to near-well regions.
Another challenge of the application of this mechanism is the power of the antenna. Heating the reservoir fluid requires significant amount of energy which is supposed to be transferred to the rock using the electromagnetic waves. Thus, the design and operation of the downhole antenna emitting the electromagnetic waves needs further investigation.
3.3 Direct rheology change
External magnetic can impact the ferrofluid viscosity (de Vicente et al., 2011), (Ashtiani et al., 2015). Thus, it is possible to alter the viscosity of the ferrofluid in subsurface to plug certain flow channels to improve the sweep efficiency. Compared to the polymers, the nanoparticles are smaller in size and has the potential to reach small pores with less formation damage concern.
Divandari et al. performed ferrofluid flooding with a static magnetic field in a micromodel and compared the oil recovery with polymer flooding (Divandari et al., 2019), (Divandari et al., 2021). However, they did not analyze whether and how the magnetic nanoparticles helped suppressing fingering, while the oil recovery could be also explained by the mechanism of magnetic nanoparticle flooding with a low-frequency magnetic field. The results were not compared with nanoparticle flooding without magnetic field, dismantling the significance of quantitative analysis. Moreover, the magnetic field in their work was so strong that the nanoparticles started to segregate from the fluid, making the ferrofluid no longer a stable suspension.
Altering the rheology of the ferrofluid by the magnetic field requires high enough concentration of magnetic nanoparticles and strong enough magnetic field. Feasibility of magnetic nanoparticle conformance control still needs much research.
3.4 Interfacial tension alteration
Nanoparticles, because of their small size, can act as a surfactant to change the superficial contact angle between two fluids. At very high temperature of 500°C, a high frequency magnetic field can further alter the interfacial tension and contact angle between the oil and the nanoparticle suspension (Adil et al., 2020). However, the interfacial tension alteration temperature is too high for current oil and gas applications and is well above the formation temperature of oil and gas (Quigley and Mackenzie, 1988). Whether the constant-temperature interfacial tension alteration by the electromagnetic waves is significant at the oil and gas reservoir temperature is not yet clear and needs further investigation.
3.5 Synergy with polymer and separation from production fluid
Stabilization of polymers using nanoparticles has been reviewed in the last section, including using magnetic nanoparticles (Bagaria et al., 2013).
The gelling behavior of polymers is a function of temperature, as shown in Figure 19 (Panthi et al., 2015). If superparamagnetic nanoparticles are attached to the polymer, the temperature of the polymer can be increased by induction heating when an alternating external magnetic field is applied (Panthi et al., 2015), (Huh et al., 2015). Thus, the gelling of polymers can be controlled by the electromagnetic waves. By selecting the electromagnetic wave direction and power, precise control of gelling zone in the underground is possible. This technique can be used to block the high permeability zone and improve the sweeping efficiency.
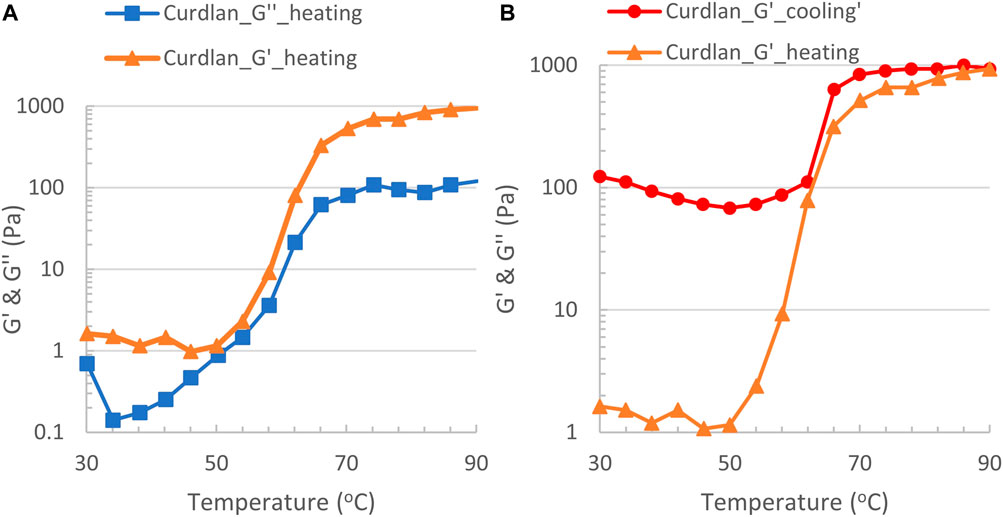
FIGURE 19. (A) Storage moduli G′ and los moduli G″ of 6 wt% curdlan suspension in water during heating, and (B) G′ during heating and cooling (based on Panthi et al., 2015).
The major challenges of this technique include 1) the stability of the polymer at the reservoir temperature, 2) matching the gelling temperature of the polymer with the reservoir temperature, and 3) design of antenna for precise electromagnetic wave emission.
