- 1The Key Laboratory of Medicinal Resources and Natural Pharmaceutical Chemistry, National Engineering Laboratory for Resource Development of Endangered Crude Drugs in Northwest China, The Ministry of Education, College of Life Sciences, Shaanxi Normal University, Xi’an, China
- 2Department of Neurology, Thomas Jefferson University, Philadelphia, PA, United States
Oligodendrocyte progenitor cells (OPCs) are a subtype of glial cells responsible for myelin regeneration. Oligodendrocytes (OLGs) originate from OPCs and are the myelinating cells in the central nervous system (CNS). OLGs play an important role in the context of lesions in which myelin loss occurs. Even though many protocols for isolating OPCs have been published, their cellular yield remains a limit for clinical application. The protocol proposed here is novel and has practical value; in fact, OPCs can be generated from a source of autologous cells without gene manipulation. Our method represents a rapid, and high-efficiency differentiation protocol for generating mouse OLGs from bone marrow-derived cells using growth-factor defined media. With this protocol, it is possible to obtain mature OLGs in 7–8 weeks. Within 2–3 weeks from bone marrow (BM) isolation, after neurospheres formed, the cells differentiate into Nestin+ Sox2+ neural stem cells (NSCs), around 30 days. OPCs specific markers start to be expressed around day 38, followed by RIP+O4+ around day 42. CNPase+ mature OLGs are finally obtained around 7–8 weeks. Further, bone marrow-derived OPCs exhibited therapeutic effect in shiverer (Shi) mice, promoting myelin regeneration and reducing the tremor. Here, we propose a method by which OLGs can be generated starting from BM cells and have similar abilities to subventricular zone (SVZ)-derived cells. This protocol significantly decreases the timing and costs of the OLGs differentiation within 2 months of culture.
Introduction
Oligodendrocyte progenitor cells (OPCs) are present in both the white and gray matter of the adult central nervous system (CNS); upon oligodendrocytes (OLGs) injury, OPCs contribute to OLGs regeneration and remyelination (Duncan et al., 2018). OPCs are characterized by expression of platelet-derived growth factor receptor alpha (PDGFRα), neural/glial antigen 2 (NG2), and A2B5 (Dietrich et al., 2002; Zhu et al., 2008; Sim et al., 2011); they represent a high proliferative cell population resident in the CNS of adult mammals and humans (Mekhail et al., 2012; Clemente et al., 2013). OLGs derived from OPCs and are the myelinating cells of the CNS. Once OPCs differentiate into mature OLGs, they extend multiple processes that individually ensheath axons and then proceed to generate the concentric layers of the modified cell membrane that compose myelin (Goldman and Kuypers, 2015). Thanks to their remyelinating ability, several groups used OPCs transplantation as a therapeutic strategy for CNS diseases (Kim et al., 2012; Najm et al., 2013; Yang et al., 2013). Indeed, OPCs can be used as a safe treatment for spinal cord injury (Nori et al., 2018), stroke (Wang et al., 2018), Parkinson’s disease (Ahmed et al., 2013), and cerebral palsy (Rumajogee et al., 2018).
The transplantation of exogenous OPCs can represent a suitable treatment for chronic demyelinating disorders (Czepiel et al., 2015). The most common methods for obtaining functional OPCs, use mouse embryonic stem cell (ESCs) or pluripotent stem cells (Li et al., 2013; Wang et al., 2013; Kuai et al., 2015; Manley et al., 2017), or reprogrammed fibroblasts expressing a defined set of transcription factors (TFs) (Najm et al., 2013; Yang et al., 2013; Yamashita et al., 2017). Even if, OPCs transplantation has shown promising result in rodents (Goldman and Kuypers, 2015), its application remains far from the clinic. Several barriers should be overcome such as, whether OPCs can successfully migrate to the lesion (Foster et al., 1995), or their reprogramming can induce aberrant phenotype with consequent side-effects, as reported in some study in which next-generation sequencing was used (Liu et al., 2019). Other well-reported approaches used SVZ, from rodent CNS, as a source for isolating functional OPCs (Dincman et al., 2012; Zhu et al., 2014; Lu et al., 2015); however, due to the low cellular yield these methods have not guaranteed any feasibility for clinical application (Yang et al., 2010). Recently, it has been demonstrated that OPCs can be generated from bone marrow (BM) (Cristofanilli et al., 2011). These bone marrow-derived OPCs, induced with specific growth factor media, have shown similar morphology and cellular markers to canonical OPCs (Nazm Bojnordi et al., 2015).
We describe here a detailed protocol for generating OPCs and mature OLGs from autologous mouse bone marrow-derived neural stem cells (NSCs). Our method consists of two main steps. The first step provides the differentiation and generation of neurospheres from bone marrow-derived NSCs; the second one consists of neurospheres-derived OPCs differentiation within 1 week, using specific media. Respect to a previous report (Douvaras and Fossati, 2015), our protocol enables to produce autologous OLGs in 52 days.
Obtaining a high number of autologous OLGs is relevant to the clinic. For addressing this aspect, we tested the myelination ability of our bone marrow-derived OPCs, in a genetic model of congenital dysmyelination, shiverer (Shi) mice. Bone marrow-derived OPCs induced remyelination and significantly reduced tremor in treated mice. According to our data, this protocol is suitable for producing in large scale autologous OPCs, research aimed at understanding oligodendrocyte biology, and finally, it may represent a screening platform for myelinating compounds.
Materials and Methods
Animals
C57BL/6 (The Fourth Military Medical University), SJL, and C3H mouse strains (The Jackson laboratory) are all appropriate for this protocol. Mice were kept in clean cages with a maximum of 5 mice per cage, in a controlled environment with 12/12 h of light/dark cycles and food ad libitum throughout the experimental procedures. All experimental procedures and protocols are approved by the Animal Management and Committee of Shaanxi Normal University. At the same time, by the approved institutional guidelines and regulations.
BM-NSCs were given by injection of single cells suspension (2 × 105 cells in 20 μl PBS/each mouse) by intracerebroventricular (i.c.v.) injection, into 1 week-old Shi mice. PBS-treated age-sex- and strain-matched mice were used as controls. All groups of animals were observed for 35 days.
Preparation of Neural Stem Cell Proliferation Media (NSC-PM)
A total of 200 ml of NSC-PM media is prepared mixing 150 ml of DMEM/F12 with 4 ml of B27, 2 ml of Penicillin-streptomycin (50 U/ml), 2 ml of Non-essential amino acid solution (0.1 mM), 2 ml HEPES (10 mM), 2 ml of sodium pyruvate (1 mM; all purchased from Thermo Fisher Scientific), 40 μl of epidermal growth factor (EGF, 20 ng/ml, stock concentration: 100 μg/ml; PeproTech), and 40 μl of basic fibroblast growth factor (bFGF, 10 ng/ml, stock concentration: 50 μg/ml; PeproTech). DMEM/F12 is added to a final volume of 200 ml, filter with a bottle-top filter (0.22 μm) and store at 4°C. We suggest to use complete media within 2 weeks.
Preparation of OPC Proliferation Media (OPC-PM)
A total of 50 ml of OPC-PM is prepared mixing 30 ml of DMEM/F12 with 1 ml of B27, 0.5 ml of N2, 0.5 ml of GlutaMax (2 mM), 0.5 ml of Penicillin-streptomycin (50 U/ml), 0.5 ml of Non-essential amino acid solution (0.1 mM), 0.5 ml of sodium pyruvate (1 mM; all purchased from Thermo Fisher Scientific), 10 μl of platelet-derived growth factor-AA (PDGF-AA, 20 ng/ml, stock concentration: 100 μg/ml; PeproTech), and 20 μl of bFGF (20 ng/ml, stock concentration: 50 μg/ml; PeproTech). DMEM/F12 is added to a final volume of 50 ml, filter with a bottle-top filter (0.22 μm) and store at 4°C. We suggest to use complete media within 2 weeks.
Preparation of Early OLGs Differentiation Media (EOLG-DM)
A total of 25 ml of EOLG-DM is prepared mixing 15 ml of DMEM/F12 with 0.5 ml of B27, 0.25 ml of N2, 0.25 ml of GlutaMax (2 mM), 0.25 ml of Penicillin-streptomycin (50 U/ml), 0.25 ml of Non-essential amino acid solution (0.1 mM), 0.25 ml of sodium pyruvate (1 mM; all purchased from Thermo Fisher Scientific), 50 μl of triiodo-l-thyronine (T3, 40 ng/ml, stock concentration: 20 μg/ml; Sigma-Aldrich), 20 μl of sonic hedgehog (Shh) (40 ng/ml stock, concentration: 50 μg/ml), 20 μl of Noggin (40 ng/ml, stock concentration: 50 μg/ml), 5 μl of insulin-like growth factor (IGF, 100 ng/ml, stock concentration: 500 μg/ml; all purchased from PeproTech), and 25 μl of neurotrophin 3 (NT-3, 10 ng/ml; Sigma-Aldrich). DMEM/F12 is added to a final volume of 25 ml, filter with a bottle-top filter (0.22 μm) and store at 4°C. We suggest to use complete media within 1 week.
