- 1Department of Molecular Physiology and Biophysics, The University of Iowa Carver College of Medicine, Iowa City, IA, United States
- 2Department of Neurology and Neuroscience, University of Wisconsin-Madison, Madison, WI, United States
- 3Department of Ophthalmology, Biochemistry and Neuroscience, West Virginia University, Morgantown, WV, United States
- 4Department of Ophthalmology and Visual Sciences, Ophthalmology and Visual Sciences, The University of Iowa Carver College of Medicine, Iowa City, IA, United States
Transducin mediates signal transduction in a classical G protein-coupled receptor (GPCR) phototransduction cascade. Interactions of transducin with the receptor and the effector molecules had been extensively investigated and are currently defined at the atomic level. However, partners and functions of rod transducin α (Gαt1) and βγ (Gβ1γ1) outside the visual pathway are not well-understood. In particular, light-induced redistribution of rod transducin from the outer segment to the inner segment and synaptic terminal (IS/ST) allows Gαt1 and/or Gβ1γ1 to modulate synaptic transmission from rods to rod bipolar cells (RBCs). Protein-protein interactions underlying this modulation are largely unknown. We discuss known interactors of transducin in the rod IS/ST compartment and potential pathways leading to the synaptic effects of light-dispersed Gαt1 and Gβ1γ1. Furthermore, we show that a prominent non-GPCR guanine nucleotide exchange factor (GEF) and a chaperone of Gα subunits, resistance to inhibitors of cholinesterase 8A (Ric-8A) protein, is expressed throughout the retina including photoreceptor cells. Recent structures of Ric-8A alone and in complexes with Gα subunits have illuminated the structural underpinnings of the Ric-8A activities. We generated a mouse model with conditional knockout of Ric-8A in rods in order to begin defining the functional roles of the protein in rod photoreceptors and the retina. Our analysis suggests that Ric-8A is not an obligate chaperone of Gαt1. Further research is needed to investigate probable roles of Ric-8A as a GEF, trafficking chaperone, or a mediator of the synaptic effects of Gαt1.
Introduction
A prototypical heterotrimeric G protein, transducin (Gαtβγ), is a key signaling mediator in the visual transduction cascade in vertebrate rod and cone photoreceptors. Traditionally, studies of transducin focused on its structure and mechanisms underlying this signaling cascade. Phototransduction takes place in a specialized ciliary compartment of photoreceptor cells called the outer segment (OS). Absorption of light by rhodopsin allows the photoexcited receptor (R∗) to stimulate GDP/GTP exchange on Gαtβγ, thus releasing GαtGTP from Gβγ and R∗. Subsequent activation of cGMP phosphodiesterase 6 (PDE6) by GαtGTP causes concentration of cytoplasmic cGMP to drop, halting conductance of cGMP-gated channels in the plasma membrane (Arshavsky et al., 2002; Fu and Yau, 2007). Besides rhodopsin and PDE6, an essential partner of transducin in the visual cascade is a photoreceptor-specific member of the RGS (regulators of G protein signaling) family, RGS9-1. RGS9-1 in complex with Gβ5L acts as a GTPase-activating protein for Gαt, and thus is a major regulator of the turn-off of the visual signal (He et al., 1998, 2000; Makino et al., 1999; Cowan et al., 2001; Martemyanov and Arshavsky, 2009; Arshavsky and Burns, 2012). Together, the interactions of transducin with R∗, PDE6, and RGS9-1/Gβ5L control the signal amplification, sensitivity, and speed of photoresponses. The remarkable molecular level of insight into these interactions has been recently elevated with solutions of the cryo-EM structures of transducin complexed with rhodopsin and PDE6 (Gao et al., 2019, 2020). Nevertheless, several important aspects of transducin biology, including its folding, trafficking, and roles outside the phototransduction cascade remain largely obscure. Rod transducin subunits Gαt1 and Gβ1γ1 are known to undergo bi-directional translocation between the OS and inner compartments of rod photoreceptors in a light-dependent manner, but its partners in the inner photoreceptor compartments, the inner segment and synaptic terminal (IS/ST), and the significance of these interactions are not well understood (Sokolov et al., 2002; Calvert et al., 2006; Artemyev, 2008; Slepak and Hurley, 2008). Light-induced translocation of Gαt1 and Gβ1γ1 was shown to reduce phototransduction gain, and thus it contributes to light adaptation of photoreceptor cells (Sokolov et al., 2002). Analyses of mouse models with impaired transducin translocation also support an important role of the phenomenon in neuroprotection of rods, presumably by reducing the metabolic stress associated with the constitutive phototransduction reactions under light conditions saturating responsiveness of rods (Fain, 2006; Peng et al., 2011; Majumder et al., 2013; Tian et al., 2014). Arguably, the most intriguing and poorly understood consequence of Gαt1 and Gβ1γ1 translocation is its modulation of the synaptic transmission from rods to rod bipolar cells (RBCs). Block of transducin translocation via additional lipid anchoring of the Gαt1 A3C mutant in a mouse model desensitized signal transmission to RBC, suggesting that transducin translocation enhances signaling to RBC in wild type mice (Majumder et al., 2013). Ultimately, the mechanism underlying the synaptic effect of transducin may involve its interactions with the synaptic machinery and/or modulation of the voltage-gated Ca2+ channels, Cav1.4. Analysis of known and identification of novel partners of transducin in the IS/ST may provide clues to this mechanism.
Transducin Partners in the Inner Photoreceptor Compartments: UNC119 and LGN/GPSM2
One well-known partner of Gαt1 outside the phototransduction cascade is Uncoordinated 119 (UNC119). UNC119 is a mammalian ortholog of C. elegans unc-119 (Maduro and Pilgrim, 1995), also known as Retina Gene 4 protein (RG4; Higashide et al., 1996). UNC119 is uniquely abundant in photoreceptor cells, specifically the rod IS/ST, and its levels in other tissues are significantly lower (Higashide et al., 1998; Swanson et al., 1998; Karim et al., 2010). Truncation mutation in UNC119 is linked to cone-rod dystrophy in human patients (Kobayashi et al., 2000), whereas knockout of UNC119 in mice causes slow retinal degeneration (Ishiba et al., 2007). UNC119 shares sequence and structural homology with the prenyl-binding protein PDE6δ (or PDE6D), both featuring immunoglobulin-like β-sandwich fold serving as a lipid-binding module (Zhang et al., 2011). Unlike PDE6δ, which is a prenyl-binding protein, UNC119 selectively binds myristoyl moiety (Zhang et al., 2004, 2011; Gopalakrishna et al., 2011). Owing its lipid-binding specificity, UNC119 is generally viewed as a carrier protein for myristoylated cargo, with a preference for cargo proteins targeted to primary cilia via an ARL3-dependent mechanism (Ismail et al., 2012; Fansa and Wittinghofer, 2016; Frederick et al., 2020). Consistent with the role of UNC119 as a trafficking chaperone of transducin, the anterograde transport of translocated Gαt1 to the OS during dark adaptation is impaired in UNC119 knockout mice (Zhang et al., 2011). Quantification of UNC119 in rods suggests that the light-dispersed Gαt1 is a major partner of UNC119 (Sinha et al., 2013).
However, the function of UNC119 in the retina appears to extend beyond that of a trafficking chaperone across the connecting cilium. Besides Gαt1, UNC119 interacts in a lipid modification-independent manner with CaBP4 (Haeseleer, 2008). Notably, the levels of UNC119 are reduced in mice lacking Gαt1 or CaBP4, suggesting that UNC119 functionally interacts with both of these proteins (Haeseleer, 2008; Sinha et al., 2013). CaBP4 is an EF-hand Ca2+ binding protein that binds to and modulates the voltage-dependence of Cav1.4 channels thereby enhancing RBC responses (Haeseleer et al., 2004). Rod and cone synaptic function is markedly diminished in CaBP4 knockout mice (Haeseleer et al., 2004), and loss-of function mutations in CaBP4 cause congenital stationary night blindness and other visual disorders in humans (Littink et al., 2010; Bijveld et al., 2013). Potentially, light-dispersed Gαt1 may relieve UNC119-dependent constraint on the CaBP4 regulation of Cav1.4 channels. Another lipid-independent interactor of UNC119 is RIBEYE, a major component of synaptic ribbons (Schmitz et al., 2000; Alpadi et al., 2008). This interaction recruits UNC119 to synaptic ribbons, and it may be essential for synaptic transmission at the rod ribbon synapse (Alpadi et al., 2008). It is not known if and how transducin modulates the binding of UNC119 to RIBEYE, but this may represent a potential pathway contributing to the synaptic effects of transducin translocation.