Shape memory polymers can switch between different states triggered by an exterior environment, including temperature (Mather et al., 2009). They can be used to bridge and seal vugs and fractures as a lost circulation material and can be removed by biodegradation (Tabatabaei et al., 2021). The possibility of using magnetic-nanoparticle-modified shape memory polymers to seal the high permeability zone is thus worth investigation.
Even with a static magnetic field, a ferrofluid of polymer coated magnetic nanoparticles can have increased viscosity when the magnetic field becomes stronger. Esmaeilnezhad et al. measured the viscosity of such a ferrofluid at different magnetic field strengths and proposed to use this technique to improve the sweep efficiency (Esmaeilnezhad et al., 2018b).
Additionally, magnetic nanoparticles are being connected to polymers for removal of the latter from produced fluid (Ko et al., 2017; Simonsen et al., 2018; Leong et al., 2020; Zhou et al., 2020). In these applications, magnetic nanoparticles are connected to polymers for flooding. No magnetic field is applied during the flooding. Using permanent or electric magnets, the polymers can be easily separated from the produced water, as shown in Figure 20 (Ko et al., 2017). As mentioned previously, magnetic nanoparticles are attracted to high magnetic field gradient region, i.e., the magnets. The recycled polymers can then be reused.
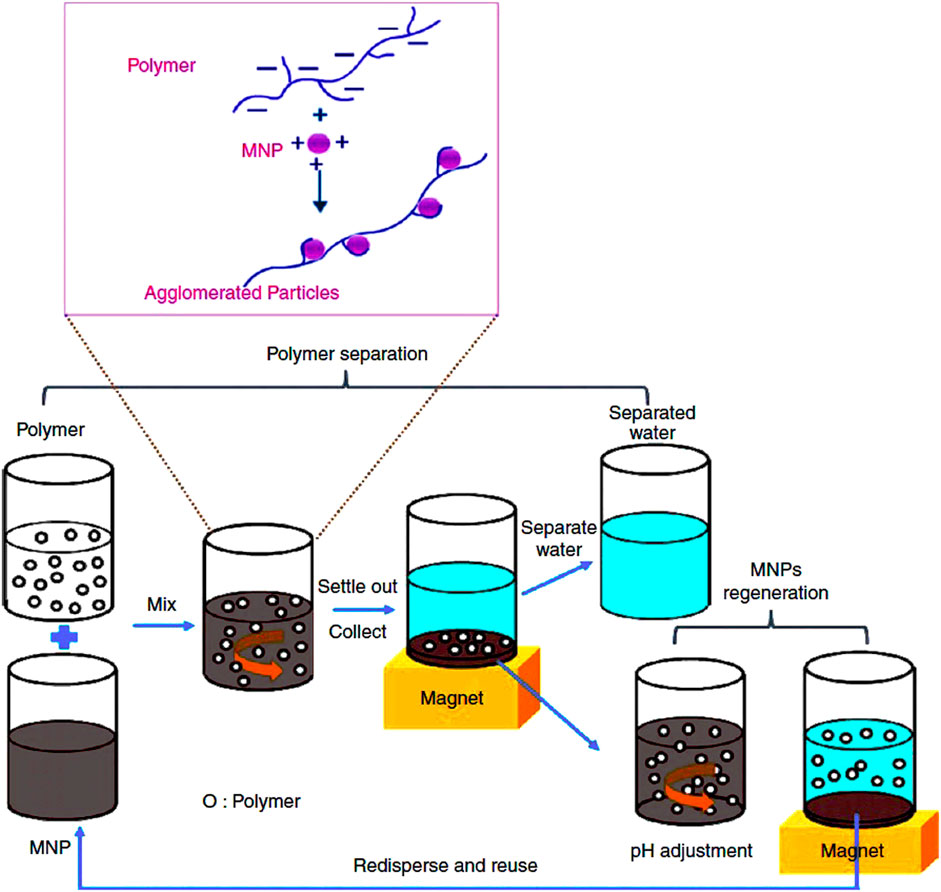
FIGURE 20. Schematic of removal and recycle of polymer and magnetic nanoparticles (based on Ko et al., 2017).
Much research is to be done to study the impact of the added magnetic nanoparticle on the polymers before these polymers can be applied in the field (Sabzi Dizajyekan et al., 2020). To recycle the polymer, the sustainability of the polymer during EOR, production, and separation need to be studied and new polymer design may be needed.
3.6 Monitoring of magnetic flooding
Oil displacement observation is significant in research and can be useful in production. Samavati et al. developed a fiber Bragg grating oil flow sensing system for magnetic nanoparticle EOR (Samavati et al., 2021). An optical fiber is planted into the rock to measure the front the flooding fluid. This method may be useful in addition to current experimental methods.
4 Conclusion
In this review, the recent research on the development of nanoparticle-enhanced polymer flooding and magnetic nanoparticle flooding has been discussed with focus on their mechanisms.