Preparation of Late OLGs Differentiation Media (LOLG-DM)
A total of 10 ml of LOLG-DM is prepared mixing 10 ml of EOLG-DM with 5 μl of 3′,5′-cyclic adenosine monophosphate (cAMP, 50 μM, stock concentration: 50 μg/ml; Sigma-Aldrich). The fresh media should be using within 1 week. For preparing Poly-D-lysine coating solution, stock at 0.1 % (wt/vol) 50 mL of sterile tissue culture grade water to 5 mg of poly-D-lysine (Sigma-Aldrich). Poly-D-lysine solution can be stored at 4°C for 3 months. Coating culture plates with the poly-D-lysine coating solution (0.5 ml per well for 24 well plate) and keep them for 2 h in 37°C incubator or overnight at 4°C. Remove coating solution and wash three times with sterile ddH2O. After poly-D-lysine coated, add 1 μg/ml laminin (Sigma-Aldrich) solution for 2 h in 37°C incubator or overnight at 4°C. Laminin should be slowly thawed at 2–8°C for avoiding any solidification process. Dilute in a balanced salt solution and coat culture surface with a minimal volume. Remove coating solution, wash three times with sterile ddH2O and dry completely in a tissue culture hood. Allow to air dry at least 5 min before introducing cells and media. Poly-D-lysine and laminin-coated coverslips: Place sterilized coverslips into the wells of a 24 well plate. The procedure followed the Poly-D-lysine coating and Laminin coating. The coverslips will float on the surface of the solution. Make sure the coverslips are completely immersed in the coating buffer to ensure the coverslips are well coated.
Isolation and Culture of NSCs From Murine SVZ and Bone Marrow
SVZ-derived NSCs were isolated from adult C57BL/6 mice as described previously with minor modification (Yang et al., 2009). Briefly, C57BL/6 mice 8 weeks old were euthanized and the SVZ region was harvested under sterile conditions and placed in DMEM media. After a brief washing with DMEM media, tissues were cut into 1 mm3 pieces and digested by neural tissue dissociation kits (Miltenyi Biotec). The cells were suspended in serum-free DMEM/F12 (Invitrogen) supplied with 2% B27 supplements (Invitrogen), 20 ng/ml EGF (Peprotech) and 10 ng/ml bFGF (Peprotech), along with 100 IU/ml penicillin and 100 μg/ml Streptomycin (Sigma).
Bone marrow cells were isolated according to a previously described protocol (Swamydas and Lionakis, 2013). Finally, the cells were suspended in NSC-PM media at 106 cells/ml, and plated on poly-D-lysine, and laminin coated 24 well plate for 4 days. We suggest to replace half of the media with fresh NSC-PM media every 4 days. The media should be changed softly and slowly to avoid lifting the adhered NSCs (This is the critical step).
The formation of cellular clusters that resemble neurospheres becomes evident after 10–14 days. For cell detachment, aspirate the media, add 500 μl accutase (Thermo Fisher Scientific), and incubate at room temperature (22–25°C) for 2 min. Use 200 μl pipette to carefully suspend the adhered cell and transfer to a 15 ml conical tube. Shake the tube 10 times/min for total 3 min and add 4.5 ml DPBS for centrifuge (300 ×g, 10 min). Next, suspend the cell pellet in NSC-PM media at 1 × 105 cells/ml, and plate the cell in non-coated 6 cm plate. After 4 days, the neurospheres can observe under the microscope. Collect suspended neurospheres from culture dish and transfer to a 15 ml conical tube. Centrifuge the cell at 100 ×g for 1 min. Gently aspirate media leaving the neurospheres at the bottom of tube. Resuspend neurospheres in 5 ml DPBS and centrifuge the cell at 100 × g for 1 min to remove the media residues. Gently aspirate media leaving the neurospheres at the bottom of tube and add 1 ml of accutase to the cell culture and incubate 5 min at room temperature. Mildly shake the tube 10 times/min. If any neurosphere remains visible, after 4 min accutase incubation, we suggest to pipette gentle up and down until all the visible neurospheres are in a single cell suspension. The accutase media will change the color from carnation to oyster white. Then, add 4 ml of fresh media to the tube and centrifuge the cells at 200 ×g for 4 min. Gently aspirate the supernatant and resuspend cells in fresh media. Transfer the cell 1 × 105 cells/ml to a new culture dish and incubate at 37°C for NSCs expanded. Cells can be frozen at this step. For visualization of results, seed the cell at 1 × 105 cells/ml in a poly-D-lysine and laminin coated 24 well plate with coverslips. Cells were fixed using 4% paraformaldehyde for immunocytochemical staining.
Bone Marrow-Derived NSCs Differentiation and Proliferation
To test the proliferation capacity of bone marrow-derived NSCs, growth curve of bone marrow-derived NSCs was determined followed previous method (Yang et al., 2010). Briefly, the newly formed neurospheres were digested to the single cells and plated at a density of 1.0 × 105 cell/ml and cultured in NSC-PM. At days 6, 11, 16 and 21, neurospheres in each well were digested into single cells; cell numbers were counted by hemocytometer. SVZ derived NSCs were used in the parallel experiment to compare the proliferate ratio.
To evaluate the differentiation ability of bone marrow-derived NSCs, single cells were plated on poly-D-lysine/laminin coated coverslip at a density of 1.0 × 104 cells/ml and cultured in specific NSCs differentiation media (Li et al., 2016). In brief, for neuron differentiation, Neurobasal media was supplemented with 2% B27, 2 mM GlutaMax-I and 0.5 mM cAMP. For astrocyte differentiation, DMEM was supplemented with 1% N2, 2 mM GlutaMax-I and 1% FBS. The oligodendrocyte differentiation media requires Neurobasal media supplemented with 2% B27, 2 mM GlutaMax-I and 20 ng/ml T3. Over 2 weeks, NSCs in differentiation media changed morphology and developed markers of neurons, astrocytes, and OLGs as determined by immunocytochemistry staining. To determine the number of cells expressing a specific antigen, five areas of each coverslip were examined, and the percentage of positive cells labeled for a specific neural marker in the total number of DAPI+ cells was expressed as the mean value of specific neural differentiation.
OPCs Differentiation
Once neurospheres reach 100–250 μm of diameter, dissociate the neurospheres with accutase. Start by stepping suspend the cell pellet in NSC-PM media at 1 × 105 cells/ml until transfer the cell 1 × 105 cells/ml to a new culture dish and incubate at 37°C for NSCs expanded to the end. Finally, The cells are seeded on the poly-D-lysine, and laminin coated plate at 5 × 103 cells/ml in the NSC-PM media. After 1–2 days, over 90% cells will be adhered to the bottom, change the media to OPC-PM. Incubate the plate in a 37°C, 5% CO2 incubator and perform media changes every 2 days for 8 days. Cells can be frozen at this step.
OLGs Maturation
At day 9, aspirate the OPC-PM media, gently rinse with DPBS to remove the growth factor (i.e., PDGF-AA and bFGF), and add fresh EOLG-DM (500 μl/well/24 well plate) for inducing differentiation of OLGs. OPCs will proliferate few times. If the cells reach 90% confluency, it might be necessary to split the cells at a 1:4–6 ratio. After 4 days, the media is changed to LOLG-DM. Adding cAMP will accelerate the branch outgrowth for OLGs differentiation. Repeat these steps for 3 times, after 2 weeks.
Immunofluorescence Staining
For immunocytochemistry, cells were fixed in 4% paraformaldehyde at room temperature for 30 min. Then, incubate with 0.3% TritonX-100 (in PBS) for 15 min at room temperature and wash with PBS. The primary antibody is diluted to a suitable concentration with a blocking solution (10% horse serum in PBS). The following primary antibodies were used: anti-A2B5 (MA1-90445, Thermo Fisher Scientific), anti-CNPase (ab44289, Abcam), anti-Ki67 (ab15580, Abcam), anti-Nestin (MAB353, Milipore), anti-NG2 (MAB5384, Milipore), anti-O4 (NL1326V, R&D), anti-RIP (ab72139, Abcam), and anti-SOX2 (ab97959, Abcam). Primary antibodies were washed out with PBS three times after overnight incubation at 4°C. The corresponding Alexa Fluor 488/594 conjugated secondary antibodies (both from Jackson ImmunoResearch Laboratories) were used for 2 h at room temperature. After wash, the ProLongTM Gold Antifade Mountant with DAPI buffer (P36931, Thermo Fisher Scientific) were used for mounting slides. Results were visualized by fluorescent microscopy (Nikon Eclipse E600; Nikon, Melville, NY, United States). Quantitative image analysis was performed using ImagePro (Media Cybernetics).
