- 1Department of Molecular and Cellular Neurobiology, Center for Neurogenomics and Cognitive Research, Amsterdam Neuroscience, Vrije Universiteit, Amsterdam, Netherlands
- 2Department of Integrative Neurophysiology, Center for Neurogenomics and Cognitive Research, Amsterdam Neuroscience, Vrije Universiteit, Amsterdam, Netherlands
G-protein-coupled receptor 158 (Gpr158) is highly expressed in striatum, hippocampus and prefrontal cortex. It gained attention as it was implicated in physiological responses to stress and depression. Recently, Gpr158 has been shown to act as a pathway-specific synaptic organizer in the hippocampus, required for proper mossy fiber-CA3 neurocircuitry establishment, structure, and function. Although rodent Gpr158 expression is highest in CA3, considerable expression occurs in CA1 especially after the first postnatal month. Here, we combined hippocampal-dependent behavioral paradigms with subsequent electrophysiological and morphological analyses from the same group of mice to assess the effects of Gpr158 deficiency on CA1 physiology and function. We demonstrate deficits in spatial memory acquisition and retrieval in the Morris water maze paradigm, along with deficits in the acquisition of extinction memory in the passive avoidance test in Gpr158 KO mice. Electrophysiological recordings from CA1 pyramidal neurons revealed normal basal excitatory and inhibitory synaptic transmission, however, Schaffer collateral stimulation yielded dramatically reduced post-synaptic currents. Interestingly, intrinsic excitability of CA1 pyramidals was found increased, potentially acting as a compensatory mechanism to the reductions in Schaffer collateral-mediated drive. Both ex vivo and in vitro, neurons deficient for or with lowered levels of Gpr158 exhibited robust reductions in dendritic architecture and complexity, i.e., reduced length, surface, bifurcations, and branching. This effect was localized in the apical but not basal dendrites of adult CA1 pyramidals, indicative of compartment-specific alterations. A significant positive correlation between spatial memory acquisition and extent of complexity of CA1 pyramidals was found. Taken together, we provide first evidence of significant disruptions in hippocampal CA1 neuronal dendritic architecture and physiology, driven by Gpr158 deficiency. Importantly, the hippocampal neuronal morphology deficits appear to support the impairments in spatial memory acquisition observed in Gpr158 KO mice.
Introduction
G-protein-coupled receptors (GPCRs) form a large family of seven transmembrane proteins. In the brain, these act as important regulators of synaptic transmission, neuronal excitability, and structural plasticity, through presynaptic and postsynaptic mechanisms of action (Huang and Thathiah, 2015; Leung and Wong, 2017). Several brain-expressed GPCRs were shown to modulate cognition and are suspect in the pathobiology of neuronal disorders (Catapano and Manji, 2007; Thompson et al., 2008). Collectively, GPCRs make up the largest drug target group to-date (Chan et al., 2015). Recently, G-protein coupled receptor 158 (Gpr158) caught attention due to its role in hippocampus-dependent memory formation via osteocalcin (OCN) signaling (Khrimian et al., 2017). Gpr158 is found highly expressed in the brain, specifically in prefrontal regions, striatum, and hippocampus (Orlandi et al., 2012; Khrimian et al., 2017; Sutton et al., 2018). Previously, we have observed Gpr158 strongly upregulated in the hippocampus synaptic membrane fraction after stress of an immediate shock (Rao-Ruiz et al., 2015). The stress-responsiveness of Gpr158 was further illustrated by its rapid and robust increase in expression upon glucocorticoid stimulation either in vitro (Patel et al., 2013), or in vivo (Sutton et al., 2018). Finally, Gpr158 was shown increased in the prefrontal cortex (PFC) in the hours to days after physical stress in mice, as well as in post-mortem prefrontal tissue from depressed patients, whereas Gpr158 KO mice exhibited resilience to unpredicted chronic mild stress (Sutton et al., 2018).
Recent work has begun shedding light on the precise function of Gpr158 (Orlandi et al., 2012; Khrimian et al., 2017; Condomitti et al., 2018; Sutton et al., 2018). Gpr158 overexpression in mouse medial PFC (mPFC) increases helplessness, illustrated by increased immobility in a tail-suspension (TST) and forced-swim tests (FST) (Sutton et al., 2018). On the contrary, global deletion of Gpr158 had an anxiolytic and anti-depressant effect, exhibited by decreased immobility in TST and FST (Sutton et al., 2018). Recordings from Gpr158 KO mPFC superficial layer pyramidals revealed increases in spontaneous excitatory postsynaptic current (sEPSC) frequency, partially supported by an increase in spine density, and an AMPA receptor (AMPAR) current-driven increase in the AMPAR/NMDAR ratio persisting regardless of dendritic spine size (Sutton et al., 2018).
Hippocampal-dependent learning appears negatively affected in Gpr158 KO mice, as observed during Morris water maze (MWM) training, and as shown by memory deficits in the novel object recognition task (NOR) (Khrimian et al., 2017). Dentate gyrus’ (DG) mossy fiber (MF) to CA3 long-term potentiation (LTP) was shown significantly impaired in these mice (Khrimian et al., 2017). Moreover, frequency and amplitude of sEPSCs, AMPAR and NMDAR evoked currents, and MF to CA3 paired pulse ratio (PPR) were all robustly reduced in Gpr158 KO CA3 pyramidals (Condomitti et al., 2018). Although CA3 spine density and MF-CA3 synapse density were paradoxically found increased, both the area and volume per MF-CA3 synapse were robustly reduced (Condomitti et al., 2018). Importantly, the aforementioned electrophysiological and morphological deficits were pathway-specific, affecting MF to CA3 synapses, but not CA3 to CA3 association/commissural synapses. The underlying mechanism for this was elegantly demonstrated by the discovery of glypican 4 (Gpc4), which is highly enriched in MFs, as the presynaptic binding partner to postsynaptic hippocampal Gpr158 (Condomitti et al., 2018).
Given the considerable CA1 expression from post-weaning onward (Condomitti et al., 2018; Ostrovskaya et al., 2018), as well as the role of Gpr158 in neuronal signaling and cognition (Khrimian et al., 2017; Sutton et al., 2018), we investigated the effect of Gpr158 deficiency on both electrophysiological properties and neuronal architecture in the hippocampus CA1 with in vitro and ex vivo approaches. We took specific advantage of measuring hippocampus-dependent behavior and performing electrophysiological recordings with cellular reconstructions in the same set of animals, allowing us to create insightful correlations between the behavior and underlying neuronal architecture observed. Taken together, our data reveal spatial learning deficits and neuronal hyperexcitability in Gpr158 KO mice, driven by the compromised dendritic architecture of CA1 pyramidal neurons.
Materials and Methods
Animals
Gpr158 knock-out (KO) mice [Gpr158TM 1(KOMP)Vlcg] were generated by replacing the Gpr158 gene with a LacZ cassette including a stop codon in the region of exon 1 and exon 2 (Orlandi et al., 2015), and were purchased from the KOMP repository (see Supplementary Figure 1). Animals were bred for >5 generations on a C57BL/6J background (Charles River, France) in the animal facility of the VU University Amsterdam. Mice were kept in standard type-2 Macrolon cages, enriched with nesting material on a 12/12 h light/dark rhythm (lights on at 7:00 AM), a constant temperature of 23 ± 1°C, and a relative humidity of 50 ± 10%. Food and water were provided ad libitum. After weaning, all mice were group-housed per sex. For behavioral experiments, male mice were single-housed 1–2 weeks prior to the first test, and received a PVC tube and wooden chew stick as additional enrichment. For all behavioral experiments, we used male 8–12 weeks old Gpr158 KO mice and wild type (WT) littermates as control, in addition to a batch of male heterozygous (HZ) Gpr158 mice and WT littermates, aged 10–11 weeks at testing, as specified in Supplementary Figure 2. Electrophysiological recording and biocytin fillings were performed on the same KO and WT males that were tested in the Morris water maze and open field, with an >8 week interval after the last test, at the age of ∼16–20 weeks. For primary hippocampal cultures, E18 embryos were taken from pregnant WT (C57BL/6J) mice. All experiments were performed in accordance to Dutch law and licensing agreements and protocols were approved by the Animal Ethics Committee of the VU University Amsterdam.
Behavioral Tests
Morris Water Maze (MWM), Passive Avoidance (PA), contextual Fear conditioning (cFC), and Open Field (OF) were carried out in four independent batches of animals (Supplementary Figure 2).
Morris Water Maze (MWM) Test
Animals were handled for 1 week, two times a day, prior to MWM training. A circular pool (125 cm in diameter) was filled with opaque-colored (non-toxic dye) water and the water temperature was kept at 23–25°C. A round transparent escape platform (9 cm in diameter) was placed in the top left quadrant (target quadrant) of the pool, hidden 0.2 cm below the water surface. Geometric visual cues (1 m from the pool) were located on the walls of a dimly lit room (20 lx). Each mouse received a total of 2 daily swim sessions, each comprised of 2 swim trials (maximum duration 60 s per trial), with an interval of 2 min between sessions for 4 consecutive days. Before starting each training day, mice were placed on the platform for 30 s and then placed into the water at a semi-random start position (out of 4 start-positions). Mice that failed to find the platform were guided and placed on the platform for 15 s by the experimenter on day one. After each swim session, mice were returned to their home-cage for 2 min. Latency and distance swam to find the platform during training, and time spent and distance swam in the target quadrant in the probe test were examined using video tracking Viewer 2 (Biobserve, Bonn, Germany). Latency and distance to find the platform during training served as read-out of spatial memory acquisition. On the 5th day, the probe test (60 s) was performed, in which the platform was removed and mice were placed into the water opposite to the location of the platform/target quadrant. The time spent in each quadrant was recorded and time spent in the target quadrant served as measure of long-term spatial memory.
Passive Avoidance Test
The pre-exposure, training, retention test, forced-exposure and 5-day extinction protocols were performed in a PA system (Model 256000, TSE-Systems, Bad Homburg, Germany). In the pre-exposure protocol, without unconditioned stimulus (US), mice were placed in the bright compartment (1000 lx) for 15 s. Then, a sliding door was opened for allowing the mice to explore the dark compartment (∼10 lx) for a maximum period of 300 s. The training and retention test were performed as described before (Baarendse et al., 2008) with minor modifications in the US intensity (0.7 mA) and duration (2 s). The forced-exposure protocol was performed depending on the maximum time spent in the dark compartment during training, totaling 600 s. The extinction protocol was performed as the pre-exposure protocol, but now for a maximum period of 600 s. During the retrieval and extinction protocols, the latency to enter the dark compartment was measured as a read-out of long-term memory. Mice that did not enter the dark compartment during retrieval and extinction tests were recorded as a latency of 600 s. Boxes were cleaned with 70% ethanol between each experiment.
Contextual Fear Conditioning
Contextual fear conditioning was carried out in a fear conditioning system (TSE-Systems, Bad Homburg, Germany) as described before (Schmitz et al., 2017). Long-term memory was assessed 24 h after training.
Open Field
The open field test (OF) was performed in a white square box (50 × 50 cm, 35 cm high, 200 lx) by placing mice in the corner of the box and recording their exploration behavior for 10 min by video tracking (Viewer 2, Biobserve GmbH, Bonn, Germany). Time spent in the center area (32.9 × 32.9 cm) and total distance moved during the 10 min test were used as parameters for anxiety-like behavior and locomotor performance. Open field boxes were cleaned with 70% ethanol between each experiment.
Primary Hippocampal Culture, Short Hairpin RNAs and Lentivirus Production
Hippocampal cultures were collected in Hanks balanced salts solution (HBSS; Sigma-Aldrich, St. Louis, MO, United States) with 7 mM HEPES (Life Technologies/Gibco, Carlsbad, CA, United States) and trypsin was added (10% final concentration; Life Technologies/Gibco) for 15–20 min at 37°C. Following two washing steps with HBSS-HEPES and one time with neurobasal medium [supplemented with 2% B-27, 1.8% HEPES, 0.25% glutamax and 0.1% penicillin/streptomycin (all from Life Technologies/Gibco)]. Cells were triturated with a fire-polished Pasteur pipette. After cell dissociation, neurons were plated at a density of 12.5 × 103 cells/well in a 96-well plate (Cellstar, Greiner Bio-One, Frickenhausen, Germany) coated with poly-D-lysine and laminin (Sigma-Aldrich) and treated with 5% heat-inactivated horse serum (Life Technologies/Gibco), or at a density of 75 × 103/well in a 12-well plate. Neurons were kept at 37°C/5% CO2.
Short hairpin RNAs (shRNAs) were purchased from Sigma-Aldrich (Mission® shRNA bacterial glycerol stock; SHCLNG-XM_140850). Five shRNA were used to target and knockdown Gpr158 gene expression and a scrambled shRNA (Sigma-Aldrich, pLKO.1-puro Non-Mammalian shRNA) was used as a control. Sequences for shRNA knockdown of the Gpr158 gene were: shRNA#1 (TRC number: TRCN0000028697, sequence: CCGGGCCAAGTACATTTC GTTGTATCTCGAGATACAACGAAATGTACTTGGCTTTTT), shRNA#2 (TRC number: TRCN0000028716, sequence: CCGGGCTCATTATCACGGCTATATTCTCGAGAATATAGCC GTGATAATGAGCTTTTT), shRNA#3 (TRC number: TRCN0000028727, sequence: CCGGCCGGTCGTTATTCTG TACTTTCTCGAGAAAGTACAGAATAACGACCGGTTTTT), shRNA#4 (TRC number: TRCN0000028742, sequence: CCGGCGGCTATATTCCATACAATTACTCGAGTAATTGTAT GGAATATAGCCGTTTTT) and shRNA#5 (TRC number: TRCN0000028751, sequence: CCGGCCTTAACAACTCAGAG TGTATCTCGAGATACACTCTGAGTTGTTAAGGTTTTT).