Nanoparticle-Enhanced Polymer Flooding: Two important observations from this brief review of recent research are: 1) In developing the nanoparticles for the above-described purposes, the core material (usually different metal oxides) generally serves merely as the substrate to attach the surface coating chemical ligands. For this reason, the silica nanoparticle whose surface with -OH sites can easily attach various chemicals (especially a variety of silanes) is the favored nanoparticles; and 2) the nanoparticle size is generally assumed to be much smaller than the size of the polymer “fluffy globule”, so that the nanoparticles can easily penetrate into the polymer chain’s inner domain. Experimental data show that the nanoparticle size is not much smaller than the size of polymer globule.
A more promising approach for nanoparticle surface functionalization would be to make the nanoparticles serve as linkers between the “fluffy globules”, as schematically shown in Figure 4B. Once the polymer globules are stringed together being connected by the nanoparticles, the viscosity of the “string” is expected to be significantly higher than that of the individual polymer globules. The above conceptual picture provides us the direction to optimize nanoparticle size and functionalization of its surface:
1) The nanoparticle size relative to the polymer’s hydrodynamic radius (rH) should not be too small. At the same time, it should not be too large to attach “several” polymer globule to its surface, because such will restrict the polymer globule’s viscosifying ability.
2) The particle surface and the polymer chains have sufficient repulsion, e.g., by the excluded volume effect of chains (Israelachvili, 2011), so that the particle would not penetrate into the “fluffy globule”.
3) At the same time, some functional groups on the nanoparticle surface have a hydrophobic attraction with, e.g., the amide groups that are still along the polymer chain in addition to the AMPS or carboxyl anions. Such will allow attachment of the nanoparticle to the polymer globule. The hydrophobic interaction (Meyer et al., 2006) between the polymer chain and the surface coating’s ligands, as well as the electrostatic interaction, should be carefully considered.
The nanoparticles with proper surface functionalization, when added at small concentrations to the injection polymer, may indeed bring forth the increase in polymer viscosity, more tolerance to high temperature, and high salinity and hardness of brine.
Magnetic Nanoparticle Flooding: Use of magnetic nanoparticles with external control capability by application of magnetic field has been actively investigated for the following potential benefits: 1) additional oil recovery due to magnetic forces and microstructures of magnetic nanoparticles, 2) improved sweep efficiency due to magnetic field heterogeneity, 3) lowered oil viscosity due to electromagnetic heating, 4) direct rheology and interfacial tension change by magnetic forces at constant temperature, and 5) synergic EOR with polymer and removal and recycle of polymer and potentially other flooding agents from the produced fluid. In addition to working solely, magnetic nanoparticle flooding has the potential to work in synergy with other EOR methods, especially other nanoparticle aided EOR methods.
Author contributions
NW and YZ: literature review and writing, MP and MB: supervision and editing, CH: writing and editing.
Acknowledgments
We would like to thank the sponsors of the Chemical EOR Industrial Affiliate Program as well as Digital Rock Petrophysics Industrial Affiliate Program in the Center for Subsurface Energy and the Environment at the University of Texas at Austin for their support.
Conflict of interest
The authors declare that the research was conducted in the absence of any commercial or financial relationships that could be construed as a potential conflict of interest.
Publisher’s note
All claims expressed in this article are solely those of the authors and do not necessarily represent those of their affiliated organizations, or those of the publisher, the editors and the reviewers. Any product that may be evaluated in this article, or claim that may be made by its manufacturer, is not guaranteed or endorsed by the publisher.
References
Abernethy, E. R. (1976). Production increase of heavy oils by electromagnetic heating. J. Can. Petroleum Technol. 15 (03), 12. doi:10.2118/76-03-12
Adil, M., Lee, K., Zaid, H. M., Latiff, N. R. A., and Alnarabiji, M. S. (2018). Experimental study on electromagnetic-assisted ZnO nanofluid flooding for enhanced oil recovery (EOR). PLOS ONE 13 (2), e0193518. doi:10.1371/journal.pone.0193518
Adil, M., Mohd Zaid, H., and Kean Chuan, L. (2020). Electromagnetically-induced change in interfacial tension and contact angle of oil droplet using dielectric nanofluids. Fuel 259, 116274. doi:10.1016/j.fuel.2019.116274
Agi, A., Junin, R., and Gbadamosi, A. (2018). Mechanism governing nanoparticle flow behaviour in porous media: Insight for enhanced oil recovery applications. Int. Nano Lett. 8 (2), 49–77. doi:10.1007/s40089-018-0237-3
Agista, M. N., Guo, K., and Yu, Z. (2018). A state-of-the-art review of nanoparticles application in petroleum with a focus on enhanced oil recovery. Appl. Sci. 8 (6), 871. doi:10.3390/app8060871
Ali, H., Soleimani, H., Yahya, N., Khodapanah, L., Sabet, M., Demiral, B. M., et al. (2020). Enhanced oil recovery by using electromagnetic-assisted nanofluids: A review. J. Mol. Liq. 309, 113095. doi:10.1016/j.molliq.2020.113095
Ashtiani, M., Hashemabadi, S. H., and Ghaffari, A. (2015). A review on the magnetorheological fluid preparation and stabilization. J. Magnetism Magnetic Mater. 374, 716–730. doi:10.1016/j.jmmm.2014.09.020
Bagaria, H. G., Xue, Z., Neilson, B. M., Worthen, A. J., Yoon, K. Y., Nayak, S., et al. (2013). Iron oxide nanoparticles grafted with sulfonated copolymers are stable in concentrated brine at elevated temperatures and weakly adsorb on silica. ACS Appl. Mat. Interfaces 5 (8), 3329–3339. doi:10.1021/am4003974
Berg, J. C. (2010). An introduction to interfaces & colloids: The bridge to nanoscience. World Scientific.