Bone Marrow NSC-Derived OPCs Transplantation Into Shi Mice
Newborn double-homozygous Shi (MbpShi/MbpShi) mice (The Jackson Laboratory, Bar Harbor, ME, United States) were injected with dissociated single OPCs. The protocol followed previous work with minor modifications (Zhang et al., 2019). Briefly, to generate the lentivirus expression of mCherry protein, the backbone of pCDH-CMV-MCS-EF1-copGFP (System Biosciences) was used. Subsequently, the copGFP was replaced by mCherry and puromycin was inserted into the multiple clone sites. The positive plasmid was confirmed by sequencing and using for lentivirus packaging. OPCs were transfected with lentiviral expressed mcherry, and positive cells were selected by puromycin. Then, mcherry OPCs+ were transplanted bilaterally in the corpus callosum of 1 week-old Shi mice (2.0 × 105 cell/mice). PBS-treated age-sex-, strain-matched mice, and sham control mice were used. After 5 weeks from the treatment, mice were anesthetized, and brains were isolated and fixed with 4% paraformaldehyde and cryoprotected using 30% sucrose. CNS coronal sections were stained with myelin basic protein (MBP) antibody (ab40390, Abcam) for evaluating the differentiation process of mcherry OPCs+. Tremor (Trembling time/total time) was analyzed for evaluating disease development. Kaplan-Meier analysis was used to assess the survival rate as described (Shinkai et al., 1992).
Statistical Analysis
Statistical analyses were performed using GraphPad Prism software (GraphPad, La Jolla, CA, United States). Data are presented as mean ± SD. Experiments with 2 groups were tested for statistical significance using unpaired, 2-tailed Student’s t-tests. When comparing two groups at different time points, data were analyzed by two-way analysis of variance (ANOVA) with Tukey’s multiple comparisons test. Comparisons between two groups were carried out with Student’s t-test. Values of p < 0.05 were considered significant.
Results
Timeline of Bone Marrow-Derived Mature Oligodendrocyte Differentiation
The timeline of our protocol is shown in Figure 1. In the first step, NSCs are differentiated from BM cells. After BM isolation, the cells are seeded at high density (5 × 105 cell per cm2) on poly-D-lysine/laminin coated 24-well plate. In vitro, the presence EGF and fibroblast growth factor-2 (FGF2) mimic the NSCs culture environment, inducing NSCs proliferation. In the second stage, NSCs are induced to become OPCs. Here, the adherent cultures are dissociated to form spheres in suspension. Although Sox2+Nestin+ cells can also form spheres, the presence of recombinant protein PDGFα will gradually enrich the NG2+A2B5+ cell population (Neri et al., 2010). The final stage is the transition from OPCs to mature OLGs, by exposing the cells to T3, NT-3, Noggin, Shh, and IGF (Najm et al., 2013; Douvaras and Fossati, 2015).
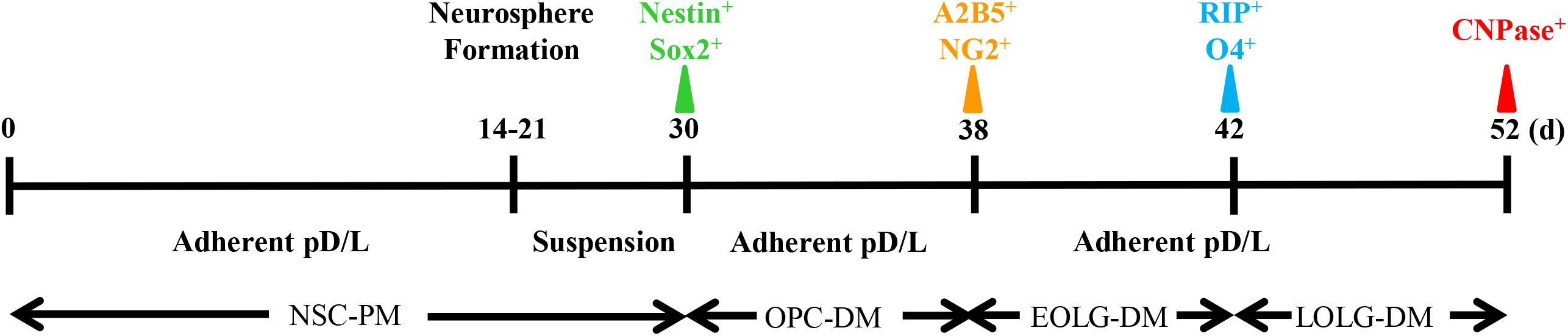
Figure 1. Timeline of oligodendrocyte differentiation. Multicolor triangles represent recommended time points for evaluating the expression of stage-specific markers through immunofluorescence.
Neurosphere Differentiation Step
For NSCs generation, BM from autologous adult mice can efficiency generate neurospheres. During neurosphere differentiation step, we are usually able to observe three stages (Figure 2A). (1) After 1 week in culture, individual cells exhibit high proliferative ability. (2) Two weeks later cells form neurospheres. (3) At 3–4 weeks, the neurospheres increased in size and gradually detached from the bottom of the culture plate. To characterize the bone marrow-derived NSCs, we compared the proliferative capacity with the SVZ-derived NSCs. We collected the neurosphere, dissociated, and re-plated at 1.0 × 105 cell/ml for a next round cell expansion. We next compared the proliferation capacity of bone marrow- and SVZ-NSCs. BM and SVZ-NSCs from passages 2 and 5 were seeded and cultured in NSC-PM. At day 6, 11, 16, and 21, the neurospheres formed and re-dissociated to single cells, and the cell number were counted by hemocytometer. The proliferation rate of BM-NSCs was significantly slower than SVZ-NSC at the early stage, whereas there was no obviously difference in proliferation capacity at passage 5 (Figure 2B). To identify the BM-NSCs, single cells were transferred onto poly-D-lysine and laminin pre-coated coverslips and used for immunostaining with Sox2 and Nestin. We can observe over 95% cells are Sox2 and Nestin-positive cells at passage 5 (Figure 2C).
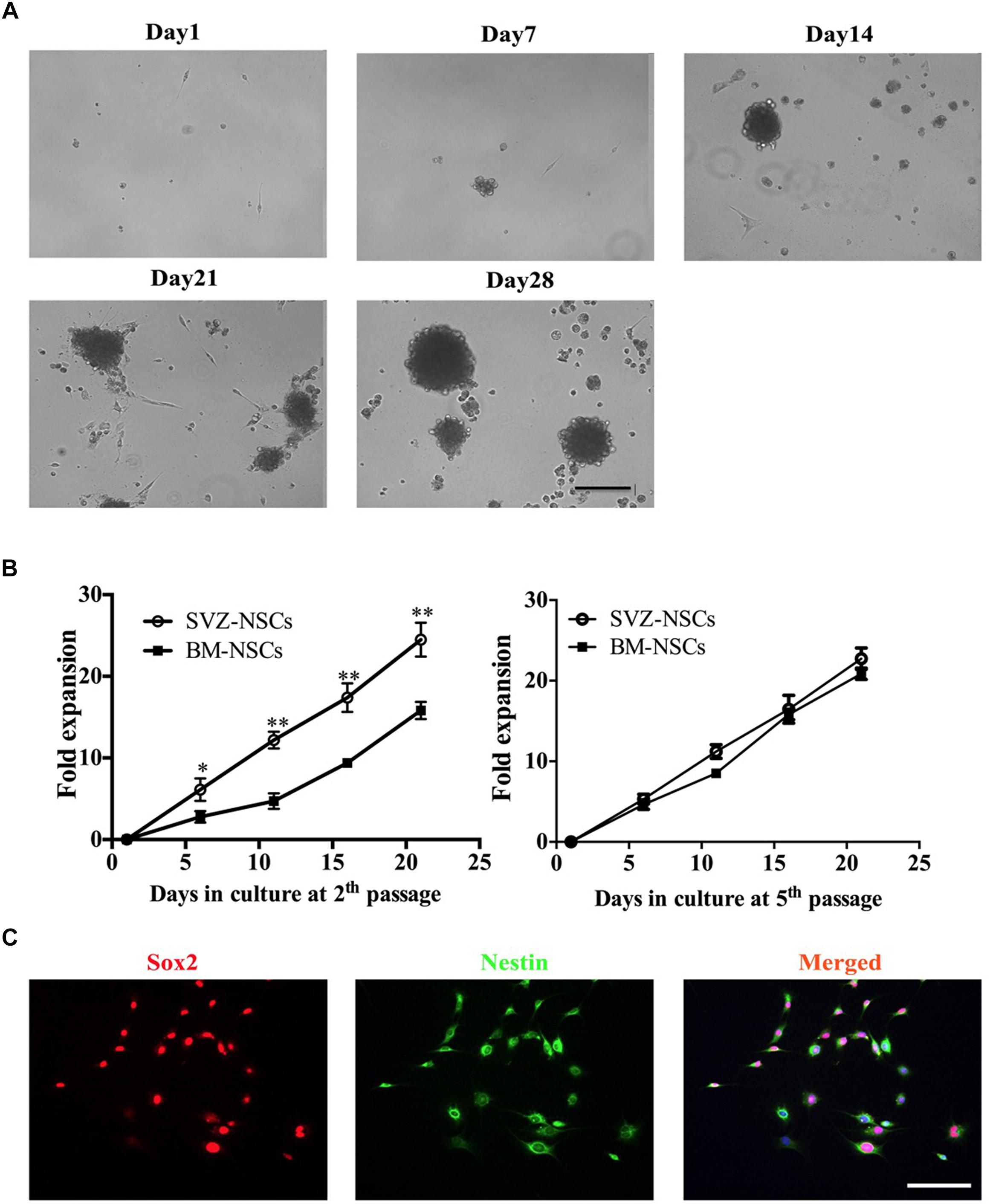
Figure 2. Generation of bone marrow-derived NSCs in vitro. BM-NSCs were isolated and expanded from bone marrow of adult C57BL/6 mice and cultured in DMEM/F12 containing 20 ng/ml EGF, 20 ng/ml Bfgf, and 2% B27 supplements. (A) Single cells were seeded in poly-D-lysine- and laminin-coated plates. After 7 days, the cells showed obvious proliferation. After 14 days, the NSCs gradually formed neurospheres. After 21–28 days, neurospheres increased size, and detached from the bottom of the culture plate. (B) Growth curves of BM-NSCs and SVZ-NSCs. Single cells at the second and fifth passages were seeded at a density of 1.0 × 105 cells/ml and cultured in proliferation media. At days 6, 11, 16 and 21, neurospheres in each well were digested into single cells; cell numbers were counted by hemocytometer. At least 5 wells were evaluated at each time point. Data represent the mean ± SD from three repeated experiments from separately generated cultures, ∗p < 0.05, ∗∗p < 0.01, as determined by two-way ANOVA with Tukey’s multiple comparison test. (C) Neurospheres dissociated with accutase and plated in the coated coverslips were immunostained for NSC markers Sox2 and Nestin. Scale bar = 50 μm.
