- 1Menzies Institute for Medical Research, University of Tasmania, Hobart, TAS, Australia
- 2Centre for Neuroscience, School of Medicine, Flinders University, Adelaide, SA, Australia
- 3The Florey Institute of Neuroscience and Mental Health, Parkville, VIC, Australia
Microtubule dynamics underpin a plethora of roles involved in the intricate development, structure, function, and maintenance of the central nervous system. Within the injured brain, microtubules are vulnerable to misalignment and dissolution in neurons and have been implicated in injury-induced glial responses and adaptive neuroplasticity in the aftermath of injury. Unfortunately, there is a current lack of therapeutic options for treating traumatic brain injury (TBI). Thus, using a clinically relevant model of mild TBI, lateral fluid percussion injury (FPI) in adult male Thy1-YFPH mice, we investigated the potential therapeutic effects of the brain-penetrant microtubule-stabilizing agent, epothilone D. At 7 days following a single mild lateral FPI the ipsilateral hemisphere was characterized by mild astroglial activation and a stereotypical and widespread pattern of axonal damage in the internal and external capsule white matter tracts. These alterations occurred in the absence of other overt signs of trauma: there were no alterations in cortical thickness or in the number of cortical projection neurons, axons or dendrites expressing YFP. Interestingly, a single low dose of epothilone D administered immediately following FPI (and sham-operation) caused significant alterations in the dendritic spines of layer 5 cortical projection neurons, while the astroglial response and axonal pathology were unaffected. Specifically, spine length was significantly decreased, whereas the density of mushroom spines was significantly increased following epothilone D treatment. Together, these findings have implications for the use of microtubule stabilizing agents in manipulating injury-induced synaptic plasticity and indicate that further study into the viability of microtubule stabilization as a therapeutic strategy in combating TBI is warranted.
Introduction
In popular media, mild traumatic brain injury (mTBI) has been referred to as a ‘silent epidemic.’ Indeed, in a majority of mTBI cases there is distinct absence of clear structural damage alongside normal neuroimaging (Iverson, 2005, 2010; Belanger et al., 2007; Jagoda et al., 2009; Gao and Chen, 2011; Smith et al., 2013). Nevertheless, subtle perturbations in brain structure likely evoke an insidious cascade of evolving widespread damage to neural circuitry, thought to culminate in long-term and ongoing neurological impairment and associated problems (Büki and Povlishock, 2006; Farkas and Povlishock, 2007; Blyth and Bazarian, 2010; Iverson, 2010; Wang and Ma, 2010; Meaney and Smith, 2011; Johnson et al., 2012a; Hill et al., 2016). This typically incorporates widespread axonal perturbation, known as traumatic axonal injury, throughout the parenchyma and particularly in the white matter tracts. This injury may include changes in the somato-dendritic compartment such as somal atrophy, distorted dendritic arbor geometry and loss of complexity, and decreased dendritic spine density (Chen et al., 2003, 2010; Stone et al., 2004; Spain et al., 2010; Gao and Chen, 2011; Gao et al., 2011; Greer et al., 2011; Campbell et al., 2012).
Data from experimental models shows that mTBI characteristically generates sparse microscopic damage, whereby neural circuits are rendered dysfunctional but not destroyed (Iverson, 2005; DeKosky and Ikonomovic, 2010; Shultz et al., 2016). This highlights an important target for therapeutic intervention. Microtubule disruption and loss is a key ultrastructural hallmark of neuronal injury (Maxwell and Graham, 1997; Tang-Schomer et al., 2012). Moreover, microtubule dynamics are fundamental to a multitude of neuro-glial responses in the aftermath of injury (Chuckowree and Vickers, 2003; Tang-Schomer et al., 2012; Brizuela et al., 2015). Thus, manipulating microtubules provides a novel multi-target approach for intervening in these processes (Brunden et al., 2012; Baas and Ahmad, 2013; Dent, 2016). Of note, microtubule-stabilizing agents of the taxane and epothilone families are used chemotherapeutically at high doses to block the growth of cancerous cells (Goodin, 2004; Michaud, 2009; Zhao et al., 2009; Khrapunovich-Baine et al., 2011). Accumulating data derived from a variety of experimental neural injury and disease paradigms reveals that when used at low doses these drugs have a range of beneficial effects, including dampening detrimental gliotic responses, preventing synapse loss and enhancing adaptive neuronal alterations as well as preserving cognitive and motor functions (Moscarello et al., 2002; Zhang et al., 2005; Andrieux et al., 2006; Ertürk et al., 2007; Brunden et al., 2010, 2011, 2012; Hellal et al., 2011; Sengottuvel et al., 2011; Barten et al., 2012; Tang-Schomer et al., 2012; Baas and Ahmad, 2013; Cartelli et al., 2013; Hur and Lee, 2014; Popovich et al., 2014; Brizuela et al., 2015; Cross et al., 2015; Ruschel et al., 2015; Jang et al., 2016; Penazzi et al., 2016).
The epothilones promote microtubule formation and stabilization, and inhibit microtubule depolymerization (Altmann et al., 2000; Chen et al., 2008). With particular relevance to the brain, epothilones are more water soluble than their taxane counterparts, are blood–brain barrier penetrant, and are retained in the central nervous system for several days after administration (Andrieux et al., 2006; Browne et al., 2011). Importantly, the efficacy of epothilones as a therapeutic strategy in the context of brain injury remains to be elucidated. To address this shortfall we explored the effect of peripherally administered epothilone D following a single mTBI using the clinically relevant lateral fluid percussion brain injury (FPI) model (Thompson et al., 2005). To visualize discrete alterations in the somato-dendritic and axonal compartments of layer 5 cortical excitatory projections neurons we used the Thy1-YFPH mouse, which revealed exquisite neuronal sub-structure (Feng et al., 2000).
Materials and Methods
Breeding and Genotyping of Thy1-YFPH Transgenic Mice
All experimental procedures involving animals were approved by the Animal Ethics Committee of the University of Tasmania (ethics approval number A0011076) and are in accordance with the Australian Code of Practice for the Care and Use of Animals for Scientific Purposes. Animals were housed in standard conditions (20°C, 12 h/12 h light/dark cycle) with access to food and water ad libitum and monitored daily for signs of stress and illness. Thy1-YFPH line mice [B6.Cg-Tg(Thy1-YFP)HJrs/J, stock number 003782] were obtained from the Jackson Laboratory (Bar Harbor, ME, United States) and maintained as a heterozygous colony. In these animals YFP is expressed under the control of the neuron-specific Thy1 promoter in ∼80% of layer 5 and 2/3 neocortical pyramidal neurons (Feng et al., 2000). Ear punches were taken at weaning (4 weeks) to determine inheritance of the YFP transgene. Tissue was mounted on a glass slide and examined with the 488 nm filter on a Leica DM LB2 microscope (Leica Microsystems Pty Ltd., North Ryde, NSW, Australia). Animals carrying the YFP transgene were identified as possessing YFP-positive (YFP+) axons within their ear clips.
Surgical Preparation
Animals were prepared in groups of four per day. Two animals received FPI while two were sham-operated. Mice were subjected to lateral FPI using an established protocol (Carbonell et al., 1998; Lifshitz et al., 2007; Alder et al., 2011). Briefly, adult male YFP-H mice (10–12 weeks, 25–30 g; n = 12 FPI/brain-injured, n = 12 sham-operated) were anesthetised in a pre-charged induction chamber containing 5% isoflurane (Isoflo, Abbot Australasia Pty Ltd., Botany, NSW, Australia) in 100% O2. Mice were removed from the induction chamber, pre-emptive analgesia, temgesic (buprenorphine hydrochloride, 0.1 mg/kg; Reckitt Benckiser, West Ryde, NSW, Australia), was administered subcutaneously and the fur covering the scalp was removed. Mice were placed on a homeothermic blanket (Stoelting, Wood Dale, IL, United States) to maintain body temperature at 37°C during surgery and stabilized in a stereotaxic frame (Narishige, Tokyo, Japan) equipped with a nose cone to maintain anesthesia (1–2% isoflurane in 100% O2). The scalp was cleaned with betadine (Sanofi-aventis Consumer Healthcare, Virginia, QLD, Australia) and 70% ethanol and the topical anesthetic bupivicaine (Bupivicaine hydrochloride, 50 μl 0.25% in sterile saline; Pfizer, West Ryde, NSW, Australia) was administered under the scalp. A midline incision was made to expose the skull from bregma to lambda. The skin was retracted and the fascia covering the skull was removed. A 3.0 mm circular craniectomy was made 2.0 mm posterior and 2.5 mm lateral to bregma on the right hand side of the skull over the somatosensory cortex via manual trephination with a pin vice equipped with a 2.7 mm trephine drill bit (AgnTho’s, Lidingo, Sweden). The underlying dura was left intact. An injury-hub was constructed over the craniectomy – a sterile Luer-Loc syringe hub was cut from a 22-gauge needle, fixed over the craniectomy using Loctite cyanoacrylate (Henkel Australia, Sydney, NSW, Australia), secured to the skull using Paladur dental acrylic (Heraeus Dental Science, Villebon, France), filled with sterile saline and capped with a male Luer-Loc fitting. Pre-, peri-, and post-surgical monitoring was performed to determine respiratory rate, confirm absence of reflexes and monitor mucous membranes/capillary refill time. Following injury-hub placement animals were removed from the stereotaxic frame and allowed to recover in a warmed cage until fully ambulatory (30–60 min) and then placed back in their home cage.