Plasmids of shRNAs were plated on Lysogeny broth (LB) agar medium supplemented with ampicillin (Sigma). Single colonies were picked and grown in LB liquid medium supplemented with ampicillin using a shaker incubator at 37°C. DNA was extracted using NucleoBond Xtra Midi kit (Bioké, Leiden, Netherlands) according to the manufacturer’s protocol. Lentivirus production were done using packaging, transducing and envelope constructs at the same time for human embryonic kidney 293T cells transfection. Lentiviral particles were collected after 2 days of transfection and concentrated using ultracentrifugation (Beckman Coulter, OptimaTM LE-80K).
Neurons were separately transduced with a Gpr158-targeting shRNA (see below) lentivirus at days in vitro 7 (DIV7). Briefly, neurons were transduced with 1:4000 titrated lentiviral particles in neurobasal medium supplemented with 2% B-27, 1.8% HEPES, 0.25% glutamax, 0.1% penicillin/streptomycin for each shRNA. Transduced neurons were kept at 37°C/5% CO2. Cells for immunostaining were fixed at DIV14 using 4% PFA, 2% saccharose (VWR chemicals) in PBS (pH 7.4) for 20 min. Cells for quantitative real time PCR (qRT-PCR) experiments were harvested using Trizol reagent (Life Technologies) at DIV14.
High-Content Screening for Neurite Morphology Analysis
Fixed neurons were washed with PBS and permeabilized with 0.5% Triton X-100 (Sigma-Aldrich) in PBS (Life Technologies) for 10 min. After washing, cells were blocked with 1% BSA (Sigma-Aldrich) and 0.1% Triton X-100 (Sigma-Aldrich) in PBS for 1 h. Neurons were stained with chicken anti-MAP2 (Bio-Connect, Huissen, Netherlands; 1:5,000) at 4°C overnight, and visualized using anti-chicken Alexa Fluor 647 (Life Technologies/Gibco; 1:400) for 90 min at RT. The nucleus was stained with Hoechst dye (1:10,000 in distilled water) for 10 min at RT.
Nuclei and neurites of neurons were imaged with Opera LX high-content screening system (PerkinElmer, Waltham, MA, United States). The 96-well plates were scanned with 10x magnification, sampling 40 images per well. All images were analyzed using Columbus software (PerkinElmer, v2.5.2). Briefly, neuron nuclei were dissociated from non-neuronal nuclei or debris based on morphological (nuclei area > 200 μm2) and intensity (nuclei intensity > 100) parameters. Analyses were made at the population level of a well, taking the mean of selected neurons. Total neurite length (traced from the soma), number of protrusions (neurites branching) from the soma, number of first bifurcations (from soma protrusions), and number of extremities (neurite branching number from the first bifurcations) were calculated (see Supplementary Figure 1).
Quantitative Real Time PCR for Gene Expression Analysis
Transduced neurons were collected with TRIZOL reagent (Life Technologies) at DIV14 and RNA isolation was performed accordingly using isopropanol (Sigma-Aldrich) precipitation (Spijker et al., 2004). RNA was quantified (NanoDrop ND-1000 spectrophotometer; NanoDrop Technologies, Wilmington, DE, United States). Random-primed (25 pmol; Eurofins MWG Operon; Ebersberg, Germany) cDNA synthesis was performed on individual RNA samples (100–125 ng total RNA) using MMLV reverse transcriptase (Promega). Real-time qPCR reactions (10 μL; Light cycler 480, Roche) and relative gene expression calculation were performed as described previously (Spijker et al., 2004; Schmitz et al., 2017). All qRT-PCR primers were designed using Primer3.0 software and are listed (forward and reverse) as follows: Gpr158: 5′-AACACAGCCTAGATCCAGAAGAC-3′ and 5′-GGGTTGTTTGTGATCATCTTTTTA-3′; Gapdh: 5′-TGCACCACCAACTGCTTAGC-3′ and 5′-GGCATGGACT GTGGTCATGA-3′; β-Actin: 5′-GCTCCTCCTGAGCGCAAG-3′ and 5′-CATCTGCTGGAAGGTGGACA-3′; Hprt: 5′-ATGGGA GGCCATCACATTGT-3′ and 5′-ATGTAATCCAGCAGGTCA GCAA-3′.
Slice Preparation, Electrophysiological Recordings and Morphological Reconstructions
Upon completion of the behavioral assessment, mice were swiftly decapitated and brains were extracted and placed for a short period, during transport to slicing room, in carbogenated (95% O2, 5% CO2) iced-cold ‘slicing buffer’ containing (in mM), 70 NaCl, 2.5 KCl, 1.25 NaH2PO4.H2O, 5 MgSO4.7H2O, 1 CaCl2.2H2O, 70 Sucrose, 25 D-Glucose, 25 NaHCO3, 1 Na-Ascorbate, 3 Na-Pyruvate; pH 7.4, 305 mOsm. Horizontal hippocampal slices (dorsal to ventral) were retrieved at 300 μm thickness, using a vibrating-blade microtome (HM-650V, Thermo Scientific), in ice-cold carbogenated ‘slicing buffer,’ where the dorsal to medial part of the hippocampus was taken. Each slice was briefly washed in ‘holding ACSF’ and placed in a slices chamber filled with carbogenated ‘holding ACSF’ containing in mM, 125 NaCl, 3 KCl, 1.25 NaH2PO4.H2O, 2 MgCl2.6H2O, 1.3 CaCl2.2H2O, 25 D-Glucose, 25 NaHCO3, 1 Na-Ascorbate, 3 Na-Pyruvate; pH 7.4, 305 mOsm. Slices were left to recover at RT for at least 1 h before recordings, and for the duration of the experimental day slices were maintained in the slice chamber containing ‘holding ACSF.’
Spontaneous Excitatory and Inhibitory Postsynaptic Current Recordings
Individual slices were transferred to a submerged recording chamber and left to equilibrate for 10 min, under continuous perfusion, at ∼2 mL/min, with carbogenated ‘recording ACSF’ containing in mM, 125 NaCl, 3 KCl, 1.25 NaH2PO4.H2O, 1 MgCl2.6H2O, 1.3 CaCl2.2H2O, 25 D-Glucose, 25 NaHCO3; pH 7.4, 305 mOsm. Hippocampal CA1 pyramidal neurons were visualized under differential interference contrast microscopy, and selected based on their morphology. For sEPSC recordings in WT 10 cells from 4 animals, and in KO 14 cells from 4 animals were analyzed. For sIPSC recordings in WT 11 cells from 4 animals, and in KO 14 cells from 4 animals were analyzed. Whole-cell patch-clamp configuration was achieved using standard borosilicate glass pipettes, ∼3–5 Mohm, filled with an Cesium-gluconate based intracellular containing in mM, 130 Cs-gluconate, 8 NaCl, 10 HEPES, 0.3 EGTA, 4 ATP-Mg, 10 K2-Phosphocreatine, 0.3 GTP, 3 QX314-Cl, 0.3% Biocytin; pH 7.3, 290 mOsm. Recordings were conducted at ∼32°C. Upon achieving a stable whole-cell configuration, up to 7 min of sEPSCs were recorded at a holding potential of −70 mV, and up to 7 min of sIPSCs were recorded at a holding potential of 0 mV (voltage clamp). The last 3 min of each trace were analyzed with mini Analysis software (Synaptosoft). Acquisition was performed using p-Clamp software (Molecular Devices), using a Multiclamp 700B amplifier (Molecular Devices), sampled at 20 kHz, low-pass filtered at 6 kHz, and digitized with an Axon Digidata 1440A (Molecular Devices). Series resistance was monitored and only cells exhibiting less than 20% change were used for analysis. Cells whose access resistance exceeded 25 Mohm were also rejected.
Paired Pulse Ratio Recordings
For PPR recordings of EPSCs, based on the observed change in decay time and similar to previously reported changes in PPR in the MF-CA3 pathway, whole-cell patch-clamp configuration was achieved with a K-gluconate based intracellular containing in mM, 148 K-gluconate, 1 KCl, 10 HEPES, 0.3 EGTA, 4 ATP-Mg, 4 K2-Phosphocreatine, 0.4 GTP, 0.3% Biocytin; pH 7.3, 290 mOsm. For this experiment in WT 10 cells from 4 animals, and in KO 10 cells from 3 animals were analyzed. Upon achieving stable whole-cell configuration, action potential profiles (current clamp) were generated for each CA1 pyramidal, through current injections of 750 ms starting from −200 pA, at steps of 20 pA. These profiles were subsequently used to retrieve the passive and active properties form these cells. The resting membrane potential was measured during a 250 ms period prior to action potential profile generation. Subsequently, a unipolar stimulating electrode loaded with ‘recording ACSF’ was lowered near the location were Schaffer collaterals meet the patched-cell’s dendrite. The electrode was moved around until a clear unisynaptic response could be observed, upon current injections mediated by a Master-9 pulse stimulator and an ISO-flex stimulus isolator (A.M.P.I). Thereafter, an input-output stimulation curve was generated by recording the patched cells’ response to increasing amounts of current injections from 20 μA to 200 μA, at steps of 20 μA. For each step, 5 sweeps were averaged. The current intensity used during the PPR protocol was approximately the current generating half-maximal amplitude responses. The two pulses for the PPR protocol were separated by 50 ms, and 20–25 sweeps were recorded per cell. Data were analyzed with in-house Matlab (Mathworks) scripts. All other electrophysiological and technical parameters were as described in the section regarding sEPSC/sIPSC recordings.
Biocytin Reconstructions
Upon completion of an electrophysiological recordings the pipette was slowly retracted to reseal the patched cell, and the slice was subsequently fixed in 4% paraformaldehyde (PFA), at 4°C for 48 h. PFA was replaced with PBS-Azide for long-term storage at 4°C until further analysis. The following set of cells was reconstructed. From the cells recorded under the PPR section, in WT 10 cells from 4 animals, and in KO 10 cells from 3 animals were analyzed. From the cells under the sEPSC/sIPSC section, in WT 8 cells form 3 animals (one animal is shared with the PPR experiment), and in KO 7 cells from 3 animals. For the per animal morphology, the aforementioned cells were averaged per animal, yielding in WT 18 cells from 6 animals, and in KO 17 cells from 6 animals. Slices containing biocytin filled cells, were incubated in 3% H2O2 in 0.05 M PB solution for 20 min, and then incubated for 48 h in 0.05 M PB containing 0.5% Triton X-100, and ABC complex solution (Vectastain; 1 drop solution A, 1 drop solution B, per 20 mL volume). Subsequently, slices were incubated in 0.05 M PB containing 3.3 mM H2O2 and 2 mM diaminobenzidine tetrahydrochloride (DAB) under visual inspection. Incubation was terminated when a clear morphology could be visualized. All steps were separated by 3x washes in 0.05 M PB. Images of labeled cells were acquired with a light microscope (Olympus) at 20X magnification, using the Surveyor software (Objective Imaging). Reconstruction of the imaged cells was performed using the Neuromantic software (Myatt et al., 2012), and analysis of the reconstructed morphologies was performed using L-Measure (Scorcioni et al., 2008).
Statistics
For genotype comparisons, two-tailed Student’s t-tests (with or without correction for unequal variation) were applied for normally distributed data and Mann–Whitney U-tests otherwise. Normality was assessed with Kolmogorov–Smirnov and Saphiro–Wilk tests. In the case of normally distributed data but with unequal variances, Welch’s unequal variance t-test was applied. Mixed ANOVA tests were carried out for genotype as between-subject factor and time as repeated measures (e.g., MWM training, PA extinction, I/O curve). A two-way ANOVA was applied to the morphological analyses at the animal level with genotype and type of dendrite as between-subject factors. All data pertaining to Principal component analysis (PCA) were generated using Matlab scripts (Mathworks). Multiple comparison correction for the correlation between cell surface and PCA variables was performed with the Bonferroni–Holm method. Three mice were removed from the MWM data set as they were floating instead of swimming [Gpr158 KO n = 1 and WT n = 1, WT n = 1 (HZ batch)]. For electrophysiology, cells with values exceeding mean ± 2xSD were excluded (PPR/Properties: Gpr158 KO n = 1, WT = 3; sEPSC/sIPSC: Gpr158 KO n = 4, WT n = 3). Statistical significance level was set for P-values < 0.05. Statistical significance was assessed using SPSS v24 IBM, or Graphpad Prism 5 software (GraphPad Software, La Jolla, CA, United States) for Bonferroni post hoc tests.