Differentiation Potential of BM-NSCs
To test the differentiation potential of BM-NSCs in vitro, neurospheres were used and cultured in specific neuron/astrocyte/oligodendrocyte differentiation media. After 2 weeks, BM-NSCs, plated in presence of differentiation media, changed morphology and developed markers of neuron, astrocytes, and OLGs, as shown by immunocytochemistry staining. We compared the differentiation capacity of BM-NSCs and SVZ-NSCs. There are no significant difference about the percentage of CNPase+, Tuj1+, and GFAP+ cells between BM-NSCs and SVZ-NSCs. These results indicated that BM-NSCs can differentiate into neural cell lineages (Figure 3).
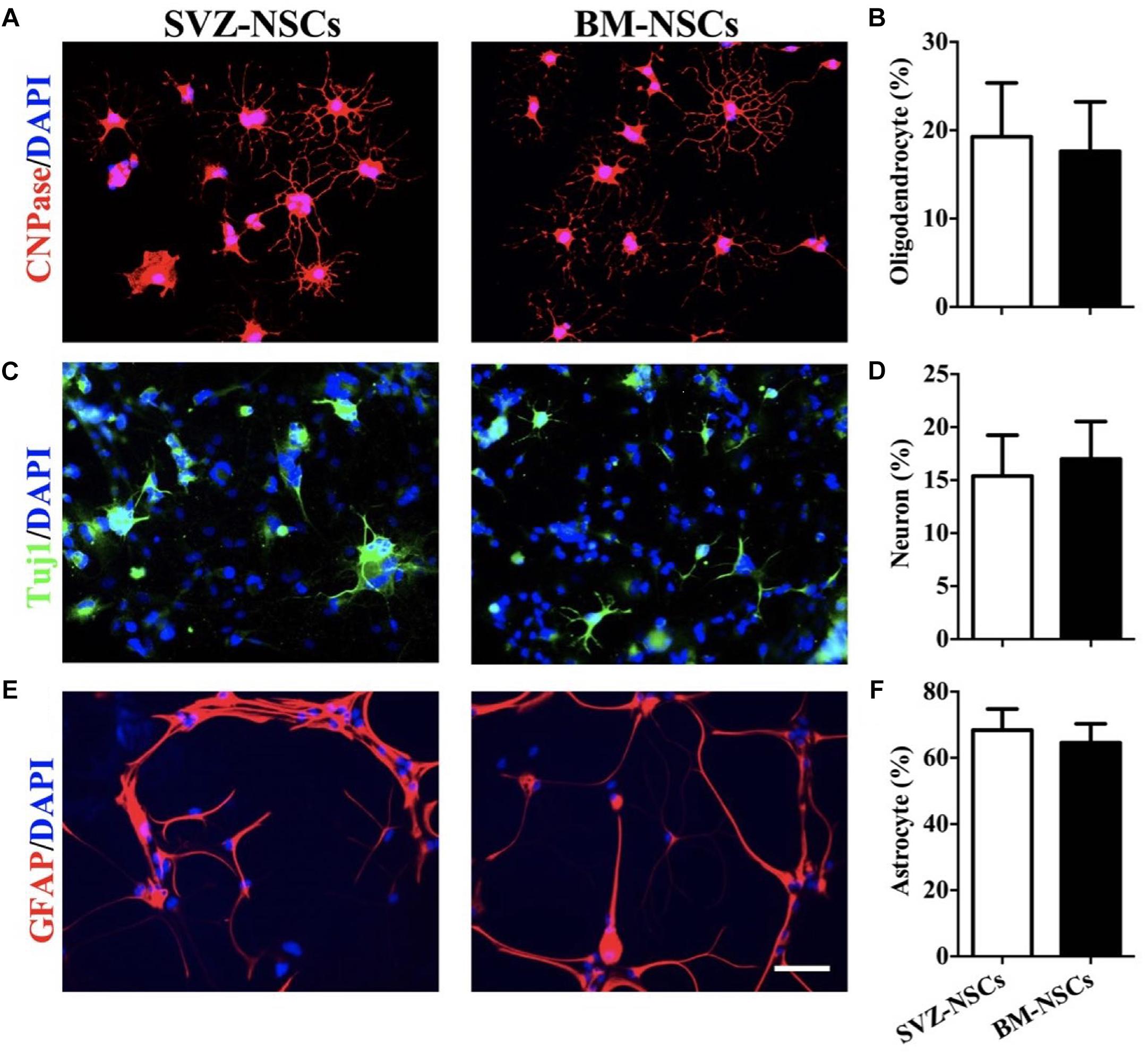
Figure 3. BM-NSCs differentiation in vitro. Examples of NSCs that differentiated into (A) OLGs (CNPase+), (C) neuron (Tuj1+), and (E) astrocytes (GFAP+), after 1 week with specific differentiation media. Scale bar = 50 μm. (B,D,F) Quantitative analysis of differentiated cells. Data are shown as mean values ± SD (n = 5 each group) and are representative of three experiments. Significance difference was analyzed by Student’s t-test.
OPCs Differentiation and Oligodendrocyte Maturation Process
To assess whether the enrichment of OPCs obtained by differentiation media could be exploited for increasing the yield of mature OLGs, OPCs grown in EOLG-DM media for 4 days were shift to LOLG-DM media for additional 10 days. Cells grown for 14 days in OPC-PM media were used as control. Cells were then analyzed for the presence of A2B5+, NG2+, RIP+, O4+, and CNPase+ cells. For OPCs differentiation, single NSCs were seed on poly-D-lysine, and laminin coated plates or coverslips in the OPCs differentiation media. Cells exhibited strong proliferation ability and grew faster under the proliferation condition (Figure 4A). These OPCs expressed A2B5 and NG2, as shown by immunostaining (Figures 4B,C). Moreover, OPC-spheres can be cultured with OPCs media without differentiation. They can further form immature OLGs (e.g., RIP+ and O4+) when are grown in OLGs differentiation media for 1 week (Figures 4D,E).