Lateral Fluid Percussion Brain Injury and Drug Treatment
Two hours following application of the injury-hub, once mice had been fully ambulatory for over an hour, each animal was re-anesthetised in a pre-charged induction chamber containing 5% isoflurane in 100% O2. Following induction of anesthesia, the animal was removed from the induction chamber, the cap was removed from the injury hub and the hub was re-filled with sterile saline and attached to the FP302 Fluid Percussion Device (AmScien Instruments, Richmond, VA, United States) via a 30 cm spacing tube filled with sterile water. The animal was placed on a heated pad and once a normal pattern of breathing resumed, but prior sensitivity to stimulation, an injury of mild severity (1.5 ± 0.1 atmospheres) was delivered to the intact dura by releasing device’s pendulum onto a fluid filled piston, causing transient displacement and deformation of the dura and underlying brain. A transducer incorporated into the device measured the pulse pressure and the peak pressure was recorded within the software. Following injury, animals were placed on their back and visually monitored for recovery of spontaneous breathing. Additionally, the time taken for animals to recover the righting reflex was recorded as a measure of transient unconsciousness/loss of consciousness. Following injury, we did not record any convulsions, mortalities or other complications. Sham-operated animals underwent identical procedures to FPI animals, however, the pendulum was not released. Mice were re-anesthetised, the injury hub was removed and the incision sutured. Immediately following suturing, animals were administered with either epothilone D (2 mg/kg, i.p.; Anita Laboratories, Hangzhou, China; n = 6 FPI and n = 6 sham-operated) or vehicle (equivalent volume DMSO; n = 6 FPI and n = 6 sham-operated). Mice received drug/vehicle treatment within 20 min following completion application of the FPI/Sham-operation. Animals were placed in a heated cage and monitored during the recovery period until fully ambulatory (30–60 min), prior to return to their home cage.
Immunohistochemistry
At 1 week post-injury/sham-operation, mice were intraperitoneally injected with a terminal dose of sodium pentobarbital (300 mg/kg; Troy Laboratories Pty Ltd., Smithfield, NSW, Australia) and transcardially perfused with 4% paraformaldehyde in 0.1 M phosphate buffer. Brains were post-fixed in vivo for 24 h at 4°C. Each brain was removed from the skull, embedded in 5% agarose in 0.01 M phosphate buffered saline (PBS) and free-floating coronal sections (50 μm) were cut using a Leica VT1000S vibratome (Leica Biosystems Australia Pty Ltd., Mount Waverly, VIC, Australia) to incorporate the entire injury impact site as well as 0.5–1.0 mm anterior and posterior to this. Sections were serially collected into Costar 24-well culture plates (Corning Life Sciences, New York, NY, United States) containing 0.01M PBS and 0.02% sodium azide and stored until required.
To perform immunohistochemistry, every sixth section from each brain was moved into a fresh culture plate well to represent the injury site. Prior to immunohistochemistry, sections were rinsed in three washes of 0.01 M PBS. Sections then underwent immunofluorescence labeling for glial fibrillary acidic protein (GFAP). Briefly, sections were incubated in rabbit anti-GFAP (1:2000; DAKO, Z0334, Glostrup, Denmark) in diluent (0.01 M PBS with 0.03% Triton X-100) at 4°C for ∼20 h, washed, incubated in goat anti-rabbit Alexa 568 (1:1000; Invitrogen BRL, Life Technologies, Grand Island, NY, United States) and DAPI (1:6000; Invitrogen, D3571) in 0.01M PBS for 1.5 h, washed and mounted serially onto slides (Livingstone International Pty Ltd., Rosebery, NSW, Australia) with Permafluor mounting media (Thermo Scientific, Scoresbury, VIC, Australia).
Microscopy and Image Analysis
Images were collected with an UltraVIEW spinning disk confocal microscope running Volocity Software (PerkinElmer Pty Ltd., Glen Waverley, VIC, Australia), equipped with a 20×/0.5 air, 40×/0.95 air and Plan Apo 60×/1.20 water objective (Nikon, New York, NY, United States). For quantitation of cortical thickness, YFPH cell number and size and degenerated/dystrophic axonal bulb number and size (in the internal and external capsules) the microscope was configured to capture large stitched images of the upper hemispheric quadrant of each brain (20 μm z-stacks, 1 μm slices) with the 20× objective from three representative sections throughout the injury, designated middle, anterior, and posterior representing the middle section (first appearance of two blades of the dentate gyrus) as well as the section 300 μm anterior and 300 μm posterior to this. Using ImageJ freeware (Schneider et al., 2012) the cortex, external and internal capsule were traced in each of the three sections and within these anatomical boarders the individual YFPH+ cells in the cortex and axonal bulbs/dystrophic neurites in the external and internal capsules were traced for quantitation of size and density. To determine the axonal degeneration index (degenerating/beaded YFPH+ axons) single 40× magnification image stacks (20 μm z-stacks, 1 μm slices) were collected from the same three sections as used for the prior analysis. Images for the external capsule were captured from the white matter on the medio-lateral boarder of the lateral ventricle and those for the internal capsule were captured half way between the dorsal and ventral boarder of the internal capsule. For quantification of astrocyte activation (percent area occupied by GFAP expressing astrocytes), 20× single image stacks (20 μm z-stacks, 1 μm slices) were collected from the same regions used for analysis of axonal denegation, in addition to layer 2/3 of the cortex directly under the impact site. Analysis of percentage area GFAP and YFPH+ axonal degeneration was performed using ImageJ freeware. For dendritic spine analysis, image stacks were captured with the 60× water objective (0.2 μm slices). Image stacks were collected from layer 4/5 directly under the injury site. These included the image in the middle of the impact site as well as an image medial and lateral to this. All layer 5 apical dendrite obliquely projecting branches were traced from each stack and the spines contained on these dendrites were traced in Neurolucida (MBF Biosciences, Williston, VT, United States). Morphology data was generated by allocating each spine to one of three categories, mushroom (prominent head, thin neck), stubby (greater width than length), and thin (greater length than width). Changes in dendritic spine density, length, and morphology were determined by loading Neurolucida data files into Neurolucida ExplorerTM (MBF Biosciences).
Statistical Analysis
Data was analyzed (and graphs created) in GraphPad Prism (version 6.0, La Jolla, CA, United States) using unpaired t-tests with Welch’s correction, or one- or two-way analysis of variance (ANOVA) followed by Tukey’s multiple comparison test. Averaged values were expressed as means ± standard error of the mean (SEM). A p-value of <0.05, designated ∗, was considered statistically significant. Figures were prepared in Adobe Illustrator CS6 (version 16.0.0, Adobe Systems, San Jose, CA, United States).
Results
A Single Mild Lateral FPI Caused a Transient Loss of Consciousness in the Absence of Overt Morphological Change
Adult male Thy1-YFPH mice received a single mild lateral FPI or sham-operation followed by epothilone D or vehicle treatment and were perfused 7 days later. In brain-injured mice the acute post-injury period was characterized by a transient loss of consciousness, including a short interval of apnoea (0.21 ± 0.04 min) and a significant delay in the righting reflex (3.64 ± 1.63 min in brain-injured relative to 0.19 ± 0.13 min in sham-operated animals; p < 0.0001, unpaired t-test with Welch’s correction). By 7 days post-injury, there were no gross morphological alterations in the brain following a single mild lateral FPI: cortical thickness, as well as the number and somal size of cortical layer 5 YFP+ projection neurons, remained unchanged (Table 1, p > 0.05 for all comparisons). Moreover, there was no change in axonal number (axons per field of view) in the external and internal capsules, or the length of apical oblique dendrites (total length of dendrite per field of view) of layer 5 pyramidal neurons (Table 1). Epothilone D treatment did not significantly (p > 0.05 for all comparisons) affect any of these parameters, with cortical thickness, number and size of YFP+ cells, number of YFP+ axons in both the external and internal capsules and length of YFP+ apical oblique dendrites remaining unaltered following peripherally administered epothilone D (Table 1).