Betancur, S., Olmos, C. M., Perez, M., Lerner, B., Franco, C. A., Riazi, M., et al. (2020). A microfluidic study to investigate the effect of magnetic iron core-carbon shell nanoparticles on displacement mechanisms of crude oil for chemical enhanced oil recovery. J. Petroleum Sci. Eng. 184, 106589. doi:10.1016/j.petrol.2019.106589
Caizer, C. (2015). “Nanoparticle size effect on some magnetic properties,” in Handbook of nanoparticles. Editor M. Aliofkhazraei (Cham: Springer International Publishing), 1–38. doi:10.1007/978-3-319-13188-7_24-1
Cao, J., Song, T., Zhu, Y., Wang, S., Wang, X., Lv, F., et al. (2018). Application of amino-functionalized nanosilica in improving the thermal stability of acrylamide-based polymer for enhanced oil recovery. Energy fuels. 32 (1), 246–254. doi:10.1021/acs.energyfuels.7b03053
Cao, J., Song, T., Zhu, Y., Wang, X., Wang, S., Yu, J., et al. (2018). Aqueous hybrids of amino-functionalized nanosilica and acrylamide-based polymer for enhanced oil recovery. RSC Adv. 8 (66), 38056–38064. doi:10.1039/c8ra07076h
Cao, J., Song, T., Wang, X., Zhu, Y., Wang, S., Zhao, M., et al. (2019). Studies on the rheological properties of amphiphilic nanosilica and a partially hydrolyzed polyacrylamide hybrid for enhanced oil recovery. Chem. Eng. Sci. 206, 146–155. doi:10.1016/j.ces.2019.05.034
Cheraghian, G. (2016). Effect of nano titanium dioxide on heavy oil recovery during polymer flooding. Petroleum Sci. Technol. 34 (7), 633–641. doi:10.1080/10916466.2016.1156125
Cheraghian, G., and Hendraningrat, L. (2016). A review on applications of nanotechnology in the enhanced oil recovery part B: Effects of nanoparticles on flooding. Int. Nano Lett. 6 (1), 1–10. doi:10.1007/s40089-015-0170-7
Cheraghian, G., and Hendraningrat, L. (2016). A review on applications of nanotechnology in the enhanced oil recovery part A: Effects of nanoparticles on interfacial tension. Int. Nano Lett. 6 (2), 129–138. doi:10.1007/s40089-015-0173-4
Cheraghian, G., Khalili Nezhad, S. S., Kamari, M., Hemmati, M., Masihi, M., and Bazgir, S. (2014). Adsorption polymer on reservoir rock and role of the nanoparticles, clay and SiO2. Int. Nano Lett. 4 (3), 114. doi:10.1007/s40089-014-0114-7
Chou, C.-K. (1990). Use of heating rate and specific absorption rate in the hyperthermia clinic. Int. J. Hyperth. 6 (2), 367–370. doi:10.3109/02656739009141144
Corredor, L. M., Husein, M. M., and Maini, B. B. (2019). A review of polymer nanohybrids for oil recovery. Adv. Colloid Interface Sci. 272, 102018. doi:10.1016/j.cis.2019.102018
Davidson, A., Huh, C., and Bryant, S. L. (2012). “Focused magnetic heating utilizing superparamagnetic nanoparticles for improved oil production applications,” in presented at the SPE International Oilfield Nanotechnology Conference and Exhibition, Noordwijk, The Netherlands, June 2012. doi:10.2118/157046-MS
de Vicente, J., Klingenberg, D. J., and Hidalgo-Alvarez, R. (2011). Magnetorheological fluids: A review. Soft Matter 7 (8), 3701–3710. doi:10.1039/C0SM01221A
Deng, Y. J., Dixon, J. B., White, G. N., Loeppert, R. H., and Juo, A. S. R. (2006). Bonding between polyacrylamide and smectite. Colloids Surfaces A Physicochem. Eng. Aspects 281 (1–3), 82–91. doi:10.1016/j.colsurfa.2006.02.030
Divandari, H., Hemmati-Sarapardeh, A., Schaffie, M., and Ranjbar, M. (2019). Integrating synthesized citric acid-coated magnetite nanoparticles with magnetic fields for enhanced oil recovery: Experimental study and mechanistic understanding. J. Petroleum Sci. Eng. 174, 425–436. doi:10.1016/j.petrol.2018.11.037
Divandari, H., Hemmati-Sarapardeh, A., Schaffie, M., Husein, M. M., and Ranjbar, M. (2021). Conformance control in oil reservoirs by citric acid-coated magnetite nanoparticles. ACS Omega 6 (13), 9001–9012. doi:10.1021/acsomega.1c00026