Results
Gpr158 KO Mice Show Aberrant Spatial Learning and Decreased Acquisition of Safety Learning
Given the significant hippocampal expression of Gpr158 (Allen brain atlas probe RP_051121_01_A10; Khrimian et al., 2017), not only in CA3 but also in the CA1 region (Ostrovskaya et al., 2018) (Supplementary Figure 1), we tested Gpr158 KO mice and WT littermates in an array of hippocampal-dependent behavioral paradigms (Supplementary Figure 2). First, we performed MWM training with male Bl6/J Gpr158 KO mice (Figures 1A,B and Supplementary Figure 2) and a probe test to assess long-term memory retainment of hidden platform location based on distal cues. During the 4-day training protocol, the distance swam to find the hidden platform was found statistically different in Gpr158 KO mice vs. WT mice (Figure 1A and Supplementary Table 1, mixed ANOVA: genotype P = 0.046; training P = 0.500, interaction P = 0.059), with post hoc testing showing increased distance in Gpr158 KO mice vs. WT mice during the last 2 training days (t-test: day 3 P = 0.031, day 4 P = 0.025). In addition, escape latency showed a similar effect (Supplementary Figure 2 and Supplementary Table 1), albeit the first training day showed an initial genotype difference in activity (Supplementary Figure 2). Whereas WT mice showed learning from day 1 to 4 (Latency: P = 0.025; Distance: P = 0.012), Gpr158 KO mice did not (Latency: P = 0.202; Distance: P = 0.831).
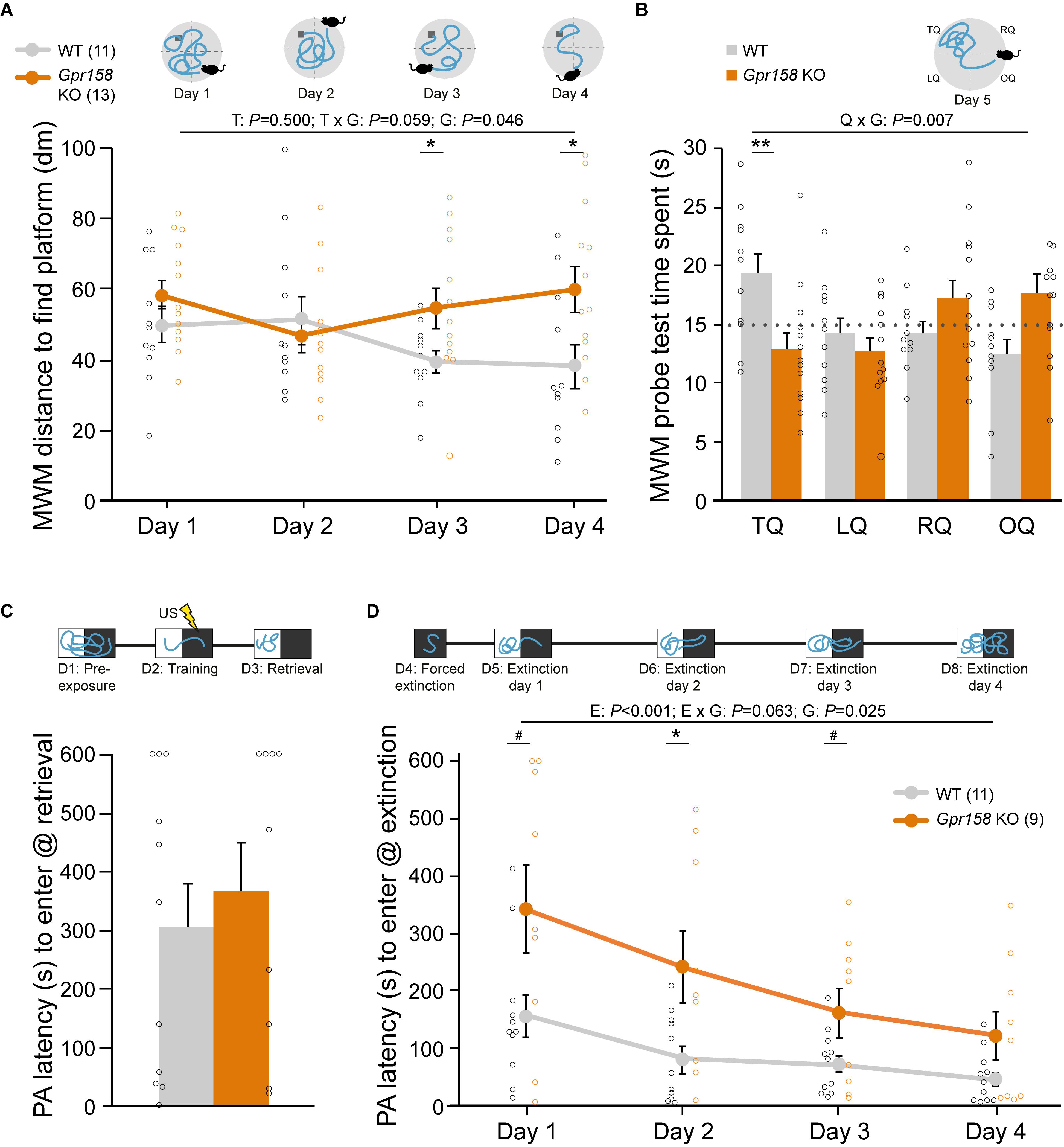
Figure 1. Gpr158 KO have a spatial memory deficit and show delayed safety learning. (A,B) Experimental set-up and data of training [see Online resource 3; (A) during four days of training, mice were placed in different locations in the circular pool and distance to reach the hidden platform (dark gray) was recorded], and probe test [B, time spent in each quadrant was recorded) in the Morris water maze (MWM]. (A) During MWM training, Gpr158 KO mice swam a longer distance to reach the platform and their performance did not reach WT levels [nWT = 11, nKO = 13; genotype (G) effect P < 0.046; training (T) effect P = 0.500, interaction P = 0.059]. (B) Gpr158 KO mice showed a long-term memory deficit during the probe test [quadrant (Q) × genotype (G) P = 0.007], and spent less time in the target quadrant (TQ, RQ: right quadrant, LQ: left quadrant, OQ: opposite quadrant). (C,D) Experimental set-up and data of PA test and latency to enter the dark compartment after training (C) and during the four days after forced extinction (D). (C) Gpr158 KO mice (n = 9) showed similar PA retention as WT mice (n = 11). (D) After forced extinction in the dark compartment, Gpr158 KO mice showed a delay in extinction learning [Extinction (E) P < 0.001, genotype (G) P = 0.025, interaction P = 0.063]. Data are presented as mean ± SEM with individual data points indicated. Asterisks and octothorpe indicate the level of significance between WT and KO assessed by Student’s t-test/MWU test (Supplementary Table 1), #P ≤ 0.100; ∗P ≤ 0.050; ∗∗P ≤ 0.010.
Long-term memory was assessed with a 24 h retention interval after training during a probe session in which the platform was removed (Supplementary Figure 2 and Supplementary Table 1). As the probe test measures the preferred location in a fixed time window it is able to dissociate memory from general activity, and as such it is relatively insensitive to altered swimming speed (Vorhees and Williams, 2006). Gpr158 KO mice spent significantly less time in the target quadrant (TQ), indicative of long-term spatial memory impairments (Figure 1B, mixed ANOVA: interaction quadrant × genotype P = 0.007; t-test: TQ P = 0.008). The WT mice display a significant effect for quadrants (P = 0.029), but the KO mice did not (P = 0.102).
In addition, we tested Gpr158 heterozygous (HZ) mice in the MWM test in order to see whether their spatial memory was affected as well (Supplementary Figure 2 and Supplementary Table 1). MWM acquisition was not affected (mixed ANOVA: genotype P = 0.312; training P < 0.01, interaction P = 0.320), and both WT and HZ mice showed learning from day 1 to 4 (WT: P < 0.001; Gpr158 HZ mice: P = 0.029). However, when tested in the MWM probe test, a deficit emerged (mixed ANOVA: interaction quadrant × genotype P = 0.038). Whereas the WT mice showed a good distinction between the former place of the escape location (quadrants P = 0.038), the HZ mice were unable to do so (quadrants P = 0.539), with a significant effect for the target quadrant (genotype P = 0.042). Overall, this indicated that the HZ Gpr158 phenotype was milder than that of the full KO.
To distinguish between disturbed spatial processing deficits and motor or visual impairments possibly contributing to the observed changes, a visual platform test was performed 1 month after the probe test using a cue to signal the platform. Latency to find the platform was the same for Gpr158 KO and WT mice during the visual platform trials (WT = 7.1 ± 1.1 s, Gpr158 KO = 10.2 ± 2.0 s; t-test genotype P = 0.201, Supplementary Table 7). Additionally, when tested in the open field arena, anxiety-like behavior measured as distance moved and anxiolytic-like behavior measured as time spent in the center, was not different between genotypes (Supplementary Figure 2). Taken together, in the absence of motor and visual deficits or overt anxiety-like behavior, Gpr158 KO mice exhibited spatial memory deficits in the MWM paradigm.
To assess context-specific memory, an independent batch of Gpr158 KO and WT mice underwent a contextual fear conditioning (cFC) paradigm (Supplementary Figure 2 and Supplementary Table 1). Gpr158 KO mice did not have any fear memory deficit, exhibiting equal distance moved and freezing as WT during the retrieval test 24 h after conditioning; distance: t-test genotype P = 0.715; freezing: t-test genotype P = 0.693). To corroborate these results and to assess extinction of the acquired aversive memory we probed PA behavior, in an independent batch of mice, in which mice learn to avoid the preferred dark environment where a foot shock was delivered (Figures 1C,D). Long-term avoidance memory was tested on day 3, after pre-exposure (day 1) and training (day 2, Figure 1C). Both Gpr158 KO and WT mice showed the same level of retention (genotype MWU P = 0.656), corroborating the lack of contextual aversive memory deficits in Gpr158 KO mice. Additionally, we assessed whether acquisition of extinction memory was different between genotypes (Figure 1D). To this end, we first performed a forced extinction session in the dark compartment in order to promote safety learning over response extinction only (Micale et al., 2017), and thereafter measured the latency to enter the dark compartment during the subsequent four days of extinction. This revealed a significant delay in extinction memory acquisition for Gpr158 KO mice (Figure 1D, mixed ANOVA: Extinction P < 0.001, genotype P = 0.025, interaction P = 0.063; t-test genotype day 1 P = 0.054, day 2 P = 0.020; day 3 P = 0.077). Taken together, whereas Gpr158 KO mice show no deficits in aversive memory acquisition, they do exhibit spatial memory deficits and difficulty in acquiring extinction memory, in the absence of motor and visual impairments or anxiety.
Normal Basal Excitatory and Inhibitory Synaptic Transmission in Gpr158 KO Mice
Abnormal synaptic transmission can alter information processing, affect learning and memory, and impact behavior (Mayford et al., 2012; Mitsushima et al., 2013). To that end, spontaneous excitatory and inhibitory post-synaptic currents (sEPSC and sIPSC) were recorded from CA1 pyramidal neurons from WT and Gpr158 KO mice after completion of behavioral tasks (Figures 2A–D and Supplementary Table 2). Basal sEPSC frequency (t-test P = 0.193) and amplitude (MWU P = 0.403) was comparable between genotypes (Figures 2A,B). Although the decay time of sEPSCs was found prolonged in Gpr158 KO cells (t-test P = 0.047; Supplementary Table 2), further analysis of the weighted tau of decay did not reveal any difference between genotypes (WT 9.79 ± 1.42 ms, KO 10.56 ± 1.91 ms, t-test P = 0.294, data not shown). Finally, rise time of sEPSCs was similar for both groups (t-test P = 0.179). Additionally, we did not observe any differences in the frequency (t-test P = 0.470) of sIPSCs (Figures 2C,D), however a trend for a reduction in amplitudes was identified (MWU P = 0.058). Inhibitory receptor kinetics were equal between genotypes (t-test, decay P = 0.995, rise P = 0.314). Taken together, our data from CA1 pyramidal neurons do not reveal any substantial difference in excitatory and inhibitory synaptic neurotransmission that could alter neuronal communication and impact behavior.