An interesting and possibly critical property of UNC119 is its ability to interact with heterotrimeric Gαt1β1γ1, promote dissociation of Gαt1 from Gβ1γ1, and release them from the membrane (Gopalakrishna et al., 2011). Analysis of the complex between UNC119 and the full-length Gαt1 by Small Angle X-ray Scattering (SAXS) and chemical crosslinking suggested an additional interface between the proteins involving the switch II region Gαt1, which overlaps with the Gβ1γ1 binding site (Cheguru et al., 2015). Thus, UNC119 apparently dissociates transducin subunits by disrupting and sterically occluding the Gβ1γ1-binding sites on Gαt1 (Cheguru et al., 2015). As a result, two species are produced, Gαt1GDP (or Gαt1GDP-UNC119 complex) and Gβ1γ1. Each of these species may now interact with new partners. In particular, Gαt1GDP is primed for interaction with Leu-Gly-Asn repeat-enriched (LGN) protein LGN/GPSM2 (Mochizuki et al., 1996). LGN/GPSM2 belongs to the class of G-protein modulators containing G-protein regulatory (GPR) or GoLoco motifs. GoLoco/GPR-proteins interact with and stabilize Gα subunits in a GDP-bound form, hence serving as guanine nucleotide dissociation inhibitors (GDIs; Natochin et al., 2000; Bernard et al., 2001; Blumer et al., 2007). LGN/GPSM2 is best known for its role in Gα-regulated positioning of a mitotic spindle during cell division (Lancaster and Knoblich, 2012; di Pietro et al., 2016). However, LGN/GPSM2 is also expressed in terminally differentiated photoreceptor cells, where it is localized to the IS/ST (Kerov et al., 2005; Nair et al., 2005). LGN/GPSM2 was shown to interact with endogenous Gαt1, and its role as a potential modulator of transducin trafficking has been proposed (Kerov et al., 2005). Evidence supporting this notion is starting to emerge (Bocchero et al., 2020). Interestingly, disruption of planar polarity mechanisms involving Gαi3 and LGN/GPSM2 collapsed the gradients of ribbon size and maximal synaptic Ca2+ influx in the inner hair cells (Jean et al., 2019), suggesting that Gαt1-LGN/GPSM2 signaling may also be involved in regulation of ribbon synapses in photoreceptors.
The synaptic regulation by translocated Gαt1 may be due to sequestration of Gβγ. In a well-characterized mechanism, the release of neurotransmitters at synapses of the central nervous system triggers negative feedback by activating presynaptic G protein-coupled receptors (GPCRs) and thereby generating free Gβγ. Gβγ in turn inhibits Ca2+ influx by binding directly to the α-subunits of presynaptic Cav2.1 and Cav2.1 channels (Catterall, 2011; Khan et al., 2013; Zamponi and Currie, 2013). In contrast, Cav1.4 is not known to be regulated directly by Gβγ. A distinct mechanism of synaptic inhibition involves interactions between Gβγ subunits and the SNARE complex, specifically SNAP25 (Blackmer et al., 2001, 2005; Yoon et al., 2007). In cone photoreceptors, activation of presynaptic metabotropic glutamate receptor reduced synaptic transmission to horizontal cells via Gβγ/SNARE interactions (Van Hook et al., 2017). Furthermore, phosducin, an abundant Gβγ-binding protein in photoreceptors, was also implicated in regulation of synaptic transmission to RBCs, as the protein knockout resulted in a reduced sensitivity of ERG responses from RBCs in dark-adapted mice (Herrmann et al., 2010). These findings though were not confirmed in recordings from retinal slices, indicating that such experimental conditions may have abolished the phenotype (Long et al., 2013). It is possible that Gαt1 and/or phosducin sequester Gβγ liberated by activation of presynaptic GPCRs and thus enhance sensitivity of the rod-RBC synaptic transmission.
Ric-8A in Photoreceptors: A GEF or a Chaperone?
In considering novel potential partners of Gαt1 in photoreceptor cells, one candidate, Ric-8A, was conspicuous. Resistance to inhibitors of cholinesterase 8 (Ric-8) proteins were originally discovered as positive regulators of G-protein signaling pathways (Miller et al., 1996, 2000). Subsequent studies demonstrated that Ric-8 proteins interact directly with the monomeric GDP-bound Gα subunits and act as non-GPCR guanine nucleotide exchange factors (GEFs; Tall et al., 2003). Two isoforms, Ric-8A and Ric-8B are encoded in vertebrate genomes. Each isoform regulates a particular subset of Gα subunits: Ric-8A interacts with Gαi/t, Gαq, and Gα12/13, whereas Ric-8B is selective for Gαs (Klattenhoff et al., 2003; Tall et al., 2003; Von Dannecker et al., 2005; Nagai et al., 2010). The interaction of Ric-8 with GDP-bound Gα stimulates release of GDP, leading to the formation of a stable intermediate complex of Ric-8 and nucleotide-free Gα. Once Gα binds GTP, it dissociates from Ric-8, and thus the nucleotide-exchange cycle on Gα is completed (Tall et al., 2003). The GEF activity of Ric-8A opposing the GDI-activity of LGN/GPSM2 might be important to the role of Ric-8A in Gα-regulated positioning of mitotic spindle (Afshar et al., 2004; David et al., 2005; Tall and Gilman, 2005). Still, the interplay between Ric-8A and LGN/GPSM2 proteins in this process is poorly understood. More recently, Ric-8A attracted attention as a chaperone of Gα subunits (Papasergi et al., 2015). In cell-free translation systems, Ric-8A was required for the expression of properly folded Gα subunits, and co-expression of Ric-8 with Gα subunits in HEK293 cells and insect cells led to significant elevations in the expression levels of Gα subunits (Chan et al., 2011, 2013; Gabay et al., 2011). Although compelling evidence has been accumulated for both the GEF and chaperone function of Ric-8A in vitro and in cell cultures, little is known about specific pathways and systems regulated by Ric-8A in vivo, and it is often unclear which of the two Ric-8A functions dominates its biological effects. This is in part due to embryonic lethality of the Ric-8A knockout mice (Tonissoo et al., 2010; Gabay et al., 2011). Conditional knockouts of Ric-8A in differentiated neuronal populations and glial cells reveal apparent and severe phenotypes, yet the exact mechanisms of the Ric-8A deficiency underlying these phenotypes could not be discerned (Ma et al., 2012; Ruisu et al., 2013). Targeted disruption of Ric-8A expression in mouse B-cells led to a loss of Gαi and Gαq and caused severe humoral immunodeficiency, a phenotype consistent with the chaperone function of the protein (Boularan et al., 2015).
