- Department of Mechano-Informatics, Graduate School of Information Science and Technology, The University of Tokyo, Tokyo, Japan
Introduction: Both tinnitus and hyperacusis, likely triggered by hearing loss, can be attributed to maladaptive plasticity in auditory perception. However, owing to their co-occurrence, disentangling their neural mechanisms proves difficult. We hypothesized that the neural correlates of tinnitus are associated with neural activities triggered by low-intensity tones, while hyperacusis is linked to responses to moderate- and high-intensity tones.
Methods: To test these hypotheses, we conducted behavioral and electrophysiological experiments in rats 2 to 8 days after traumatic tone exposure.
Results: In the behavioral experiments, prepulse and gap inhibition tended to exhibit different frequency characteristics (although not reaching sufficient statistical levels), suggesting that exposure to traumatic tones led to acute symptoms of hyperacusis and tinnitus at different frequency ranges. When examining the auditory cortex at the thalamocortical recipient layer, we observed that tinnitus symptoms correlated with a disorganized tonotopic map, typically characterized by responses to low-intensity tones. Neural correlates of hyperacusis were found in the cortical recruitment function at the multi-unit activity (MUA) level, but not at the local field potential (LFP) level, in response to moderate- and high-intensity tones. This shift from LFP to MUA was associated with a loss of monotonicity, suggesting a crucial role for inhibitory synapses.
Discussion: Thus, in acute symptoms of traumatic tone exposure, our experiments successfully disentangled the neural correlates of tinnitus and hyperacusis at the thalamocortical recipient layer of the auditory cortex. They also suggested that tinnitus is linked to central noise, whereas hyperacusis is associated with aberrant gain control. Further interactions between animal experiments and clinical studies will offer insights into neural mechanisms, diagnosis and treatments of tinnitus and hyperacusis, specifically in terms of long-term plasticity of chronic symptoms.
1 Introduction
Hearing loss is often associated with a high comorbidity of tinnitus and hyperacusis. For instance, more than 60% of patients with tinnitus also experience hyperacusis, and conversely, over 80% of patients with hyperacusis suffer from chronic tinnitus (Anari et al., 1999; Dauman and Bouscau-Faure, 2005; Sztuka et al., 2010; Schecklmann et al., 2014). Both tinnitus and hyperacusis likely result from maladaptive changes in gain control within the auditory system. This maladaptive gain control is characterized by an increase in the activity of the central auditory pathway, which is triggered by a decrease in peripheral input (Eggermont and Roberts, 2004; Schaette and Kempter, 2006; Robinson and McAlpine, 2009; Roberts et al., 2010; Zeng, 2013; Auerbach et al., 2014; Shore et al., 2016; Auerbach et al., 2019; Herrmann and Butler, 2021; Auerbach and Gritton, 2022). The maladaptive gain control may be attributed to homeostatic plasticity, a mechanism that maintains baseline activity levels following perturbations (Turrigiano and Nelson, 2004; Turrigiano, 2012). In the context of hearing loss, this homeostatic plasticity can distort neural representations and lead to significant auditory perceptual challenges (Schaette and Kempter, 2006; Norena, 2011; Nahmani and Turrigiano, 2014; Eggermont, 2017a,b; Herrmann and Butler, 2021). This distortion can involve synaptic sensitization through receptor upregulation (Sturm et al., 2017; Balaram et al., 2019), synaptic disinhibition (Sarro et al., 2008; Sanes and Kotak, 2011; Sturm et al., 2017; Balaram et al., 2019), and increased intrinsic excitability (burstiness; Pilati et al., 2012; Yang et al., 2012; Li et al., 2013, 2015; Shore et al., 2016; Wu et al., 2016). Additionally, non-homeostatic regulation of sound intensity may also play a role, as gain control following acoustic trauma is heterogeneous among pyramidal neurons in the auditory cortex (McGill et al., 2022).