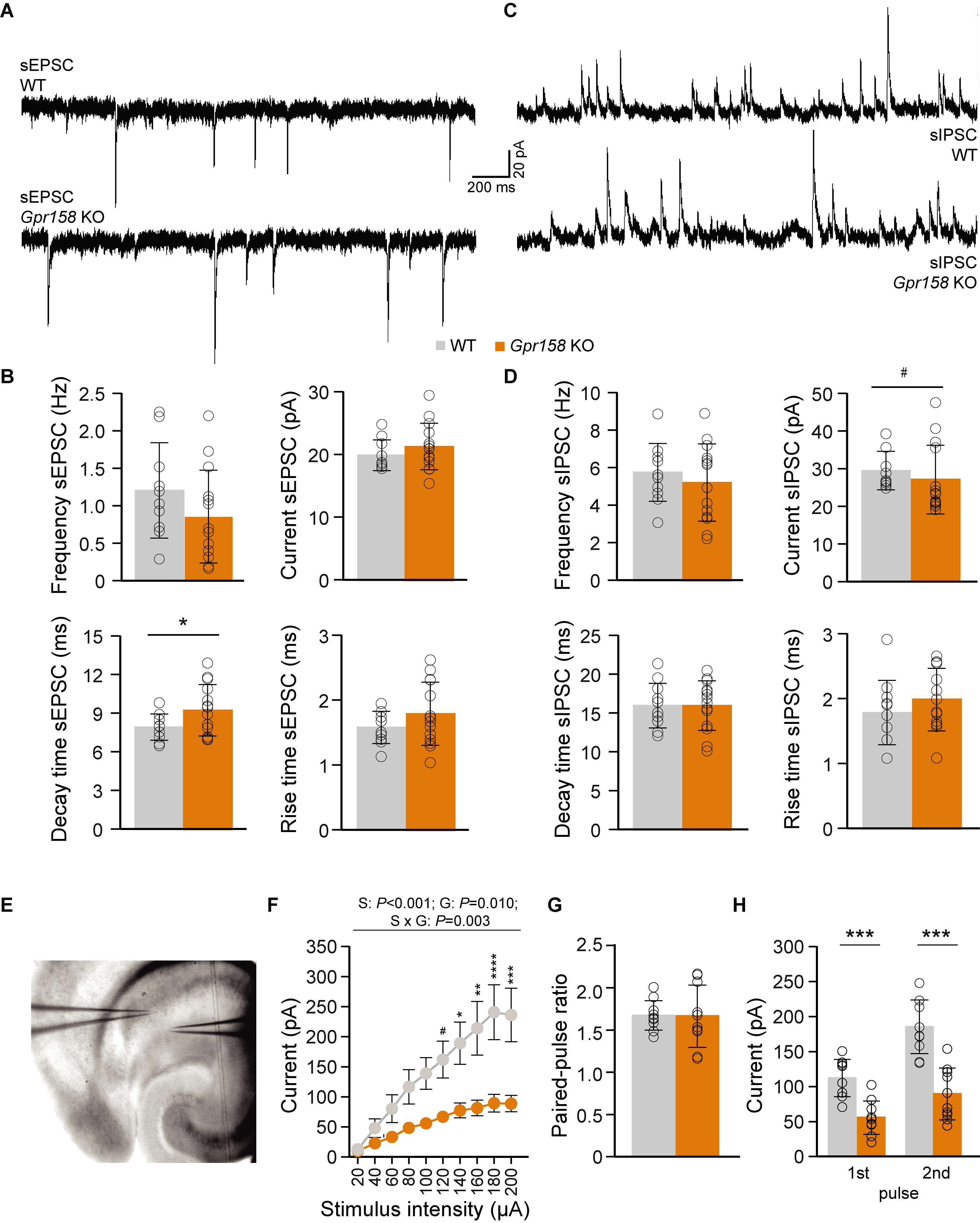
Figure 2. Electrophysiological assessment of Gpr158 KO CA1 pyramidal incoming synaptic transmission. (A–D) A cesium-gluconate-based intracellular was used to record both excitatory (A,B, WT n = 10 cells from 4 animals, KO n = 14 cells from 4 animals) and inhibitory (C,D, WT n = 11 cells from 4 animals, KO n = 14 cells from 4 animals) postsynaptic currents from CA1 pyramidal cells. Example traces of xcitatory (A) and inhibitory activity (C) recorded from WT and Gpr158 KO mice. Frequency, amplitude, and rise time of sEPSCs were equal between genotypes (B). A small increase in sEPSC decay time was observed in Gpr158 KO (Supplementary Table 2). No difference was observed in sIPSC frequency, decay time, or rise time (D). However, a trend for decreased sIPSC amplitude was observed in Gpr158 KO (Supplementary Table 2). (E–H) For PPR recordings, a potassium-gluconate based intracellular was used for the recording pipette, while the stimulating pipette was loaded with aCSF and placed in a location where a clear unisynaptic response could be generated in the postsynaptic cell. Example configuration for I/O curve and PPR recordings (E; WT n = 10 cells from 4 animals, KO n = 10 cells from 3 animals). An I/O stimulation curve was generated by gradually increasing stimulation intensity in steps of 20 μA (F). A clear reduction in postsynaptic responses was observed in Gpr158 KO upon Schaffer collateral stimulation [stimulus (S): P < 0.001, genotype (G) P = 0.010; interaction P = 0.003] that became significant from 140 μA onward. A succession of two stimulations separated by 50 ms was applied at stimulation intensity approximately half-maximal to that generated during the I/O curve. The ratio between the 1st and 2nd response amplitude was equal between genotypes (G). The raw amplitudes recorded during half-maximal stimulation during the PPR protocol differed significantly (H). For panel (F) data are presented as mean ± SEM. For all other panels data are presented as mean ± SD with individual data points indicated. Asterisks and octothorpe indicate the level of significance between WT and KO assessed by Student’s t-test or MWU (Supplementary Table 2), #P ≤ 0.100; ∗P ≤ 0.050; ∗∗∗P ≤ 0.001.
Normal Release Probability but Reduced Schaffer Collateral-Mediated Responses in Gpr158 KO Mice
A central feature in synaptic transmission is its ability to adapt to rapidly successive stimuli, which is regulated mainly by presynaptic mechanisms (Zucker and Regehr, 2002). The frequency of stimulation combined with synapse-specific release probabilities can filter activity, by allowing information transfer only when the appropriate conditions are met (Abbott et al., 1997; Fortune and Rose, 2001; Zucker and Regehr, 2002). To determine the excitability of CA3 to CA1 connections, an input-output (I/O) stimulation curve was generated, by stimulating Schaffer collaterals (SC) with increasing intensity, while recording from CA1 pyramidals (Figure 2E). Gpr158 KO responses exhibited robust reduction for nearly all stimulation intensities, reaching plateau at below 100 pA, while WT responses plateau at just below 250 pA (Figure 2F, 2W-RMANOVA: genotype P = 0.01, stimulation P < 0.001, interaction P = 0.003; Supplementary Table 2). The reduction in responses was not due to differences in the placement of the stimulating electrode, since the distance between recording and stimulating electrodes was equal between groups (WT 77.51 ± 19.13 px, KO 86.39 ± 18.46 px, P = 0.305, data not shown). Subsequently, two successive stimuli were delivered, separated by 50 ms, at half-maximal stimulation intensities; current injected was not significantly different between genotypes (WT 90 ± 21.60 μA, KO 102 ± 12.76 μA, P = 0.247, data not shown). Surprisingly, the paired-pulse ratio (PPR), was equal between the two genotypes (Figure 2G, t-test P = 0.945), however, the raw amplitudes recorded during the PPR protocol where robustly different between WT and Gpr158 KO mice (Figure 2H, t-test, 1st Pulse P < 0.0001, 2nd Pulse P < 0.0001). Therefore, our data suggest that even though presynaptic release probability and machinery could be intact, compromised SC integrity, synapse numbers, or vesicle load could drive the attenuated SC-CA1 responses.
Gpr158 Is Implicated in Hippocampal Neuronal Morphology
Dendritic architecture is central to the neuron’s capacity to integrate signals, can influence cell intrinsic properties, and thus can impact learning and memory (Bekkers and Häusser, 2007). To that end, we investigated neuronal morphology both in vitro and ex vivo by analyzing WT hippocampal primary culture morphology after Gpr158 knock-down (KD) (Figure 3), and by analyzing biocytin-loaded CA1 pyramidals in Gpr158 KO and WT hippocampal slices (Figure 4). We used shRNA KD as approach because in vitro analysis of Gpr158 KO primary neurons was hampered due to a severe reduction in viability (up to 95%).
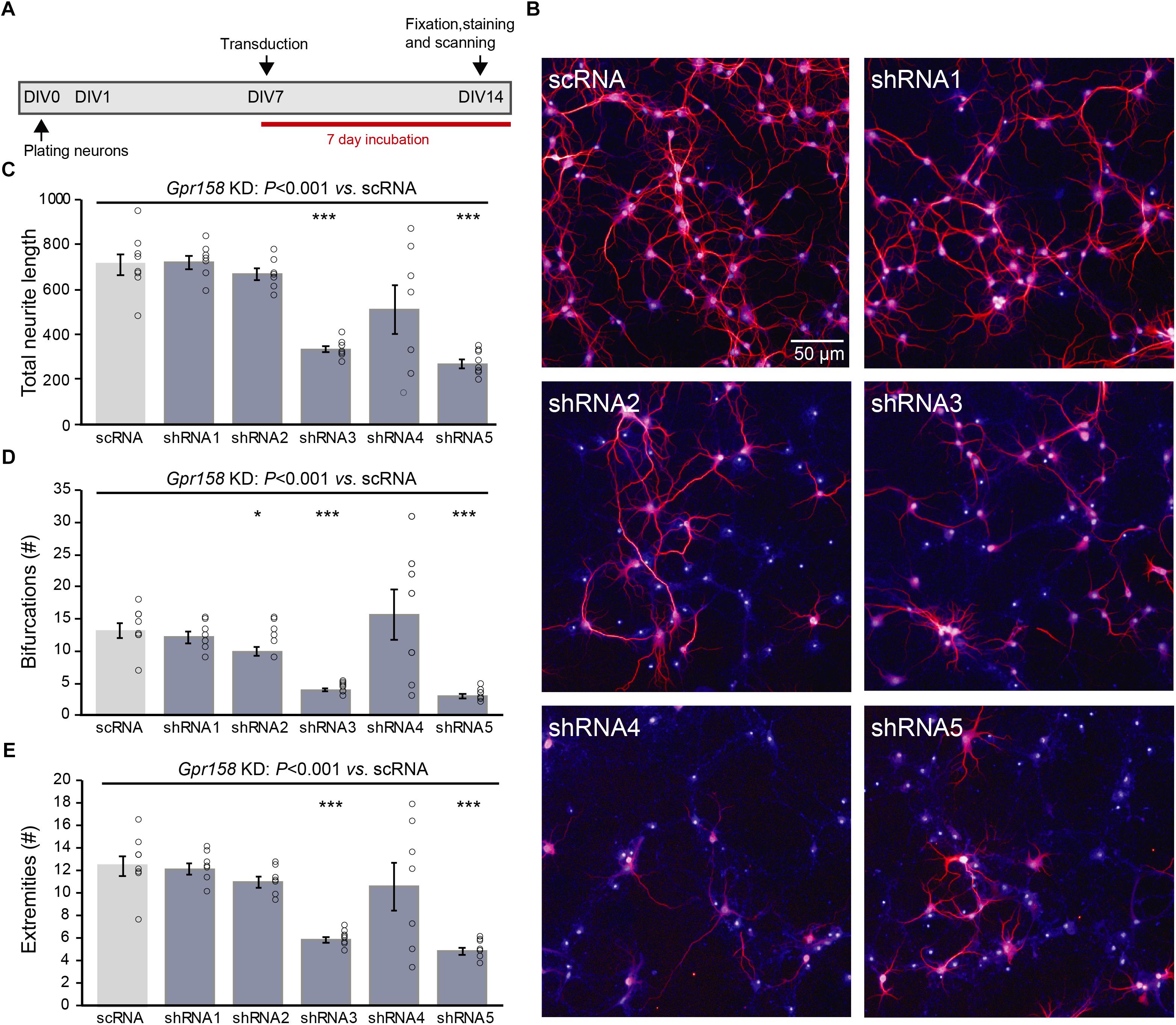
Figure 3. Gpr158 knock down leads to reduced neuronal outgrowth of hippocampal primary neurons. (A) Experimental set-up of in vitro Gpr158 knockdown (KD). Viral transduction with independent shRNAs occurred from DIV7 to DIV14, and morphological analysis occurred at DIV14. (B) Representative images for control (scRNA) and Gpr158 KD (shRNA1–5) neurons (red: MAP2, blue: nuclei). (C–E) Morphological analysis after Gpr158 KD at DIV14 (n = 7–8 wells; see Online Resource 6) shows an overall effect of the shRNA treatment for all parameters [neurite length (C), number of bifurcations (D) and number of extremities (E)], as tested by Kruskal–Wallis (P < 0.001). Whereas shRNA3 affected all 4 parameters, the other shRNAs had a more limited effect for 1–3 of these parameters. Apart from a significant downregulation in number of protrusions, shRNA5 gave a similar strong effect as shRNA3, indicative of a Gpr158-related effect. Data are presented as mean ± SEM with individual data points indicated. Asterisks indicate significant differences compared with control (scRNA) assessed by Student’s t-test (Supplementary Table 3), ∗P ≤ 0.050; ∗∗∗P ≤ 0.001.