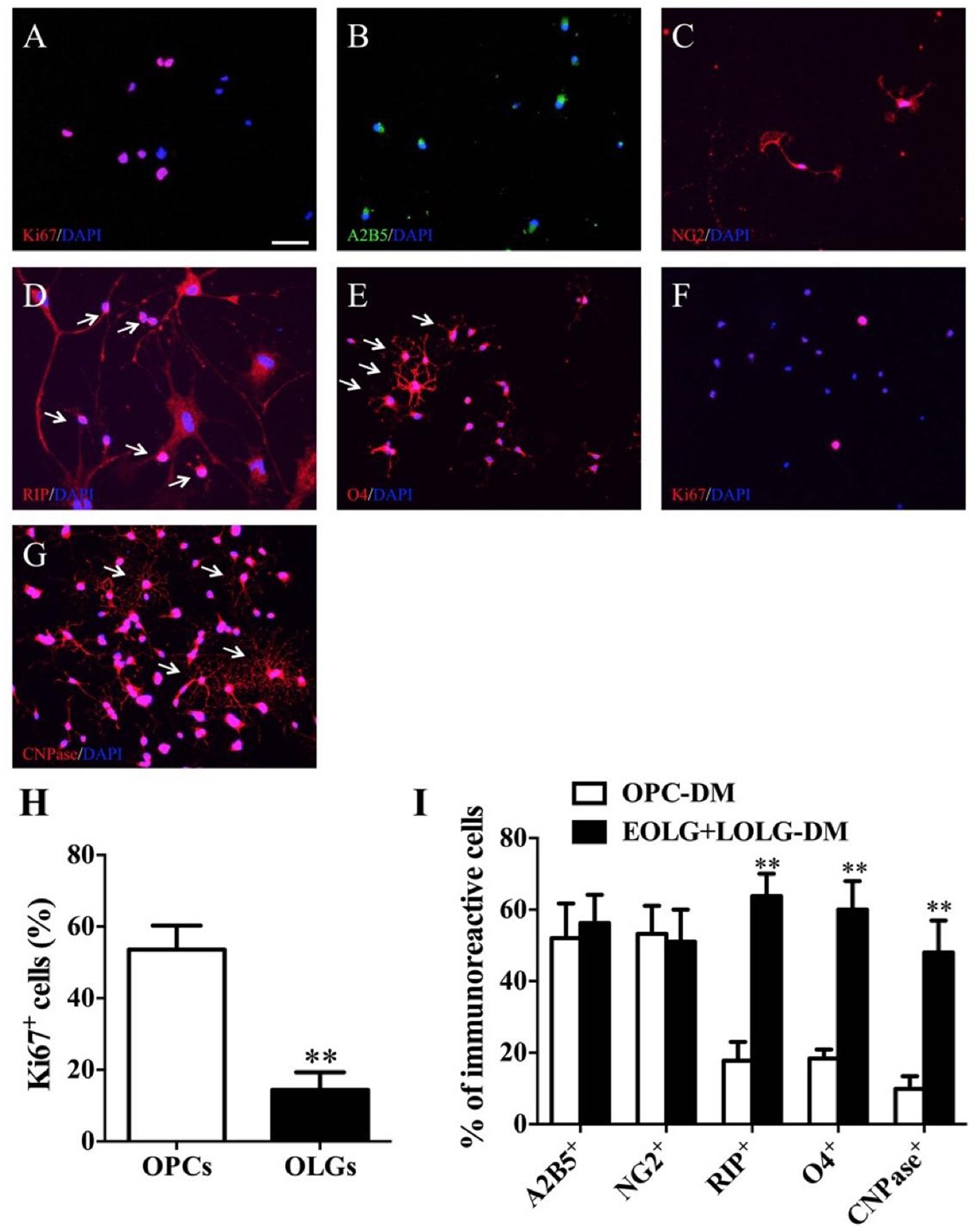
Figure 4. Fundamental steps of BM-NSCs OLGs differentiation. (A) Expression of Ki-67 at day 38, as shown by immunofluorescence analysis. (B,C) Immunofluorescence staining of progenitor cells at day 38, expressing A2B5, and NG2. (D,E) RIP and O4 staining show cells ramified morphology. (F) Expression of Ki-67 which in late stage of OLGs differentiation (day 52). (G) At the end of differentiation stage, CNPase+ OLGs show typical ramified morphology. (H) OPCs and OLGs proliferation (A,F) was quantified as percentage of Ki67-positive cells. Scale bar = 50 μm. (I) The percentages of A2B5+, NG2+, RIP+, O4+, and CNPase+ cells are present in EOLG+LOLG-DM cultures as compared to OPC-PM cultures. Data are expressed as the mean ± SD, three independent experiments, ∗∗p < 0.01, Student’s t-test.
For OLGs maturation, OPCs were grown in presence of oligodendrocyte differentiation media. Cells gradually stopped to proliferate (Ki67+) (Figures 4F,H) and started to differentiate into mature OLGs (CNPase+). About 50 % of the CNPase+ OLGs exhibited complex membrane morphology indicating OPCs differentiation into mature OLGs successfully (Figures 4G,I).
OPCs and Mature OLGs Generation From Autologous Mouse Bone Marrow-Derived NSCs
Our method allows to isolate OPCs, using a selective detachment procedure, generate mature OLGs in vitro (Figure 5A), and induce remyelination after transplantation into the dysmyelinated Shi mice (Figure 5B). Although immunofluorescence analysis showed abundant MBP+mCherry+ cells, the transplanted cells were more like “non-completedly myelinating” cells. We hypothesized that these cells would become mature at later time points and express more MBP+ myelin internodes. To define whether our BM-NSCs had the potential to form functional OLGs in vivo, the tremor and survival rate were test in the subsequent experiment. After OPCs transplantation, the tremor was significantly decreased compared the untreated Shi mice (Figure 5C and Supplementary Videos 1, 2). As shown in Figure 5D, the survival rate of mice transplanted with OPCs was increased, with a range of 106–135 days. All PBS-treated Shi mice died, over a range of 90–125 days postnatally. These results indicated that BM-OPCs could successfully differentiate into functionally OLGs, induce remyelination, and increase survival rate in Shi mice. The experimental design overview for this protocol is shown in Figure 6.
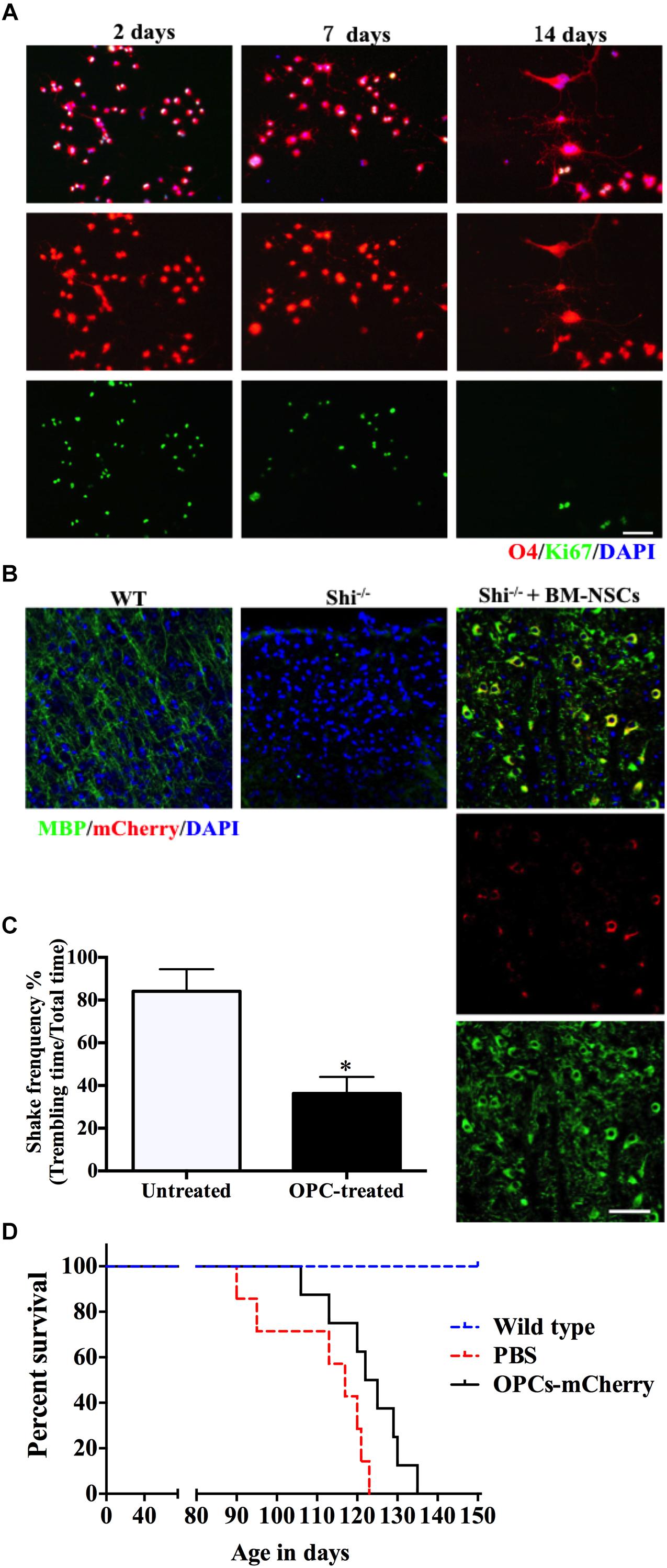
Figure 5. BM-NSCs OLGs differentiation in vitro and transplantation in Shi mice. (A) BM-NSCs were cultured in oligodendrocyte differentiation media for 48 h, 1 week, and 2 weeks for O4 (differentiation marker) and Ki-67 (proliferation marker) staining. (B) Fluorescence staining of BM-NSCs (mCherry+ cells), after 35 days post injection, show myelination (MBP+) in the contralateral striatum. Scale bar = 50 μm. (C) Quantification of tremor of Shi mice treated with OPCs. (D) Transplanted OPCs prolong the survival of Shi mice compared with PBS-treated (n = 9). Symbols represent mean ± SD. ∗p < 0.05, Student’s t-test.
