- 1Sophisticated Analytical Instrumentation Facility (SAIF)/CIL, Panjab University Chandigarh, Chandigarh, India
- 2Department of Chemistry and Centre of Advanced Studies in Chemistry, Panjab University Chandigarh, Chandigarh, India
- 3E-YUVA Centre, Panjab University, Chandigarh, India
Microbial infection and antibiotic resistance is recognized as a serious problem to society from both an economical perspective and a health concern. To tackle this problem, “nanotechnology,” a multidisciplinary field of research, has provided a plethora of nanomaterials for potential applications in the antimicrobial sector. This letter discusses how antimicrobial nanomaterials are shaping this challenging field and being evaluated as therapeutic and medication delivery agents. The recently designed smart antimicrobial surfaces with switchable features that displayed synergistic antibacterial action were also highlighted. To end, we provide the current scenario and future perspectives with regards to emerging antimicrobial nano-engineered materials and nanotechnology.
Introduction
In human history, various pathogenic microorganisms such as bacteria, viruses, protozoa, and fungi have been the leading cause of morbidity and mortality around the globe (Luo and Gao, 2020). To combat with these pathogen related infections, many generations of antibiotics have been developed. However, the indiscriminate and excessive usage of antibiotics has accelerated the advent of antibiotic-resistant microbes, putting a substantial strain on the public healthcare system and communities. In fact, most of antibiotics are losing their effectiveness against the drug-resistant bacteria. Moreover, the situation is further exacerbated by the withdrawal of biopharmaceutical industries from exploring new antibiotics due to economic and regulatory obstacles (Gao and Zhang, 2021). This slowed progression of effective medicine may revert us to the pre-antibiotic era, when microbial infections were potentially fatal. For these reasons, there is a critical need for the design and development of new antimicrobial technologies that can be used as an alternatives or in conjunction with conventional antimicrobial treatments (Kaushik, 2019).
Over the last few decades, nanotechnology has been seminal in the field of biomedical research and emerged as a new solution to unmet the medical needs. The advancement in nanotechnology has brought the fabrication of nano-sized materials with high surface-to-volume ratios. A broad range of nanomaterials such as carbon and metal-based nanomaterials, nanocomposites, dendrimers, polymers, nanoemulsions, micelles, liposomes, etc. have been shown to exhibit a remarkable antimicrobial properties (Chauhan et al., 2019; Kaur et al., 2019; Garg et al., 2020; Garg et al., 2021; Makabenta et al., 2021; Singh et al., 2022). They are considered more capable as compared to their micro/macroscale counterparts in terms of inhibiting microbial growth (Zare et al., 2015). Nanomaterials, as an antimicrobial therapeutic, provide distinct benefits over traditional antibiotics in terms of countering microbial resistance mechanisms (Liu et al., 2019; Tiwari et al., 2021). Similarly, nanomaterials have shown remarkable potential in the delivery of drugs to specific target sites in the body. Moreover, nanomaterials can adapt various bactericidal trials which make them less prone to microbial resistance than conventional antibiotics. Besides antimicrobial nanomaterials are nascent as nanomedicine that has broad impact in the biomedical applications including targeting, imaging, therapy, etc.
Antimicrobial Nanomaterials as an Alternative
Nanomaterials access antimicrobial modalities that are novel to microbes and thus are not in their natural defensive arsenal. With their high-speed developments, nanomaterials have now been identified as nontraditional antimicrobial agents for tackling the microbial resistant to traditional antibiotic drugs. The size and high surface-to-volume ratios of various nanomaterials are likely to allow for multivalent interactions with microbial biomolecules that can be regulated by surface functionalization. The shape of nanomaterials can influence their intrinsic properties like plasmonic, photocatalytic and magnetic properties, also resonates the antimicrobial activity against the microbes (Dhau et al., 2021; Kush et al., 2021). With the rapid advancement in nanofabrication techniques, a diverse array of nanostructures have been designed for instance nanodots, nanoparticles, nanosheets, nanocubes, nanodumbells, nanorods, nanowires, nanocones, nanoshells, nanoneedles, nanocages, nanoflakes, nanoflowers, nanoplates and so on (Chauhan et al., 2020; Cheeseman et al., 2020; Chakraborty et al., 2021).