Growing evidence for the important biological roles of Ric-8 spurred the interest in understanding the molecular and structural basis underlying its activities. This work culminated in solution of the atomic structures initially of Ric-8A in complex with a Gαt1 mimetic, and subsequently in complex with the full-length Gα subunits (Srivastava and Artemyev, 2019; Srivastava et al., 2019; Zeng et al., 2019; McClelland et al., 2020; Seven et al., 2020). The structure of the active nearly full length Ric-8A1-492 revealed two main domains of Ric-8A: an armadillo-like core (residues about 1–426) and an unstructured C-terminal tail (residues 427–492; Srivastava et al., 2019). The armadillo-like core of Ric-8A is a mixture of canonical ARM repeats and ARM-related HEAT repeats folded into a ribbon-like superhelix featuring a concave surface (Srivastava et al., 2019; Zeng et al., 2019). The concave surface of Ric-8A forms an extensive interface with the Gαt1 C-terminus (Figure 1A). Critical contacts between Ric-8A and the Gα C-terminus are made by the very C-terminal residue F350 of Gαt1 (Figure 1A; Srivastava et al., 2019). Mutations interfering with the interaction network of the C-terminal residue of Gα ablate the binding of Ric-8A to folded GαGDP (Srivastava et al., 2019), as well as disrupt the chaperone activity of Ric-8A (Seven et al., 2020). As a GEF, Ric-8A interacts with the folded GαGDP and induces partial unfolding of the latter accompanied by the large dislocation of the Gα C-terminal α5-helix from the β-sheet core of the Ras-like domain, disorganization of the nucleotide-binding site and release of GDP (Srivastava and Artemyev, 2019; McClelland et al., 2020; Seven et al., 2020; Figure 1B). As a chaperone of nascent Gα, Ric-8A would interact with a partially folded intermediate of Gα in which the α5-helix has not yet assumed its position with the β-sheet cradle (Seven et al., 2020). The Gαt1 C-terminal region is unstructured as a part of MBP-fusion protein, but it forms the α5-helix upon binding to Ric-8A (Srivastava et al., 2019). Therefore, the chaperone activity of Ric-8A may involve folding of the Gα C-terminal region into an α5-helix and stabilization of the β-sheet core of the Ras-like domain thereby preparing Gα for the first time GTP-binding event (Srivastava et al., 2019; McClelland et al., 2020; Seven et al., 2020). Upon binding of GTP, Gα is released from Ric-8A and the α5-helix replaces Ric-8A in stabilizing the β-sheet core of Gα. The GTP-binding site in the Ric-8A/Gα complex is disorganized to a greater extent compared to that in the GPCR/Gαβγ complexes (McClelland et al., 2020; Seven et al., 2020; Srivastava and Artemyev, 2020). Remarkably, the distal portion of the C-terminal tail of Ric-8A forms a unique smaller secondary interface with the switch II/α3-helix region of Gα, which appears to assist GTP-binding (Srivastava and Artemyev, 2019; McClelland et al., 2020; Seven et al., 2020; Figure 1C). Altogether, the structures of the Ric-8A/Gα complex are consistent with both proposed functions of Ric-8A, as a GEF and a chaperone. Specific function of Ric-8A would have to be determined in the context of a cellular activity under investigation.
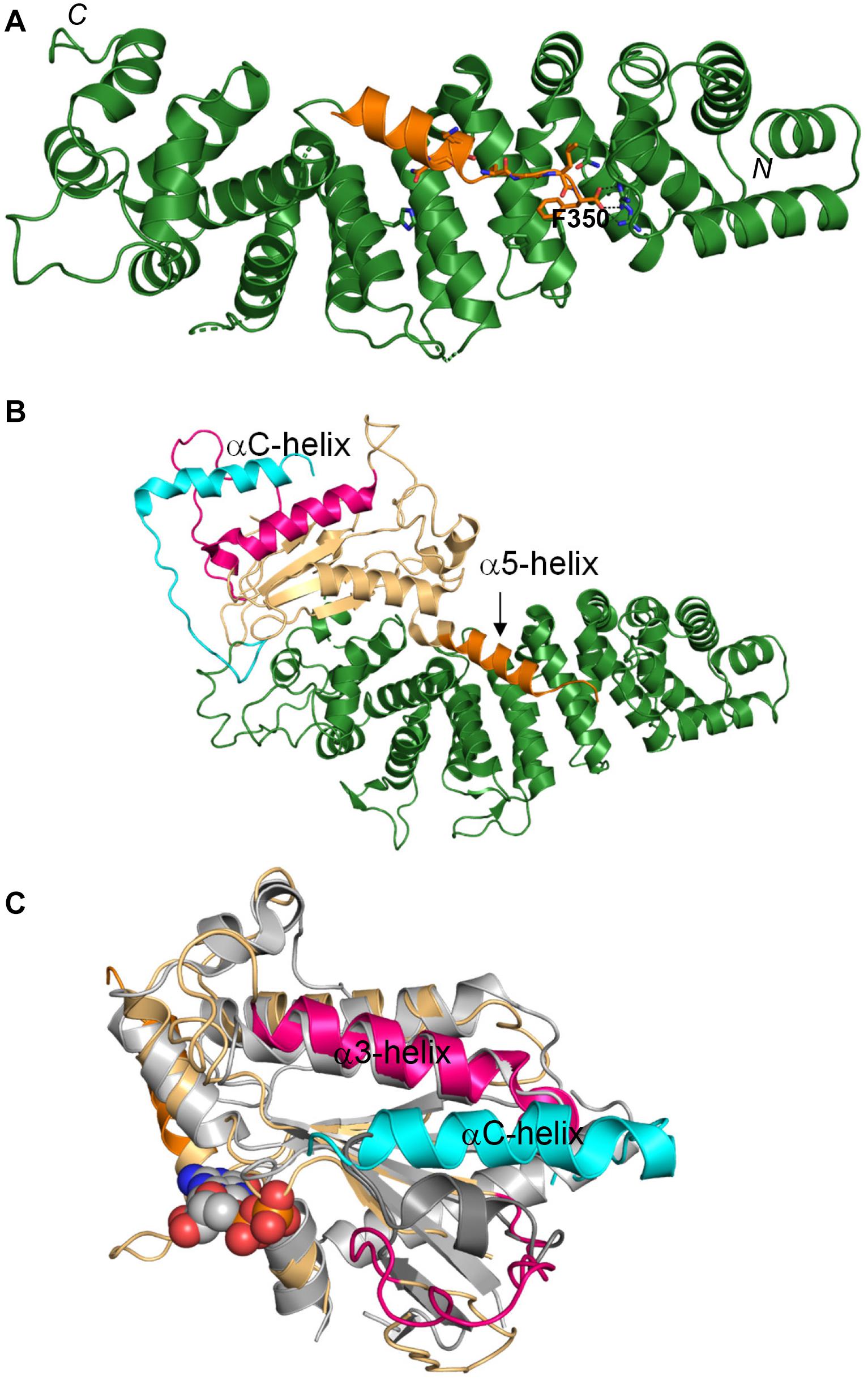
Figure 1. Interaction of Ric-8A with Gα. (A) The α5-helix of Gαt1 (orange) bound to the concave surface of the armadillo core domain of Ric-8A (green; based on PDB 6N85). The C-terminal F350 of Gαt1 forms multiple contacts with Ric-8A. (B) Crystal structure of the complex of Ric-8A with Gαi (PDB 6TYL). Only the Ras-like domain of Gαi is resolved in the structure (wheat). The a5-helix of Gαi (orange) is dislodged from the β-sheet core. The C-terminal helix of Ric-8A (αC, cyan) interacts with the switch II/α3 helix of Gαi (pink). (C) Top view of the Gαi complex with Ric-8A. Only the C-terminus of Ric-8A is shown for clarity. The αC-helix of Ric-8A (cyan) interacts with the switch II/a3-helix region (pink) of Gαi (wheat). This interaction may facilitate the binding of GTP to Gα bound to Ric-8A. The GPCR-bound structure of the engineered minimized Gα (gray, GDP – spheres, and PDB 5G53) is superimposed with Gαi to indicate position of the nucleotide binding site.