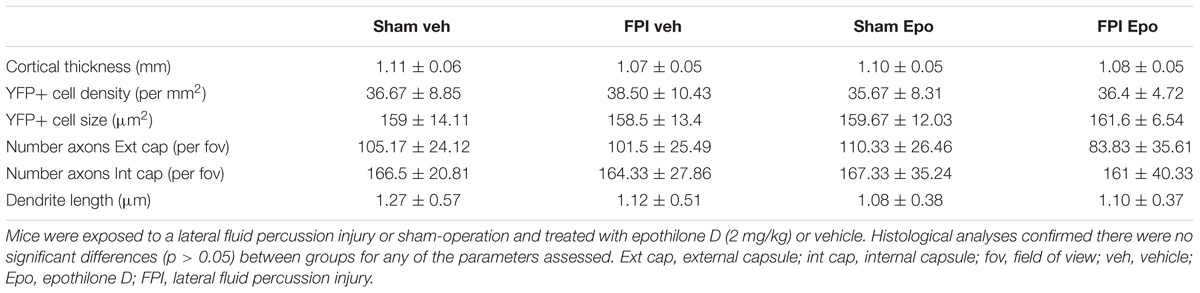
TABLE 1. Histological analyses comparing brain-injured and sham-operated animals following drug treatment.
Epothilone D Treatment Altered Dendritic Spine Length, density, and Morphology
Dendritic spines were analyzed from radially projecting/oblique branches of layer 5 projection neuron apical dendrites at the layer 4/5 boarder (Figures 1A,B). All major morphological spine classes (mushroom, stubby, thin) were represented in all mouse groups (Figure 1C). Initial analysis segregated the total spine population by length – spines (<2.5 μm) and filopodia (>2.5 μm) (Hering and Sheng, 2001) – and revealed a significant decrease (p < 0.05) in average spine length (Figure 1D), but not filopodial length (Figure 1E), in response to epothilone D treatment relative to vehicle treatment in both brain-injured and sham-operated animals. Furthermore, binning the spines by length showed that epothilone D treatment resulted in a significantly higher proportion (p < 0.05) of shorter (<1.5 μm) spines and significantly lower proportion (p < 0.05) of longer spines (1.5–2.5 μm) (Figure 1F). With respect to density, spine density was significantly increased (p < 0.05) in sham-operated, but not brain-injured animals (Figure 1G) in response to epothilone D treatment, whereas filopodial density was unaltered in both sham-operated and brain-injured animals (Figure 1H). Analysis of morphological sub-class revealed a significant increase (p < 0.05) specifically in mushroom spines in both brain-injured and sham-operated animals in response to epothilone D treatment (Figure 1I).
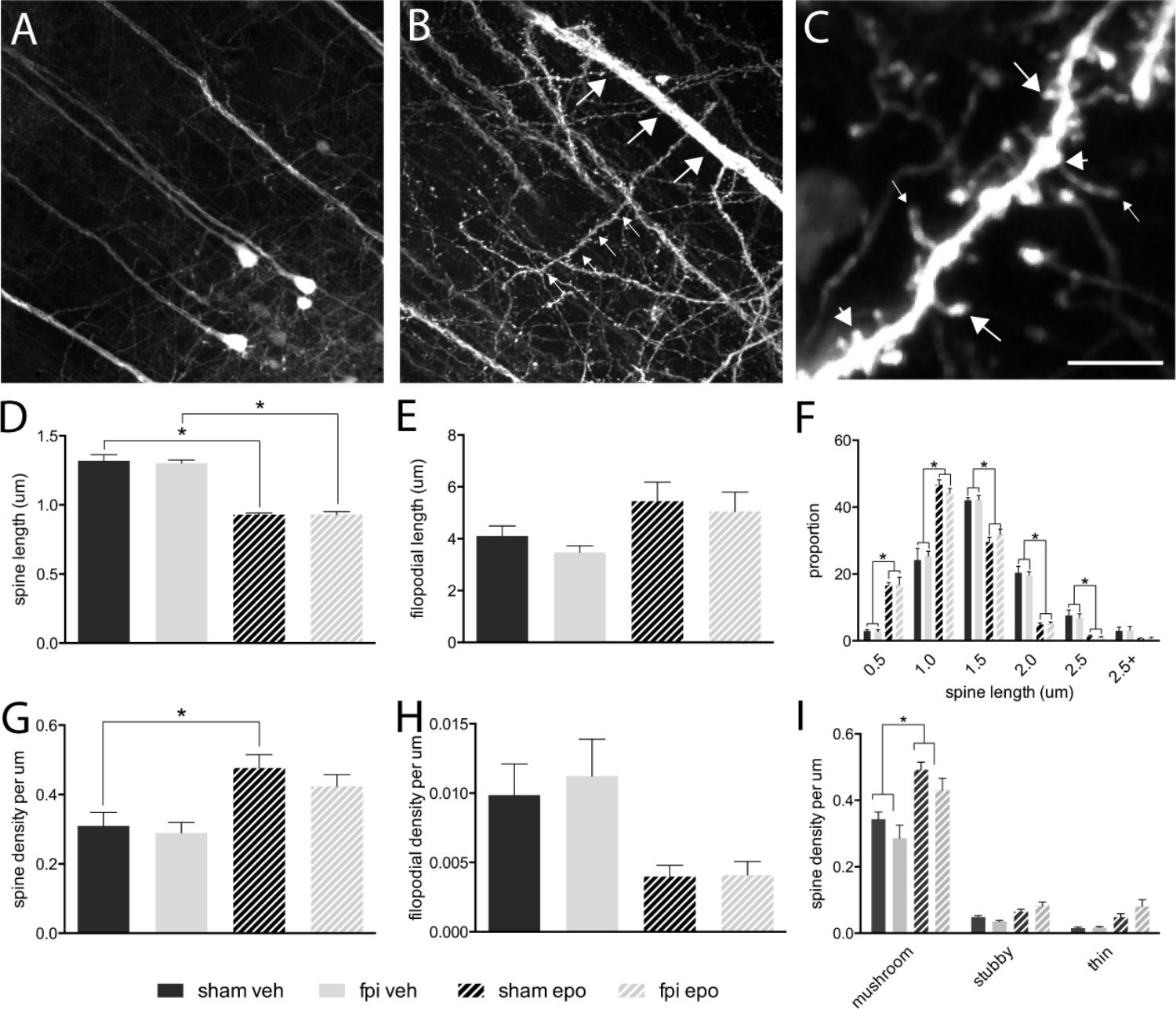
FIGURE 1. Dendritic spine alterations in layer 5 pyramidal neurons in the adult mouse brain following mild lateral fluid percussion brain injury and epothilone D treatment. Analysis was performed on layer 5 YFP-expressing neuron (A) apical oblique dendrites, which are laterally projecting spiney dendrites in layer 4/5 (small arrows, B) protruding from layer 5 neuron apical dendrites (large arrows, B). For analysis, dendritic protrusions/spines were classified both by length (spines <2.5 μm, D,F; filopodia > 2.4 μm, E,G) and morphology (C,I): either mushroom (large arrows in C), stubby (short arrows in C), or thin (small arrows in C). Epothilone D treatment resulted in a significant decrease in spine length in both brain-injured and sham-operated animals, relative to their vehicle-treated controls (D), whereas filopodial length was unaffected (E). Binning the data by length revealed that epothilone D treatment resulted in a significantly higher proportion of shorter spines (up to 1.5 μm) and significantly lower proportion of longer spines (1.5–2.5 μm) (F). Moreover, epothilone D treatment significantly increased the density of dendritic spines in sham-operated, but not brain-injured animals (G), while filopodial density was unaffected (H). Analysis by morphological sub-class showed that the epothilone D treatment resulted in increased density of mushroom spines in both brain-injured and sham-operated animals relative to their vehicle-treated counterparts, while density of stubby and thin spines was unaffected (I). Data are presented as mean ± SEM and were analyzed by one-way (D,E,G,H) or two-way (F,I) ANOVA, followed by Tukey’s multiple comparison test. A p-value of < 0.05 was considered significant (∗). Scale bar (A) = 85 μm; (B) = 45 μm; (C) = 3.5 μm. Yellow fluorescent protein (YFPH); sham-operated, vehicle-treated (sham veh); fluid percussion injury, vehicle-treated (fpi veh), sham-operated, epothilone D-treated (sham epo); fluid percussion injury, epothilone D-treated (fpi epo).