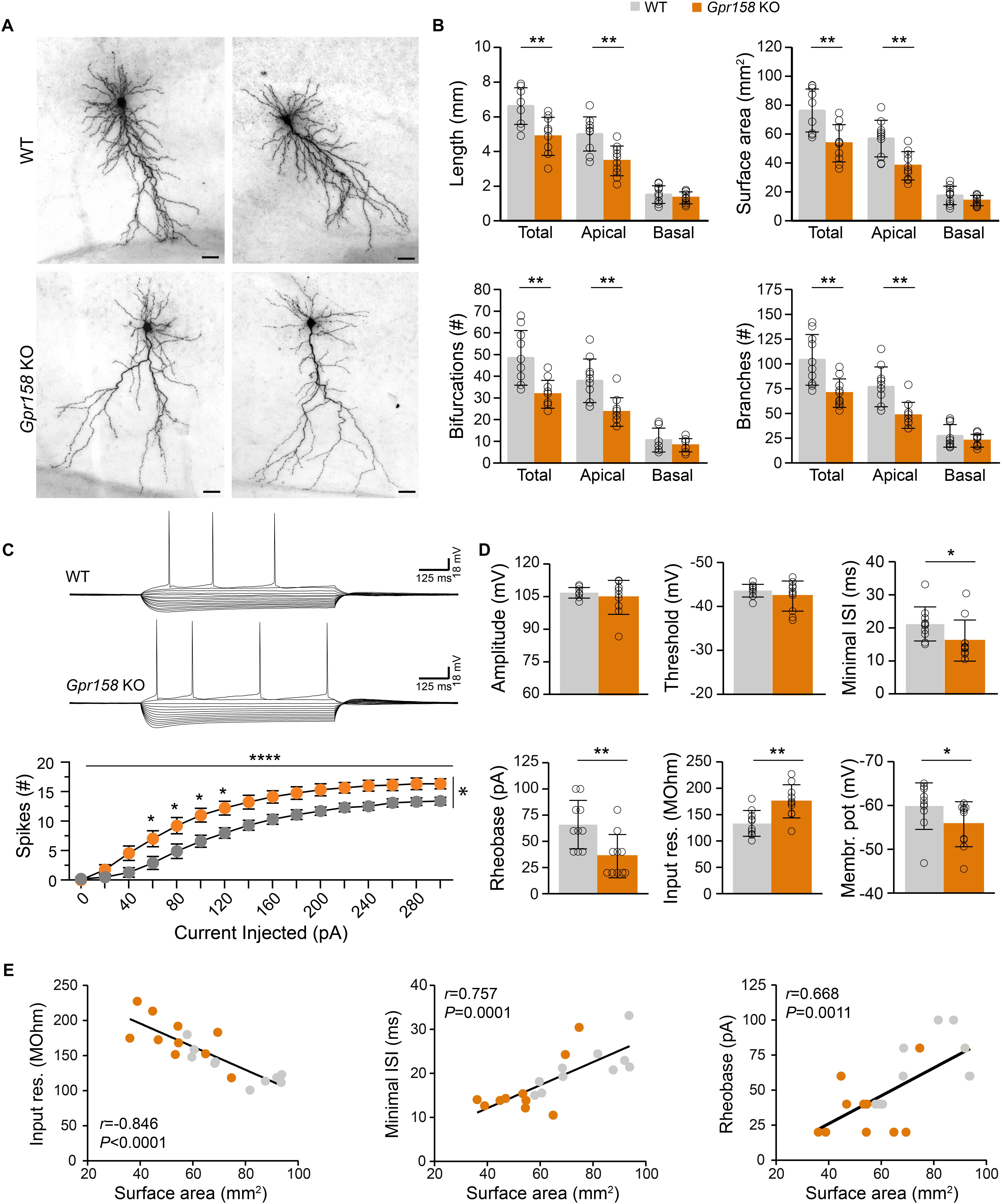
Figure 4. Reduced dendritic architecture supports hyperexcitability of Gpr158 KO CA1 pyramidal neurons. (A) Examples of CA1 pyramidal cells loaded with biocytin during electrophysiological recordings from WT (n = 10 cells from 4 animals) and Gpr158 KO (n = 10 cells from 3 animals) hippocampal slices. Cells were subsequently reconstructed and their basic morphological measures were extracted and analyzed (Online Resource 8). (B) Total dendritic length and surface area were significantly reduced in Gpr158 KO CA1 pyramidals and the effect was specific to the apical compartment. A significant reduction in total and apical but not basal compartments was documented for the number of bifurcations and number of branches. (C,D) For each reconstructed cell, the full action potential profile was generated and analyzed. (C) Example traces of WT and Gpr158 KO action potential profiles generated by current injections in steps of 20 pA. Note that increased number of spikes were observed for all current steps tested in the Gpr158 KO. (D) Action potential amplitude and threshold was unaffected by genotype. Minimum inter spike interval and rheobase were significantly reduced in Gpr158 KO pyramidal cells. In addition, input resistance was increased in Gpr158 KO and the membrane potential was more depolarized in Gpr158 KO pyramidal cells. (E) Significant Pearson correlations (r) and linear regression (Supplementary Figure 4) between cell surface area and input resistance (r = −0.846, P < 0.0001), minimum inter spike interval (r = 0.757, P = 0.0001), and rheobase (r = 0.668, P = 0.0011). Data are presented as mean ± SD; individual data points are indicated. Asterisks indicate significant differences compared with WT cells assessed by Student’s t-test or MWU (Supplementary Tables 4, 5), ∗P ≤ 0.050; ∗∗P ≤ 0.010; ∗∗∗∗P < 0.001.
Hippocampal primary neurons were initially transduced from day in vitro 1 (DIV1) until DIV14, with one of five different shRNA constructs (shRNA1-5) targeting different parts of Gpr158 mRNA sequence, or a scrambled control shRNA (scRNA). This reduced cell viability to a similar extent as in Gpr158 KO neurons. Hence, we transduced from day in vitro (DIV) 7 until DIV14 instead (Figures 3A,B). This window for transduction (DIV7–DIV14) seemed appropriate based on the in vitro developmental gene expression of Gpr158 (Supplementary Table 3), and the efficiency of shRNA-mediated KD of Gpr158 by DIV14 (Supplementary Figure 3). The KD effect as measured by gene expression was small, possibly due to an incomplete transduction, or by acting of these shRNAs on ribosomal entry of transcripts for translation, leaving mRNA largely intact. As such, the obtained data on the effect of the shRNA KD might be underestimated, in the context of the large effects on morphology.
Different parameters were measured with respect to in vitro neuronal morphological development: total neuron number, total neurite length, number of protrusions from the soma, their bifurcations, and the number of extremities resulting from this (Figure 3, Supplementary Figure 3, and Supplementary Table 3). Although Gpr158 KD reduced the survival of neurons for all shRNAs, shRNA4 and shRNA5 were the strongest affected, showing the largest response variability. For shRNA1-3 neuronal survival was compromised less, hence allowing for full morphological analyses (Online Resource 7, Kruskal–Wallis P < 0.001). All morphological parameters measured were affected by KD of Gpr158 (Figures 3C–E and Supplementary Figure 3, Kruskal–Wallis, all P < 0.001). Specifically, neurite length was reduced by shRNA3 (t-test P < 0.001) and shRNA5 (t-test P < 0.001; Supplementary Figure 3). The number of bifurcations was reduced by shRNA2 (t-test, P = 0.039), shRNA3 (t-test, P < 0.001), and shRNA5 (t-test, P < 0.001; Supplementary Figure 3). Finally, the resulting number of extremities was reduced by shRNA3 (t-test, P < 0.001) and shRNA5 (t-test, P < 0.001; Supplementary Figure 3). Taken together, in the reduced conditions of primary culture, Gpr158 KD disturbed development of neuronal morphology.
Polarized Reductions in Gpr158 KO CA1 Pyramidal Cell Dendritic Architecture
Given the impact that Gpr158 KD had on neuronal morphology in vitro, we subsequently reconstructed the dendritic morphology from biocytin-loaded CA1 pyramidal neurons in hippocampal slices from adult Gpr158 KO and WT mice (Figure 4A). In line with the observations in vitro, Gpr158 KO CA1 pyramidals exhibited reduced total dendritic length (Figure 4B, t-test P = 0.002), as well as total surface area (Figure 4B, t-test P = 0.002; Supplementary Table 4). Moreover, the observed reductions in total length and surface area were supported by reductions specifically in the apical but not the basal dendritic compartment (Figure 4B, t-test: length [apical P = 0.001, basal P = 0.346], surface [apical P = 0.002, basal P = 0.136]). Furthermore, the general complexity of dendritic arborizations in Gpr158 KO was reduced, reflected by the decrease in the total number of bifurcations per cell (Figure 4B, t-test: total P = 0.002, apical P = 0.001, MWU: basal P = 0.481). In contrast to the variable effect observed in vitro, the number of dendrites emerging from the cell body was equal (WT 7.40 ± 1.78, KO 7.70 ± 1.95, t-test P = 0.723, data not shown). The total number of branches was significantly reduced in Gpr158 KO and the effect was specific to the apical dendritic compartment (Figure 4B, t-tests: total P = 0.003, apical P = 0.001; MWU: basal P = 0.529). Thus, the biocytin reconstructions of Gpr158 KO CA1 pyramidal neurons ex vivo, illustrated reductions in dendritic architecture, paralleling in vitro observations, and localized the effect specifically to the apical dendritic compartment.
Reduced Dendritic Architecture Correlates With Augmented Intrinsic Excitability of Gpr158 KO CA1 Pyramidal Cells
Neuronal morphology contributes substantially to the integrative properties of neurons, and can have a major influence on neuronal firing by altering intrinsic cell properties (Bekkers and Häusser, 2007; Kowalski et al., 2016). Given the robust changes in neuronal architecture we documented both in vitro and ex vivo, we analyzed the action potential (AP) profiles from WT and Gpr158 KO CA1 pyramidal cells in hippocampal slices (Figure 4C) used for morphological assessment. For all positive current steps tested, the number of action potentials elicited was higher in the Gpr158 KO CA1 pyramidals compared to their WT littermates (Figure 4C, 2W-RMANOVA: genotype P = 0.010, current injected P < 0.0001, interaction P < 0.0001; Supplementary Table 4). AP amplitude (Figure 4D, P = 0.439, Supplementary Table 4) and threshold (Figure 4D, P = 0.311) did not differ for genotype. However, both the minimum inter-spike interval (Figure 4D, ISI, P = 0.015) and minimum current required to elicit the first AP (Figure 4D, rheobase, P = 0.009) were reduced, underlying increased excitability of Gpr158 KO pyramidals. Moreover, the input resistance (Ri) of Gpr158 KO cells was significantly increased, further substantiating and promoting their increased excitability (Figure 4D, P = 0.004). Neuronal cell membrane time constant did not differ between the two groups (WT: 29.92 ± 4.84 ms, KO: 27.77 ± 4.42 ms, P = 0.312, data not shown). Finally, the resting membrane potential of Gpr158 KOs was found more depolarized than in WT (Figure 4D, P = 0.035). Notably, ISI was also significantly reduced when cells were clamped at −70 mV (WT: 24.8 ms, KO: 16.5 ms; P = 0.001) and rheobase was reduced as a strong trend (WT: 90 pA, KO: 75 pA; P = 0.055) (Supplementary Figure 4 and Supplementary Table 4), excluding confounding effects that our reported difference in resting membrane potential could cause.
We then applied PCA on the morphological and electrophysiological parameters collected from the same cells to dissect the most significant determinant underlying the changes observed (Supplementary Figure 5). For all components, cell surface area was the variable with the most significant contribution (Supplementary Figure 5, first 3 components shown). A subsequent correlation matrix of surface area against all other morphological and electrophysiological variables demonstrated significant correlations with all morphological measures, with cell excitability, and with PPR raw amplitudes (Supplementary Figure 5). However, upon multiple comparison correction, the correlation between surface area and raw PPR amplitudes did not retain significance, while the rest retained high statistical significance (Supplementary Figure 5). Subsequent linear regression demonstrated that surface area negatively correlated with Ri (Figure 4E, r = −0.846, P < 0.0001), whereas a positive relationship was demonstrated with minimum ISI (Figure 4E, r = 0.757, P < 0.001), and rheobase (Figure 4E, r = 0.668, P = 0.001). Taken together, our data demonstrated that Gpr158 KO CA1 pyramidal cells exhibit increased excitability, and that this increase might be driven by their notable reduction in dendritic architecture.
Reduced Architecture of CA1 Pyramidal Neurons Correlates With MWM Learning Deficits
Changes in the excitability of neurons can alter information flow, whereas changes in dendritic architecture can also impact the integrative capacity of neurons (Magee, 2000; Bekkers and Häusser, 2007; van Elburg and van Ooyen, 2010). Moreover, dendritic complexity appears to increase with evolution and with increasing cognitive demands, with human prefrontal pyramidals exhibiting the largest cortical dendritic complexity (Elston et al., 2001; Mohan et al., 2015). Furthermore, increasing dendritic complexity of human cortical pyramidals appears correlated with increasing intelligence quotient, in a small sample of surgically resected brain tissue (Goriounova et al., 2018). Since the electrophysiological datasets reported here were generated from mice that underwent behavioral assessment (Supplementary Figure 2), we then averaged the morphology data of all cells collected to generate an overview of dendritic architectures for n = 6 animals per genotype (Figure 5A). Analysis of the combined morphologies further substantiated the robust reduction in dendritic architecture of Gpr158 KO hippocampal neurons [Figure 5A, 2W-ANOVA, genotype (G) effect; length P = 0.002; surface P < 0.001; bifurcations P < 0.001; branches P = 0.001; Supplementary Table 5]. Furthermore, the effect was specific to the apical but not the basal dendritic compartment [Figure 5A, 2W-ANOVA, dendritic compartment (DC) effect; for all measures P < 0.001]. Apical (P < 0.001) but not basal dendritic length (all P > 0.300) was reduced in Gpr158 KO mice, as well as surface area, number of branches, and number of dendritic bifurcations in the same manner (Supplementary Table 5).