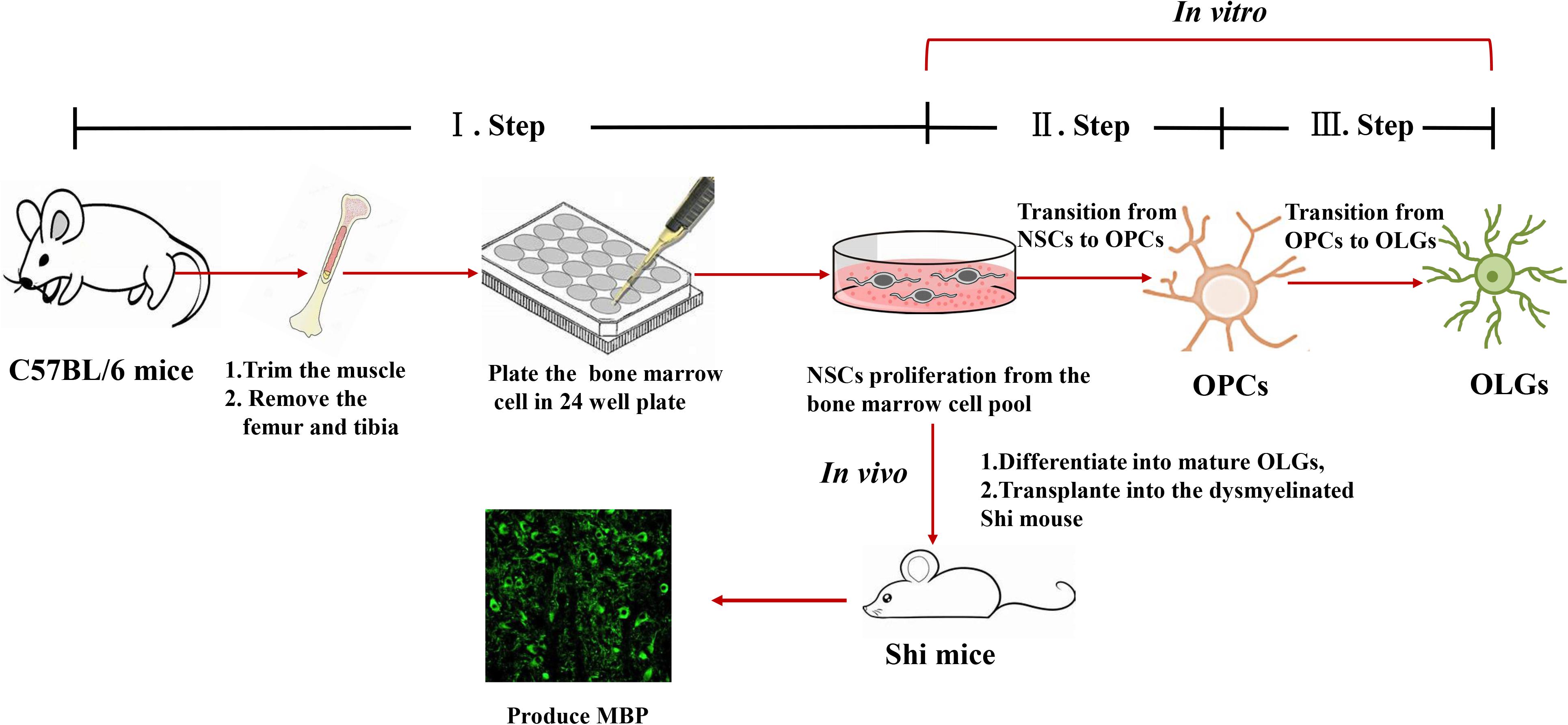
Figure 6. Representative scheme of the protocol for generating OPCs and OLGs from autologous mouse bone marrow-derived NSCs.
Discussion
Oligodendrocyte progenitor cells can be isolated from the SVZ of adult mammalian and expanded in vitro for producing mature OLGs (Chen et al., 2007). However, with this method, a little number of mature OLGs can be obtained. Recent studies showed that it is possible to differentiate OPCs from BM cells (Yang et al., 2010; Nazm Bojnordi et al., 2015). Indeed, bone marrow-derived OPCs showed distinct neural cell markers and morphology (Abbaszadeh et al., 2013). The possibility to get a high number of mature OLGs, starting from bone marrow, represents an accessible, and alternative manner respect to canonical methods in which OPCs are generated from SVZ-derived cells. OPCs transplantation either intravenous (i.v.) or i.c.v. has shown to promote remyelination in vivo (Sher et al., 2012; Wu et al., 2012); thus its therapeutic potential is relevant for demyelination disorders.
Currently, there are several methods for obtaining functional OLGs (Garcia-Leon and Verfaillie, 2016). Nazm Bojnordi et al. (2015) used BM stromal cells for producing functional Olig2 and O4+ cells. These OPCs successfully promoted the remyelination, in LPS-induced demyelination model; however, the efficiency was unclear. Further, OPCs isolation from ESCs or induced pluripotent stem cells (iPSCs), is an effective way for repairing and preventing the progression of demyelinating lesions (Douvaras et al., 2014; Thiruvalluvan et al., 2016). However, ESCs-derived OPCs may lack of stability and safety. It has been shown that NSCs possess less risk of potential tumorigenicity than ESCs or iPSCs (Zhao et al., 2015). The generation of OPCs from BM is consistent with what demonstrated so far (Yang et al., 2010; Hojjat-Allah et al., 2013; Nazm Bojnordi et al., 2015). Our method mainly consists of three steps, bone marrow-derived NSCs generation, NSCs-OPCs differentiation, and OLGs maturation. These data also focused on the derivation of OLGs from OPCs using assorted agents. In all of these steps, we use conditioned-media containing essential growth factors such as T3, Shh, IGF, and NT-3. We, also, use Noggin for negatively regulating bone morphogenetic protein (BMP) pathway, which blocked oligodendrocyte maturation (Najm et al., 2013). Although this protocol does not significantly improve the differentiation efficiency, compared with previous work, the timing is shorter (from 75 to 95 and 52 days) (Chen et al., 2007; Douvaras and Fossati, 2015). Our findings provide an alternative, fast strategy for obtaining functional OLGs from BM cells after just 40–50 days of differentiation. Here, we use the congenital dysmyelination model of Shi mice to show that BM-OPCs improved the mice survival. Our protocol can generate OPCs from BM-NSCs providing sufficient cell sources from autologous and inducing remyelination.
One generally OPCs generating strategies is that the cell need transduction of several TFs by lentiviral (Han et al., 2012; Najm et al., 2013). Virus-mediated gene delivery required higher biosafety levels and still need to be considered for clinical application (Capetian et al., 2016). In our protocol, we choose a well-established, and effective custom differentiation media system (Najm et al., 2013; Baskin et al., 2016). These differentiation media contain several growth factors (EGF, FGF, PDGF, Shh, Noggin, IGF, and NT-3) which used in different proliferation or differentiation stage were sufficient for inducing cell differentiation into mature OLGs. The manner of using growth factor is safer than viral or small molecules to treat cell or neurodegeneration disease (Tuszynski et al., 2015; De et al., 2017). The specificity of growth factors to recognize its target is better than small molecules especially in high dose (such as concentration > 10 μM) (Weiss et al., 2007). Also, when withdrawing the growth factors such as FGF, EGF et al., cell will cease to proliferate and avoid the risk of formation tumor cell. In a previous report, T3 was used as an inducer to differentiate into oligodendrocyte-like cells using autologous BM stromal cells (Kaka et al., 2012; Hojjat-Allah et al., 2013). Compared with their researches, we have demonstrated that mouse OLGs can be produced from bone marrow-derived cells in vitro, indicating that our method is more reliable. Together, this protocol indicates that our bone marrow-derived OPCs represent a simple, safe, valuable and promising cell-based therapy strategy for myelin repair and neurodegeneration disease.
Ethics Statement
All experimental procedures and protocols are approved by the Animal Management and Committee of Shaanxi Normal University. At the same time, in accordance with the approved institutional guidelines and regulations.