Axonal Degeneration in the External and Internal White Matter Tracts Was a Major Feature of the Injured Brain and Was Not Altered by Epothilone D Treatment
By 7 days post-injury, a single mild lateral FPI had generated a distinct pattern of ipsilateral axonal damage throughout the external (Figure 2A) and internal (Figure 2B) capsule white matter tracts. Although the majority (85–90%) of YFP expressing axons remained intact, a significant proportion (p < 0.05) of axons showed a degenerating, beaded morphology or a disconnected, degenerated and bulbar axonal fragment morphology within the ipsilateral external capsule of brain-injured relative to sham-operated animals (Figures 2C,D). These axonal changes after mild FPI did not reach significance in the ipsilateral internal capsule (Figure 2D) or contralateral external capsule (not shown). Further analysis of the axonal response to mild FPI revealed that number and size of degenerated/dystrophic bulbar axonal fragments was significantly increased (p < 0.05) in the ipsilateral external capsule (Figures 2E,F). Moreover, degenerated axonal bulb number, but not size, was significantly increased (p < 0.05) in the ipsilateral internal capsule (Figures 2E,F) after mild FPI. Interestingly, epothilone D treatment did not alter any aspect of the axonal response to mild FPI (Figures 2D–F).
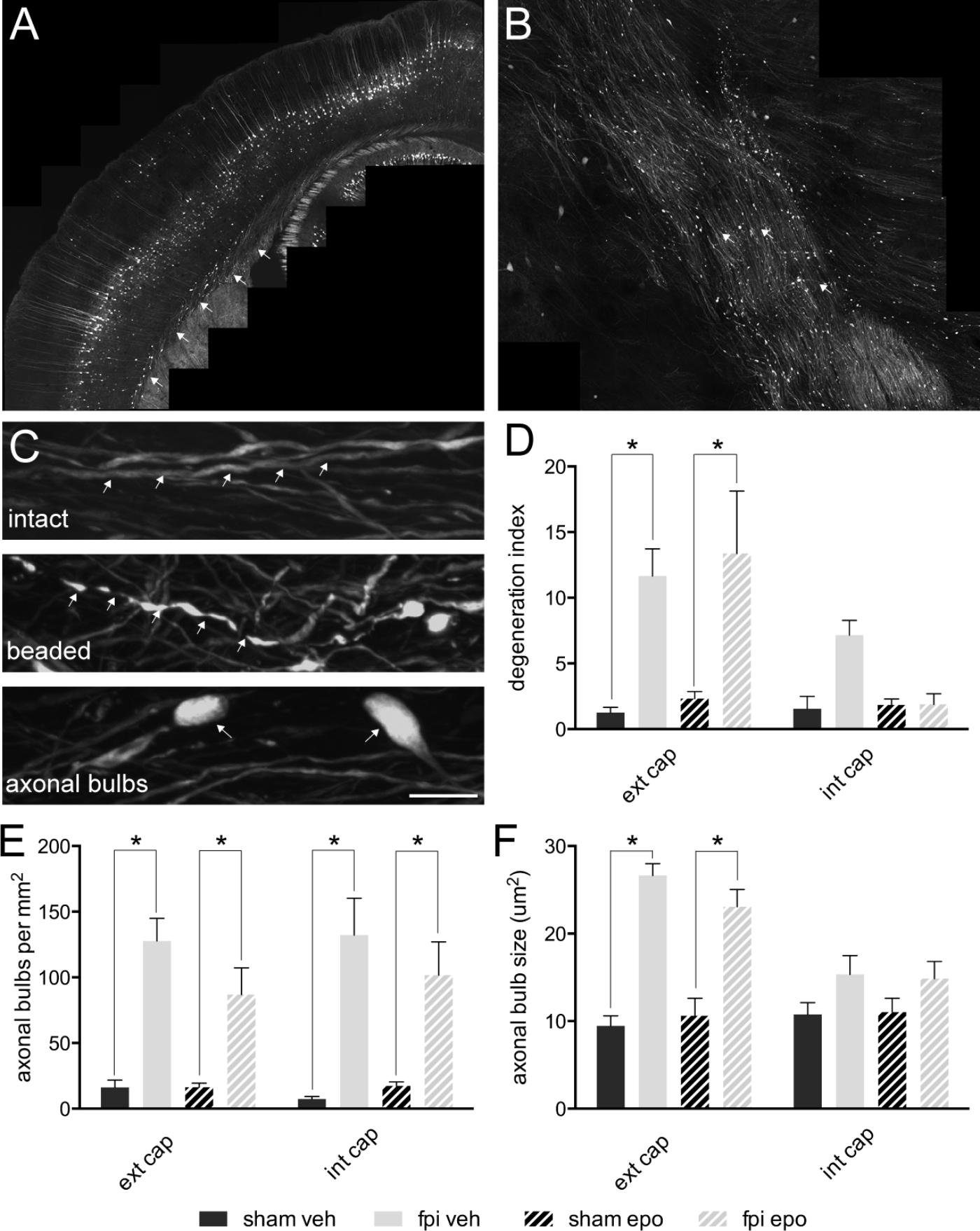
FIGURE 2. Axonal alterations in major white matter tracts in the adult mouse brain following mild lateral fluid percussion brain injury and epothilone D treatment. Mild lateral fluid percussion injury to the adult mouse brain generated a stereotypical and widespread pattern of axonal damage in the external (A) and internal (B) capsule white matter tracts. At 7 days post-injury the majority of axons remained intact (arrows, upper panel in C), while a proportion showed a degenerating, beaded morphology (arrows, middle panel in C) or a degenerated, fragmented and disconnected ‘axonal bulb’ morphology (arrows, lower panel in C). The proportion of beaded axons (out of the total axon population) was significantly increased in the ipsilateral external capsule of brain-injured animals relative to their sham-operated counterparts, but was unaltered in the ipsilateral internal capsule (D). Analysis of axonal bulbs indicated that there were significantly more axonal bulbs in both the external and internal capsules of brain-injured animals relative to their sham-operated counterparts (E). Moreover, axonal bulbs were significantly larger in the external, but not internal, capsule of brain injured animals relative to their sham-operated counterparts (F). Data are presented as mean ± SEM and were analysed by two-way ANOVA (D–F) followed by Tukey’s multiple comparison test. A p-value < 0.05 was considered significant (∗). Scale bar (A) = 400 μm; (B) = 125 μm; (C) = 10 μm. Sham-operated, vehicle-treated (sham veh); fluid percussion injury, vehicle-treated (fpi veh); sham-operated, epothilone D-treated (sham epo); fluid percussion injury, epothilone D-treated (fpi epo); ipsilateral external capsule (ext cap); ipsilateral internal capsule (int cap).
Mild Lateral FPI Evoked a Limited Ipsilateral Astrogliotic Response That Was Not Influenced BY Epothilone D Treatment
Astrocyte activation was quantitated as the area occupied by GFAP immunoreactive profiles at 7 days post-injury. GFAP was significantly increased (p < 0.05) in the ipsilateral vs. contralateral cortex of all mice (sham-operated vehicle treated ipsilateral cortex, 6.20 ± 0.68% vs. sham-operated vehicle treated contralateral cortex, 2.98 ± 0.37%; brain-injured vehicle treated ipsilateral cortex, 6.13 ± 0.79% vs. brain-injured vehicle treated contralateral cortex, 3.23 ± 0.54%; sham-operated epothilone treated ipsilateral cortex, 5.91 ± 0.64% vs. sham-operated epothilone treated contralateral cortex, 5.61 ± 0.32%; brain-injured epothilone treated ipsilateral cortex, 5.61 ± 0.32% vs. brain-injured epothilone treated contralateral cortex, 2.87 ± 0.25%), indicating that the craniectomy itself generated mild astroglial activation. More interestingly, GFAP immunoreactive profiles (as measured by percentage area occupied by GFAP immunoreactivity) were significantly increased in the external capsule of brain-injured animals (vehicle-treated 5.79 ± 0.49%; epothilone-treated 5.20 ± 0.55%) relative to their sham-operated (vehicle-treated 3.58 ± 0.05%; epothilone-treated 3.63 ± 0.53%) controls; however, this change did not extend to the internal capsule.