Given the high rate of transducin synthesis and transport in photoreceptors (Frederick et al., 2020), and the likely existence of the quality control chaperone mechanism, we first investigated expression of Ric-8A in retina and photoreceptor cells. In homogenates of C57Bl/6 mouse retina specific monoclonal Ric-8A antibody 3E1 recognized a single band of the predicted MW of 60 kDa (Figure 2A; Gabay et al., 2011). Immunofluorescence staining of cryosections of the C57Bl/6 retina with 3E1 antibody revealed that Ric-8A is distributed throughout the retina, including in the IS, OPL, INL, and IPL (Figure 2B). Expression of Ric-8A in the photoreceptor IS/ST was also confirmed by Western blot analysis of tangential retina sections (Figure 2C; Sokolov et al., 2002). The retinal distribution of Ric-8A suggested that it can serve as a chaperone for newly synthesized Gαt1 and/or a GEF for light-translocated Gαt1 in rods, as well as chaperone/GEF for Gαo in RBCs. Gαo is abundant in RBCs, where it mediates signaling via the mGluR6 cascade, which couples a decrease in glutamate levels in the synaptic cleft with opening of the TRPM1 cation channels in the dendritic tips of RBCs (Dhingra et al., 2000, 2002). To test the former hypothesis, we generated a mouse model with conditional knockout of Ric-8A in rods. To achieve specific deletion of Ric-8A in rods, Ric-8AF/F mice in which exons 2–4 of the gene are floxed (Ma et al., 2012) were crossed to iCre-75+/– mice, in which expression of Cre is driven by a 4-kb mouse rod opsin promoter (Li et al., 2005). The iCre-75+/– driver strain is commonly used for RP-specific conditional KO, as it provides for robust and uniform expression of Cre in rods (Li et al., 2005; Lai et al., 2013; Sundermeier et al., 2014; He et al., 2016). Immunofluorescence staining of retina cryosections from Ric-8AF/FCre+ mice confirmed robust expression of Cre (Supplementary Figure 1) and deletion of Ric-8A in mutant rods (Figure 2B and Supplementary Figure 2). Western blot analysis of Ric-8A in dark-adapted Ric-8AF/FCre+ retinas indicated that the protein level is reduced by ∼36% (Figure 2D). The extent of reduction in the protein level of Ric-8A in the entire retina is consistent with the localization of a major fraction of the protein to the inner retina. Surprisingly, the ablation of Ric-8A expression in rods caused only a modest ∼22% reduction in the protein level of Gαt1 (Figure 2E). Furthermore, the majority of Gαt1 was properly targeted to the OS in the absence of Ric-8A (Figure 2F). Supporting the functional folding of transducin in rods lacking Ric-8A, the a- and b-wave ERG responses of mutant mice were comparable to those from control mice (Figures 2G,H). Thus, our results indicate that although Ric-8A may slightly increase the abundance of Gαt1 in rods, the proper folding of Gαt1 in the absence of Ric-8A proceeds efficiently enough to support the photoreceptor function. Although mouse rods lacking Ric-8A may express Ric-8B, the latter isoform does not interact with Gαt1 (Papasergi et al., 2015; Srivastava et al., 2019). One caveat to this conclusion needs to be noted. It cannot be excluded that Ric-8A is a catalytic chaperone that can efficiently assist folding of Gαt1 even when present in trace amounts. Trace amounts of Ric-8A in mutant rods may result if the Ric-8A protein expressed prior to the gene excision persists for a long time or if the gene excision is incomplete.
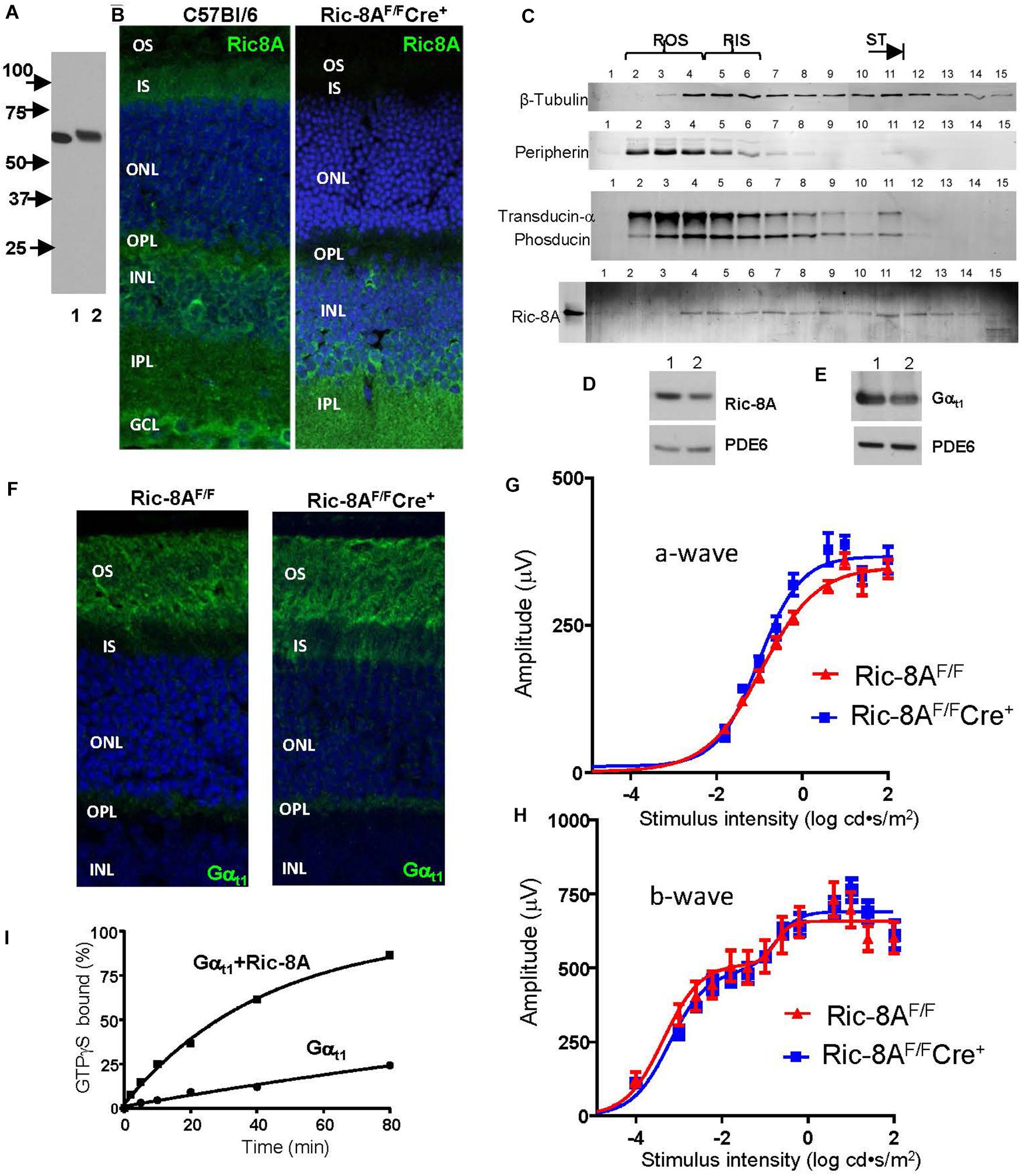
Figure 2. Expression of Ric-8A in the retina and conditional knockout of the protein in mouse rods. (A) Western blot with anti-Ric-8A monoclonal antibody 3E1; lane 1 – recombinant human Ric-8A (50 ng), lane 2 – C57Bl retina homogenate (50 μg). (B) Immunofluorescence (IF) staining of mouse retina cryosections with 3E1 monoclonal antibody. Ric-8A is present throughout the retina in 2-month old WT mice and absent in the inner segment (IS), outer nuclear layer (ONL), and outer plexiform layer (OPL) in 2-month old Ric-8AF/FCre+ mice. INL, inner nuclear layer; IPL, inner plexiform layer, GCL, ganglion cell layer; and blue, To-Pro3 nuclear stain. (C) Western blot analysis of tangential sections of WT retina indicates the presence of Ric-8A in the IS (approx. lanes 5–6), the inner compartments of rods (approx. lanes 7–11), and the bipolar cell layer (approx. lanes 12–15). Ric-8A is largely excluded from the outer segment (OS; approx. lanes 2–4). Lane 0 – recombinant Ric-8A. (D, E) Western blot analyses of equal fractions of total retina extract from control 2-month old Ric-8AF/F mice (lane 1) and littermate Ric-8AF/FCre+ mice (lane 2) with anti-Ric-8A antibody 3E1 (D) and anti-Gαt1 K-20 antibody (SCBT; E). PDE6 – loading control. The bands were quantified with ImageJ. From three similar experiments, the average reductions of Ric-8A and Gαt1 in the Ric-8AF/FCre+ retina were 36 ± 3% and 22 ± 4%, respectively. (F) IF staining of retina cryosections from dark-adapted mice with anti-Gαt1 K-20 antibody. Gαt1 is localized mainly to the rod OS in 2-month old Ric-8AF/FCre+ mice. (G, H) a-wave amplitudes and b-wave amplitudes measured from recordings of dark-adapted mice. Points represent the mean ± SEM (n = 6, left and right eyes from 3 mice of each genotype). Curves represent fits from single (G) or double (H) sigmoidal functions. For each flash strength, there were no statistically significant differences (adjusted P value > 0.05) between Ric-8AF/FCre+ and control mice. (I) Kinetics of GTPγS binding to chimeric Gαt1 (Skiba et al., 1996; Natochin et al., 1998) alone (1 μM) and in the presence of Ric-8A (3 μM). Representative experiment. For n = 3 experiments, the kapp values are: Gαt1 0.005 ± 0.001 min– 1 and Gαt1+Ric-8A 0.025 ± 0.002 min– 1; unpaired t-test.