As an antimicrobial therapeutic agent, nanomaterials are increasingly being touted as new line of defense to combat microbes. Many antibiotic resistance mechanisms have been reported in microbes, including changes in membrane permeability, modification of the antimicrobial drug receptor, over-expression of specific efflux pumps, antibiotic degradation, and antibiotic target site modification (Dhau et al., 2014). The fast evolution of microbial antibiotic resistance has been accelerated by the life-cycle of the microbes. Nanomaterials have the ability to carry out multiple antimicrobial actions (i.e. cellular membrane disintegration, ROS formation, ATP depletion, and damage to intracellular biomolecules such as nucleic acid, proteins, ribosomes, enzymes etc.) at the same time (Figure 1). The utilization of these multiple mechanisms makes the resistance development to nanomaterials difficult since multiple gene mutations would be required simultaneously in the same bacterial cell for acquiring resistance (Pelgrift and Friedman, 2013). For instance, Yuan et al. studied the high antibacterial efficacy of synthesized Silver (Ag) nanoparticles (NPs) against P. aeruginosa and S. aureus bacterial cells, extracted from milk samples produced by mastitis-infected goats. Results demonstrated that the antibacterial activity of Ag NPs were due to the production of malondialdehyde (MDA), reactive oxygen species (ROS) and proteins leakage in the bacterial cells. In addition, it was observed that Ag NP-treated cells had significantly lower lactate dehydrogenase activity (LDH) and adenosine triphosphate (ATP) levels compared to the standard. These physiological and biochemical measurements were suggesting that Ag NPs can induce bacterial cell death by different mechanisms (Yuan et al., 2017). Likely, Zanni et al. created a unique nanomaterial (ZnGs) made up of zinc oxide nanorods (ZnO-NRs) and graphene nanoplatelets (GNP) that kill S. mutans in both planktonic and biofilm forms. As shown in Figure 2A, the killing mechanism may be caused to the mechanical damage produced by the ZnO-NRs. The increased lateral size of the GNPs with growth orientation of ZnO-NRs helps to increase the nanostructures adherence to the cell wall and the penetration of the ZnO-NRs through the cell membrane, resulting in more puncturing and damage to the bacterial surface (Zanni et al., 2016).
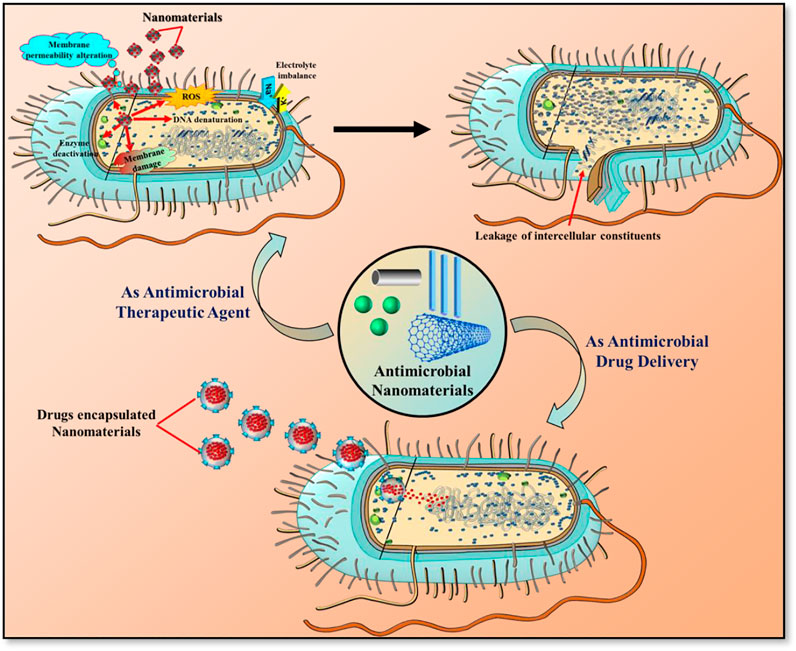
FIGURE 1. Potential applications of antimicrobial nanomaterials as therapeutic agent and drug delivery carrier.