Discussion
Despite the absence of overt structural damage and the presence of normal neuroimaging in a majority of mTBI cases, subtle widespread and progressing damage within neural circuits is thought to underlie the development, and potentially ongoing evolution, of impairments in neurosensory, cognitive, psychosocial, and physical function (Iverson, 2005, 2010; Farkas and Povlishock, 2007; Dean and Sterr, 2013; McMahon et al., 2014; Hill et al., 2016; Shultz et al., 2016; Wang and Li, 2016; Wilde et al., 2016; de Koning et al., 2017). The cellular alterations contributing to pathology in the aftermath of mTBI are not fully understood and there exists no treatment to halt or reverse the damage to neural circuits. Thus, we investigated the discrete subcellular reactions of axonal and somato-dendritic compartments following transient structural brain injury and epothilone D-induced microtubule stabilization. We should note that this study represents a proof-of-concept investigation demonstrating that peripherally administered epothilone D can enter the brain and have an effect on neurons. In accordance with established protocols (Carbonell et al., 1998; Spain et al., 2010; Alder et al., 2011) we showed that a single episode of mild TBI generated significant loss of consciousness, as measured by injury-induced apnoea and delayed righting, in the absence of overt macroscopic change. At the cellular level there was no significant loss of YFP+ neurons, axons or dendrites or dendritic spines and no evidence of neuronal atrophy by 7 days post injury. Moreover, the dose of epothilone D was well tolerated and there were no overt indications of ill health or drug-induced cellular degeneration.
Dendritic spine loss is a characteristic feature of a variety of diseases and injuries of the nervous system (Fiala et al., 2002a; Campbell et al., 2012). Moreover, synapse loss is a likely key candidate for the emergence of functional deficits following TBI. Correct functioning of the neuronal cytoskeleton is crucial for the maintenance of neuronal integrity. Actin has a well-established and integral role in the function of dendritic spines (Matus, 2000; Hotulainen and Hoogenraad, 2010; Lei et al., 2016). More recently, microtubules have been implicated in spine formation and maintenance (Gu et al., 2008; Jaworski et al., 2009; Kapitein et al., 2010; Dent, 2016). To determine the effect of microtubule stabilization at the level of dendritic spines, and to avoid any potential confounding effects of the craniotomy, we examined spines on the radially projecting branches, residing in layer 4/5, of layer 5 YFP+ cortical projection neuron apical dendrites. Interestingly, while filopodia remained unaltered in terms of both length and density, epothilone D had dramatic effects on the spine population, causing an overall reduction in spine length, a shift to a greater proportion of short spines, and an increase in the formation of mushroom spines. The increase in spine density, specifically of mushroom spines, was an unanticipated response to epothilone D treatment and was observed both within the brain-injured and sham-operated cortex. This may indicate a more generalized effect of microtubule stabilization at the subtle level of the dendritic spine, rather than a neuroprotective effect of the drug evoked by injury. It is notable that the increase in spine density occurred specifically in the mushroom spines, which have previously been shown to be a relatively stable population likely to host synapses (Hering and Sheng, 2001; Fiala et al., 2002b). Although we were unable to trace axons to determine innervation interactions between degenerating axons and newly formed spines or determine the functionality of the newly formed spines using the current methodology, future studies quantifying colocalization of synaptic markers, ultrastructural and electorphysiological analysis as well as in vivo imaging of synaptic turnover could be used to determine the presense/absence and functionality of synapses on newly formed spines and validate the therapeutic potential of epothilone D.
Although the current study did not directly address the mechanism by which epothilone D increased spine density, it is plausibile that this may be due to a direct effect on the spines themselves, the axons innervating the spines or a more indirect effect. Nonetheless, in terms of therapeutic potential, microtubule stabilization may be useful for preserving spine and synapse integrity following injury. In accordance with this, we revealed a distinct increase in spine density following epothilone treatment. Moreover, spine restoration has been demonstrated following epothilone D treatment in an Alzheimer’s disease model (Penazzi et al., 2016). Although further investigations into the mechanisms of spine generation and maintenance by epothilone D are required, we postulate that microtubule stabilization and polymerization with epothilone D may prevent microtubule disassembly and catastrophe within both the dendrite shaft and spines, preserving spine integrity and reducing spine loss. If spine generation continues, this may contribute to an overall increase in spine density. Whether this is a detrimental or beneficial response in the aftermath of mTBI remains to be elucidated. Interestingly, since changes in spine morphology have been linked to alterations in synaptic strength and implicated in learning and memory (Bourne and Harris, 2007; Gu et al., 2008; Jaworski et al., 2009), manipulation of microtubule dynamics could potentially be used to modulate these parameters. As the epothilone D induced increase in spine density was unanticipated, based on previous literature future studies should determine whether these alterations evoke measureable functional responses in terms of electrophysiological and functional output.
Mild TBI has been described as a progressive disorder. Although we did not see a significant loss of axons, dendrites or spines by 7 days post-injury in the current study, there was certainly observable axonal damage throughout the ipsilateral cortex and white matter. Interestingly, the epothilone D treatment regime used in the current study did not have a protective effect on degenerating axons and our previous study using a mouse model of amyotrophic lateral sclerosis showed chronic epothilone D exposure may be detrimental to axons (Clark et al., 2018). However, using an in vitro model of structural axonal injury we have previously shown that low dose epothilone D has a protective effect on injured axons by increasing injury-induced axonal sprouting (Brizuela et al., 2015), indicating that microtubule stabilization within damaged axons may be partially responsible for the previously described neuroprotective effect of epothilone D and may have feed forward effects with regard to dendritic spine innervation. If followed to later post-injury time points, it is possible we may have observed evidence of neuronal atrophy, progressive axonal degeneration and spine loss following mild lateral FPI, as has been shown in other studies of brain injury (Fiala et al., 2002a; Chen et al., 2010; Gao and Chen, 2011; Gao et al., 2011; Greer et al., 2011; Campbell et al., 2012; Johnson et al., 2012b; Winston et al., 2013) and the previously described therapeutic effects of epothilone D may have been revealed. In our hands, and in accordance with previous studies, mild lateral FPI generated widespread traumatic axonal injury, which was particularly evident in the external and internal capsule white matter tracts (Smith and Meaney, 2000; Spain et al., 2010; Wang and Ma, 2010; Ekmark-Lewen et al., 2013; Smith et al., 2013; Hill et al., 2016). This included both beaded, degenerating axons and degenerated, dystrophic axonal fragments/bulbs. Both phenotypes have been reported in the literature and may represent stages of degeneration, differences in vulnerability between different axonal sub-classes or brain regions, or be specific to the mechanical forces sustained during injury (Reeves et al., 2005; Browne et al., 2011; Hånell et al., 2014).
Interestingly, axonal sprouting has been observed in certain classes of damaged axons in experimental models of brain injury (Salin et al., 1995; Batchelor et al., 2002; Deller et al., 2006; Dickson et al., 2007; Blizzard et al., 2011; Greer et al., 2011). Although not observed in the current study, it is possible that epothilone D could be used to modulate this response. Indeed, using an in vitro model, we have shown that epothilone D has dose-dependent effects on regenerating cortical neurons (Brizuela et al., 2015). This concept has important implications for dose-dependent modulation of axonal sprouting in vivo, for example enhancing adaptive regenerative attempts or dampening maladaptive sprouting, which may underlie the development of epileptic activity in the aftermath of injury (Santhakumar et al., 2001; Kharatishvili et al., 2006; Bolkvadze and Pitkänen, 2012).
In response to FPI we observed mild ipsilateral astroglial activation in the absence of glial scar formation. Contrary to previous studies, astroglial activation was not influenced by microtubule stabilization in the current study (Hellal et al., 2011; Popovich et al., 2014; Ruschel et al., 2015). This may have been due to a range of factors including the type of agent used (a taxane versus an epothilone), the concentration or timing of the epothilone D dose, the mild nature of the glial activation, or the context of the injury (for example, brain versus spinal cord). The absence of an observable injury-induced alteration in dendritic spine density in the current study may have been due to the spine population investigated, the proximity of the spines to the site of impact, the brain region assessed and its proximity to the injury, as well as the time point at which analysis was performed. Therefore, to elucidate the efficacy of microtubule stabilization as a therapeutic intervention for mTBI further investigations will be required to reveal the full repertoire of effects of microtubule stabilization in both the acute and chronic phases of the injury response. Future studies will use a range of dose regimes, including various concentrations and times of administration throughout the post-injury sequalae, to capture the dynamic aspects of injury-induced degeneration and remodeling.
In summary, our findings indicate that peripherally administered microtubule-stabilizing drugs alter synaptic plasticity at the level of the dendritic spine. This has important implications for controlling neuroplasticity in the aftermath of brain injury. More generally, microtubule-stabilizing agents may be useful for manipulating various aspects of the neuro-glial response to injury and disease, as well as providing a modulatory tool for investigating microtubule dynamics per se. Due to the ubiquity of microtubules it will be imperative to consider the full repertoire of roles in which they are involved, and take into consideration the interplay between intrinsic factors such as neuronal class and age and extrinsic factors such as the type and severity of injury.