El-Diasty, A. I., and Aly, A. M., “Understanding the mechanism of nanoparticles applications in enhanced oil recovery,” SPE North Africa Technical Conference and Exhibition, Cairo, Egypt, September 2015, D021S009R004, Day 2 Tue September 15, 2015. doi:10.2118/175806-ms
El-hoshoudy, A. N., Desouky, S. E. M., Betiha, M. A., and Alsabagh, A. M. (2016). Use of 1-vinyl imidazole based surfmers for preparation of polyacrylamide–SiO2 nanocomposite through aza-Michael addition copolymerization reaction for rock wettability alteration. Fuel 170, 161–175. doi:10.1016/j.fuel.2015.12.036
Esmaeilnezhad, E., Van, S. L., Chon, B. H., Choi, H. J., Schaffie, M., Gholizadeh, M., et al. (2018). An experimental study on enhanced oil recovery utilizing nanoparticle ferrofluid through the application of a magnetic field. J. Industrial Eng. Chem. 58, 319–327. doi:10.1016/j.jiec.2017.09.044
Esmaeilnezhad, E., Choi, H. J., Schaffie, M., Gholizadeh, M., and Ranjbar, M. (2018). Polymer coated magnetite-based magnetorheological fluid and its potential clean procedure applications to oil production. J. Clean. Prod. 171, 45–56. doi:10.1016/j.jclepro.2017.10.004
Franco, C. A., Franco, C. A., Zabala, R. D., Bahamón, Í., Forero, Á., and Cortés, F. B. (2021). Field applications of nanotechnology in the oil and gas industry: Recent advances and perspectives. Energy fuels. 35 (23), 19266–19287. doi:10.1021/acs.energyfuels.1c02614
Gbadamosi, A. O., Junin, R., Manan, M. A., Yekeen, N., and Augustine, A. (2019). Hybrid suspension of polymer and nanoparticles for enhanced oil recovery. Polym. Bull. Berl. 76 (12), 6193–6230. doi:10.1007/s00289-019-02713-2
Ghosh, P., and Mohanty, K. K. (2020). Laboratory treatment of HPAM polymers for injection in low permeability carbonate reservoirs. J. Petroleum Sci. Eng. 185, 106574. doi:10.1016/j.petrol.2019.106574
Greff, J. H., and Babadagli, T. (2011). “Catalytic effects of nano-size metal ions in breaking asphaltene molecules during thermal recovery of heavy-oil,” in SPE Annual Technical Conference and Exhibition, Denver, Colorado, USA, October 2011. vol. All Days. doi:10.2118/146604-ms
Hassan, Y. M., Guan, B. H., Zaid, H. M., Hamza, M. F., Adil, M., Adam, A. A., et al. (2021). Application of magnetic and dielectric nanofluids for electromagnetic-assistance enhanced oil recovery: A review. Crystals 11 (2), 106. doi:10.3390/cryst11020106
Hendraningrat, L., and Torsæter, O. (2015). Metal oxide-based nanoparticles: Revealing their potential to enhance oil recovery in different wettability systems. Appl. Nanosci. 5 (2), 181–199. doi:10.1007/s13204-014-0305-6
Hendraningrat, L., and Torsæter, O. (2016). A study of water chemistry extends the benefits of using silica-based nanoparticles on enhanced oil recovery. Appl. Nanosci. 6 (1), 83–95. doi:10.1007/s13204-015-0411-0
Hu, Z., Haruna, M., Gao, H., Nourafkan, E., and Wen, D. (2017). Rheological properties of partially hydrolyzed polyacrylamide seeded by nanoparticles. Ind. Eng. Chem. Res. 56 (12), 3456–3463. doi:10.1021/acs.iecr.6b05036
Huang, T., Yao, J., Huang, Z., Yin, X., Xie, H., and Zhang, J. (2017). Numerical simulation on ferrofluid flow in fractured porous media based on discrete-fracture model. Open Phys. 15 (1), 370–378. doi:10.1515/phys-2017-0041
Huh, C., Panthi, K. K., Mohanty, K. K., and Bryant, S. L. (2015). Methods and compositions for conformance control using temperature-triggered polymer gel with magnetic nanoparticles. US20150159079A1 Accessed: Feb. 16, 2022. [Online]. Available: https://patents.google.com/patent/US20150159079A1/en.
Huh, C., Daigle, H., Prigiobbe, V., and Prodanovic, M. (2019). Practical Nanotechnology for petroleum engineers. New York, NY: CRC Press.
Ishida, S., Yang, Y., Meng, F., and Matsunaga, D. (2021). Field-controlling patterns of sheared ferrofluid droplets. arXiv:2112.13362 [cond-mat, physics:physics] Accessed: Jan. 06, 2022. [Online]. Available: http://arxiv.org/abs/2112.13362.