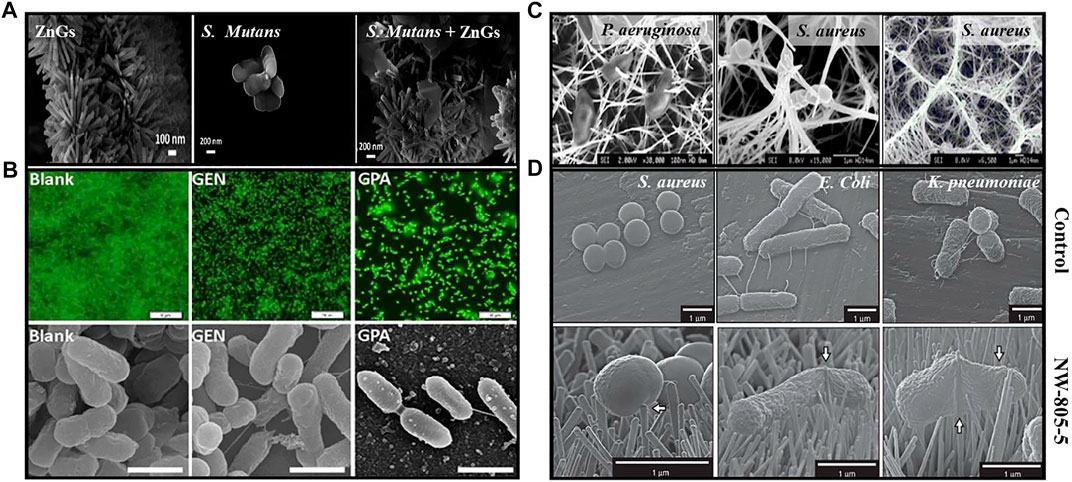
FIGURE 2. (A) FE-SEM images of ZnGs, pure S. mutans and S. mutans in the presence of ZnGs (Zanni et al., 2016); (B) Confocal and FE-SEM images of P. aeruginosa biofilm (as blank), with gentamicin (GEN) and with Au NPs loaded gentamicin drug (GPA) (Mu et al., 2016); (C) FE-SEM images of Nanowire-pierced bacterial cells after 1h incubations (Diu et al., 2014); (D) FE-SEM images of Bacteria following 3-h static incubation on flat titanium alloy (control) and TiO2 nanopillar surface (NW-805–5) (Jenkins et al., 2020).
Nanomaterials can be utilized not just to overcome microbial resistance, but also as a “medium and carrier” for drugs (Figure 1). Average sized drug have less influence on the intracellular microbe due to the poor membrane transport of antibiotics. Moreover, non-specific delivery of drug at the infection areas usually leads to overuse and evolution of drug-resistant bacteria. These challenges can be addressed by projecting nanomaterials as drug-carrying mediators to the infected areas. Nanomaterials can minimize the systemic side effects, premature drug release and entail the active or passive targeting of drugs to an infection site. Recently, Park et al. used the gold (Au) NPs-DNA conjugates loaded with antimicrobial peptides Lys AB2 P3-His to inhibit the growth of A. baumannii infection in mice. It was observed that the colonization of bacterium was reduced in the mouse organs, leading to enhanced the survival rate and time of the mice as compared to the mice treated with Au NP-DNA or Lys AB2 P3-His only (Park et al., 2022). Likewise, Yaqub et al. have reported the more pronounced antimicrobial activity of copper (Cu) NPs conjugated with doxycycline as an compared to Cu NPs alone against P. aeruginosa, although similar to those with the drug alone (Yaqub et al., 2020). Mu et al. fabricated the phosphatidylcholine-decorated Au NPs loaded with gentamicin drug and reported a superior antibiotic activities against established biofilms as well as further inhibiting the biofilm formation of pathogens including Gram-positive and Gram-negative bacteria (Mu et al., 2016). Moreover, it was found that they had good biocompatibility and higher killing capability against multiple pathogenic bacteria in infected macrophages than gentamicin did alone as visualized in confocal microscopy and FE-SEM images (Figure 2B).