Author Contributions
All authors have made a substantial contribution to the work and approved it for publication. Specifically JC, TD, CB conceived and designed the experiments, wrote the manuscript and analyzed and interpreted the data. JC, ZZ, MB, and KL performed the experiments and acquired the data.
Funding
This research was supported by the National Health and Medical Research Council of Australia, Select Foundation, Brain Foundation Australia, Motor Accident Insurance Board Tasmania, Flack Foundation, Internal Research Grant Scheme (University of Tasmania), and Tasmanian Masonic Centenary Medical Research Foundation.
Conflict of Interest Statement
The authors declare that the research was conducted in the absence of any commercial or financial relationships that could be construed as a potential conflict of interest.
Acknowledgments
We would like to thank Dr. Katherine Lewis for technical editing and proofreading the manuscript.
References
Alder, J., Fujioka, W., Lifshitz, J., Crockett, D. P., and Thakker-Varia, S. (2011). Lateral fluid percussion: model of traumatic brain injury in mice. J. Vis. Exp. 54:3063. doi: 10.3791/3063
Altmann, K. H., Wartmann, M., and O’Reilly, T. (2000). Epothilones and related structures – a new class of microtubule inhibitors with potent in vivo antitumor activity. Biochim. Biophys. Acta 1470, M79–M91. doi: 10.1016/S0304-419X(00)00009-3
Andrieux, A., Salin, P., Schweitzer, A., Bégou, M., Pachoud, B., Brun, P., et al. (2006). Microtubule stabilizer ameliorates synaptic function and behavior in a mouse model for schizophrenia. Biol. Psychiatry 60, 1224–1230. doi: 10.1016/j.biopsych.2006.03.048
Baas, P. W., and Ahmad, F. J. (2013). Beyond taxol: microtubule-based treatment of disease and injury of the nervous system. Brain 136, 2937–2951. doi: 10.1093/brain/awt153
Barten, D. M., Fanara, P., Andorfer, C., Hoque, N., Wong, P. Y. A., Husted, K. H., et al. (2012). Hyperdynamic microtubules, cognitive deficits, and pathology are improved in Tau transgenic mice with low doses of the microtubule-stabilizing agent BMS-241027. J. Neurosci. 32, 7137–7145. doi: 10.1523/JNEUROSCI.0188-12.2012
Batchelor, P., Porritt, M., Martinello, P., Parish, C., Liberatore, G., Donnan, G., et al. (2002). Macrophages and microglia produce local trophic gradients that stimulate axonal sprouting toward but not beyond the wound edge. Mol. Cell. Neurosci. 21, 436–453. doi: 10.1006/mcne.2002.1185
Belanger, H. G., Vanderploeg, R. D., Curtiss, G., and Warden, D. L. (2007). Recent neuroimaging techniques in mild traumatic brain injury. J. Neuropsychiatry Clin. Neurosci. 19, 5–20. doi: 10.1176/jnp.2007.19.1.5
Blizzard, C. A., Chuckowree, J. A., King, A. E., Hosie, K. A., Mccormack, G. H., Chapman, J. A., et al. (2011). Focal damage to the adult rat neocortex induces wound healing accompanied by axonal sprouting and dendritic structural plasticity. Cereb. Cortex 21, 281–291. doi: 10.1093/cercor/bhq091
Blyth, B. J., and Bazarian, J. J. (2010). traumatic alterations in consciousness: traumatic brain injury. Emerg. Med. Clin. North Am. 28, 571–594. doi: 10.1016/j.emc.2010.03.003
Bolkvadze, T., and Pitkänen, A. (2012). Development of post-traumatic epilepsy after controlled cortical impact and lateral fluid-percussion-induced brain injury in the mouse. J. Neurotrauma 29, 789–812. doi: 10.1089/neu.2011.1954
Bourne, J., and Harris, K. M. (2007). Do thin spines learn to be mushroom spines that remember? Curr. Opin. Neurobiol. 17, 381–386. doi: 10.1016/j.conb.2007.04.009
Brizuela, M., Blizzard, C. A., Chuckowree, J. A., Dawkins, E., Gasperini, R. J., Young, K. M., et al. (2015). The microtubule-stabilizing drug Epothilone D increases axonal sprouting following transection injury in vitro. Mol. Cell. Neurosci. 66(Pt B), 129–140. doi: 10.1016/j.mcn.2015.02.006
Browne, K. D., Chen, X.-H., Meaney, D. F., and Smith, D. H. (2011). Mild traumatic brain injury and diffuse axonal injury in swine. J. Neurotrauma 28, 1747–1755. doi: 10.1089/neu.2011.1913
Brunden, K. R., Ballatore, C., Lee, V. M., Smith, A. B., and Trojanowski, J. Q. (2012). Brain-penetrant microtubule-stabilizing compounds as potential therapeutic agents for tauopathies. Biochem. Soc. Trans. 40, 661–666. doi: 10.1523/JNEUROSCI.0780-09.2009
Brunden, K. R., Yao, Y., Potuzak, J. S., Ferrer, N. I., Ballatore, C., James, M. J., et al. (2011). The characterization of microtubule-stabilizing drugs as possible therapeutic agents for Alzheimer’s disease and related tauopathies. Pharmacol. Res. 63, 341–351. doi: 10.1016/j.phrs.2010.12.002
Brunden, K. R., Zhang, B., Carroll, J., Yao, Y., Potuzak, J. S., Hogan, A.-M., et al. (2010). Epothilone D improves microtubule density, axonal integrity, and cognition in a transgenic mouse model of tauopathy. J. Neurosci. 30, 13861–13866. doi: 10.1523/JNEUROSCI.3059-10.2010
Büki, A., and Povlishock, J. T. (2006). All roads lead to disconnection?–Traumatic axonal injury revisited. Acta Neurochir. 148, 181–193; discussion193–184. doi: 10.1007/s00701-005-0674-4
Campbell, J. N., Register, D., and Churn, S. B. (2012). Traumatic brain injury causes an FK506-sensitive loss and an overgrowth of dendritic spines in rat forebrain. J. Neurotrauma 29, 201–217. doi: 10.1089/neu.2011.1761
Carbonell, W. S., Maris, D. O., McCall, T., and Grady, M. S. (1998). Adaptation of the fluid percussion injury model to the mouse. J. Neurotrauma 15, 217–229. doi: 10.1089/neu.1998.15.217
Cartelli, D., Casagrande, F., Busceti, C. L., Bucci, D., Molinaro, G., Traficante, A., et al. (2013). Microtubule alterations occur early in experimental parkinsonism and the microtubule stabilizer epothilone D is neuroprotective. Sci. Rep. 3:1837. doi: 10.1038/srep01837
Chen, J. R., Wang, T. J., Wang, Y. J., and Tseng, G. F. (2010). The immediate large-scale dendritic plasticity of cortical pyramidal neurons subjected to acute epidural compression. Neuroscience 167, 414–427. doi: 10.1016/j.neuroscience.2010.02.028
Chen, J.-R., Wang, Y.-J., and Tseng, G.-F. (2003). The effect of epidural compression on cerebral cortex: a rat model. J. Neurotrauma 20, 767–780. doi: 10.1089/089771503767869999
Chen, Q. H., Ganesh, T., Brodie, P., Slebodnick, C., Jiang, Y., Banerjee, A., et al. (2008). Design, synthesis and biological evaluation of bridged epothilone D analogues. Org. Biomol. Chem. 6, 4542–4552. doi: 10.1039/b814823f
Chuckowree, J. A., and Vickers, J. C. (2003). Cytoskeletal and morphological alterations underlying axonal sprouting after localized transection of cortical neuron axons in vitro. J. Neurosci. 23, 3715–3725. doi: 10.1523/JNEUROSCI.23-09-03715.2003
Clark, J. A., Blizzard, C. A., Breslin, M. C., Yeaman, E. J., Lee, K. M., Chuckowree, J. A., et al. (2018). Epothilone D accelerates disease progression in the SOD1G93A mouse model of amyotrophic lateral sclerosis. Neuropathol. Appl. Neurobiol. doi: 10.1111/nan.12473 [Epub ahead of print].