Author Contributions
YZ and XL conceived and designed the experiments. YZ, X-YL, JT, J-JH, and Z-QY carried out the experiments. TY, L-YJ, and XL analyzed the data. YZ, X-YL, GC, JT, and XL wrote the manuscript. AR and XL supervised the study and revised the manuscript. All authors read and approved the final manuscript.
Funding
This study was supported by the Chinese National Natural Science Foundation (Grant Nos. 81771345 and 81501062), the Natural Science Foundation of Shaanxi Province, China (Grant Nos. 2018JZ3001, 2018JQ8033, and 2017043) and the Fundamental Research Funds for the Central Universities (Grant Nos. GK201903062, GK20182010, GK201701009, 2018CSLZ018, 2018CSLZ019, and 2018CSLZ020).
Conflict of Interest Statement
The authors declare that the research was conducted in the absence of any commercial or financial relationships that could be construed as a potential conflict of interest.
Supplementary Material
The Supplementary Material for this article can be found online at: https://www.frontiersin.org/articles/10.3389/fncel.2019.00247/full#supplementary-material
References
Abbaszadeh, H. A., Tiraihi, T., Delshad, A. R., Saghedi Zadeh, M., and Taheri, T. (2013). Bone marrow stromal cell transdifferentiation into oligodendrocyte-like cells using triiodothyronine as a inducer with expression of platelet-derived growth factor alpha as a maturity marker. Iran. Biomed. J. 17, 62–70.
Ahmed, Z., Asi, Y. T., Lees, A. J., Revesz, T., and Holton, J. L. (2013). Identification and quantification of oligodendrocyte precursor cells in multiple system atrophy, progressive supranuclear palsy and Parkinson’s disease. Brain Pathol. 23, 263–273. doi: 10.1111/j.1750-3639.2012.00637.x
Baskin, J. M., Wu, X., Christiano, R., Oh, M. S., Schauder, C. M., Gazzerro, E., et al. (2016). The leukodystrophy protein FAM126A (hyccin) regulates PtdIns(4)P synthesis at the plasma membrane. Nat. Cell Biol. 18, 132–138. doi: 10.1038/ncb3271
Capetian, P., Azmitia, L., Pauly, M. G., Krajka, V., Stengel, F., Bernhardi, E. M., et al. (2016). Plasmid-based generation of induced neural stem cells from adult human fibroblasts. Front. Cell Neurosci. 10:245. doi: 10.3389/fncel.2016.00245
Chen, Y., Balasubramaniyan, V., Peng, J., Hurlock, E. C., Tallquist, M., Li, J., et al. (2007). Isolation and culture of rat and mouse oligodendrocyte precursor cells. Nat. Protoc. 2, 1044–1051. doi: 10.1038/nprot.2007.149
Clemente, D., Ortega, M. C., Melero-Jerez, C., and de Castro, F. (2013). The effect of glia-glia interactions on oligodendrocyte precursor cell biology during development and in demyelinating diseases. Front. Cell Neurosci. 7:268. doi: 10.3389/fncel.2013.00268
Cristofanilli, M., Harris, V. K., Zigelbaum, A., Goossens, A. M., Lu, A., Rosenthal, H., et al. (2011). Mesenchymal stem cells enhance the engraftment and myelinating ability of allogeneic oligodendrocyte progenitors in dysmyelinated mice. Stem Cells Dev. 20, 2065–2076. doi: 10.1089/scd.2010.0547
Czepiel, M., Boddeke, E., and Copray, S. (2015). Human oligodendrocytes in remyelination research. Glia 63, 513–530. doi: 10.1002/glia.22769
De, D., Halder, D., Shin, I., and Kim, K. K. (2017). Small molecule-induced cellular conversion. Chem. Soc. Rev. 46, 6241–6254. doi: 10.1039/c7cs00330g
Dietrich, J., Noble, M., and Mayer-Proschel, M. (2002). Characterization of A2B5+ glial precursor cells from cryopreserved human fetal brain progenitor cells. Glia 40, 65–77. doi: 10.1002/glia.10116
Dincman, T. A., Beare, J. E., Ohri, S. S., and Whittemore, S. R. (2012). Isolation of cortical mouse oligodendrocyte precursor cells. J. Neurosci. Methods 209, 219–226. doi: 10.1016/j.jneumeth.2012.06.017
Douvaras, P., and Fossati, V. (2015). Generation and isolation of oligodendrocyte progenitor cells from human pluripotent stem cells. Nat. Protoc. 10, 1143–1154. doi: 10.1038/nprot.2015.075
Douvaras, P., Wang, J., Zimmer, M., Hanchuk, S., O’Bara, M. A., Sadiq, S., et al. (2014). Efficient generation of myelinating oligodendrocytes from primary progressive multiple sclerosis patients by induced pluripotent stem cells. Stem Cell Rep. 3, 250–259. doi: 10.1016/j.stemcr.2014.06.012
Duncan, I. D., Radcliff, A. B., Heidari, M., Kidd, G., August, B. K., and Wierenga, L. A. (2018). The adult oligodendrocyte can participate in remyelination. Proc. Natl. Acad. Sci. U.S.A. 115, E11807–E11816. doi: 10.1073/pnas.1808064115
Foster, L. M., Landry, C., Phan, T., and Campagnoni, A. T. (1995). Conditionally immortalized oligodendrocyte cell lines migrate to different brain regions and elaborate ‘myelin-like’ membranes after transplantation into neonatal shiverer mouse brains. Dev. Neurosci. 17, 160–170. doi: 10.1159/000111284
Garcia-Leon, J. A., and Verfaillie, C. M. (2016). Stem cell-derived oligodendroglial cells for therapy in neurological diseases. Curr. Stem Cell Res. Ther. 11, 569–577. doi: 10.2174/1574888x10666150902095312
Goldman, S. A., and Kuypers, N. J. (2015). How to make an oligodendrocyte. Development 142, 3983–3995. doi: 10.1242/dev.126409
Han, D. W., Tapia, N., Hermann, A., Hemmer, K., Hoing, S., Arauzo-Bravo, M. J., et al. (2012). Direct reprogramming of fibroblasts into neural stem cells by defined factors. Cell Stem Cell 10, 465–472. doi: 10.1016/j.stem.2012.02.021
Hojjat-Allah, A., Ali Reza, D., Majid Saghedi, Z., and Taher, T. (2013). Bone marrow stromal cell transdifferentiation into oligodendrocyte-like cells using triiodothyronine as a inducer with expression of platelet-derived growth factor α as a maturity marker. Iran. Biomed. J. 17, 62–70. doi: 10.6091/ibj.11162.2013
Kaka, G. R., Tiraihi, T., Delshad, A., Arabkheradmand, J., and Kazemi, H. (2012). In vitro differentiation of bone marrow stromal cells into oligodendrocyte-like cells using triiodothyronine as inducer. Int. J. Neurosci. 122, 237–247. doi: 10.3109/00207454.2011.642037
Kim, H., Walczak, P., Kerr, C., Galpoththawela, C., Gilad, A. A., Muja, N., et al. (2012). Immunomodulation by transplanted human embryonic stem cell-derived oligodendroglial progenitors in experimental autoimmune encephalomyelitis. Stem Cells 30, 2820–2829. doi: 10.1002/stem.1218
Kuai, X. L., Ni, R. Z., Zhou, G. X., Mao, Z. B., Zhang, J. F., Yi, N., et al. (2015). Transplantation of mouse embryonic stem cell-derived oligodendrocytes in the murine model of globoid cell leukodystrophy. Stem Cell Res. Ther. 6:30. doi: 10.1186/s13287-015-0024-2
Li, X., Zhang, Y., Yan, Y., Ciric, B., Ma, C. G., Gran, B., et al. (2016). Neural stem cells engineered to express three therapeutic factors mediate recovery from chronic stage CNS autoimmunity. Mol. Ther. 24, 1456–1469. doi: 10.1038/mt.2016.104
Li, Y., Gautam, A., Yang, J., Qiu, L., Melkoumian, Z., Weber, J., et al. (2013). Differentiation of oligodendrocyte progenitor cells from human embryonic stem cells on vitronectin-derived synthetic peptide acrylate surface. Stem Cells Dev. 22, 1497–1505. doi: 10.1089/scd.2012.0508
Liu, C., Hu, X., Li, Y., Lu, W., Li, W., Cao, N., et al. (2019). Conversion of mouse fibroblasts into oligodendrocyte progenitor-like cells through a chemical approach. J. Mol. Cell Biol. doi: 10.1093/jmcb/mjy088 [Epub ahead of print].