Joonaki, E., and Ghanaatian, S. (2014). The application of nanofluids for enhanced oil recovery: Effects on interfacial tension and coreflooding process. Petroleum Sci. Technol. 32 (21), 2599–2607. doi:10.1080/10916466.2013.855228
Jordan, A., Wust, P., Fählin, H., John, W., Hinz, A., and Felix, R. (1993). Inductive heating of ferrimagnetic particles and magnetic fluids: Physical evaluation of their potential for hyperthermia. Int. J. Hyperth. 9 (1), 51–68. doi:10.3109/02656739309061478
Jouenne, S., Levache, B., Joly, M., Hourcq, C., Questel, M., and Heurteux, G. (2019). “Universal viscosifying behavior of acrylamide-based polymers used in EOR - application for QA/QC, viscosity predictions and field characterization,” in Proceeding of the IOR 2019 – 20th European Symposium on Improved Oil Recovery, Apr 2019 (Pau, France: European Association of Geoscientists & Engineers), 1–23. doi:10.3997/2214-4609.20190014020191
Kazemzadeh, Y., Shojaei, S., Riazi, M., and Sharifi, M. (2019). Review on application of nanoparticles for EOR purposes: A critical review of the opportunities and challenges. Chin. J. Chem. Eng. 27 (2), 237–246. doi:10.1016/j.cjche.2018.05.022
Ko, S., Lee, H., and Huh, C. (2017). Efficient removal of enhanced-oil-recovery polymer from produced water with magnetic nanoparticles and regeneration/reuse of spent particles. SPE Prod. Operations 32 (03), 374–381. doi:10.2118/179576-PA
Kumar, D., Ganat, T., Lashari, N., Ayoub, M. A., Kalam, S., Chandio, T. A., et al. (2022). Experimental investigation of GO-HPAM and SiO2-HPAM composite for cEOR: Rheology, interfacial tension reduction, and wettability alteration. Colloids Surfaces A Physicochem. Eng. Aspects 637, 128189. doi:10.1016/j.colsurfa.2021.128189
Lakatos, I., Lakatos-Szabó, J., and Tóth, J. (1981). “Factors influencing polyacrylamide adsorption in porous media and their effect on flow behavior,” in Surface phenomena in enhanced oil recovery. Editor D. O. Shah (Boston, MA: Springer US), 821–842. doi:10.1007/978-1-4757-0337-5_37
Latiff, N. R. A., Yahya, N., Zaid, H. M., and Demiral, B. (2011). “Novel enhanced oil recovery method using dielectric zinc oxide nanoparticles activated by electromagnetic waves,” in Proceeding of the 2011 National Postgraduate Conference, Perak, Malaysia, September 2011 (IEEE), 1–7. doi:10.1109/NatPC.2011.6136450
Latiff, N. R. A., Soleimani, H., Zaid, H. M., and Adil, M. (2016). Magnetoviscous effect of ferrite-based magnetic fluid for EOR application. AIP Conf. Proc. 1787 (1), 050021. doi:10.1063/1.4968119
Leong, S. S., Ahmad, Z., Low, S. C., Camacho, J., Faraudo, J., and Lim, J. (2020). Unified view of magnetic nanoparticle separation under magnetophoresis. Langmuir 36 (28), 8033–8055. doi:10.1021/acs.langmuir.0c00839
Levitt, D., and Pope, G. A. (2008). Selection and screening of polymers for enhanced-oil recovery. Tulsa, Oklahoma: U.S.A., 1–18. doi:10.2118/113845-MS
Lohne, A., Nødland, O., Stavland, A., and Hiorth, A. (2017). A model for non-Newtonian flow in porous media at different flow regimes. Comput. Geosci. 21 (5), 1289–1312. doi:10.1007/s10596-017-9692-6
Maghzi, A., Kharrat, R., Mohebbi, A., and Ghazanfari, M. H. %J. F. (2014). The impact of silica nanoparticles on the performance of polymer solution in presence of salts in polymer flooding for heavy oil recovery. Fuel (Lond). 123, 123–132. doi:10.1016/j.fuel.2014.01.017
Mather, P. T., Luo, X., and Rousseau, I. A. (2009). Shape memory polymer research. Annu. Rev. Mat. Res. 39 (1), 445–471. doi:10.1146/annurev-matsci-082908-145419
Meyer, E. E., Rosenberg, K. J., and Israelachvili, J. (2006). Recent progress in understanding hydrophobic interactions. Proc. Natl. Acad. Sci. U. S. A. 103 (43), 15739–15746. doi:10.1073/pnas.0606422103
Mohanty, U. S., Awan, F. U. R., Ali, M., Aftab, A., Keshavarz, A., and Iglauer, S. (2021). Physicochemical characterization of zirconia nanoparticle-based sodium alginate polymer suspension for enhanced oil recovery. Energy fuels. 35 (23), 19389–19398. doi:10.1021/acs.energyfuels.1c02724
Panchal, H., Patel, H., Patel, J., and Shah, M. (2021). A systematic review on nanotechnology in enhanced oil recovery. Petroleum Res. 6 (3), 204–212. doi:10.1016/j.ptlrs.2021.03.003
Panthi, K., Mohanty, K. K., and Huh, C. (2015). “Precision control of gel formation using superparamagnetic nanoparticle-based heating,” in presented at the SPE Annual Technical Conference and Exhibition, Houston, Texas, USA, September 2015. doi:10.2118/175006-MS