Nevertheless, it is possible that the main function of Ric-8A in rods is linked to its GEF activity. We confirmed that Ric-8A is a GEF for the Gαt similarly as it is for other members of the Gαi family by measuring the kinetics of GTPγS-binding to a transducin-like chimeric Gαt1GDP in the presence or absence of Ric-8A. The apparent rate of the nucleotide exchange was increased by ∼5-fold in the presence of Ric-8A (Figure 2I). Future studies are needed to investigate functional significance of the Ric-8A GEF activity toward Gαt1.
Discussion
The structures of the Ric-8A complexes with Gα subunits reveal the molecular underpinnings of its chaperone and GEF activities (Srivastava and Artemyev, 2019; Srivastava et al., 2019; McClelland et al., 2020; Seven et al., 2020). Depending on the Ric-8A function in a given system, the structures can represent either the GEF complex intermediate with an empty-pocket for nucleotide binding or the folding intermediate of Gα during its biosynthesis. The Ric-8A/Gα complexes reveal two remarkable features: a large displacement of the α5-helix of Gα from the β-sheet cradle of the Ras-like domain, and a unique interaction of the C-terminal helix of Ric-8A with the switch II/α3-helix region of Gα (Srivastava and Artemyev, 2019, 2020; McClelland et al., 2020; Seven et al., 2020). The interaction of Ric-8A with the C-terminus/α5-helix of Gα is central to both the GEF and the chaperone activity. When Ric-8A acts as a GEF, this interaction initiates GDP-release, just like GPCRs cause GDP-release during activation of heterotrimeric G-proteins. When Ric-8A acts as a chaperone, this interaction induces the folding of the α5-helix outside the β-sheet cradle of partially folded Gα (Srivastava et al., 2019; McClelland et al., 2020). The interaction of the C-terminal helix of Ric-8A with the switch II/α3-helix region of Gα likely promotes GTP-binding to Gα thereby concluding either the GEF or the chaperone cycle of Ric-8A (Srivastava and Artemyev, 2019, 2020; McClelland et al., 2020; Seven et al., 2020). We demonstrated that Ric-8A is expressed throughout the retina. Thus, retina represents an excellent opportunity to dissect the roles of Ric-8A. The conditional knockout of Ric-8A in mouse rods argues against its role as an essential chaperone of Gαt1. Yet, as a GEF, Ric-8A may play roles in transducin trafficking and/or modulation of the rod-RBC synaptic transmission. The role of Ric-8A in RBCs and its potential influence on the abundance of Gαo and the mGluR6-mediated cascade remains to be investigated. Gαq/11 is the Gα subfamily that is most stringently dependent on Ric-8A as a chaperone (Gabay et al., 2011). Therefore, defining role of Ric-8A in intrinsically photosensitive retinal ganglion cells and the Gαq-mediated melanopsin signaling cascade is of particular interest (Do and Yau, 2010; Schmidt et al., 2011).
Data Availability Statement
All datasets presented in this study are included in the article/Supplementary Material.
Ethics Statement
The animal study was reviewed and approved by the University of Iowa Animal Care and Use Committee.
Author Contributions
NA wrote the manuscript. All authors contributed to the study, read and approved the manuscript.
Funding
This work was supported by the National Institutes of Health grant RO1 EY-12682 to NA.
Conflict of Interest
The authors declare that the research was conducted in the absence of any commercial or financial relationships that could be construed as a potential conflict of interest.
Acknowledgments
We would like to thank Dr. Gregory G. Tall for kindly providing anti Ric-8A antibody.
Supplementary Material
The Supplementary Material for this article can be found online at: https://www.frontiersin.org/articles/10.3389/fncel.2020.589494/full#supplementary-material
References
Afshar, K., Willard, F. S., Colombo, K., Johnston, C. A., McCudden, C. R., Siderovski, D. P., et al. (2004). RIC-8 is required for GPR-1/2-dependent Galpha function during asymmetric division of C. elegans embryos. Cell 119, 219–230. doi: 10.1016/j.cell.2004.09.026
Alpadi, K., Magupalli, V. G., Kappel, S., Koblitz, L., Schwarz, K., Seigel, G. M., et al. (2008). RIBEYE recruits Munc119, a mammalian ortholog of the Caenorhabditis elegans protein unc119, to synaptic ribbons of photoreceptor synapses. J. Biol. Chem. 283, 26461–26467. doi: 10.1074/jbc.M801625200
Arshavsky, V. Y., and Burns, M. E. (2012). Photoreceptor signaling: supporting vision across a wide range of light intensities. J. Biol. Chem. 287, 1620–1626. doi: 10.1074/jbc.R111.305243
Arshavsky, V. Y., Lamb, T. D., and Pugh, E. N. Jr. (2002). G proteins and phototransduction. Annu. Rev. Physiol. 64, 153–187. doi: 10.1146/annurev.physiol.64.082701.102229
Artemyev, N. O. (2008). Light-dependent compartmentalization of transducin in rod photoreceptors. Mol. Neurobiol. 37, 44–51. doi: 10.1007/s12035-008-8015-2
Bernard, M. L., Peterson, Y. K., Chung, P., Jourdan, J., and Lanier, S. M. (2001). Selective interaction of AGS3 with G-proteins and the influence of AGS3 on the activation state of G-proteins. J. Biol. Chem. 276, 1585–1593. doi: 10.1074/jbc.m005291200
Bijveld, M. M., Florijn, R. J., Bergen, A. A., van den Born, L. I., Kamermans, M., Prick, L., et al. (2013). Genotype and phenotype of 101 dutch patients with congenital stationary night blindness. Ophthalmology 120, 2072–2081. doi: 10.1016/j.ophtha.2013.03.002
Blackmer, T., Larsen, E. C., Bartleson, C., Kowalchyk, J. A., Yoon, E. J., Preininger, A. M., et al. (2005). G protein betagamma directly regulates SNARE protein fusion machinery for secretory granule exocytosis. Nat. Neurosci. 8, 421–425. doi: 10.1038/nn1423
Blackmer, T., Larsen, E. C., Takahashi, M., Martin, T. F., Alford, S., and Hamm, H. E. (2001). G protein betagamma subunit-mediated presynaptic inhibition: regulation of exocytotic fusion downstream of Ca2+ entry. Science 292, 293–297. doi: 10.1126/science.1058803
Blumer, J. B., Smrcka, A. V., and Lanier, S. M. (2007). Mechanistic pathways and biological roles for receptor-independent activators of G-protein signaling. Pharmacol. Ther. 113, 488–506. doi: 10.1016/j.pharmthera.2006.11.001
Bocchero, U., Campla, C., Strickland, R., Dey, P., Swaroop, A., and Pahlberg, J. (2020). Transducin translocation and the role of Frmpd1 and Gpsm2 in rod to rod bipolar cell synaptic transmission. Invest. Ophthalmol. Vis. Sci. 61, 1102–1102.