A plausibly more effective nanomaterial-based delivery system involves a stimuli-responsive strategy that responds to the distinct micro-environment of the infection site, allowing the release of drugs in a spatio-temporally controlled manner (Canaparo et al., 2019). Generally, the microenvironment around the microbial infection sites has been proven to be markedly different from that of normal tissue. Thus many endogenous stimuli factors such as enzymes, lower pH, hydrogen peroxide and release toxins, etc. have been employed for responsive drug release to the microbial infection regions (Figure 3). The acidic metabolic products released from bacteria can lead to increase in the local acidity i.e. pH lower than 7.4. Radovic-Moreno et al. developed a pH-responsive and surface charge-switching vancomycin encapsulated poly (d,l-lactic-co-glycolic acid)-b-poly (l-histidine)-b-poly (ethylene glycol) NPs for the treatment of S. aureus bacterial infection. The results suggested that at pH 6, the NPs avidly bind to bacteria and delivered the vancomycin to the infection sites as compared to pH 7.4 (Radovic-Moreno et al., 2012). Likewise, the elevated H2O2 level at the microbial infection site can be exploited to build H2O2-responsive drug delivery systems. Along with these stimulus factors produced in vivo, exogenous stimuli-responsive nanomaterials can also be designed for controlled drug release by using a series of external triggers such as heat, light, microwave, ultrasound, electric and magnetic fields, etc. These artificially controllable stimuli provide a remarkably different perspective for designing nanocarriers and making them effective for combating biofilm infections (Ding et al., 2021).
The ability of bacteria to persist on surfaces, both in healthcare facilities and on common public surfaces, is one of the major areas of concern in the transmission of infectious diseases. Since most of the serious infections such as COVID-19 are facilitated by the high touch surfaces and microbial colonization of surfaces (Dubey et al., 2022). As the nanotechnology continually advances, various innovative approaches have focused on the designing of nanomaterial surfaces which can prevent the microbial transmission and biofilm formation on the surfaces by killing or inhibiting attachment of microorganisms (Imani et al., 2020). Over the years, the ‘race to the surface’ concept has been applied to define the contest between host cells and pathogenic microbes to inhabit the biomaterial surfaces. In these concern, the coating of antimicrobial nanomaterials on the implant surfaces have become well-established approach to inhibit the growth of bacteria and prevents microbial colonization around the implants. These biocidal surfaces, often termed as ‘release-based coatings,’ slowly release chemicals into the immediate surroundings of the implant materials, preventing microbial infection. However, uncontrolled release of the antibiotic from these in-situ drug releasing materials may sometimes result into cytotoxicity, inflammation responses and the evolution of microbial resistance.
The micro/nano-structuring of surfaces have provided an interesting alternative to widely used approaches for constraining microbial adherence or for killing microbes via cell-surface interaction (Figure 4). These hierarchical nanopatterned surfaces were initially inspired by natural biomaterials, such as lotus leaves, gecko feet and insect wings that are known to have variety of functions including super-hydrophobicity, ultra-low/high adhesion, self-cleaning and bio-fouling (Xu et al., 2021). The physical interaction between the microbial cell wall and the nano-engineered surface drives the mechano-bactericidal activity of these surfaces (Linklater et al., 2021). To date, a wide range of nanofabrication techniques has been employed to create bactericidal nanopatterned surfaces on black silicon, carbon nanotubes, graphene, polymers and titanium, indicating that these materials could be used as future biomaterials (Bhardwaj et al., 2021). For instance, Diu et al. prepared two titanium based nanostructured surfaces i.e. persistent nanowire coverage (nano-brush) and isolated nano-wire pockets (nano niche) by hydrothermal process on a flat titania surface. These titania nano-patterns have a relatively low aspect ratio (<2) and more order of magnitude taller than nanopillers. The results indicated that these nanostructured titania surfaces have selective bactericidal activity against P. aeruginosa bacterial cells and less activity for S. aureus (Figure 2C). Moreover, it is clear that P. aeruginosa was more resistant to nanostructured surfaces at passive, static incubation conditions than incubation with agitation, dynamic condition (Diu et al., 2014). Jenkins et al. have generated the titania nanopillars on titanium alloy (grade 5) by using thermal oxidation technique which mimics the nanoprotrusions found on dragonfly wings. This study observed that titania nanopillar induced cell impedance which is expected to reduce the capacity of bacteria to replicate on nanopillar surfaces (Figure 2D), and thus enhance the anti-biofilm properties of nanopillar surfaces (Jenkins et al., 2020).