Acoustic trauma reduces spontaneous and sound-evoked activities at the auditory nerve (Kiang et al., 1970; Wake et al., 1993; Wang et al., 1997; Heinz and Young, 2004; Heinz et al., 2005; Hickox and Liberman, 2014). However, in response to this trauma, it induces hyperactivity and synchrony in spontaneous and sound-evoked activities at the cochlear nucleus (Kaltenbach and McCaslin, 1996; Kaltenbach and Afman, 2000; Kaltenbach et al., 2000; Cai et al., 2009; Wu et al., 2016), the inferior colliculus (Salvi et al., 1990; Bauer et al., 2008; Mulders and Robertson, 2009; Sun et al., 2011; Hickox and Liberman, 2014; Hesse et al., 2016; Xiong et al., 2017), and the auditory cortex (Popelar et al., 1987; Syka et al., 1994; Komiya and Eggermont, 2000; Qiu et al., 2000; Norena et al., 2003; Norena and Eggermont, 2003; Seki and Eggermont, 2003; Kotak et al., 2005; Yang et al., 2011; Sun et al., 2012; Basura et al., 2015; Chambers et al., 2016; Resnik and Polley, 2017; Asokan et al., 2018; Wong et al., 2020; Resnik and Polley, 2021; Parameshwarappa et al., 2022). Furthermore, resting-state fMRI in rats with drug-induced depression of the cochlea has revealed hyperactivity in various brain regions, including the cerebellum, reticular formation, amygdala, hippocampus, and the higher-order auditory pathway, which encompasses the inferior colliculus, medial geniculate body, and auditory cortex (Chen et al., 2015).
The aberrant neural activities are believed to underlie hyperacusis, defined as “a reduced tolerance to sounds that are perceived as normal by the majority of the population” (Adams et al., 2021). In hyperacusis, moderate-intensity sounds are perceived as intolerably loud, aversive, or even painful (Anari et al., 1999; Baguley, 2003; Auerbach et al., 2014; Pienkowski, 2017, 2019; Eggermont, 2017a,b; Auerbach et al., 2019). Human imaging studies further support the presence of sound-evoked hyperactivity across multiple auditory nuclei in patients with hyperacusis (Lockwood et al., 1998; Lanting et al., 2008; Hwang et al., 2009; Melcher et al., 2009; Gu et al., 2010; Koops and van Dijk, 2021; Bigras et al., 2023). This hyperactivity is also associated with a steep growth function of sound-evoked activities concerning test intensity (Zeng, 2013, 2020; Auerbach et al., 2019).
While hyperacusis arises from abnormal gain of evoked responses, one of possible mechanisms of tinnitus is increased central noise, independent of gain (Gu et al., 2010; Knipper et al., 2013; Zeng, 2013) [but some studies note that changes in firing rates are not necessarily tinnitus specific (Coomber et al., 2014; Ropp et al., 2014; Longenecker and Galazyuk, 2016)]. This model predicts gain reduction (Hofmeier et al., 2018) and a dissociation between cortical and subcortical activities (Boyen et al., 2014) in tinnitus frequency. Conversely, it predicts gain increase (Diehl and Schaette, 2015) and hyperactivity in the frequency related to hyperacusis, affecting both subcortical and cortical activities (Gu et al., 2010; Knipper et al., 2013; Ruttiger et al., 2013; Chen et al., 2015). However, it can be challenging to disentangle these effects, primarily owing to the co-occurrence of tinnitus and hyperacusis (Lanting et al., 2008; Melcher et al., 2009; Schecklmann et al., 2014; Cederroth et al., 2020). Consequently, hyperactivity within a specific region on the tonotopic map in the auditory cortex is considered a neural signature of either tinnitus or hyperacusis (Norena, 2011; Auerbach et al., 2019; Herrmann and Butler, 2021; McGill et al., 2022), which has not been reliably distinguished based on their associated symptoms.
In this study, we aimed to disentangle the neural correlates of tinnitus and hyperacusis within the auditory cortex of noise-exposed rats. Several behavioral tests have been employed to estimate tinnitus and hyperacusis in animal models (Hayes et al., 2014). Specifically, prepulse and gap inhibitions (PPI and GPI) of acoustic startles have been established as behavioral indicators of hyperacusis and tinnitus symptoms in animals, respectively, in a similar fashion of experiments (Turner and Larsen, 2016). Furthermore, despite being a reflexive measure, we recently demonstrated that PPI can predict subjective pure-tone audiometry based on operant conditioning in rats (Wake et al., 2021).