Boularan, C., Hwang, I. Y., Kamenyeva, O., Park, C., Harrison, K., Huang, Z., et al. (2015). B lymphocyte-specific loss of Ric-8A results in a Galpha protein deficit and severe humoral immunodeficiency. J. Immunol. 195, 2090–2102. doi: 10.4049/jimmunol.1500523
Calvert, P. D., Strissel, K. J., Schiesser, W. E., Pugh, E. N. Jr., and Arshavsky, V. Y. (2006). Light-driven translocation of signaling proteins in vertebrate photoreceptors. Trends Cell Biol. 16, 560–568. doi: 10.1016/j.tcb.2006.09.001
Catterall, W. A. (2011). Voltage-gated calcium channels. Cold Spring Harb. Perspect. Biol. 3:a003947. doi: 10.1101/cshperspect.a003947
Chan, P., Gabay, M., Wright, F. A., Kan, W., Oner, S. S., Lanier, S. M., et al. (2011). Purification of heterotrimeric G protein alpha subunits by GST-Ric-8 association: primary characterization of purified G alpha(olf). J. Biol. Chem. 286, 2625–2635. doi: 10.1074/jbc.M110.178897
Chan, P., Thomas, C. J., Sprang, S. R., and Tall, G. G. (2013). Molecular chaperoning function of Ric-8 is to fold nascent heterotrimeric G protein alpha subunits. Proc. Natl. Acad. Sci. U.S.A. 110, 3794–3799. doi: 10.1073/pnas.1220943110
Cheguru, P., Majumder, A., Yadav, R., Gopalakrishna, K. N., Gakhar, L., and Artemyev, N. O. (2015). The solution structure of the transducin-alpha-uncoordinated 119 protein complex suggests occlusion of the Gbeta(1)gamma(1)-binding sites. FEBS J. 282, 550–561. doi: 10.1111/febs.13161
Cowan, C. W., He, W., and Wensel, T. G. (2001). RGS proteins: lessons from the RGS9 subfamily. Prog. Nucleic Acid Res. Mol. Biol. 65, 341–359. doi: 10.1016/s0079-6603(00)65009-2
David, N. B., Martin, C. A., Segalen, M., Rosenfeld, F., Schweisguth, F., and Bellaiche, Y. (2005). Drosophila Ric-8 regulates Galphai cortical localization to promote Galphai-dependent planar orientation of the mitotic spindle during asymmetric cell division. Nat. Cell Biol. 7, 1083–1090. doi: 10.1038/ncb1319
Dhingra, A., Jiang, M., Wang, T. L., Lyubarsky, A., Savchenko, A., Bar-Yehuda, T., et al. (2002). Light response of retinal ON bipolar cells requires a specific splice variant of Galpha(o). J. Neurosci. 22, 4878–4884. doi: 10.1523/jneurosci.22-12-04878.2002
Dhingra, A., Lyubarsky, A., Jiang, M., Pugh, E. N. Jr., Birnbaumer, L., Sterling, P., et al. (2000). The light response of ON bipolar neurons requires G[alpha]o. J. Neurosci. 20, 9053–9058. doi: 10.1523/jneurosci.20-24-09053.2000
di Pietro, F., Echard, A., and Morin, X. (2016). Regulation of mitotic spindle orientation: an integrated view. EMBO Rep. 17, 1106–1130. doi: 10.15252/embr.201642292
Do, M. T., and Yau, K. W. (2010). Intrinsically photosensitive retinal ganglion cells. Physiol. Rev. 90, 1547–1581. doi: 10.1152/physrev.00013.2010
Fain, G. L. (2006). Why photoreceptors die (and why they don’t). Bioessays 28, 344–354. doi: 10.1002/bies.20382
Fansa, E. K., and Wittinghofer, A. (2016). Sorting of lipidated cargo by the Arl2/Arl3 system. Small GTPases 7, 222–230. doi: 10.1080/21541248.2016.1224454
Frederick, J. M., Hanke-Gogokhia, C., Ying, G., and Baehr, W. (2020). Diffuse or hitch a ride: how photoreceptor lipidated proteins get from here to there. Biol. Chem. 401, 573–584. doi: 10.1515/hsz-2019-0375
Fu, Y., and Yau, K. W. (2007). Phototransduction in mouse rods and cones. Pflugers Arch. 454, 805–819. doi: 10.1007/s00424-006-0194-y
Gabay, M., Pinter, M. E., Wright, F. A., Chan, P., Murphy, A. J., Valenzuela, D. M., et al. (2011). Ric-8 proteins are molecular chaperones that direct nascent G protein alpha subunit membrane association. Sci. Signal. 4:ra79. doi: 10.1126/scisignal.2002223
Gao, Y., Eskici, G., Ramachandran, S., Poitevin, F., Seven, A. B., Panova, O., et al. (2020). Structure of the visual signaling complex between transducin and phosphodiesterase 6. bioRxiv [Preprint]
Gao, Y., Hu, H., Ramachandran, S., Erickson, J. W., Cerione, R. A., and Skiniotis, G. (2019). Structures of the rhodopsin-transducin complex: insights into G-protein activation. Mol. Cell 75, 781–790.e3. doi: 10.1016/j.molcel.2019.06.007
Gopalakrishna, K. N., Doddapuneni, K., Boyd, K. K., Masuho, I., Martemyanov, K. A., and Artemyev, N. O. (2011). Interaction of transducin with uncoordinated 119 protein (UNC119): implications for the model of transducin trafficking in rod photoreceptors. J. Biol. Chem. 286, 28954–28962. doi: 10.1074/jbc.M111.268821
Haeseleer, F. (2008). Interaction and colocalization of CaBP4 and Unc119 (MRG4) in photoreceptors. Invest. Ophthalmol. Vis. Sci. 49, 2366–2375. doi: 10.1167/iovs.07-1166
Haeseleer, F., Imanishi, Y., Maeda, T., Possin, D. E., Maeda, A., Lee, A., et al. (2004). Essential role of Ca2+-binding protein 4, a Cav1.4 channel regulator, in photoreceptor synaptic function. Nat. Neurosci. 7, 1079–1087. doi: 10.1038/nn1320
He, F., Agosto, M. A., Anastassov, I. A., Tse, D. Y., Wu, S. M., and Wensel, T. G. (2016). Phosphatidylinositol-3-phosphate is light-regulated and essential for survival in retinal rods. Sci. Rep. 6:26978. doi: 10.1038/srep26978
He, W., Cowan, C. W., and Wensel, T. G. (1998). RGS9, a GTPase accelerator for phototransduction. Neuron 20, 95–102. doi: 10.1016/s0896-6273(00)80437-7
He, W., Lu, L., Zhang, X., El-Hodiri, H. M., Chen, C. K., Slep, K. C., et al. (2000). Modules in the photoreceptor RGS9-1.Gbeta 5L GTPase-accelerating protein complex control effector coupling, GTPase acceleration, protein folding, and stability. J. Biol. Chem. 275, 37093–37100. doi: 10.1074/jbc.M006982200
Herrmann, R., Lobanova, E. S., Hammond, T., Kessler, C., Burns, M. E., Frishman, L. J., et al. (2010). Phosducin regulates transmission at the photoreceptor-to-ON-bipolar cell synapse. J. Neurosci. 30, 3239–3253. doi: 10.1523/JNEUROSCI.4775-09.2010
Higashide, T., McLaren, M. J., and Inana, G. (1998). Localization of HRG4, a photoreceptor protein homologous to Unc-119, in ribbon synapse. Invest. Ophthalmol. Vis. Sci. 39, 690–698.