In recent years, a new class of smart antimicrobial surfaces with multiple functionalities have been developed with switchable properties that can kill and inhibit the microbes, simultaneously. Such antifouling surfaces can be designed by incorporating microbicides and stimuli-responsive materials. Similarly, the smart surfaces with the ability to switch between two distinct functions i.e. switchable bacteria-killing and bacteria-releasing capabilities have been developed with promising potential for use in the biomedical field. Likely, Wei et al. have prepared the smart antibacterial surfaces composed of azobenzene (Azo) groups as a photoswitchable platform and β-cyclodextrin derivative containing seven quaternary ammonium salt (QAS) groups, as a biocide. These surfaces were reported to achieve a strong bactericidal effect that can kill more than 90% of attached bacteria. On irradiation with UV light, the Azo groups switched to cis form, resulting in the dissociation of the Azo/CD-QAS inclusion complex and release of dead bacteria from the surface. (Wei et al., 2017).
Future Perspectives
In the current state of art, biomedical application of antimicrobial nanomaterials is currently primarily at the academic research level, with limited translation into the clinical stage. They are emerging ‘outside of the box’ approach to combat pathogenic microbes and recalcitrant antibiotic resistance. Their tunability and ability to interact multivalently maximize their therapeutic effect and minimize toxicity. In addition, their coating on medical devices has drastically reduced the surface-associated microbial infection and biofilm formation. Moreover, the emergence of stimuli-activated antimicrobial nanomaterials is an attractive alternative for replacing or supplementing current treatment techniques. Their potential impact for the detection of bacterial infection, antibiotics delivery and the development of medical devices with antimicrobial coatings has already been demonstrated by the clinical approval.
Various advocacy groups state that antimicrobial nanomaterials are quite benign but still, there is doubt about the impact of antimicrobial nanomaterials on the environment, health and safety. Even after 6 decades, this doubt has not been reduced because there is no specific mechanism of nanomaterials have been addressed which causes cell death. In addition, there are still many controversies regarding the metal toxicity and secondary pollution generated by antimicrobial nanomaterials that will contaminate water resources and could interact with humans, animals, and the environment. Future perspectives should be focused on the optimization of antimicrobial efficacies and make checklists to determine if nanomaterials pose adverse health effects or environmental damages. More efforts are necessary to design metal-free facile recyclable antibacterial nanomaterials in the future. It is worth noting that the majority of antimicrobial research conducted against the Gram-positive and -negative bacteria. In future, more research should be performed on the disinfection activities of viruses, fungus, and even parasites with these antimicrobial nanomaterials.
While several recent studies have advanced the field of drug delivery, the primary limits for stimuli-responsive antimicrobial drug carrier are the ability to penetrate deeper layers of tissue and avoid unwanted tissue damage. In addition, the antimicrobial drug carrier are mainly impacted by in vivo factors. Therefore, we need to invest more efforts for studying the effect of drug carriers on the normal activities of the human body and achieving accurate stimuli response for the drug release time, locations and drug concentration.
Despite the anticipated potential of antimicrobial nanomaterials in the medical field, there are several bottlenecks still exist to achieve clinical translation. There are no clinically viable antibacterial nanoformulations, such as vaccinations for treating pandemic infectious diseases or as antimicrobial drug (Makvandi et al., 2020). These technologies are still in their early stages, and future research will necessitate systematic studies and in vivo trials to better optimize and analyze the “real world” uses of such therapeutics (Cheeseman et al., 2020). We believe nanomaterials based therapeutic strategies will foster the antimicrobial technologies on the next level if future research should be more comprehensively engrossed on the antimicrobial nanoformulations which address the particular gaps in the literature.
Data Availability Statement
The original contributions presented in the study are included in the article/Supplementary Material, further inquiries can be directed to the corresponding authors.
Author Contributions
GC and MC devised the concept. The manuscript was jointly drafted by PG and MC. PA contributed to the preparation of the images. GC and RS contributed to the supervision and final revision of the work. All authors gave final approval for publication.
Conflict of Interest
The authors declare that the research was conducted in the absence of any commercial or financial relationships that could be construed as a potential conflict of interest.
Publisher’s Note
All claims expressed in this article are solely those of the authors and do not necessarily represent those of their affiliated organizations, or those of the publisher, the editors and the reviewers. Any product that may be evaluated in this article, or claim that may be made by its manufacturer, is not guaranteed or endorsed by the publisher.
Acknowledgments
GRC would like to acknowledge the support of UGC, India under INDO-US 21st Century knowledge Initiative project [F.No. 194-2/2016 (IC)]. MC is thankful to BIRAC (Biotechnology Industry Research Assistance Council) for financial support in the form of Postdoctoral Fellowship [Ref. No. 4073/GP].