Our initial focus was to confirm the distinct characteristics of PPI and GPI in rats with noise-induced hearing loss, which suggest that acoustic trauma made hyperacusis and tinnitus in different frequencies. Subsequently, we delved into the neural correlates of PPI (i.e., hyperacusis symptoms) and GPI (tinnitus) within the auditory cortex using high-density microelectrode array mapping. Given that tinnitus is associated with increased central noise, we expected to find the neural correlates of tinnitus in response to faint tones with a low signal-to-noise (S/N) ratio. Accordingly, we hypothesized that GPI is correlated with the extent of spatial disorganization of the characteristic frequency (CF) in the auditory cortex, which is typically characterized with low-intensity tones. Furthermore, we postulated that, unlike the putative neural correlates of tinnitus, hyperacusis-like symptoms are associated with neural gain from synaptic inputs to spike outputs in response to moderate- and high-intensity tones.
To investigate this, we measured neural activities at both the local field potential (LFP) and multi-unit activity (MUA) levels, and attempted to characterize the neural gain from LFP to MUA. We here assumed that the first negative deflection of auditory-evoked LFPs in layer 4 reflected synaptic inputs of thalamo-cortical projection rather than suprathreshold discharges, based on previous physiological and simulation studies (Einevoll et al., 2013; Mazzoni et al., 2015; Haider et al., 2016). Assuming that LFP and MUA represent cortical inputs and cortical responses, respectively, our hypothesis predicts that the neural gain from LFP to MUA is correlated with the enhancement of PPI induced by trauma. To estimate the neural gain, we quantified the cortical recruitment, or the activation level of the entire auditory cortex, from either tone-evoked LFP or MUA. We attempted to compare the cortical recruitment between LFP and MUA to investigate how efficiently the thalamo-cortical synaptic inputs were converted into cortical discharges.
2 Materials and methods
This study was conducted in accordance with the National Institutes of Health guide for the care and use of Laboratory animals (NIH Publications No. 8023, revised 1978) and following the recommendations of the ARRIVE guidelines.1 All the procedures that involved the care and use of animals were approved by the Committee on the Ethics of Animal Experiments at the Research Center for Advanced Science and Technology, The University of Tokyo (RAC170005). Surgery, traumatic noise exposure, and neural recording were performed under isoflurane anesthesia (3% for induction and 1%–2% for maintenance), and every effort was made to minimize the suffering of the animals. All experiments were carried out in a sound-attenuating chamber (AMC-4015; O’Hara & Co. Ltd., Tokyo, Japan), where the background noise level was 32.1 dB (A-weighted equivalent continuous sound level; MT-325, Mothertool Co., Ltd., Nagano, Japan).
2.1 Animals
A total of 16 male Wistar rats (7–10 weeks old, body weight, 250-460 g) were used in this study. In eight of the 16 rats, acoustic trauma was induced in the left ear by exposing them to a 10-kHz tone with an intensity of 125 dB SPL (Sound Pressure Level in dB with respect to 20 μPa) for 1 h using a loudspeaker (Selenium ST 400, Los Angeles, CA; Wake et al., 2019). To safeguard the hearing in the right ear during exposure, a silicone impression material (Dent Silicone-V, Shohu, Kyoto, Japan) was injected into the right ear canal. PPI and GPI in these exposed animals were recorded before and 2–8 days after the traumatic noise exposure to measure changes in their hearing sensitivity to tones. Immediately following the recording of both PPI and GPI for post-exposure measurements, LFP and MUA were recorded in the fourth layer of the right auditory cortex. The remaining eight animals were designated as a control group, and PPI, GPI, LFP, and MUA were recorded without exposing them to noise.
2.2 Behavioral experiments
The procedures for measuring PPI were described in detail in our previous work (Wake et al., 2021). Briefly, an acoustic startle stimulus presented through a speaker (DDL-RT16C, Alpine, Tokyo, Japan) was used as white noise (95-dB SPL, 10 ms). The startle reflex was measured using a force sensor attached to the floor where the animals were placed (PW6C 5KG; Unipulse Corp., Tokyo, Japan). The responses of the startle reflex were recorded using a DA converter (USB-6461, National Instruments, Austin, TX) and quantified by measuring the peak-to-peak magnitude of the force sensor output from 100 ms before to 200 ms after the startle stimulus emission. For each tone frequency (f [kHz]), which included both with and without prepulse conditions, PPI (and GPI) were defined as follows in Equation (1):
where Rw and Rw/o denote startle reflexes with and without the prepulse (and gap), respectively. Since the spontaneous movements of animals could sometimes interfere with the accurate detection of startle reflexes during certain trials (Tziridis et al., 2012), trials where the startle reflexes exceeded 2σ of the mean were excluded. The same formula and criteria were also applied to GPI(f), where startle reflexes were measured both with and without gaps when background tones with different frequencies were presented. Both PPI(f) and GPI(f) yield positive values when a prepulse or gap inhibits the startle reflexes.