Higashide, T., Murakami, A., McLaren, M. J., and Inana, G. (1996). Cloning of the cDNA for a novel photoreceptor protein. J. Biol. Chem. 271, 1797–1804. doi: 10.1074/jbc.271.3.1797
Ishiba, Y., Higashide, T., Mori, N., Kobayashi, A., Kubota, S., McLaren, M. J., et al. (2007). Targeted inactivation of synaptic HRG4 (UNC119) causes dysfunction in the distal photoreceptor and slow retinal degeneration, revealing a new function. Exp. Eye Res. 84, 473–485. doi: 10.1016/j.exer.2006.10.016
Ismail, S. A., Chen, Y. X., Miertzschke, M., Vetter, I. R., Koerner, C., and Wittinghofer, A. (2012). Structural basis for Arl3-specific release of myristoylated ciliary cargo from UNC119. EMBO J. 31, 4085–4094. doi: 10.1038/emboj.2012.257
Jean, P., Ozcete, O. D., Tarchini, B., and Moser, T. (2019). Intrinsic planar polarity mechanisms influence the position-dependent regulation of synapse properties in inner hair cells. Proc. Natl. Acad. Sci. U.S.A. 116, 9084–9093. doi: 10.1073/pnas.1818358116
Karim, Z., Vepachedu, R., Gorska, M., and Alam, R. (2010). UNC119 inhibits dynamin and dynamin-dependent endocytic processes. Cell. Signal. 22, 128–137. doi: 10.1016/j.cellsig.2009.09.022
Kerov, V. S., Natochin, M., and Artemyev, N. O. (2005). Interaction of transducin-alpha with LGN, a G-protein modulator expressed in photoreceptor cells. Mol. Cell. Neurosci. 28, 485–495. doi: 10.1016/j.mcn.2004.10.010
Khan, S. M., Sleno, R., Gora, S., Zylbergold, P., Laverdure, J. P., Labbe, J. C., et al. (2013). The expanding roles of Gbetagamma subunits in G protein-coupled receptor signaling and drug action. Pharmacol. Rev. 65, 545–577. doi: 10.1124/pr.111.005603
Klattenhoff, C., Montecino, M., Soto, X., Guzman, L., Romo, X., Garcia, M. A., et al. (2003). Human brain synembryn interacts with Gsalpha and Gqalpha and is translocated to the plasma membrane in response to isoproterenol and carbachol. J. Cell. Physiol. 195, 151–157. doi: 10.1002/jcp.10300
Kobayashi, A., Higashide, T., Hamasaki, D., Kubota, S., Sakuma, H., An, W., et al. (2000). HRG4 (UNC119) mutation found in cone-rod dystrophy causes retinal degeneration in a transgenic model. Invest. Ophthalmol. Vis. Sci. 41, 3268–3277.
Lai, C. W., Kolesnikov, A. V., Frederick, J. M., Blake, D. R., Jiang, L., Stewart, J. S., et al. (2013). Phosducin-like protein 1 is essential for G-protein assembly and signaling in retinal rod photoreceptors. J. Neurosci. 33, 7941–7951. doi: 10.1523/JNEUROSCI.5001-12.2013
Lancaster, M. A., and Knoblich, J. A. (2012). Spindle orientation in mammalian cerebral cortical development. Curr. Opin. Neurobiol. 22, 737–746. doi: 10.1016/j.conb.2012.04.003
Li, S., Chen, D., Sauve, Y., McCandless, J., Chen, Y. J., and Chen, C. K. (2005). Rhodopsin-iCre transgenic mouse line for Cre-mediated rod-specific gene targeting. Genesis 41, 73–80. doi: 10.1002/gene.20097
Littink, K. W., Koenekoop, R. K., van den Born, L. I., Collin, R. W., Moruz, L., Veltman, J. A., et al. (2010). Homozygosity mapping in patients with cone-rod dystrophy: novel mutations and clinical characterizations. Invest. Ophthalmol. Vis. Sci. 51, 5943–5951. doi: 10.1167/iovs.10-5797
Long, J. H., Arshavsky, V. Y., and Burns, M. E. (2013). Absence of synaptic regulation by phosducin in retinal slices. PLoS One 8:e83970. doi: 10.1371/journal.pone.0083970
Ma, S., Kwon, H. J., and Huang, Z. (2012). Ric-8a, a guanine nucleotide exchange factor for heterotrimeric G proteins, regulates bergmann glia-basement membrane adhesion during cerebellar foliation. J. Neurosci. 32, 14979–14993. doi: 10.1523/JNEUROSCI.1282-12.2012
Maduro, M., and Pilgrim, D. (1995). Identification and cloning of Unc-119, a gene expressed in the Caenorhabditis elegans nervous system. Genetics 141, 977–988.
Majumder, A., Pahlberg, J., Boyd, K. K., Kerov, V., Kolandaivelu, S., Ramamurthy, V., et al. (2013). Transducin translocation contributes to rod survival and enhances synaptic transmission from rods to rod bipolar cells. Proc. Natl. Acad. Sci. U.S.A. 110, 12468–12473. doi: 10.1073/pnas.1222666110
Makino, E. R., Handy, J. W., Li, T., and Arshavsky, V. Y. (1999). The GTPase activating factor for transducin in rod photoreceptors is the complex between RGS9 and type 5 G protein beta subunit. Proc. NatL. Acad. Sci. U.S.A. 96, 1947–1952. doi: 10.1073/pnas.96.5.1947
Martemyanov, K. A., and Arshavsky, V. Y. (2009). Biology and functions of the RGS9 isoforms. Prog. Mol. Biol. Transl. Sci. 86, 205–227. doi: 10.1016/s1877-1173(09)86007-9
McClelland, L. J., Zhang, K., Mou, T. C., Johnston, J., Yates-Hansen, C., Li, S., et al. (2020). Structure of the G protein chaperone and guanine nucleotide exchange factor Ric-8A bound to Galphai1. Nat. Commun. 11:1077.
Miller, K. G., Alfonso, A., Nguyen, M., Crowell, J. A., Johnson, C. D., and Rand, J. B. (1996). A genetic selection for Caenorhabditis elegans synaptic transmission mutants. Proc. Natl. Acad. Sci. U.S.A. 93, 12593–12598. doi: 10.1073/pnas.93.22.12593
Miller, K. G., Emerson, M. D., McManus, J. R., and Rand, J. B. (2000). RIC-8 (Synembryn): a novel conserved protein that is required for G(q)alpha signaling in the C. elegans nervous system. Neuron 27, 289–299. doi: 10.1016/s0896-6273(00)00037-4
Mochizuki, N., Cho, G., Wen, B., and Insel, P. A. (1996). Identification and cDNA cloning of a novel human mosaic protein, LGN, based on interaction with G alpha i2. Gene 181, 39–43. doi: 10.1016/s0378-1119(96)00456-8
Nagai, Y., Nishimura, A., Tago, K., Mizuno, N., and Itoh, H. (2010). Ric-8B stabilizes the alpha subunit of stimulatory G protein by inhibiting its ubiquitination. J. Biol. Chem. 285, 11114–11120. doi: 10.1074/jbc.M109.063313
Nair, K. S., Mendez, A., Blumer, J. B., Rosenzweig, D. H., and Slepak, V. Z. (2005). The presence of a Leu-Gly-Asn repeat-enriched protein (LGN), a putative binding partner of transducin, in ROD photoreceptors. Invest. Ophthalmol. Vis. Sci. 46, 383–389. doi: 10.1167/iovs.04-1006
Natochin, M., Granovsky, A. E., and Artemyev, N. O. (1998). Identification of effector residues on photoreceptor G protein, transducin. J. Biol. Chem. 273, 21808–21815. doi: 10.1074/jbc.273.34.21808
Natochin, M., Lester, B., Peterson, Y. K., Bernard, M. L., Lanier, S. M., and Artemyev, N. O. (2000). AGS3 inhibits GDP dissociation from α subunits of the Gi family and rhodopsin-dependent activation of transducin. J. Biol. Chem. 275, 40981–40985. doi: 10.1074/jbc.m006478200
Papasergi, M. M., Patel, B. R., and Tall, G. G. (2015). The G protein alpha chaperone Ric-8 as a potential therapeutic target. Mol. Pharmacol. 87, 52–63. doi: 10.1124/mol.114.094664
Peng, Y. W., Zallocchi, M., Wang, W. M., Delimont, D., and Cosgrove, D. (2011). Moderate light-induced degeneration of rod photoreceptors with delayed transducin translocation in shaker1 mice. Invest. Ophthalmol. Vis. Sci. 52, 6421–6427. doi: 10.1167/iovs.10-6557