References
Bhardwaj, S. K., Mujawar, M., Mishra, Y. K., Hickman, N., Chavali, M., and Kaushik, A. (2021). Bio-inspired Graphene-Based Nano-Systems for Biomedical Applications. Nanotechnology 32, 502001. doi:10.1088/1361-6528/ac1bdb
Canaparo, R., Foglietta, F., Giuntini, F., Della Pepa, C., Dosio, F., and Serpe, L. (2019). Recent Developments in Antibacterial Therapy: Focus on Stimuli-Responsive Drug-Delivery Systems and Therapeutic Nanoparticles. Molecules 24 (10), 1991. doi:10.3390/molecules24101991
Chakraborty, U., Bhanjana, G., Kannu, N., Kaur, N., Sharma, R., Kaur, G., et al. (2021). Microwave-assisted Assembly of Ag2O-ZnO Composite Nanocones for Electrochemical Detection of 4-Nitrophenol and Assessment of Their Photocatalytic Activity towards Degradation of 4-Nitrophenol and Methylene Blue Dye. J. Hazard. Mater. 416, 125771. doi:10.1016/j.jhazmat.2021.125771
Chauhan, M., Jasrotia, T., Kaur, G., Prakash, C., Kumar, R., Dilbaghi, N., et al. (2020). Investigating the Efficiency of α-Bismuth Zinc Oxide Heterostructure Composite/UV-LED in Methylene Blue Dye Removal and Evaluation of its Antimicrobial Activity. Environ. Res. 180, 108857. doi:10.1016/j.envres.2019.108857
Chauhan, M., Sharma, B., Kumar, R., Chaudhary, G. R., Hassan, A. A., and Kumar, S. (2019). Green Synthesis of CuO Nanomaterials and Their Proficient Use for Organic Waste Removal and Antimicrobial Application. Environ. Res. 168, 85–95. doi:10.1016/j.envres.2018.09.024
Cheeseman, S., Christofferson, A. J., Kariuki, R., Cozzolino, D., Daeneke, T., Crawford, R. J., et al. (2020). Antimicrobial Metal Nanomaterials: From Passive to Stimuli‐Activated Applications. Adv. Sci. 7, 1902913. doi:10.1002/advs.201902913
Dhau, J. S., Singh, A., Brandão, P., and Felix, V. (2021). Synthesis, Characterization, X-ray crystal Structure and Antibacterial Activity of Bis [3-(4-Chloro-N, N-Diethylpyridine-2-Carboxamide)] Diselenide. Inorg. Chem. Commun. 133, 108942. doi:10.1016/j.inoche.2021.108942
Dhau, J. S., Singh, A., Singh, A., Sooch, B. S., Brandão, P., and Félix, V. (2014). Synthesis and Antibacterial Activity of Pyridylselenium Compounds: Self-Assembly of Bis (3-Bromo-2-Pyridyl) Diselenide via Intermolecular Secondary and π⋯ π Stacking Interactions. J. Organomet. Chem. 766, 57–66. doi:10.1016/j.jorganchem.2014.05.009
Ding, M., Zhao, W., Song, L. J., and Luan, S. F. (2021). Stimuli-responsive Nanocarriers for Bacterial Biofilm Treatment. Rare Met. 4, 1–17. doi:10.1007/s12598-021-01802-4
Diu, T., Faruqui, N., Sjöström, T., Lamarre, B., Jenkinson, H. F., Su, B., et al. (2014). Cicada-inspired Cell-Instructive Nanopatterned Arrays. Sci. Rep. 4, 7122–7156. doi:10.1038/srep07122
Dubey, A. K., Chaudhry, S. K., Singh, H. B., Gupta, V. K., and Kaushik, A. (2022). Perspectives on Nano-Nutraceuticals to Manage Pre and post COVID-19 Infections. Biotechnol. Rep. 33, e00712. doi:10.1016/j.btre.2022.e00712
Gao, W., and Zhang, L. (2021). Nanomaterials Arising amid Antibiotic Resistance. Nat. Rev. Microbiol. 19, 5–6. doi:10.1038/s41579-020-00469-5
Garg, P., Kaur, B., Kaur, G., Saini, S., and Chaudhary, G. R. (2021). A Study of the Spectral Behaviour of Eosin Dye in Three States of Metallosurfactants: Monomeric, Micelles and Metallosomes. Colloids Surf. A: Physicochemical Eng. Aspects 610, 125697. doi:10.1016/j.colsurfa.2020.125697