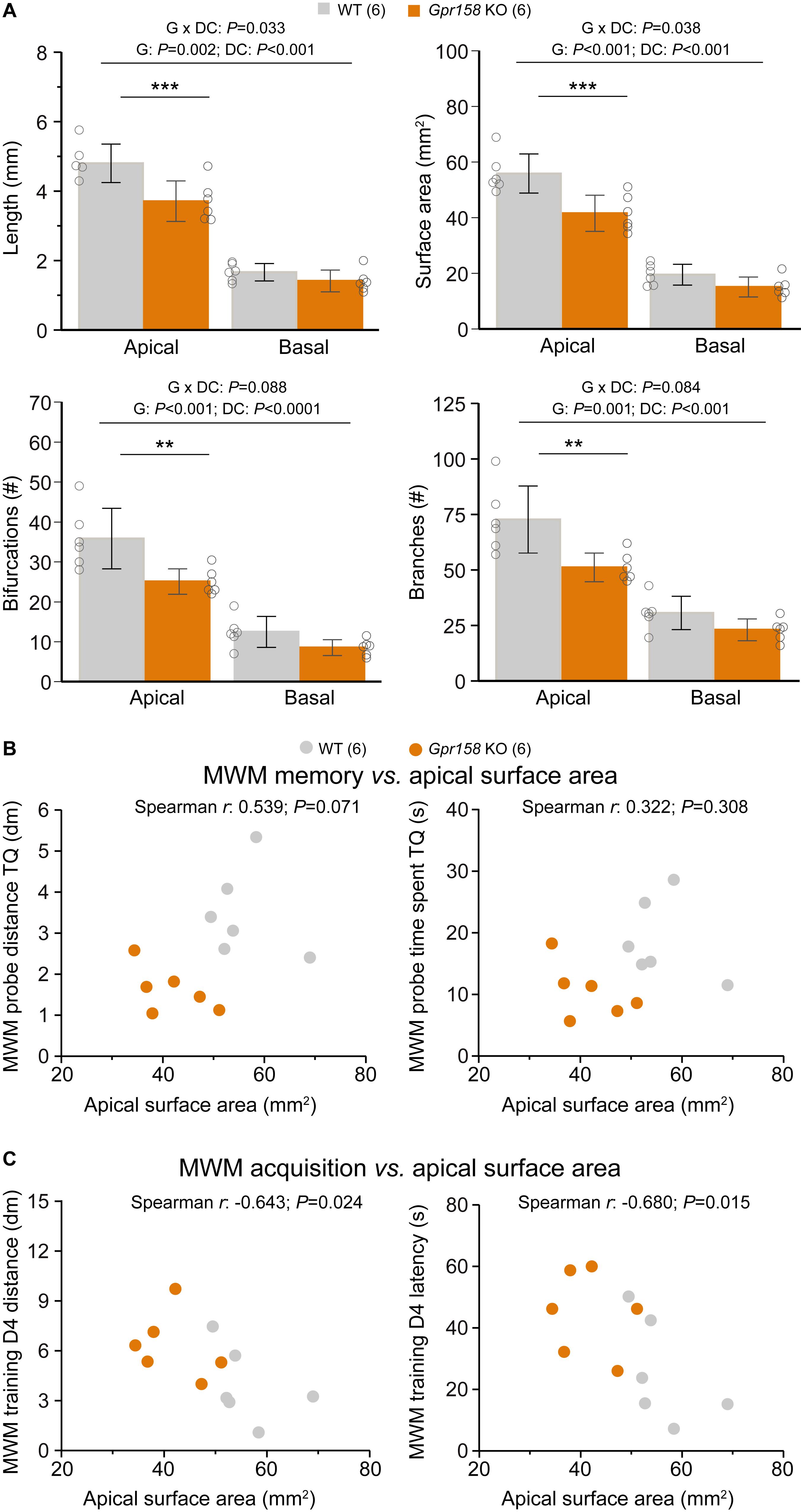
Figure 5. Reduced dendritic architecture negatively impacts learning during MWM training. (A) Cell morphology from all the electrophysiological data sets described were averaged to generate an overview of hippocampal dendritic architecture per animal (WT n = 6 animals, 18 cells averaged, KO n = 6 animals, 17 cells averaged). Apical but not basal hippocampal dendritic length, surface area, bifurcations and branches were significantly reduced in Gpr158 KO animals. (B) Apical surface area did not (significantly) correlate with either the distance covered (P = 0.071) or the time spent (P = 0.308) in the target quadrant (TQ) during the MWM probe test assessing long-term memory. (C) During acquisition of the MWM task, the apical surface area did significantly and negatively correlate with both the distance covered to reach the platform (P = 0.024) and the latency (P = 0.015) reaching the platform on the last training day (D4). Data are presented as mean ± SD with individual data points indicated. Asterisks indicate significant differences between WT and KO assessed by Student’s t-test (Supplementary Table 5), ∗∗P ≤ 0.010; ∗∗∗P ≤ 0.001.
Given the emergence of surface area as major determinant for the morphological and electrophysiological differences observed, we further probed its relationship with MWM parameters for the entire group of mice. For the MWM probe test, neither the distance covered in the TQ (Figure 5B, Spearman r = 0.538, P = 0.071), nor the time spent in the TQ (Figure 5B, Spearman r = 0.322, P = 0.308) were significantly correlated with the animal’s apical dendritic surface area. However, for the acquisition of the MWM task, surface area did significantly and negatively correlate with both the distance covered to reach the platform (Figure 5C, Spearman r = −0.643, P = 0.024), and with the latency to reach the platform (Figure 5C, Spearman r = −0.680, P = 0.015). Therefore, the reduction in general dendritic architecture in Gpr158 KO CA1 neurons, although not directly affecting long-term MWM memory, demonstrated a significant and negative correlation with the acquisition of this spatial memory task.
Discussion
In this study we report that mice deficient of Gpr158 exhibit impairments in the acquisition and retrieval of spatial memory in the MWM paradigm, as well as deficits in the learning of an extinction memory in the PA task. Although hippocampal CA1 basal excitatory and inhibitory neurotransmission was found normal, we report a remarkable reduction in the excitability of SC synapses onto CA1 pyramidals, whereas we observed a potential compensatory increase in the intrinsic excitability of these neurons. Strikingly, both in vitro and ex vivo we observe a large effect of reduced Gpr158 levels on dendritic morphology. Ex vivo, the dendritic architecture reductions of CA1 neurons was specific for the apical but not the basal compartment of these cells. Finally, the reduced apical complexity of these neurons appears to support the spatial memory acquisition deficits in these mice, since reduced neuronal surface area positively correlated with poor performance during acquisition of the MWM task.
Behavioral Analysis
For Gpr158 KO, a robust anxiety phenotype was detected previously (Khrimian et al., 2017). Here, we do not report such anxiety-like phenotype, neither in a novel open field, nor when placed in the fear conditioning box prior to conditioning. This might relate to the fact that the genetic background of mice of the Knockout Mouse Project (KOMP) repository is C57BL/6NTac whereas Khrimian et al. (2017) kept their mice on a mixed 129-Sv/C57BL/6J background. The 129-Sv background is known for its anxiety (Tarantino et al., 2000; Rodgers et al., 2002), even when used in a mixed background (Võikar et al., 2001), and anxiety can be a confounding factor in cognitive performance (Brooks et al., 2005).
Neither did we find an anxiolytic phenotype as previously measured in the EPM, TST and FST (Sutton et al., 2018) using the same KO line and strain, when considering the classical open field test, or when analyzing locomotor data from other tests. Given the observed genotype effect only in acquisition of safety memory, but not in initial acquisition of the avoidance memory in the same task, our read-out on cognitive function is unrelated to the emotional impact of the test. As such, the lack of a baseline anxiolytic phenotype allows for explicit interpretation of the different contextual fear paradigms. Initial fear acquisition and acquisition of safety memory (see below) likely represent different forms of learning, in which the first is apparently not dependent on the Gpr158 circuitry, but the latter, as well as acquisition and recall of spatial memory is.
Acquisition of a Safety Memory and Spatial Memory Are Affected in Gpr158 KO Mice
Non-spatial memory acquisition and expression, as measured in the PA test, and in contextual fear conditioning with context as the foreground stimulus, was not affected in Gpr158 KO mice. However, normalization of avoidance behavior after forced exposure to the conditioning context was delayed in Gpr158 KO mice. This safety learning procedure has been shown to rely on hippocampus CB1 receptors (Micale et al., 2017), in contrast to response extinction learning, i.e., repeated execution of the avoided response in absence of negative consequences, that is largely cortically mediated. Single intrahippocampal injection of cannabinoid-related compounds prior to safety learning acquisition could alter the effect of safety learning (Micale et al., 2017). Additionally, these compounds affected the propagation of signaling from the DG via area CA3 to the CA1 subfield upon perforant pathway stimulation (Micale et al., 2017). Thus, the reduced SC-mediated responses we observed in hippocampal slices of Gpr158 KO mice might indicate that information processing within the trisynaptic circuitry of the hippocampus is disturbed. As such this precipitates not only in impaired safety learning, but also in poor spatial memory acquisition and expression, which is also dependent on balanced cannabinoid signaling in the hippocampus (Abush and Akirav, 2010).
During training in the MWM paradigm, Gpr158 KO mice exhibited robust deficits in task acquisition, demonstrated by their increased distance covered and time spent to reach the platform. This is in line with a previous observation of impaired MWM acquisition in these mice (Khrimian et al., 2017). We additionally report that Gpr158 KO mice demonstrated a long-term spatial memory deficit, highlighted by the reduced performance during the MWM probe trial. During the probe test, the platform is removed and the animal is requested to rely on spatial information to relocate the area of the previous escape platform after a 24 h interval. The hippocampus plays a major role in spatial learning, with involvement of both the CA1 and CA3 regions (Florian and Roullet, 2004), in which Gpr158 is expressed (Khrimian et al., 2017; Condomitti et al., 2018). Theta frequency coupling between CA3 and CA1 is enhanced during MWM learning, when there is a clear improvement in task performance (Hernández-Pérez et al., 2016). The changes in CA1 morphology and excitability we report along with the changes in CA3 physiology (Condomitti et al., 2018) could alter such coupling, hence impacting learning in Gpr158 KO mice. Although the CA3 region is involved in spatial memory acquisition, it is not required for long-term memory recall during the probe test (Florian and Roullet, 2004) that we here report as also being affected in Gpr158 KO mice. Enhanced CA1 LTP, is shown to improve MWM probe test performance (Okada et al., 2003). Although not yet demonstrated to be different in the CA1 in Gpr158 KO mice, LTP deficits in the CA1 region akin to those described in CA3 (Condomitti et al., 2018) are likely – as discussed below, and could support deficits in spatial memory acquisition.
Reduced Dendritic Architecture Supports Spatial Memory Learning Deficits
Changes in spine density and size have been previously demonstrated in Gpr158 KO mice in the CA3 area of the hippocampus (Condomitti et al., 2018), in a pathway-specific manner. Despite the increase in spine density, there was mostly an increase in thin spines, and at the ultrastructural level, synapses showed reduced active zone and PSD length. This suggests that at least in the MF-CA3 pathway Gpr158 KO mice show an immature morphological phenotype, similar to our in vitro data. Whether similar changes in dendrite length occur in the CA3 as they do in the CA1 remains to be determined.
Central to our observations are the striking reductions in dendritic architecture and complexity of Gpr158 deficient neurons in vitro as well as ex vivo in the CA1 region. Albeit that the effects in vitro in hippocampal cultures was variable across the 5 different shRNAs used, which may include possible off-target effects, this morphological phenotype was largely replicated ex vivo in reconstructed CA1 pyramidals within hippocampal slices. Complex dendritic architecture is essential to proper learning and memory (Elston et al., 2001). Dendritic complexity increases with evolution and with increasing cognitive demands (Tronel et al., 2010), and impairments in dendritic architecture have been observed in disorders with reduced cognitive capacities (Kulkarni and Firestein, 2012). Moreover, dendritic structure impacts the integrative ability of neurons, through pathway-specific distinct input domains, coincidence detection, synaptic scaling of distal inputs, and targeted dendritic inhibition, to name a few (Spruston, 2008).
Importantly, the reduced architecture we observed, negatively correlated with the last days of spatial memory acquisition in the MWM paradigm but not significantly with probe test performance. Spatial learning itself has been shown to enhance dendritic complexity by increasing length, bifurcations, and branches of DG new born granule cells, in rats trained in MWM and in the delayed matching-to-place task (Tronel et al., 2010). Mostly these learning-induced changes are relatively small and short-lasting. Instead, genetic-induced mutations that affect dendritic architecture during development could have a higher impact. Hence mutation-associated learning deficits could arise through changing specific plasticity mechanisms. Mutant mice for tyrosine kinase Fyn, involved in normal cell physiology, exhibit deficits in MWM acquisition and recall, along with impairments in CA1 LTP, and reduced compaction and organization of CA1 apical dendrites (Grant et al., 1992). In addition, mice double mutant for cysteine proteases calpain 1 and 2 exhibit impaired spatial memory in MWM, in the absence of visual or motor deficits or anxiety-like behavior in the open field (Amini et al., 2013). Electrophysiological analysis revealed that CA1 pyramidal dendritic complexity, LTP, and CA3 to CA1 I/O curve were robustly reduced in these mutants (Amini et al., 2013). It is therefore likely that the reduced dendritic architecture of Gpr158 KO CA1 pyramidals we report here could faithfully underlie the deficits in spatial memory acquisition, potentially through impairments in the amplitude and temporal course of EPSPs and induction and expression of LTP.