GPI was first measured with five distinct background sounds; four continuous pure tones (each at 60 dB SPL) with frequencies of 4, 8, 16, and 32 kHz, along with broadband noise (BBN; also at 60 dB SPL) spanning from 0.1 to 64 kHz. A 50-ms silent gap was introduced after 20 ± 2 s of exposure to a background tone or noise. The time interval between the initiation of the startle stimulus and the gap period was set at 100 ms. At the beginning of GPI recording, rats were given a 120-s period to acclimate to the experiment environment. Following this, the startle stimuli were presented twice for habituation (Ison et al., 1973). The GPI recording encompassed 10 trials, each involving background sounds presented in a random order, both with and without gap conditions.
Subsequently, PPI was recorded after GPI. Five types of prepulses were used, including 50-ms tone bursts of 4, 8, 16, and 32 kHz (each at 60 dB SPL), along with a 50-ms BBN (60 dB SPL). The time interval between the startle stimulus and a prepulse was 100 ms. The startle stimulus was presented every 20 ± 2 s. Similar to the GPI measurement, the PPI measurement started with a 120-s acclimatization period and two habituation stimuli, and consisted of 10 trials. Each trial tested the five prepulses in a random order, both with and without prepulse conditions. For PPI, seven of the exposed animals were analyzed, owing to missing PPI data for one subject.
2.3 Electrophysiology
The procedures for recording neural activity in the auditory cortex were described in detail in our previous work (Wake et al., 2019). Briefly, rats were anesthetized with isoflurane. Then, cisternal cerebrospinal fluid drainage was performed to avoid cerebral edema. After that, the auditory cortex was surgically exposed, and the dura mater over the auditory cortex was removed. Atropine sulfate (Abbott Japan Co. Ltd., Tokyo, Japan; 0.1 mg/kg) was administered at the beginning of the surgery to reduce the bronchial secretion viscosity, whereas xylocaine was subcutaneously administered for local anesthesia when necessary. After locating the auditory cortex through surface recording, a microelectrode array (ICS-96; Blackrock Microsystems, Salt Lake City, UT, United States) was inserted at the depth of 600 μm, specifically within the fourth layer, to record the auditory-evoked MUA and LFP in the auditory cortex. The array was equipped with 10 × 10 recording electrodes, featuring 400-μm inter-electrode spacing and covering an area of 4 × 4 mm2 that spanned the entire auditory cortex. The Cerebus Data Acquisition System (Cyberkinetics, Inc., Salt Lake City, UT, United States) amplified neural signals 1,000 times and recorded LFP and MUA. The filter passband and the sampling rates were set at 0.3–500 Hz and 1 kHz for LFP and 250–7,500 Hz and 30 kHz for MUA, respectively. In online processing, multi-unit spikes were detected as threshold-crossing events with a threshold set at −5.65 times the root mean square from the average. No spike sorting was applied offline, assuming that MUA can be considered as spatial pooling of single unit activities as far as the number of units in MUA did not vary across recording sites. This assumption is reasonably valid in our low-impedance microelectrode recording (Noda and Takahashi, 2015).
The test stimuli consisted of tone bursts (5-ms rise/fall, 20-ms plateau) with varied frequencies and SPLs: 18 frequencies between 1.6 and 64 kHz with a 1/3-octave interval and seven SPLs from 20- to 80-dB SPL with a 10-dB interval. These test stimuli were calibrated with a 1/4-inch microphone at the pinna (4939, Brüel & Kjær, Nærum, Denmark) and were presented bilaterally through a speaker (DDLiner; Alpine Electronics of Australia Pty. Ltd., Hallam, Melbourne, VIC, Australia). For each combination of frequency and SPL, the test stimuli were presented 20 times in a pseudo-random fashion. The frequency response area (FRA) at each recording site was then determined by assessing the magnitude of neural responses as a function of tone frequency and SPL. The MUA magnitude was quantified as the number of tone-evoked spikes, defined as the difference between the total spike counts within 100 ms from the tone onset and the spontaneous spike counts within 4 ms from the tone onset (Guo et al., 2012; Noda and Takahashi, 2015). Additionally, LFP was characterized as the grand average across 20 trials, then the maximum amplitude (the first negative deflection) within the 10–60 ms time window was taken as the LFP magnitude (Takahashi et al., 2004, 2005a).