Garg, P., Kaur, G., Sharma, B., and Chaudhary, G. R. (2020). Fluorescein-Metal Hybrid Surfactant Conjugates as a Smart Material for Antimicrobial Photodynamic Therapy against Staphylococcus aureus. ACS Appl. Bio Mater. 3, 4674–4683. doi:10.1021/acsabm.0c00586
Imani, S. M., Ladouceur, L., Marshall, T., Maclachlan, R., Soleymani, L., and Didar, T. F. (2020). Antimicrobial Nanomaterials and Coatings: Current Mechanisms and Future Perspectives to Control the Spread of Viruses Including SARS-CoV-2. ACS nano 14, 12341–12369. doi:10.1021/acsnano.0c05937
Jenkins, J., Mantell, J., Neal, C., Gholinia, A., Verkade, P., Nobbs, A. H., et al. (2020). Antibacterial Effects of Nanopillar Surfaces Are Mediated by Cell Impedance, Penetration and Induction of Oxidative Stress. Nat. Commun. 11, 1626. doi:10.1038/s41467-020-15471-x
Kaur, G., Garg, P., Kaur, B., Chaudhary, G. R., Kumar, S., Dilbaghi, N., et al. (2019). Synthesis, thermal and Surface Activity of Cationic Single Chain Metal Hybrid Surfactants and Their Interaction with Microbes and Proteins. Soft matter 15, 2348–2358. doi:10.1039/c9sm00046a
Kaushik, A. (2019). Biomedical Nanotechnology Related Grand Challenges and Perspectives. Front. Nanotechnol. 1, 1. doi:10.3389/fnano.2019.00001
Kush, P., Kumar, P., Singh, R., and Kaushik, A. (2021). Aspects of High-Performance and Bio-Acceptable Magnetic Nanoparticles for Biomedical Application. Asian J. Pharm. Sci. 16, 704–737. doi:10.1016/j.ajps.2021.05.005
Linklater, D. P., Baulin, V. A., Juodkazis, S., Crawford, R. J., Stoodley, P., and Ivanova, E. P. (2021). Mechano-bactericidal Actions of Nanostructured Surfaces. Nat. Rev. Microbiol. 19, 8–22. doi:10.1038/s41579-020-0414-z
Liu, Y., Shi, L., Su, L., van der Mei, H. C., Jutte, P. C., Ren, Y., et al. (2019). Nanotechnology-based Antimicrobials and Delivery Systems for Biofilm-Infection Control. Chem. Soc. Rev. 48, 428–446. doi:10.1039/c7cs00807d
Luo, G., and Gao, S. J. (2020). Global Health Concerns Stirred by Emerging Viral Infections. J. Med. Virol. 92, 399–400. doi:10.1002/jmv.25683
Makabenta, J. M. V., Nabawy, A., Li, C.-H., Schmidt-Malan, S., Patel, R., and Rotello, V. M. (2021). Nanomaterial-based Therapeutics for Antibiotic-Resistant Bacterial Infections. Nat. Rev. Microbiol. 19, 23–36. doi:10.1038/s41579-020-0420-1
Makvandi, P., Wang, C. Y., Zare, E. N., Borzacchiello, A., Niu, L. N., and Tay, F. R. (2020). Metal‐Based Nanomaterials in Biomedical Applications: Antimicrobial Activity and Cytotoxicity Aspects. Adv. Funct. Mater. 30, 1910021. doi:10.1002/adfm.201910021
Mu, H., Tang, J., Liu, Q., Sun, C., Wang, T., and Duan, J. (2016). Potent Antibacterial Nanoparticles against Biofilm and Intracellular Bacteria. Sci. Rep. 6 (1), 18877–18879. doi:10.1038/srep18877
Park, J., Shin, E., Yeom, J.-H., Choi, Y., Joo, M., Lee, M., et al. (2022). Gold Nanoparticle-DNA Aptamer-Assisted Delivery of Antimicrobial Peptide Effectively Inhibits Acinetobacter Baumannii Infection in Mice. J. Microbiol. 60 (1), 128–136. doi:10.1007/s12275-022-1620-3
Pelgrift, R. Y., and Friedman, A. J. (2013). Nanotechnology as a Therapeutic Tool to Combat Microbial Resistance. Adv. Drug Deliv. Rev. 65, 1803–1815. doi:10.1016/j.addr.2013.07.011