Cross, D. J., Garwin, G. G., Cline, M. M., Richards, T. L., Yarnykh, V., Mourad, P. D., et al. (2015). Paclitaxel improves outcome from traumatic brain injury. Brain Res. 1618, 299–308. doi: 10.1016/j.brainres.2015.06.006
de Koning, M. E., Scheenen, M. E., van der Horn, H. J., Hageman, G., Roks, G., Spikman, J. M., et al. (2017). Non-hospitalized patients with mild traumatic brain injury: the forgotten minority. J. Neurotrauma 34, 257–261. doi: 10.1089/neu.2015.4377
Dean, P. J., and Sterr, A. (2013). Long-term effects of mild traumatic brain injury on cognitive performance. Front. Hum. Neurosci. 7:30. doi: 10.3389/fnhum.2013.00030
DeKosky, S. T., and Ikonomovic, M. D. (2010). Traumatic brain injury—football, warfare, and long-term effects. N. Engl. J. Med. 363, 1293–1296. doi: 10.1056/NEJMp1007051
Deller, T., Haas, C., Freiman, T., Phinney, A., Jucker, M., and Frotscher, M. (2006). Lesion-induced axonal sprouting in the central nervous system. Adv. Exp. Med. Biol. 557, 101–121. doi: 10.1007/0-387-30128-3_6
Dent, E. W. (2016). Of microtubules and memory: implications for microtubule dynamics in dendrites and spines. Mol. Biol. Cell 28, 1–8. doi: 10.1091/mbc.E15-11-0769
Dickson, T., Chung, R., McCormack, G., Staal, J., and Vickers, J. (2007). Acute reactive and regenerative changes in mature cortical axons following injury. Neuroreport 18, 283. doi: 10.1097/WNR.0b013e3280143cdb
Ekmark-Lewen, S., Flygt, J., Kiwanuka, O., Meyerson, B. J., Lewén, A., Hillered, L., et al. (2013). Traumatic axonal injury in the mouse is accompanied by a dynamic inflammatory response, astroglial reactivity and complex behavioral changes. J. Neuroinflammation 10:44. doi: 10.1186/1742-2094-10-44
Ertürk, A., Hellal, F., Enes, J., and Bradke, F. (2007). Disorganized microtubules underlie the formation of retraction bulbs and the failure of axonal regeneration. J. Neurosci. 27, 9169–9180. doi: 10.1523/JNEUROSCI.0612-07.2007
Farkas, O., and Povlishock, J. (2007). Cellular and subcellular change evoked by diffuse traumatic brain injury: a complex web of change extending far beyond focal damage. Prog. Brain Res. 161, 43–59. doi: 10.1016/S0079-6123(06)61004-2
Feng, G., Mellor, R. H., Bernstein, M., Keller-Peck, C., Nguyen, Q. T., Wallace, M., et al. (2000). Imaging neuronal subsets in transgenic mice expressing multiple spectral variants of GFP. Neuron 28, 41–51. doi: 10.1016/S0896-6273(00)00084-2
Fiala, J. C., Allwardt, B., and Harris, K. M. (2002a). Dendritic spines do not split during hippocampal LTP or maturation. Nat. Neurosci. 5, 297–298. doi: 10.1038/nn830
Fiala, J. C., Spacek, J., and Harris, K. M. (2002b). Dendritic spine pathology: cause or consequence of neurological disorders? Brain Res. Brain Res. Rev. 39, 29–54. doi: 10.1016/S0165-0173(02)00158-3
Gao, X., and Chen, J. (2011). Mild traumatic brain injury results in extensive neuronal degeneration in the cerebral cortex. J. Neuropathol. Exp. Neurol. 70, 183–191. doi: 10.1097/NEN.0b013e31820c6878
Gao, X., Deng, P., Xu, Z. C., and Chen, J. (2011). Moderate traumatic brain injury causes acute dendritic and synaptic degeneration in the hippocampal dentate gyrus. PLoS One 6:e24566. doi: 10.1371/journal.pone.0024566.s002
Goodin, S. (2004). Epothilones: mechanism of action and biologic activity. J. Clin. Oncol. 22, 2015–2025. doi: 10.1200/JCO.2004.12.001
Greer, J. E., Mcginn, M. J., and Povlishock, J. T. (2011). Diffuse traumatic axonal injury in the mouse induces atrophy, c-jun activation, and axonal outgrowth in the axotomized neuronal population. J. Neurosci. 31, 5089–5105. doi: 10.1523/JNEUROSCI.5103-10.2011
Gu, J., Firestein, B. L., and Zheng, J. Q. (2008). Microtubules in dendritic spine development. J. Neurosci. 28, 12120–12124. doi: 10.1523/JNEUROSCI.2509-08.2008
Hånell, A., Greer, J. E., Mcginn, M. J., and Povlishock, J. T. (2014). Traumatic brain injury-induced axonal phenotypes react differently to treatment. Acta Neuropathol. 129, 317–332. doi: 10.1007/s00401-014-1376-x
Hellal, F., Hurtado, A., Ruschel, J., Flynn, K. C., Laskowski, C. J., Umlauf, M., et al. (2011). microtubule stabilization reduces scarring and causes axon regeneration after spinal cord injury. Science 331, 928–931. doi: 10.1126/science.1201148
Hering, H., and Sheng, M. (2001). Dendritic spines: structure, dynamics and regulation. Nat. Rev. Neurosci. 2, 880–888. doi: 10.1038/35104061
Hill, C. S., Coleman, M. P., and Menon, D. K. (2016). Traumatic axonal injury: mechanisms and translational opportunities. Trends Neurosci. 39, 311–324. doi: 10.1016/j.tins.2016.03.002
Hotulainen, P., and Hoogenraad, C. C. (2010). Actin in dendritic spines: connecting dynamics to function. J. Cell Biol. 189, 619–629. doi: 10.1038/nn1630
Hur, E.-M., and Lee, B. D. (2014). Microtubule-targeting agents enter the Central Nervous System (CNS): double-edged swords for treating CNS injury and disease. Int. Neurourol. J. 18, 171–178. doi: 10.5213/inj.2014.18.4.171
Iverson, G. L. (2005). Outcome from mild traumatic brain injury. Curr. Opin. Psychiatry 18, 301–317. doi: 10.1097/01.yco.0000165601.29047.ae
Iverson, G. L. (2010). Clinical and methodological challenges with assessing mild traumatic brain injury in the military. J. Head Trauma Rehabil. 25, 313–319. doi: 10.1097/HTR.0b013e3181d6f9bd
Jagoda, A. S., Bazarian, J. J., Bruns, JJ Jr, Cantrill, S. V., Gean, A. D., Howard, P. K., et al. (2009). Clinical policy: neuroimaging and decisionmaking in adult mild traumatic brain injury in the acute setting. J. Emerg. Nurs. 35, e5–e40. doi: 10.1016/j.jen.2008.12.010
Jang, E.-H., Sim, A., Im, S.-K., and Hur, E.-M. (2016). Effects of microtubule stabilization by epothilone B depend on the type and age of neurons. Neural Plast. 2016:5056418. doi: 10.1155/2016/5056418
Jaworski, J., Kapitein, L. C., Gouveia, S. M., Dortland, B. R., Wulf, P. S., Grigoriev, I., et al. (2009). Dynamic microtubules regulate dendritic spine morphology and synaptic plasticity. Neuron 61, 85–100. doi: 10.1016/j.neuron.2008.11.013
Johnson, V. E., Stewart, W., and Smith, D. H. (2012a). Axonal pathology in traumatic brain injury. Exp. Neurol. 246, 35–43. doi: 10.1016/j.expneurol.2012.01.013
Johnson, V. E., Stewart, W., and Smith, D. H. (2012b). Widespread tau and amyloid-Beta pathology many years after a single traumatic brain injury in humans. Brain Pathol. 22, 142–149. doi: 10.1111/j.1750-3639.2011.00513.x
Kapitein, L. C., Yau, K. W., and Hoogenraad, C. C. (2010). Microtubule dynamics in dendritic spines. Methods Cell Biol. 97, 111–132. doi: 10.1016/S0091-679X(10)97007-6
Kharatishvili, I., Nissinen, J. P., McIntosh, T. K., and Pitkänen, A. (2006). A model of posttraumatic epilepsy induced by lateral fluid-percussion brain injury in rats. Neuroscience 140, 685–697. doi: 10.1016/j.neuroscience.2006.03.012
Khrapunovich-Baine, M., Menon, V., Yang, C.-P., Northcote, P. T., Miller, J. H., Angeletti, R. H., et al. (2011). Hallmarks of molecular action of microtubule stabilizing agents: effects of epothilone B, ixabepilone, peloruside A, and laulimalide on microtubule conformation. J. Biol. Chem. 286, 11765–11778. doi: 10.1074/jbc.M110.162214
Lei, W., Omotade, O. F., Myers, K. R., and Zheng, J. Q. (2016). Actin cytoskeleton in dendritic spine development and plasticity. Curr. Opin. Neurobiol. 39, 86–92. doi: 10.1016/j.conb.2016.04.010