Phenrat, T., Cihan, A., Kim, H.-J., Mital, M., Illangasekare, T., and Lowry, G. V. (2010). Transport and deposition of polymer-modified Fe0 nanoparticles in 2-D heterogeneous porous media: Effects of particle concentration, Fe0 content, and coatings. Environ. Sci. Technol. 44 (23), 9086–9093. doi:10.1021/es102398e
Phenrat, T., Thongboot, T., and Lowry, G. V. (2016). Electromagnetic induction of zerovalent iron (ZVI) powder and nanoscale zerovalent iron (NZVI) particles enhances dechlorination of trichloroethylene in contaminated groundwater and soil: Proof of concept. Environ. Sci. Technol. 50 (2), 872–880. doi:10.1021/acs.est.5b04485
Prodanovic, M., Ryoo, S., Rahmani, A. R., Kuranov, R., Kotsmar, C., Milner, T. E., et al. (2010). “Effects of magnetic field on the motion of multiphase fluids containing paramagnetic nanoparticles in porous media,” in presented at the SPE Improved Oil Recovery Symposium, Tulsa, Oklahoma, USA, April 2010. doi:10.2118/129850-MS
Quigley, T. M., and Mackenzie, A. S. (1988). The temperatures of oil and gas formation in the sub-surface. Nature 333 (6173), 549–552. doi:10.1038/333549a0
Robbes, A.-S., Cousin, F., Meneau, F., Dalmas, F., Boué, F., and Jestin, J. (2011). Nanocomposite materials with controlled anisotropic reinforcement triggered by magnetic self-assembly. Macromolecules 44 (22), 8858–8865. doi:10.1021/ma201096u
Sabzi Dizajyekan, B., Jafari, A., Hasani, M., Vafaei-Sefti, M., Fakhroueian, Z., and Baghbansalehi, M. (2020). Surface modification of synthesized Fe3O4 super-paramagnetic nanoparticles and performance investigation in gelation parameters enhancement: Application in enhanced oil recovery. Appl. Nanosci. 10 (3), 955–969. doi:10.1007/s13204-019-01187-y
Saint-Martin de Abreu Soares, F. (2015). A pore scale study of ferrofluid-driven mobilization of oil,” Thesis. Austin, TX, USA: The University of Texas at Austin. doi:10.15781/T2RP7F
Samavati, A., Velashjerdi, M., Ismail, A. F., Othman, M., Eisaabadi B., G., Awang, A., et al. (2021). Continuous monitoring of crude oil movement in an electromagnetic-assisted enhanced oil recovery process using a modified fiber Bragg grating sensor. Sensors Actuators A Phys. 318, 112428. doi:10.1016/j.sna.2020.112428
Sharma, T., Iglauer, S., and Sangwai, J. S. (2016). Silica nanofluids in an oilfield polymer polyacrylamide: Interfacial properties, wettability alteration, and applications for chemical enhanced oil recovery. Ind. Eng. Chem. Res. 55 (48), 12387–12397. doi:10.1021/acs.iecr.6b03299
Silva, I. P. G., Aguiar, A. A., Rezende, V. P., Monsores, A. L. M., and Lucas, E. F. (2018). A polymer flooding mechanism for mature oil fields: Laboratory measurements and field results interpretation. J. Petroleum Sci. Eng. 161, 468–475. doi:10.1016/j.petrol.2017.12.008
Simonsen, G., Strand, M., and Øye, G. (2018). Potential applications of magnetic nanoparticles within separation in the petroleum industry. J. Petroleum Sci. Eng. 165, 488–495. doi:10.1016/j.petrol.2018.02.048
Sirk, K. M., Saleh, N. B., Phenrat, T., Kim, H. J., Dufour, B., Ok, J., et al. (2009). Effect of adsorbed polyelectrolytes on nanoscale zero valent iron particle attachment to soil surface models. Environ. Sci. Technol. 43 (10), 3803–3808. doi:10.1021/es803589t
Soares, P. I. P., Alves, A. M., Pereira, L. C., Coutinho, J. T., Ferreira, I. M., Novo, C. M., et al. (2014). Effects of surfactants on the magnetic properties of iron oxide colloids. J. Colloid Interface Sci. 419, 46–51. doi:10.1016/j.jcis.2013.12.045
Soleimani, H., Ahmad Latiff, N. R., Yahya, N., Zaid, H. M., Sabet, M., Guan, B. H., et al. (2014). Effect of annealing temperature on the crystallization of hematite-alumina (Fe2O3-Al2O3) nanocomposite and its influence in EOR application. J. Nano Res. 29, 105–113. doi:10.4028/www.scientific.net/JNanoR.29.105
Tabatabaei, M., Taleghani, A. D., Li, G., and Zhang, T. (2021). Shape memory polymers as lost circulation materials for sealing wide-opened natural fractures. SPE Drill. Complet. 36 (04), 931–942. doi:10.2118/205514-PA
Tracy, J. B., and Crawford, T. M. (2013). Magnetic field-directed self-assembly of magnetic nanoparticles. MRS Bull. 38 (11), 915–920. doi:10.1557/mrs.2013.233
Wang, N. (2021). Ferrofluid applications in petroleum engineering,” Thesis. Austin, TX, USA: The University of Texas at Austin.