Ruisu, K., Kask, K., Meier, R., Saare, M., Raid, R., Veraksits, A., et al. (2013). Ablation of RIC8A function in mouse neurons leads to a severe neuromuscular phenotype and postnatal death. PLoS One 8:e74031. doi: 10.1371/journal.pone.0074031
Schmidt, T. M., Do, M. T., Dacey, D., Lucas, R., Hattar, S., and Matynia, A. (2011). Melanopsin-positive intrinsically photosensitive retinal ganglion cells: from form to function. J. Neurosci. 31, 16094–16101. doi: 10.1523/JNEUROSCI.4132-11.2011
Schmitz, F., Konigstorfer, A., and Sudhof, T. C. (2000). RIBEYE, a component of synaptic ribbons: a protein’s journey through evolution provides insight into synaptic ribbon function. Neuron 28, 857–872. doi: 10.1016/s0896-6273(00)00159-8
Seven, A. B., Hilger, D., Papasergi-Scott, M. M., Zhang, L., Qu, Q., Kobilka, B. K., et al. (2020). Structures of Galpha proteins in complex with their chaperone reveal quality control mechanisms. Cell Rep. 30, 3699–3709.e6. doi: 10.1016/j.celrep.2020.02.086
Sinha, S., Majumder, A., Belcastro, M., Sokolov, M., and Artemyev, N. O. (2013). Expression and subcellular distribution of UNC119a, a protein partner of transducin alpha subunit in rod photoreceptors. Cell. Signal. 25, 341–348. doi: 10.1016/j.cellsig.2012.10.005
Skiba, N. P., Bae, H., and Hamm, H. E. (1996). Mapping of effector binding sites of transducin alpha-subunit using G alpha t/G alpha i1 chimeras. J. Biol. Chem. 271, 413–424. doi: 10.1074/jbc.271.1.413
Slepak, V. Z., and Hurley, J. B. (2008). Mechanism of light-induced translocation of arrestin and transducin in photoreceptors: interaction-restricted diffusion. IUBMB Life 60, 2–9. doi: 10.1002/iub.7
Sokolov, M., Lyubarsky, A. L., Strissel, K. J., Savchenko, A. B., Govardovskii, V. I., Pugh, E. N., et al. (2002). Massive light-driven translocation of transducin between the two major compartments of rod cells: a novel mechanism of light adaptation. Neuron 34, 95–106. doi: 10.1016/s0896-6273(02)00636-0
Srivastava, D., and Artemyev, N. O. (2019). Large-scale conformational rearrangement of the alpha5-helix of Galpha subunits in complex with the guanine nucleotide exchange factor Ric8A. J. Biol. Chem. 294, 17875–17882. doi: 10.1074/jbc.AC119.011135
Srivastava, D., and Artemyev, N. O. (2020). Ric-8A, a GEF, and a chaperone for g protein alpha-subunits: evidence for the two-faced interface. Bioessays 42:e1900208. doi: 10.1002/bies.201900208
Srivastava, D., Gakhar, L., and Artemyev, N. O. (2019). Structural underpinnings of Ric8A function as a G-protein alpha-subunit chaperone and guanine-nucleotide exchange factor. Nat. Commun. 10:3084. doi: 10.1038/s41467-019-11088-x
Sundermeier, T. R., Zhang, N., Vinberg, F., Mustafi, D., Kohno, H., Golczak, M., et al. (2014). DICER1 is essential for survival of postmitotic rod photoreceptor cells in mice. FASEB J. 28, 3780–3791. doi: 10.1096/fj.14-254292
Swanson, D. A., Chang, J. T., Campochiaro, P. A., Zack, D. J., and Valle, D. (1998). Mammalian orthologs of C. elegans unc-119 highly expressed in photoreceptors. Invest. Ophthalmol. Vis. Sci. 39, 2085–2094.
Tall, G. G., and Gilman, A. G. (2005). Resistance to inhibitors of cholinesterase 8A catalyzes release of Galphai-GTP and nuclear mitotic apparatus protein (NuMA) from NuMA/LGN/Galphai-GDP complexes. Proc. Natl. Acad. Sci. U.S.A. 102, 16584–16589. doi: 10.1073/pnas.0508306102
Tall, G. G., Krumins, A. M., and Gilman, A. G. (2003). Mammalian Ric-8A (synembryn) is a heterotrimeric Galpha protein guanine nucleotide exchange factor. J. Biol. Chem. 278, 8356–8362. doi: 10.1074/jbc.M211862200
Tian, M., Wang, W., Delimont, D., Cheung, L., Zallocchi, M., Cosgrove, D., et al. (2014). Photoreceptors in whirler mice show defective transducin translocation and are susceptible to short-term light/dark changes-induced degeneration. Exp. Eye Res. 118, 145–153. doi: 10.1016/j.exer.2013.10.021
Tonissoo, T., Lulla, S., Meier, R., Saare, M., Ruisu, K., Pooga, M., et al. (2010). Nucleotide exchange factor RIC-8 is indispensable in mammalian early development. Dev. Dyn. 239, 3404–3415. doi: 10.1002/dvdy.22480
Van Hook, M. J., Babai, N., Zurawski, Z., Yim, Y. Y., Hamm, H. E., and Thoreson, W. B. (2017). A presynaptic group III mGluR recruits Gbetagamma/SNARE interactions to inhibit synaptic transmission by cone photoreceptors in the vertebrate retina. J. Neurosci. 37, 4618–4634. doi: 10.1523/JNEUROSCI.2948-16.2017
Von Dannecker, L. E., Mercadante, A. F., and Malnic, B. (2005). Ric-8B, an olfactory putative GTP exchange factor, amplifies signal transduction through the olfactory-specific G-protein Galphaolf. J. Neurosci. 25, 3793–3800. doi: 10.1523/JNEUROSCI.4595-04.2005
Yoon, E. J., Gerachshenko, T., Spiegelberg, B. D., Alford, S., and Hamm, H. E. (2007). Gbetagamma interferes with Ca2+-dependent binding of synaptotagmin to the soluble N-ethylmaleimide-sensitive factor attachment protein receptor (SNARE) complex. Mol. Pharmacol. 72, 1210–1219. doi: 10.1124/mol.107.039446
Zamponi, G. W., and Currie, K. P. (2013). Regulation of Ca(V)2 calcium channels by G protein coupled receptors. Biochim. Biophys. Acta 1828, 1629–1643. doi: 10.1016/j.bbamem.2012.10.004
Zeng, B., Mou, T. C., Doukov, T. I., Steiner, A., Yu, W., Papasergi-Scott, M., et al. (2019). Structure, function, and dynamics of the Galpha binding domain of Ric-8A. Structure 27, 1137–1147.e5. doi: 10.1016/j.str.2019.04.013
Zhang, H., Constantine, R., Vorobiev, S., Chen, Y., Seetharaman, J., Huang, Y. J., et al. (2011). UNC119 is required for G protein trafficking in sensory neurons. Nat. Neurosci. 14, 874–880. doi: 10.1038/nn.2835
Keywords: retina, G protein, GPCR, transducin, Ric-8A, GEF, chaperone
Citation: Srivastava D, Yadav RP, Inamdar SM, Huang Z, Sokolov M, Boyd K and Artemyev NO (2020) Transducin Partners Outside the Phototransduction Pathway. Front. Cell. Neurosci. 14:589494. doi: 10.3389/fncel.2020.589494
Received: 30 July 2020; Accepted: 10 September 2020;
Published: 14 October 2020.
Edited by:
Wallace B. Thoreson, University of Nebraska Medical Center, United StatesReviewed by:
Kirill Martemyanov, The Scripps Research Institute, United StatesBela Volgyi, University of Pécs, Hungary
Copyright © 2020 Srivastava, Yadav, Inamdar, Huang, Sokolov, Boyd and Artemyev. This is an open-access article distributed under the terms of the Creative Commons Attribution License (CC BY). The use, distribution or reproduction in other forums is permitted, provided the original author(s) and the copyright owner(s) are credited and that the original publication in this journal is cited, in accordance with accepted academic practice. No use, distribution or reproduction is permitted which does not comply with these terms.
*Correspondence: Nikolai O. Artemyev, bmlrb2xhaS1hcnRlbXlldkB1aW93YS5lZHU=