Based on the FRA, the CF at each recording site was determined as the test frequency where evoked MUAs were observed at the lowest SPL or where the largest evoked MUA was recorded at 20 dB SPL. The tonotopic maps within the auditory cortex were subsequently generated by spatially mapping these CF. Given that CF was characterized at the lowest possible SPL, these tonotopic maps were primarily characterized by low-intensity tones.
Additionally, cortical recruitment functions (CRFs) of tone-evoked MUA and LFP were also determined as a measure of the activation level of the entire auditory cortex for every condition of frequency and SPL (Kilgard and Merzenich, 1998; Takahashi et al., 2011). The CRFs were characterized across the entire range from the lowest SPL to the highest SPL tones. To construct the CRFs, the FRA was binarized based on a specified threshold, classifying each recording site as either active (1) or inactive (0) in response to each stimulus. The threshold to binarize the FRA was defined as previously described: For MUA, it was set as the inflection point of a smoothed z-score histogram of MUA (Guo et al., 2012; Noda and Takahashi, 2015); for LFP, it was determined as 1.2 times the baseline fluctuations within the 400–500 ms window after the stimulus onset (Liu et al., 2015). Using this binarized FRA (bFRA), CRFMUA and CRFLFP were defined as the proportion of active sites for a given stimulus, at the levels of MUA and LFP, respectively.
3 Results
3.1 Behavioral signature of hearing loss, hyperacusis, and tinnitus
In our behavioral experiments, based on PPI and GPI measurements taken before and after the traumatic noise exposure were used to interpret changes in hearing loss, tinnitus, and hyperacusis. A decrease in PPI, a decrease in GPI and an increase in PPI after noise exposure were considered indicators of hearing loss, tinnitus, and hyperacusis, respectively. After 1-h exposure to a 125-dB SPL, 10-kHz tone, a significant decrease in PPI to broadband noise was observed (pre- vs. post-exposure, p = 0.0379, two-tailed Mann–Whitney U test), indicating the presence of noise-induced hearing loss (Figure 1A). Although no significant changes were observed in PPI responses to individual pure tones, the difference in tone PPI (ΔPPI) between the pre- and post-noise exposure time points displayed a U-shaped pattern, with PPI decreases particularly evident in the 8–16 kHz range, suggesting a distinct hearing in this frequency range (Figure 1B). Conversely, PPI values at 4 kHz and 32 kHz occasionally increased after noise exposure, implying that the noise-exposed animals became more sensitive to these specific tones than their unexposed counterparts. However, these signs of hyperacusis varied widely across subjects and did not reach statistical confirmation. The difference in GPI (ΔGPI) between pre- and post-exposure tended to decrease with the test frequency, and post-exposure GPI was marginally significantly smaller than pre-exposure GPI at 32 kHz (two-tailed Mann–Whitney U test, p = 0.0499; Figures 1C,D). This trend suggested that the acoustic trauma induced tinnitus, particularly in the high-frequency range. No significant correlation was observed between ΔPPI and ΔGPI (Supplementary Figure S1; R = 0.231; t-test, p = 0.238). Notably, the variance of ΔPPI tended to be larger than that of ΔGPI (F-test, p = 0.0458), indicating that hyperacusis symptoms exhibited more substantial variations across subjects compared to tinnitus symptoms.