In the SC to CA1 network, brain-derived neurotrophic factor (BDNF) is required for the induction and maintenance of LTP brought about by repeated dopamine stimulation in apical but not in basal dendrites (Navakkode et al., 2012), and hippocampal BDNF levels in Gpr158 KO are found reduced (Khrimian et al., 2017). To date, impaired PPR and LTP in Gpr158 KOs has been reported for the MF to CA3 pathway (Khrimian et al., 2017; Condomitti et al., 2018), but LTP has not been investigated for the CA3 to CA1 pathway. Although we report normal PPR for this pathway, given the robust reduction in CA3 to CA1 stimulation and deficient dendritic architecture we observe, along with reduced hippocampal BDNF levels (Khrimian et al., 2017), CA3 to CA1 LTP could be impaired in Gpr158 KO as well, and as such be driving impairments in spatial memory.
Normal Basal Synaptic Transmission and Increased Excitability of Gpr158 KO CA1 Pyramidals
Along with the changes in dendritic architecture, we observed an overall increase in the excitability of the reconstructed cells. Gpr158 KO pyramidals elicited more action potentials than WT pyramidals for all positive current steps. Furthermore, rheobase as well as minimal ISI were significantly reduced in Gpr158 KO CA1 pyramidals, independent of the resting membrane potential, whereas input resistance was increased and resting membrane potential was more depolarized. Moreover, PCA revealed that these changes were strongly correlated with the cell surface area. The contribution of the dendritic tree to neuronal excitability has been elegantly demonstrated, whereby pinching or severing neuronal dendrites significantly increased action potential frequency and input resistance, while reducing rheobase (Bekkers and Häusser, 2007). As such, our observations of increased CA1 pyramidal excitability in Gpr158 KOs could be governed by their reduced dendritic morphology.
The increased excitability observed in Gpr158 KO could possibly compensate for the reduced SC drive, as previously reported in an epilepsy model (Dinocourt et al., 2011). A hallmark of CA1 neurons is the ability to show action potential backpropagation, which is important for the integration of synaptic input and the induction of synaptic plasticity. Dendritic architecture, and specifically the number of dendritic branchpoints, showed a strong relationship with the functional threshold of backpropagation (Vetter et al., 2001), with lower number of branchpoints having a lower requirement of Na-channels to induce backpropagation as shown by computational modeling. As such, a lower threshold to induce backpropagation could compensate for the reduced SC drive. The reduced SC drive, as measured by the lowered I/O response, in Gpr158 KO animals could relate to putative changes in the total number and/or the density of excitatory synapses (akin to that observed in CA3, see above), although this conclusion is not supported by a change in the sEPSC frequency. Alternatively, changes in passive and active properties of CA1 PC dendrites might play a role. Taken together, these morphological changes could contribute to the integrative capacity of CA1 pyramidal neurons.
Basal synaptic transmission onto CA1 pyramidal cells appears unaffected in Gpr158 KO hippocampus, with no notable changes in either spontaneous excitatory or inhibitory frequency and amplitude observed. This is in contrast the reductions observed in sEPSC frequency and amplitude in Gpr158 KO CA3 (Condomitti et al., 2018). Along with the reductions in evoked AMPA and NMDA CA3 currents (Condomitti et al., 2018) as well as the reduced SC mediated responses we observe, it is conceivable that when the network is further challenged, or when miniature release is assessed, such changes may become apparent in CA1 as well. Albeit, reduced CA3 to CA1 input does not always impact basal synaptic transmission (Grant et al., 1992; Amini et al., 2013). In the mPFC L2/3, increased spontaneous excitatory frequency (Sutton et al., 2018) is observed alongside increased excitability (Song:2019ft). In particular the latter phenotype, which is linked via cAMP-dependent phosphorylation to the A-type potassium channels shows layer-specificity, highlighting the possibility of brain area and pathway-specific alternations caused by Gpr158 deficiency.
Is Gpr158 an Inducer of Presynaptic Organization in CA1 Akin to Its Role in CA3?
A major contributing factor in affecting Gpr158 KO CA1 pyramidal morphology is incoming presynaptic activity. In the rodent hippocampus, mechanical perforant pathway denervation leads to sustained reductions in dendritic length and complexity of the postsynaptic dentate gyrus granule cells (Vuksic et al., 2011). Importantly, these reductions were observed primarily in the outer molecular layer where denervation had occurred. Additionally, deafness or facial nerve lesions resulted in pathway-specific reductions in dendritic morphology, in either primary auditory cortex (Bose et al., 2010), or primary motor cortex pyramidal cells (Urrego et al., 2015), respectively. The attenuated responses of SC stimulation we report, along with the reported reduction in CA3 pyramidal excitability (Condomitti et al., 2018), could lead to reduced SC output. Yet, our reconstruction of Gpr158 KO CA1 pyramidals revealed that structural reductions were specific to the apical but not basal dendritic compartments. Consequently, attenuated excitation of CA1 apical dendrites by SC might promote the compartment-specific morphological alternations we report here. However, whether this stems from pathway-specificity and how such specificity is obtained is not clear, as CA3 SC project both to apical and basal dendrites. Albeit, Gpr158 could be required for the formation of SC to apical but not basal CA1 dendritic synapses.
In the MF-CA3 pathway, Gpr158 functions as a post-synaptic organizer (Condomitti et al., 2018), where it has a specific role in shaping synapse morphology and function in apical dendrites within the MF-CA3 pathway, but not within the CA3-CA3 recurrent pathway. This is largely ascribed to the enriched subcellular localization of Gpr158 to apical dendrites in the stratum lucidum (Condomitti et al., 2018). With the specific effect in the apical part of CA1 dendrites in Gpr158 KO mice, one could assume that subcellular localization of Gpr158 in CA1 neurons is similar to that of CA3 neurons. As such, it raises the possibility that the CA1 dendritic phenotype is independent of SC input and instead that SC input is induced by malformed synaptic contacts due to a lack of normally enriched levels of Gpr158. Nonetheless, future experiments using conditional KO mice can be aimed to precisely dissect the pre- vs. postsynaptic role of Gpr158 in CA1 circuitry in more detail. Our in vitro KD data also suggest a morphological role during development of Gpr158. Transduction of Gpr158 shRNAs at hippocampal primary neurons at DIV7 showed at least in two out of five a major effect on dendritic outgrowth and maintenance in the weeks thereafter. Taken together, lack of the post-synaptic organizer Gpr158 itself, and/or the resulting altered dendritic morphology, could subsequently shape presynaptic SC input.
Recently, to the growing set of post-synaptic organizers of the hippocampus CA1 (Thakar et al., 2017) another three important factors have been added recently (Schroeder et al., 2018), i.c. Flrt2, Lrrtm1, and Slitrk1. The authors elegantly showed that these factors affect synaptic organization in a different manner, and that they are expressed in overlapping sets of neurons, with a ∼23% probability of being expressed all together at the same synapse with PSD-95. Gpr158 expression in CA1 has a temporal shift compared with that in CA3. Knowing that synapse formation starts at PD7 and continues until PD28, and that Gpr158 expression in CA1 starts at PD10-14 and reaches adult-like levels in term of CA1/CA3 ratio at PD28 (Khrimian et al., 2017; Condomitti et al., 2018), Gpr158 could well function together with the growing set of CA1 postsynaptic organizers in a subset of SC-CA1 connections. An outstanding question remains which CA3 expressed presynaptic organizer interacts with postsynaptic Gpr158 to form CA3-CA1 synapses. From the recently reported 129 interactors (Orlandi et al., 2018), 12 were extracellular matrix-related, and of these several are expressed at low-high levels in CA3 (Supplementary Table 6).
Taken together, we here showed that apart from the previously reported important role of Gpr158 in the MF-CA3 pathway, also the CA3-CA1 pathway is affected by loss of Gpr158. Specifically, we showed that CA1 dendritic morphology and synaptic function is related to the spatial learning deficit that Gpr158 KO mice display.
Data Availability Statement
All datasets generated for this study are included in the article/Supplementary Material.
Ethics Statement
All animal experiments were performed in accordance with Dutch law. The licensing agreements and protocols were reviewed and approved by the Animal Ethics Committee of the VU University Amsterdam.
Author Contributions
DÇ, RL, and SS performed the behavioral experiments. DÇ analyzed the results. TG made the hippocampal cultures. DÇ performed and analyzed the in vitro studies and was supervised by TG and SS. YG produced and tested the virus. IK performed and analyzed the electrophysiological studies as well as the morphological reconstructions. IK, DÇ, AS, KL, and SS participated in the design of the study. SS, AS, KL, and HM supervised the project. IK and SS performed full statistical analyses of the data (electrophysiology/morphology and behavior/in vitro culture, respectively) and wrote the manuscript. All authors read, helped to revise and approved the final version of the manuscript. SS confirms to have seen and approved the raw data of all experiments (Supplementary Table 7).
Funding
DÇ was supported by CognitionNet EU-ITN MEST-CT-2013-607508. IK was supported by the NWO-TOP grant (91215030). AS, HM, and SS received support from HEALTH-2009-2.1.2-1 EU-FP7 ‘SynSys’ (#242167). AS and RL were supported by the NBSIK PharmaPhenomics grant LSH framework FES0908. HM was supported by an NWO VICI grant (ALW-Vici 865.13.002) and the ERC grant BrainSignals (281443). IK and SS were supported by an NWO VICI grant (ALW-Vici 016.150.673/865.14.002). The authors declare that this study received funding from the EU, NWO, and the LSH framework. The funders had no involvement in study design, data collection and analysis, decision to publish, or preparation of the manuscript.
Conflict of Interest
AS participates in a holding that owns shares of Sylics BV.
The remaining authors declare that the research was conducted in the absence of any commercial or financial relationships that could be construed as a potential conflict of interest.
Acknowledgments
We thank Joost Hoetjes, Frank den Oudsten, Robert Zalm, Marion Sassen, as well as the AARC personnel of the VU for their technical assistance. Additionally, we would like to thank Dr. Christian de Kock for access to the morphology acquisition recourses and Dr. Oliver Stiedl for setting up the passive avoidance test. This manuscript has been released as a preprint at bioRxiv (Çetereisi et al., 2018).
Supplementary Material
The Supplementary Material for this article can be found online at: https://www.frontiersin.org/articles/10.3389/fncel.2019.00465/full#supplementary-material
References
Abbott, L. F., Varela, J. A., Sen, K., and Nelson, S. B. (1997). Synaptic depression and cortical gain control. Science 275, 220–224.
Abush, H., and Akirav, I. (2010). Cannabinoids modulate hippocampal memory and plasticity. Hippocampus 20, 1126–1138. doi: 10.1002/hipo.20711
Amini, M., Ma, C.-L., Farazifard, R., Zhu, G., Zhang, Y., Vanderluit, J., et al. (2013). Conditional disruption of calpain in the CNS alters dendrite morphology, impairs LTP, and promotes neuronal survival following injury. J. Neurosci. 33, 5773–5784. doi: 10.1523/JNEUROSCI.4247-12.2013
Baarendse, P. J. J., Van Grootheest, G., Jansen, R. F., Pieneman, A. W., Ogren, S. O., Verhage, M., et al. (2008). Differential involvement of the dorsal hippocampus in passive avoidance in C57bl/6J and DBA/2J mice. Hippocampus 18, 11–19. doi: 10.1002/hipo.20356
Bekkers, J. M., and Häusser, M. (2007). Targeted dendrotomy reveals active and passive contributions of the dendritic tree to synaptic integration and neuronal output. Proc. Natl. Acad. Sci. U S A. 104, 11447–11452. doi: 10.1073/pnas.0701586104
Bose, M., Muñoz-Llancao, P., Roychowdhury, S., Nichols, J. A., Jakkamsetti, V., Porter, B., et al. (2010). Effect of the environment on the dendritic morphology of the rat auditory cortex. Synapse 64, 97–110. doi: 10.1002/syn.20710
Brooks, S. P., Pask, T., Jones, L., and Dunnett, S. B. (2005). Behavioural profiles of inbred mouse strains used as transgenic backgrounds. II: cognitive tests. Genes Brain Behav. 4, 307–317. doi: 10.1111/j.1601-183X.2004.00109.x
Catapano, L. A., and Manji, H. K. (2007). G protein-coupled receptors in major psychiatric disorders. Biochim. Biophys. Acta 1768, 976–993. doi: 10.1016/j.bbamem.2006.09.025
Çetereisi, D., Kramvis, I., Gebuis, T., van der Loo, R. J., Gouwenberg, Y., Manvelder, H. D., et al. (2018). Gpr158 Deficiency Impacts Hippocampal Ca1 Neuronal Excitability, Dendritic Architecture, and Affects Spatial Learning Biorxiv [preprint] doi: 10.1101/506295
Chan, W. K. B., Zhang, H., Yang, J., Brender, J. R., Hur, J., Özgür, A., et al. (2015). GLASS: a comprehensive database for experimentally validated GPCR-ligand associations. Bioinformatics 31, 3035–3042. doi: 10.1093/bioinformatics/btv302
Condomitti, G., Wierda, K. D., Schroeder, A., Rubio, S. E., Vennekens, K. M., Orlandi, C., et al. (2018). An input-specific orphan receptor gpr158-hspg interaction organizes hippocampal mossy fiber-ca3 synapses. Neuron 100, 201.e–215.e. doi: 10.1016/j.neuron.2018.08.038
Dinocourt, C., Aungst, S., Yang, K., and Thompson, S. M. (2011). Homeostatic increase in excitability in area CA1 after schaffer collateral transection in vivo. Epilepsia 52, 1656–1665. doi: 10.1111/j.1528-1167.2011.03113.x
Elston, G. N., Benavides-Piccione, R., and DeFelipe, J. (2001). The pyramidal cell in cognition: a comparative study in human and monkey. J. Neurosci. 21:RC163.