Lu, Y., Yang, Y., Wang, Z., Wang, C., Du, Q., Wang, Q., et al. (2015). Isolation and culture of human oligodendrocyte precursor cells from neurospheres. Brain Res. Bull. 118, 17–24. doi: 10.1016/j.brainresbull.2015.08.008
Manley, N. C., Priest, C. A., Denham, J., Wirth, E. D. III, and Lebkowski, J. S. (2017). Human embryonic stem cell-derived oligodendrocyte progenitor cells: preclinical efficacy and safety in cervical spinal cord injury. Stem Cells Transl. Med. 6, 1917–1929. doi: 10.1002/sctm.17-0065
Mekhail, M., Almazan, G., and Tabrizian, M. (2012). Oligodendrocyte-protection and remyelination post-spinal cord injuries: a review. Prog. Neurobiol. 96, 322–339. doi: 10.1016/j.pneurobio.2012.01.008
Najm, F. J., Lager, A. M., Zaremba, A., Wyatt, K., Caprariello, A. V., Factor, D. C., et al. (2013). Transcription factor-mediated reprogramming of fibroblasts to expandable, myelinogenic oligodendrocyte progenitor cells. Nat. Biotechnol. 31, 426–433. doi: 10.1038/nbt.2561
Nazm Bojnordi, M., Ghasemi, H. H., and Akbari, E. (2015). Remyelination after lysophosphatidyl choline-induced demyelination is stimulated by bone marrow stromal cell-derived oligoprogenitor cell transplantation. Cell. Tissues Org. 200, 300–306. doi: 10.1159/000437350
Neri, M., Maderna, C., Ferrari, D., Cavazzin, C., Vescovi, A. L., and Gritti, A. (2010). Robust generation of oligodendrocyte progenitors from human neural stem cells and engraftment in experimental demyelination models in mice. PLoS One 5:e10145. doi: 10.1371/journal.pone.0010145
Nori, S., Khazaei, M., Ahuja, C. S., Yokota, K., Ahlfors, J. E., Liu, Y., et al. (2018). Human oligodendrogenic neural progenitor cells delivered with chondroitinase abc facilitate functional repair of chronic spinal cord injury. Stem Cell Rep. 11, 1433–1448. doi: 10.1016/j.stemcr.2018.10.017
Rumajogee, P., Altamentova, S., and Li, L. (2018). Exogenous neural precursor cell transplantation results in structural and functional recovery in a hypoxic-ischemic hemiplegic mouse model. eNeuro 5:ENEURO.369–ENEURO.318. doi: 10.1523/eneuro.0369-18.2018
Sher, F., Amor, S., Gerritsen, W., Baker, D., Jackson, S. L., Boddeke, E., et al. (2012). Intraventricularly injected Olig2-NSCs attenuate established relapsing-remitting EAE in mice. Cell Trans. 21, 1883–1897. doi: 10.3727/096368911x637443
Shinkai, Y., Rathbun, G., Lam, K. P., Oltz, E. M., Stewart, V., Mendelsohn, M., et al. (1992). RAG-2-deficient mice lack mature lymphocytes owing to inability to initiate V(D)J rearrangement. Cell 68, 855–867. doi: 10.1016/0092-8674(92)90029-c
Sim, F. J., McClain, C. R., Schanz, S. J., Protack, T. L., Windrem, M. S., and Goldman, S. A. (2011). CD140a identifies a population of highly myelinogenic, migration-competent and efficiently engrafting human oligodendrocyte progenitor cells. Nat. Biotechnol. 29, 934–941. doi: 10.1038/nbt.1972
Swamydas, M., and Lionakis, M. S. (2013). Isolation, purification and labeling of mouse bone marrow neutrophils for functional studies and adoptive transfer experiments. J. Vis. Exp. 10:e50586. doi: 10.3791/50586
Thiruvalluvan, A., Czepiel, M., Kap, Y. A., Mantingh-Otter, I., Vainchtein, I., Kuipers, J., et al. (2016). Survival and Functionality of Human Induced Pluripotent Stem Cell-Derived Oligodendrocytes in a Nonhuman Primate Model for Multiple Sclerosis. Stem Cells Transl. Med. 5, 1550–1561. doi: 10.5966/sctm.2016-0024
Tuszynski, M. H., Yang, J. H., Barba, D., Hs, U., Bakay, R. A., Pay, M. M., et al. (2015). Nerve growth factor gene therapy: activation of neuronal responses in alzheimer disease. JAMA Neurol. 72, 1139–1147. doi: 10.1001/jamaneurol.2015.1807
Wang, S., Bates, J., Li, X., Schanz, S., Chandler-Militello, D., Levine, C., et al. (2013). Human iPSC-derived oligodendrocyte progenitor cells can myelinate and rescue a mouse model of congenital hypomyelination. Cell Stem Cell 12, 252–264. doi: 10.1016/j.stem.2012.12.002
Wang, X., Li, R., Zacharek, A., Landschoot-Ward, J., Wang, F., Wu, K. H., et al. (2018). Administration of downstream ApoE attenuates the adverse effect of brain ABCA1 deficiency on stroke. Int. J. Mol. Sci. 19:3368. doi: 10.3390/ijms19113368
Weiss, W. A., Taylor, S. S., and Shokat, K. M. (2007). Recognizing and exploiting differences between RNAi and small-molecule inhibitors. Nat. Chem. Biol. 3, 739–744. doi: 10.1038/nchembio1207-739
Wu, B., Sun, L., Li, P., Tian, M., Luo, Y., and Ren, X. (2012). Transplantation of oligodendrocyte precursor cells improves myelination and promotes functional recovery after spinal cord injury. Injury 43, 794–801. doi: 10.1016/j.injury.2011.09.013
Yamashita, T., Miyamoto, Y., Bando, Y., Ono, T., Kobayashi, S., Doi, A., et al. (2017). Differentiation of oligodendrocyte progenitor cells from dissociated monolayer and feeder-free cultured pluripotent stem cells. PLoS One 12:e0171947. doi: 10.1371/journal.pone.0171947
Yang, J., Jiang, Z., Fitzgerald, D. C., Ma, C., Yu, S., Li, H., et al. (2009). Adult neural stem cells expressing IL-10 confer potent immunomodulation and remyelination in experimental autoimmune encephalitis. J. Clin. Invest. 119, 3678–3691. doi: 10.1172/jci37914
Yang, J., Yan, Y., Ciric, B., Yu, S., Guan, Y., Xu, H., et al. (2010). Evaluation of bone marrow- and brain-derived neural stem cells in therapy of central nervous system autoimmunity. Am. J. Pathol. 177, 1989–2001. doi: 10.2353/ajpath.2010.091203
Yang, N., Zuchero, J. B., Ahlenius, H., Marro, S., Ng, Y. H., Vierbuchen, T., et al. (2013). Generation of oligodendroglial cells by direct lineage conversion. Nat. Biotechnol. 31, 434–439. doi: 10.1038/nbt.2564
Zhang, Y., Lu, X. Y., Ye, Z. Q., Ciric, B., Ma, C. G., Rostami, A., et al. (2019). Combination therapy with fingolimod and neural stem cells promotes functional myelination in vivo through a non-immunomodulatory mechanism. Front. Cell Neurosci. 13:14. doi: 10.3389/fncel.2019.00014
Zhao, Y., Zhao, T., Guan, J., Zhang, X., Fu, Y., Ye, J., et al. (2015). A XEN-like state bridges somatic cells to pluripotency during chemical reprogramming. Cell 163, 1678–1691. doi: 10.1016/j.cell.2015.11.017
Zhu, B., Zhao, C., Young, F. I., Franklin, R. J., and Song, B. (2014). Isolation and long-term expansion of functional, myelinating oligodendrocyte progenitor cells from neonatal rat brain. Curr. Protoc. Stem Cell Biol. 31, 1–15. doi: 10.1002/9780470151808.sc02d17s31
Keywords: oligodendrocyte progenitor cells, oligodendrocytes, neurospheres, bone marrow, autologous cells
Citation: Zhang Y, Lu X-Y, Casella G, Tian J, Ye Z-Q, Yang T, Han J-J, Jia L-Y, Rostami A and Li X (2019) Generation of Oligodendrocyte Progenitor Cells From Mouse Bone Marrow Cells. Front. Cell. Neurosci. 13:247. doi: 10.3389/fncel.2019.00247
Received: 14 December 2018; Accepted: 17 May 2019;
Published: 05 June 2019.
Edited by:
Paulo Henrique Rosado-de-Castro, D’Or Institute for Research and Education (IDOR), BrazilReviewed by:
Jorge Matias-Guiu, Complutense University of Madrid, SpainEnrica Boda, University of Turin, Italy
Copyright © 2019 Zhang, Lu, Casella, Tian, Ye, Yang, Han, Jia, Rostami and Li. This is an open-access article distributed under the terms of the Creative Commons Attribution License (CC BY). The use, distribution or reproduction in other forums is permitted, provided the original author(s) and the copyright owner(s) are credited and that the original publication in this journal is cited, in accordance with accepted academic practice. No use, distribution or reproduction is permitted which does not comply with these terms.
*Correspondence: Xing Li, eGluZ2xpX3hpYW5AMTI2LmNvbQ==
†These authors have contributed equally to this work