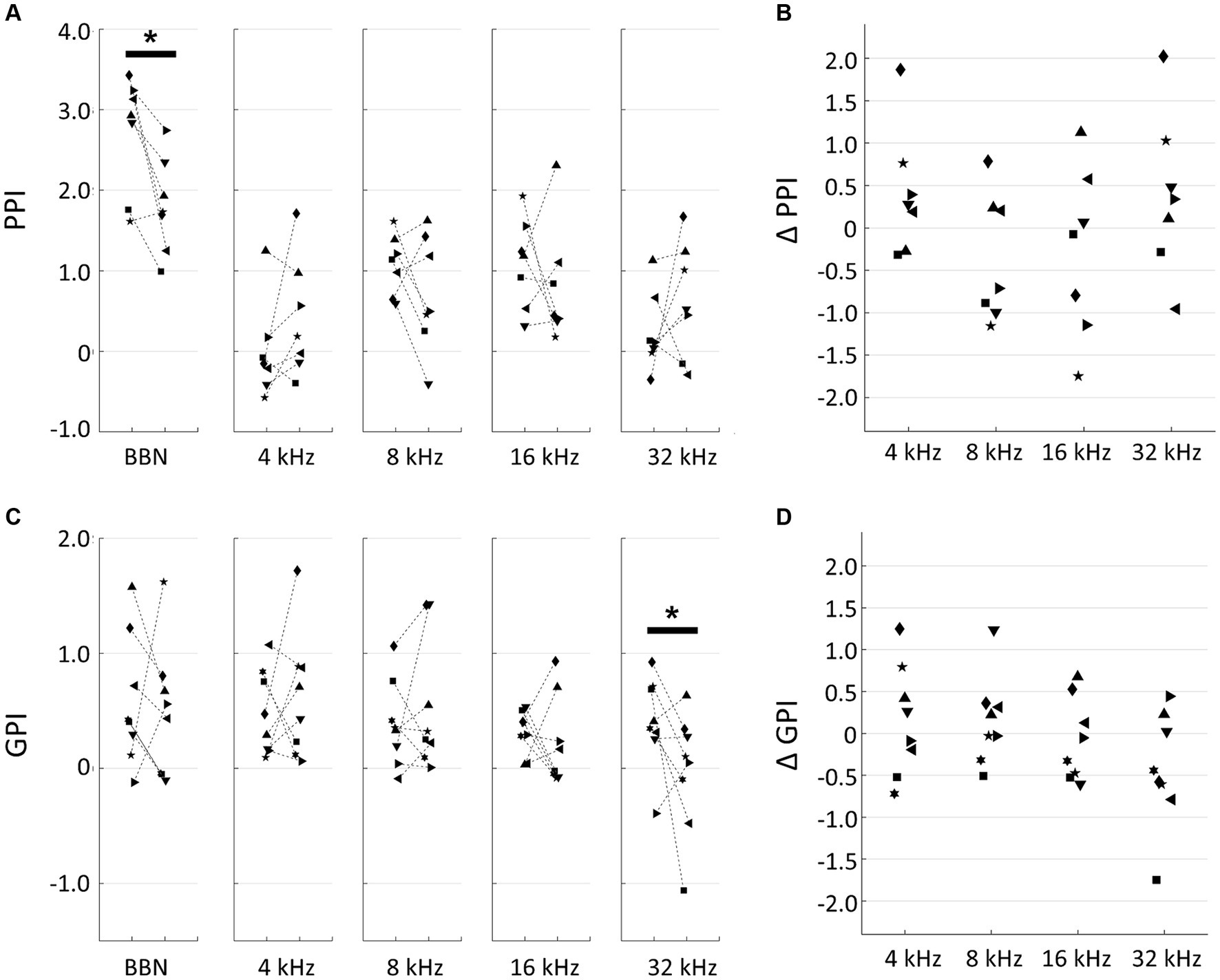
Figure 1. Behavioral experiments. (A) Prepulse inhibition (PPI) of pre- and post-exposure conditions. Broadband noise (BBN) and tones with indicated frequencies were used for prepulse. Each symbol indicates a different animal. Asterisks represent statistical differences between groups (p < 0.05, Mann–Whitney U test). (B) PPI differences (ΔPPI) between pre- and post-treatment of acoustic trauma. (C) Gap-inhibition (GPI) of pre- and post-exposure conditions. (D) GPI differences (ΔGPI) between pre- and post-exposure.
3.2 Map disorganization
In the neural activity characterization, recording sites with CFs were considered to be within the auditory cortex and were used in subsequent analyses. Figure 2A displays representative CF maps in both the control and noise-exposed groups (see also Figure 3A for the post-stimulus histogram of MUA for each test stimulus, which was used to determine CF). Consistent with previous studies (Takahashi et al., 2005a, 2011; Funamizu et al., 2013; Shiramatsu et al., 2016), the auditory cortex exhibited organization into several auditory fields. The primary auditory cortex (A1) and the anterior auditory field (AAF) were characterized by short post-stimulus latency and showed a mirror image of distinct tonotopic gradients: A1 displayed a posterior-to-anterior gradient of low-to-high frequency in the dorsoposterior region, while AAF exhibited a high-to-low-frequency gradient in the ventro-anterior region (Figure 2A, control). Other auditory fields showed longer latency than A1 and AAF and displayed tonotopic discontinuity in relation to the main tonotopic axes in A1 and AAF.