Florian, C., and Roullet, P. (2004). Hippocampal CA3-region is crucial for acquisition and memory consolidation in Morris water maze task in mice. Behav. Brain Res. 154, 365–374. doi: 10.1016/j.bbr.2004.03.003
Fortune, E. S., and Rose, G. J. (2001). Short-term synaptic plasticity as a temporal filter. Trends Neurosci. 24, 381–385. doi: 10.1016/s0166-2236(00)01835-x
Goriounova, N. A., Heyer, D. B., Wilbers, R., Verhoog, M. B., Giugliano, M., Verbist, C., et al. (2018). Large and fast human pyramidal neurons associate with intelligence. eLife 7:e41714. doi: 10.7554/eLife.41714
Grant, S. G., O’Dell, T. J., Karl, K. A., Stein, P. L., Soriano, P., and Kandel, E. R. (1992). Impaired long-term potentiation, spatial learning, and hippocampal development in fyn mutant mice. Science 258, 1903–1910. doi: 10.1126/science.1361685
Hernández-Pérez, J. J., Gutiérrez-Guzmán, B. E., and Olvera-Cortés, M. E. (2016). Hippocampal strata theta oscillations change their frequency and coupling during spatial learning. Neuroscience 337, 224–241. doi: 10.1016/j.neuroscience.2016.09.003
Huang, Y., and Thathiah, A. (2015). Regulation of neuronal communication by G protein-coupled receptors. FEBS Lett. 589, 1607–1619. doi: 10.1016/j.febslet.2015.05.007
Khrimian, L., Obri, A., Ramos-Brossier, M., Rousseaud, A., Moriceau, S., Nicot, A.-S., et al. (2017). Gpr158 mediates osteocalcin’s regulation of cognition. J. Exp. Med. 214:jem.20171320. doi: 10.1084/jem.20171320
Kowalski, J., Gan, J., Jonas, P., and Pernía-Andrade, A. J. (2016). Intrinsic membrane properties determine hippocampal differential firing pattern in vivo in anesthetized rats. Hippocampus 26, 668–682. doi: 10.1002/hipo.22550
Kulkarni, V. A., and Firestein, B. L. (2012). The dendritic tree and brain disorders. Mol. Cell. Neurosci. 50, 10–20. doi: 10.1016/j.mcn.2012.03.005
Leung, C. C. Y., and Wong, Y. H. (2017). Role of G protein-coupled receptors in the regulation of structural plasticity and cognitive function. Molecules 22:1239. doi: 10.3390/molecules22071239
Magee, J. C. (2000). Dendritic integration of excitatory synaptic input. Nat. Rev. Neurosci. 1, 181–190. doi: 10.1038/35044552
Mayford, M., Siegelbaum, S. A., and Kandel, E. R. (2012). Synapses and memory storage. Cold Spring Harb. Perspect. Biol. 4, a005751–a005751. doi: 10.1101/cshperspect.a005751
Micale, V., Stepan, J., Jurik, A., Pamplona, F. A., Marsch, R., Drago, F., et al. (2017). Extinction of avoidance behavior by safety learning depends on endocannabinoid signaling in the hippocampus. J. Psychiatr. Res. 90, 46–59. doi: 10.1016/j.jpsychires.2017.02.002
Mitsushima, D., Sano, A., and Takahashi, T. (2013). A cholinergic trigger drives learning-induced plasticity at hippocampal synapses. Nat. Commun. 4:2760. doi: 10.1038/ncomms3760
Mohan, H., Verhoog, M. B., Doreswamy, K. K., Eyal, G., Aardse, R., Lodder, B. N., et al. (2015). Dendritic and axonal architecture of individual pyramidal neurons across layers of adult human neocortex. Cereb. Cortex 25, 4839–4853. doi: 10.1093/cercor/bhv188
Myatt, D. R., Hadlington, T., Ascoli, G. A., and Nasuto, S. J. (2012). Neuromantic-from semi-manual to semi-automatic reconstruction of neuron morphology. Front. Neuroinform. 6:4. doi: 10.3389/fninf.2012.00004
Navakkode, S., Sajikumar, S., Korte, M., and Soong, T. W. (2012). Dopamine induces LTP differentially in apical and basal dendrites through BDNF and voltage-dependent calcium channels. Learn. Mem. 19, 294–299. doi: 10.1101/lm.026203.112
Okada, T., Yamada, N., Tsuzuki, K., Horikawa, H. P. M., Tanaka, K., and Ozawa, S. (2003). Long-term potentiation in the hippocampal CA1 area and dentate gyrus plays different roles in spatial learning. Eur. J. Neurosci. 17, 341–349. doi: 10.1046/j.1460-9568.2003.02458.x
Orlandi, C., Omori, Y., Wang, Y., Cao, Y., Ueno, A., Roux, M. J., et al. (2018). Transsynaptic binding of orphan receptor gpr179 to dystroglycan-pikachurin complex is essential for the synaptic organization of photoreceptors. Cell Rep. 25, 130–145.e5. doi: 10.1016/j.celrep.2018.08.068
Orlandi, C., Posokhova, E., Masuho, I., Ray, T. A., Hasan, N., Gregg, R. G., et al. (2012). GPR158/179 regulate G protein signaling by controlling localization and activity of the RGS7 complexes. J. Cell Biol. 197, 711–719. doi: 10.1083/jcb.201202123
Orlandi, C., Xie, K., Masuho, I., Fajardo-Serrano, A., Lujan, R., and Martemyanov, K. A. (2015). Orphan receptor GPR158 is an allosteric modulator of Regulator of G Protein Signaling 7 (RGS7) catalytic activity with essential role in dictating its expression and localization in the brain. J. Biol. Chem. 290, 13622–13639. doi: 10.1074/jbc.M115.645374
Ostrovskaya, O. I., Orlandi, C., Fajardo-Serrano, A., Young, S. M., Lujan, R., and Martemyanov, K. A. (2018). Inhibitory signaling to ion channels in hippocampal neurons is differentially regulated by alternative macromolecular complexes of RGS7. J. Neurosci. 38, 1318–1378. doi: 10.1523/JNEUROSCI.1378-18.2018
Patel, N., Itakura, T., Gonzalez, J. M., Schwartz, S. G., and Fini, M. E. (2013). GPR158, an orphan member of G protein-coupled receptor family C: glucocorticoid-stimulated expression and novel nuclear role. PLoS One 8:e57843. doi: 10.1371/journal.pone.0057843
Rao-Ruiz, P., Carney, K. E., Pandya, N., van der Loo, R. J., Verheijen, M. H. G., van Nierop, P., et al. (2015). Time-dependent changes in the mouse hippocampal synaptic membrane proteome after contextual fear conditioning. Hippocampus 25, 1250–1261. doi: 10.1002/hipo.22432
Rodgers, R. J., Boullier, E., Chatzimichalaki, P., Cooper, G. D., and Shorten, A. (2002). Contrasting phenotypes of C57BL/6JOlaHsd, 129S2/SvHsd and 129/SvEv mice in two exploration-based tests of anxiety-related behaviour. Physiol. Behav. 77, 301–310. doi: 10.1016/s0031-9384(02)00856-9
Schmitz, L. J. M., Klaassen, R. V., Ruiperez-Alonso, M., Zamri, A. E., Stroeder, J., Rao-Ruiz, P., et al. (2017). The AMPA receptor-associated protein Shisa7 regulates hippocampal synaptic function and contextual memory. eLife 6:e24192. doi: 10.7554/eLife.24192
Schroeder, A., Vanderlinden, J., Vints, K., Ribeiro, L. F., Vennekens, K. M., Gounko, N. V., et al. (2018). A Modular Organization of LRR Protein-Mediated synaptic adhesion defines synapse identity. Neuron 99, 329–344.e7. doi: 10.1016/j.neuron.2018.06.026
Scorcioni, R., Polavaram, S., and Ascoli, G. A. (2008). L-Measure: a web-accessible tool for the analysis, comparison and search of digital reconstructions of neuronal morphologies. Nat. Protoc. 3, 866–876. doi: 10.1038/nprot.2008.51
Spijker, S., Houtzager, S. W. J., De Gunst, M. C. M., De Boer, W. P. H., Schoffelmeer, A. N. M., and Smit, A. B. (2004). Morphine exposure and abstinence define specific stages of gene expression in the rat nucleus accumbens. FASEB J. 18, 848–850. doi: 10.1096/fj.03-0612fje
Spruston, N. (2008). Pyramidal neurons: dendritic structure and synaptic integration. Nat. Rev. Neurosci. 9, 206–221. doi: 10.1038/nrn2286
Sutton, L. P., Orlandi, C., Song, C., Oh, W. C., Muntean, B. S., Xie, K., et al. (2018). Orphan receptor GPR158 controls stress-induced depression. eLife 7:e33273. doi: 10.7554/eLife.33273
Tarantino, L. M., Gould, T. J., Druhan, J. P., and Bucan, M. (2000). Behavior and mutagenesis screens: the importance of baseline analysis of inbred strains. Mamm. Genome 11, 555–564. doi: 10.1007/s003350010107
Thakar, S., Wang, L., Yu, T., Ye, M., Onishi, K., Scott, J., et al. (2017). Evidence for opposing roles of Celsr3 and Vangl2 in glutamatergic synapse formation. Proc. Natl. Acad. Sci. U.S.A. 114, E610–E618. doi: 10.1073/pnas.1612062114
Thompson, M. D., Percy, M. E., McIntyre Burnham, W., and Cole, D. E. C. (2008). G protein-coupled receptors disrupted in human genetic disease. Methods Mol. Biol. 448, 109–137. doi: 10.1007/978-1-59745-205-2_7
Tronel, S., Fabre, A., Charrier, V., Oliet, S. H. R., Gage, F. H., and Abrous, D. N. (2010). Spatial learning sculpts the dendritic arbor of adult-born hippocampal neurons. Proc. Natl. Acad. Sci. U S A. 107, 7963–7968. doi: 10.1073/pnas.0914613107
Urrego, D., Troncoso, J., and Múnera, A. (2015). Layer 5 pyramidal neurons’ dendritic remodeling and increased microglial density in primary motor cortex in a murine model of facial paralysis. Biomed. Res. Int. 2015:482023. doi: 10.1155/2015/482023
van Elburg, R. A. J., and van Ooyen, A. (2010). Impact of dendritic size and dendritic topology on burst firing in pyramidal cells. PLoS Comput. Biol. 6:e1000781. doi: 10.1371/journal.pcbi.1000781
Vetter, P., Roth, A., and Häusser, M. (2001). Propagation of action potentials in dendrites depends on dendritic morphology. J. Neurophysiol. 85, 926–937. doi: 10.1152/jn.2001.85.2.926
Võikar, V., Kõks, S., Vasar, E., and Rauvala, H. (2001). Strain and gender differences in the behavior of mouse lines commonly used in transgenic studies. Physiol. Behav. 72, 271–281. doi: 10.1016/s0031-9384(00)00405-4
Vorhees, C. V., and Williams, M. T. (2006). Morris water maze: procedures for assessing spatial and related forms of learning and memory. Nat. Protoc. 1, 848–858. doi: 10.1038/nprot.2006.116
Vuksic, M., Del Turco, D., Vlachos, A., Schuldt, G., Müller, C. M., Schneider, G., et al. (2011). Unilateral entorhinal denervation leads to long-lasting dendritic alterations of mouse hippocampal granule cells. Exp. Neurol. 230, 176–185. doi: 10.1016/j.expneurol.2011.04.011
Keywords: cognition, memory, hippocampus, behavior, morphology, electrophysiology, knock out, knock down
Citation: Çetereisi D, Kramvis I, Gebuis T, van der Loo RJ, Gouwenberg Y, Mansvelder HD, Li KW, Smit AB and Spijker S (2019) Gpr158 Deficiency Impacts Hippocampal CA1 Neuronal Excitability, Dendritic Architecture, and Affects Spatial Learning. Front. Cell. Neurosci. 13:465. doi: 10.3389/fncel.2019.00465
Received: 28 June 2019; Accepted: 30 September 2019;
Published: 25 October 2019.
Edited by:
Josef Bischofberger, University of Basel, SwitzerlandReviewed by:
Marco Fuenzalida, Valparaíso University, ChileGrzegorz Wiera, Wrocław Medical University, Poland
Copyright © 2019 Çetereisi, Kramvis, Gebuis, van der Loo, Gouwenberg, Mansvelder, Li, Smit and Spijker. This is an open-access article distributed under the terms of the Creative Commons Attribution License (CC BY). The use, distribution or reproduction in other forums is permitted, provided the original author(s) and the copyright owner(s) are credited and that the original publication in this journal is cited, in accordance with accepted academic practice. No use, distribution or reproduction is permitted which does not comply with these terms.
*Correspondence: Sabine Spijker, cy5zcGlqa2VyQHZ1Lm5s
†These authors have contributed equally to this work