Lifshitz, J., Witgen, B., and Grady, M. (2007). Acute cognitive impairment after lateral fluid percussion brain injury recovers by 1 month: evaluation by conditioned fear response. Behav. Brain Res. 177, 347–357. doi: 10.1016/j.bbr.2006.11.014
Matus, A. (2000). Actin-based plasticity in dendritic spines. Science 290, 754–758. doi: 10.1126/science.290.5492.754
Maxwell, W. L., and Graham, D. I. (1997). Loss of axonal microtubules and neurofilaments after stretch-injury to guinea pig optic nerve fibers. J. Neurotrauma 14, 603–614. doi: 10.1089/neu.1997.14.603
McMahon, P. J., Hricik, A., Yue, J. K., Puccio, A. M., Inoue, T., Lingsma, H. F., et al. (2014). Symptomatology and functional outcome in mild traumatic brain injury: results from the prospective TRACK-TBI study. J. Neurotrauma 31, 26–33. doi: 10.1089/neu.2013.2984
Meaney, D. F., and Smith, D. H. (2011). Biomechanics of concussion. Clin. Sports Med. 30, 19–31. doi: 10.1016/j.csm.2010.08.009
Michaud, L. B. (2009). The epothilones: how pharmacology relates to clinical utility. Ann. Pharmacother. 43, 1294–1309. doi: 10.1345/aph.1M005
Moscarello, M. A., Mak, B., Nguyen, T. A., Wood, D. D., Mastronardi, F., and Ludwin, S. K. (2002). Paclitaxel (Taxol) attenuates clinical disease in a spontaneously demyelinating transgenic mouse and induces remyelination. Mult. Scler. 8, 130–138. doi: 10.1191/1352458502ms776oa
Penazzi, L., Tackenberg, C., Ghori, A., Golovyashkina, N., Niewidok, B., Selle, K., et al. (2016). A&beta-mediated spine changes in the hippocampus are microtubule-dependent and can be reversed by a subnanomolar concentration of the microtubule-stabilizing agent epothilone D. Neuropharmacology 105, 84–95. doi: 10.1016/j.neuropharm.2016.01.002
Popovich, P. G., Tovar, C. A., Lemeshow, S., Yin, Q., and Jakeman, L. B. (2014). Independent evaluation of the anatomical and behavioral effects of Taxol in rat models of spinal cord injury. Exp. Neurol. 261, 97–108. doi: 10.1016/j.expneurol.2014.06.020
Reeves, T. M., Phillips, L. L., and Povlishock, J. T. (2005). Myelinated and unmyelinated axons of the corpus callosum differ in vulnerability and functional recovery following traumatic brain injury. Exp. Neurol. 196, 126–137. doi: 10.1016/j.expneurol.2005.07.014
Ruschel, J., Hellal, F., Flynn, K. C., Dupraz, S., Elliott, D. A., Tedeschi, A., et al. (2015). Systemic administration of epothilone B promotes axon regeneration after spinal cord injury. Science 348, 347–352. doi: 10.1126/science.aaa2958
Salin, P., Tseng, G., Hoffman, S., Parada, I., and Prince, D. (1995). Axonal sprouting in layer V pyramidal neurons of chronically injured cerebral cortex. J. Neurosci. 15, 8234–8245. doi: 10.1523/JNEUROSCI.15-12-08234.1995
Santhakumar, V., Ratzliff, A. D., Jeng, J., Toth, Z., and Soltesz, I. (2001). Long-term hyperexcitability in the hippocampus after experimental head trauma. Ann. Neurol. 50, 708–717. doi: 10.1002/ana.1230
Schneider, C. A., Rasband, W. S., and Eliceiri, K. W. (2012). NIH Image to ImageJ: 25 years of image analysis. Nat. Methods 9, 671–675. doi: 10.1038/nmeth.2089
Sengottuvel, V., Leibinger, M., Pfreimer, M., Andreadaki, A., and Fischer, D. (2011). Taxol facilitates axon regeneration in the mature CNS. J. Neurosci. 31, 2688–2699. doi: 10.1523/JNEUROSCI.4885-10.2011
Shultz, S. R., McDonald, S. J., Haar, C. V., Meconi, A., Vink, R., van Donkelaar, P., et al. (2016). The potential for animal models to provide insight into mild traumatic brain injury: translational challenges and strategies. Neurosci. Biobehav. Rev. 76(Pt B), 1–19. doi: 10.1016/j.neubiorev.2016.09.014
Smith, D., and Meaney, D. (2000). Axonal damage in traumatic brain injury. Neuroscientist 6, 483–495. doi: 10.1177/107385840000600611
Smith, D. H., Hicks, R., and Povlishock, J. T. (2013). Therapy development for diffuse axonal injury. J. Neurotrauma 30, 307–323. doi: 10.1089/neu.2012.2825
Spain, A., Daumas, S., Lifshitz, J., Rhodes, J., Andrews, P., Horsburgh, K., et al. (2010). Mild fluid percussion injury in mice produces evolving selective axonal pathology and cognitive deficits relevant to human brain injury. J. Neurotrauma 27, 1429–1438. doi: 10.1089/neu.2010.1288
Stone, J., Okonkwo, D., Dialo, A., Rubin, D., Mutlu, L., Povlishock, J., et al. (2004). Impaired axonal transport and altered axolemmal permeability occur in distinct populations of damaged axons following traumatic brain injury. Exp. Neurol. 190, 59–69. doi: 10.1016/j.expneurol.2004.05.022
Tang-Schomer, M. D., Johnson, V. E., Baas, P. W., Stewart, W., and Smith, D. H. (2012). Partial interruption of axonal transport due to microtubule breakage accounts for the formation of periodic varicosities after traumatic axonal injury. Exp. Neurol. 233, 364–372. doi: 10.1016/j.expneurol.2011.10.030
Thompson, H. J., Lifshitz, J., Marklund, N., Grady, M. S., Graham, D. I., Hovda, D. A., et al. (2005). Lateral fluid percussion brain injury: a 15-year review and evaluation. J. Neurotrauma 22, 42–75. doi: 10.1089/neu.2005.22.42
Wang, H.-C., and Ma, Y.-B. (2010). Experimental models of traumatic axonal injury. J. Clin. Neurosci. 17, 157–162. doi: 10.1016/j.jocn.2009.07.099
Wang, M. L., and Li, W. B. (2016). Cognitive impairment after traumatic brain injury: the role of MRI and possible pathological basis. J. Neurol. Sci. 370, 244–250. doi: 10.1016/j.jns.2016.09.049
Wilde, E. A., Li, X., Hunter, J. V., Narayana, P. A., Hasan, K., Biekman, B., et al. (2016). Loss of consciousness is related to white matter injury in mild traumatic brain injury. J. Neurotrauma 33, 2000–2010. doi: 10.1089/neu.2015.4212
Winston, C. N., Chellappa, D., Wilkins, T., Barton, D. J., Washington, P. M., Loane, D. J., et al. (2013). Controlled cortical impact results in an extensive loss of dendritic spines that is not mediated by injury-induced amyloid-beta accumulation. J. Neurotrauma 30, 1966–1972. doi: 10.1089/neu.2013.2960
Zhang, B., Maiti, A., Shively, S., Lakhani, F., McDonald-Jones, G., Bruce, J., et al. (2005). Microtubule-binding drugs offset tau sequestration by stabilizing microtubules and reversing fast axonal transport deficits in a tauopathy model. Proc. Natl. Acad. Sci. U.S.A. 102, 227–231. doi: 10.1073/pnas.0406361102
Keywords: traumatic brain injury, fluid percussion injury, neuroplasticity, microtubule stabilization, epothilone D, dendritic spine, cortical projection neuron, mushroom spine
Citation: Chuckowree JA, Zhu Z, Brizuela M, Lee KM, Blizzard CA and Dickson TC (2018) The Microtubule-Modulating Drug Epothilone D Alters Dendritic Spine Morphology in a Mouse Model of Mild Traumatic Brain Injury. Front. Cell. Neurosci. 12:223. doi: 10.3389/fncel.2018.00223
Received: 17 April 2018; Accepted: 09 July 2018;
Published: 30 July 2018.
Edited by:
Jesus Avila, Universidad Autónoma de Madrid, SpainReviewed by:
Kevin R. Jones, University of Colorado Boulder, United StatesJennifer Larimore, Agnes Scott College, United States
Copyright © 2018 Chuckowree, Zhu, Brizuela, Lee, Blizzard and Dickson. This is an open-access article distributed under the terms of the Creative Commons Attribution License (CC BY). The use, distribution or reproduction in other forums is permitted, provided the original author(s) and the copyright owner(s) are credited and that the original publication in this journal is cited, in accordance with accepted academic practice. No use, distribution or reproduction is permitted which does not comply with these terms.
*Correspondence: Tracey C. Dickson, VHJhY2V5LkRpY2tzb25AdXRhcy5lZHUuYXU=