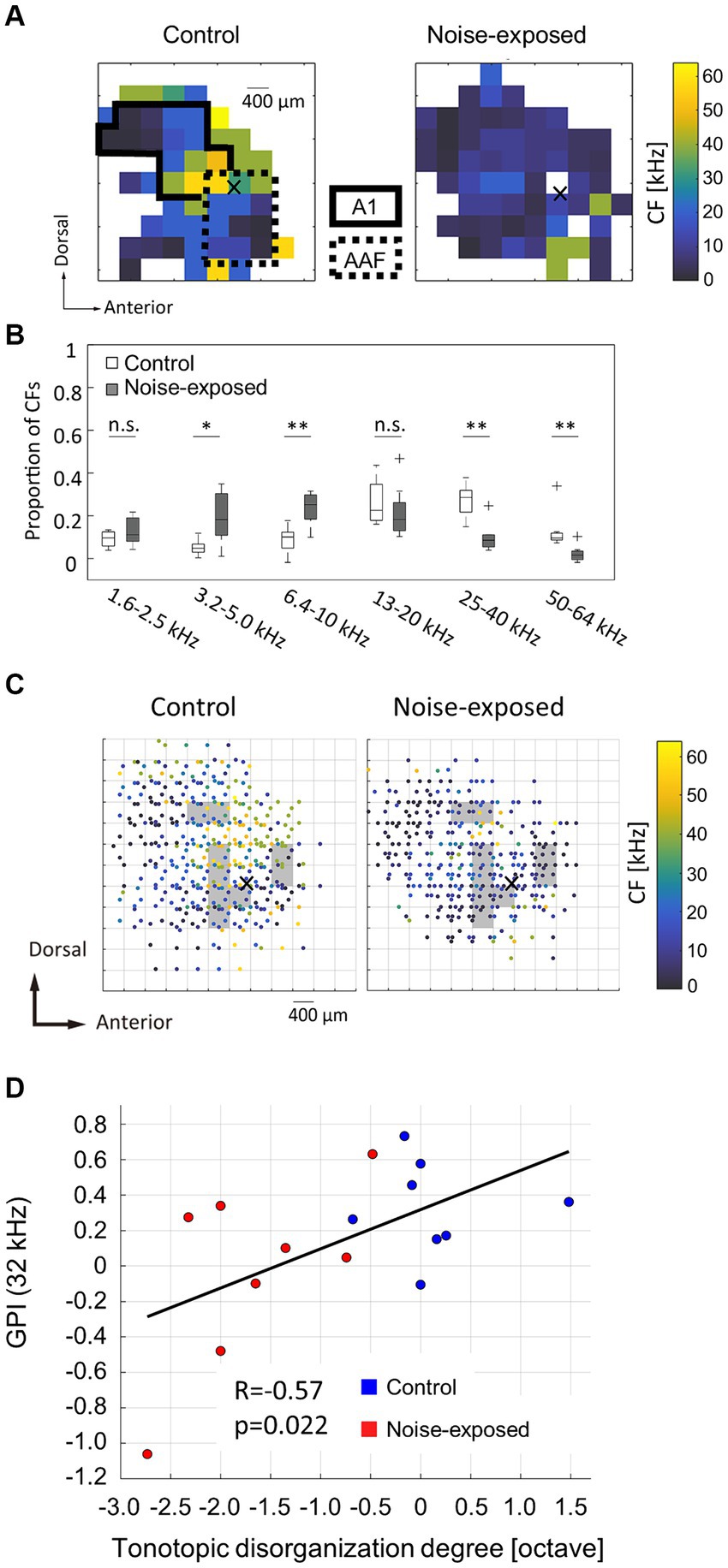
Figure 2. Acoustic trauma-induced disorganization represented in a tonotopic map. (A) Representative maps of characteristic frequency (CF) in the auditory cortex. Cross marks represent the activation focus of click-evoked LFP, which is used as a reference point to pool CF maps across animals. (B) Proportion of CFs in the control and exposed groups. Asterisks represent statistical difference between groups (*p < 0.05; **p < 0.01, Mann–Whitney U test). (C) Pooled CF maps. Statistical significances between the control and exposed groups were observed in shaded regions (p < 0.05). (D) Correlation between the tonotopic disorganization degree and behavioral metrics of tinnitus (GPI).