Wang, N., Liu, Y., Cha, L., Prodanovic, M., and Balhoff, M. (2020). “Microfluidic and numerical investigation of trapped oil mobilization with hydrophilic magnetic nanoparticles,” in presented at the SPE Annual Technical Conference and Exhibition, Virtual, October 2020. doi:10.2118/201365-MS
Wang, N., Liu, Y., Cha, L., Balhoff, M. T., and Prodanovic, M. (2021). Experimental investigation of trapped oil mobilization with ferrofluid. SPE J. 27, 753–770. doi:10.2118/201365-PA
Wang, N., and Prodanovic, M. (2017). “A three-dimensional pore-scale model for non-wetting phase mobilization with ferrofluid,”. AGU fall meeting abstracts, 13. [Online]. Available: http://adsabs.harvard.edu/abs/2017AGUFM.H13E1430W.
Xu, K., Zhu, P., Colon, T., Huh, C., and Balhoff, M. (2017). A microfluidic investigation of the synergistic effect of nanoparticles and surfactants in macro-emulsion-based enhanced oil recovery. SPE J. 22 (02), 459–469. doi:10.2118/179691-PA
Xu, Z.-X., Li, S.-Y., Li, B.-F., Chen, D.-Q., Liu, Z.-Y., and Li, Z.-M. (2020). A review of development methods and EOR technologies for carbonate reservoirs. Pet. Sci. 17 (4), 990–1013. doi:10.1007/s12182-020-00467-5
Yahya, N., Kashif, M., Nasir, N., Niaz Akhtar, M., and Yusof, N. M. (2012). Cobalt ferrite nanoparticles: An innovative approach for enhanced oil recovery application. JNanoR. 17, 115–126. doi:10.4028/www.scientific.net/JNanoR.17.115
Yahya, N., Kashif, M., Shafie, A., Soleimani, H., Zaid, H. M., and Latiff, N. R. A. (2014). Improved oil recovery by high magnetic flux density subjected to iron oxide nanofluids. JNanoR. 26, 89–99. doi:10.4028/www.scientific.net/JNanoR.26.89
Zaid, H. M., Yahya, N., and Latiff, N. R. A. (2013). The effect of nanoparticles crystallite size on the recovery efficiency in dielectric nanofluid flooding. J. Nano Res. 21, 103–108. doi:10.4028/www.scientific.net/JNanoR.21.103 accessed Jan. 06, 2019)
Zheng, C., Cheng, Y., Wei, Q., Li, X., and Zhang, Z. (2017). Suspension of surface-modified nano-SiO2 in partially hydrolyzed aqueous solution of polyacrylamide for enhanced oil recovery. Colloids Surfaces A Physicochem. Eng. Aspects 524, 169–177. doi:10.1016/j.colsurfa.2017.04.026
Zhou, M., Zou, J., Gu, Y., Yi, R., and Tu, H. (2020). Preparation of magnetic polymer nanosphere and its profile control. J. Dispersion Sci. Technol. 41 (4), 557–565. doi:10.1080/01932691.2019.1593860
Keywords: nanoparticle, enhanced oil recovery, polymer, magnetic nanoparticle, subsurface engineering, porous media
Citation: Wang N, Zhao Y, Prodanović M, Balhoff MT and Huh C (2022) 12012 fundamental mechanisms behind nanotechnology applications in oil and gas: Emerging nano-EOR processes. Front. Nanotechnol. 4:887715. doi: 10.3389/fnano.2022.887715
Received: 01 March 2022; Accepted: 20 July 2022;
Published: 26 August 2022.
Edited by:
Wei Wang, Aramco Services Company, United StatesReviewed by:
Tanapon Phenrat, Naresuan University, ThailandSubhasis Roy, University of Calcutta, India
Copyright © 2022 Wang, Zhao, Prodanović, Balhoff and Huh. This is an open-access article distributed under the terms of the Creative Commons Attribution License (CC BY). The use, distribution or reproduction in other forums is permitted, provided the original author(s) and the copyright owner(s) are credited and that the original publication in this journal is cited, in accordance with accepted academic practice. No use, distribution or reproduction is permitted which does not comply with these terms.
*Correspondence: Ningyu Wang, bmluZ3l1d0B1dGV4YXMuZWR1; Matthew T. Balhoff, YmFsaG9mZkBtYWlsLnV0ZXhhcy5lZHU=