Radovic-Moreno, A. F., Lu, T. K., Puscasu, V. A., Yoon, C. J., Langer, R., and Farokhzad, O. C. (2012). Surface Charge-Switching Polymeric Nanoparticles for Bacterial Cell wall-targeted Delivery of Antibiotics. ACS Nano 6, 4279–4287. doi:10.1021/nn3008383
Singh, A., Kaushik, A., Dhau, J. S., and Kumar, R. (2022). Exploring Coordination Preferences and Biological Applications of Pyridyl-Based Organochalcogen (Se, Te) Ligands. Coord. Chem. Rev. 450, 214254. doi:10.1016/j.ccr.2021.214254
Tiwari, S., Juneja, S., Ghosal, A., Bandara, N., Khan, R., Wallen, S. L., et al. (2022). Antibacterial and Antiviral High-Performance Nanosystems to Mitigate New SARS-CoV-2 Variants of Concern. Curr. Opin. Biomed. Eng. 21, 100363. doi:10.1016/j.cobme.2021.100363
Wei, T., Tang, Z., Yu, Q., and Chen, H. (2017). Smart Antibacterial Surfaces with Switchable Bacteria-Killing and Bacteria-Releasing Capabilities. ACS Appl. Mater. Inter. 9 (43), 37511–37523. doi:10.1021/acsami.7b13565
Xu, J., Pan, Z., Peng, S., Zhao, Y., Jiang, S., Chen, Y. j., et al. (2021). Remarkable Bactericidal Traits of a Metal-Ceramic Composite Coating Elated by Hierarchically Structured Surface. Iscience 24, 101942. doi:10.1016/j.isci.2020.101942
Yaqub, A., Malkani, N., Shabbir, A., Ditta, S. A., Tanvir, F., Ali, S., et al. (2020). Novel Biosynthesis of Copper Nanoparticles Using Zingiber and Allium Sp. With Synergic Effect of Doxycycline for Anticancer and Bactericidal Activity. Curr. Microbiol. 77 (9), 2287–2299. doi:10.1007/s00284-020-02058-4
Yuan, Y.-G., Peng, Q.-L., and Gurunathan, S. (2017). Effects of Silver Nanoparticles on Multiple Drug-Resistant Strains of Staphylococcus aureus and Pseudomonas aeruginosa from Mastitis-Infected Goats: an Alternative Approach for Antimicrobial Therapy. Ijms 18 (3), 569. doi:10.3390/ijms18030569
Zanni, E., Chandraiahgari, C., De Bellis, G., Montereali, M., Armiento, G., Ballirano, P., et al. (2016). Zinc Oxide Nanorods-Decorated Graphene Nanoplatelets: A Promising Antimicrobial Agent against the Cariogenic Bacterium Streptococcus Mutans. Nanomaterials 6, 179. doi:10.3390/nano6100179
Keywords: nanomaterials, antimicrobial, bacteria, drug, antimicrobial surfaces
Citation: Garg P, Attri P, Sharma R, Chauhan M and Chaudhary GR (2022) Advances and Perspective on Antimicrobial Nanomaterials for Biomedical Applications. Front. Nanotechnol. 4:898411. doi: 10.3389/fnano.2022.898411
Received: 17 March 2022; Accepted: 14 April 2022;
Published: 03 May 2022.
Edited by:
Yogendra Kumar Mishra, University of Southern Denmark, DenmarkReviewed by:
Avtar Singh, Molekule Inc., United StatesVivek Anand Kamat, Florida International University, United States
Copyright © 2022 Garg, Attri, Sharma, Chauhan and Chaudhary. This is an open-access article distributed under the terms of the Creative Commons Attribution License (CC BY). The use, distribution or reproduction in other forums is permitted, provided the original author(s) and the copyright owner(s) are credited and that the original publication in this journal is cited, in accordance with accepted academic practice. No use, distribution or reproduction is permitted which does not comply with these terms.
*Correspondence: Ganga Ram Chaudhary, Z3JjMjJAcHUuYWMuaW4=; Moondeep Chauhan, bW9vbmRlZXAxOTg1QGdtYWlsLmNvbQ==