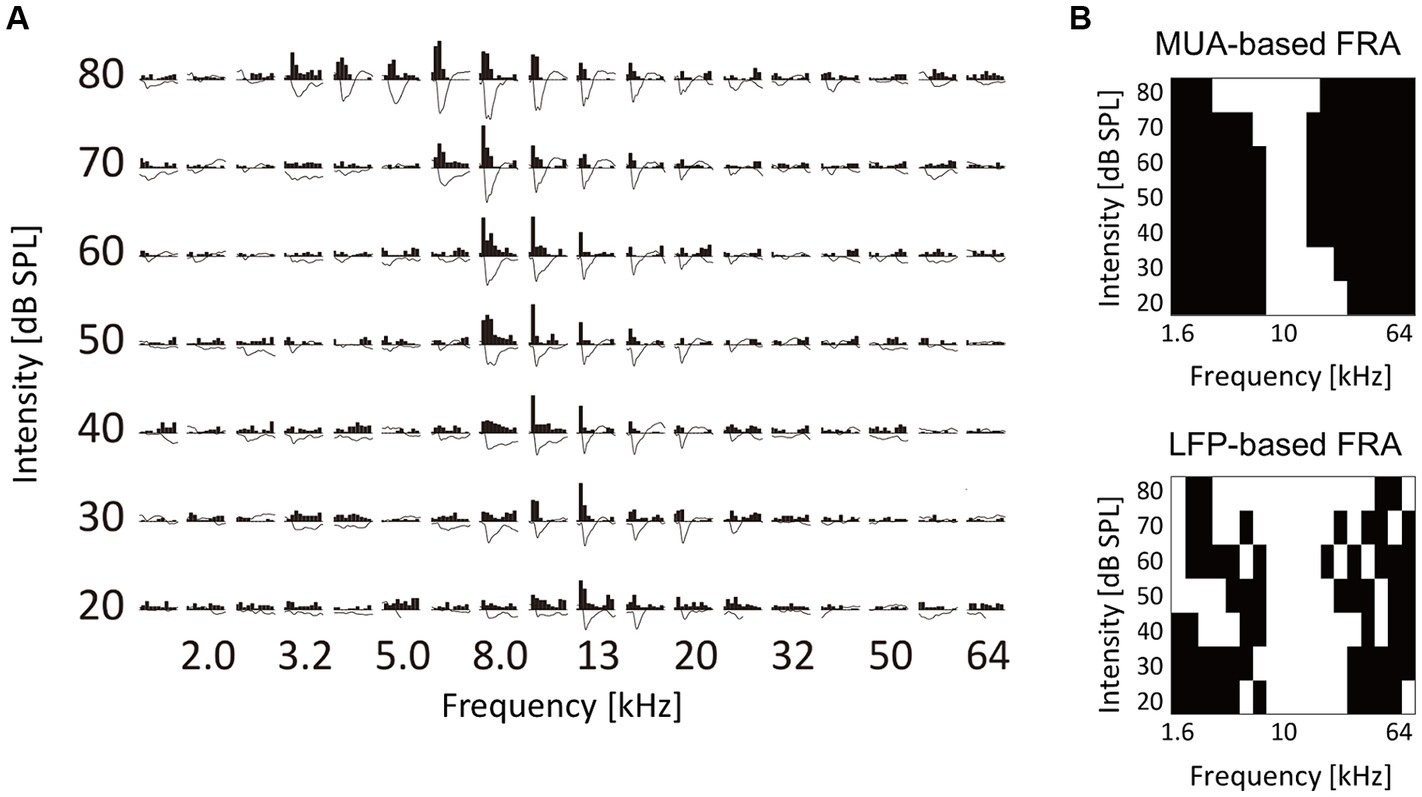
Figure 3. Characterization of tone-evoked neural activity. (A) Data from a representative recording site. Post-stimulus time histograms of MUA and stimulus-evoked LFP traces in response to test tones with different frequency and intensity are shown. MUA and LFP within the first 100 ms post-stimulus latency were characterized. (B) Binarized frequency response area (bFRA) of MUA and LFP. White colors represent activity (1) while black colors represent inactivity (0).
The noise-exposed group exhibited a tendency to have fewer high-frequency regions (25–64 kHz) than those in the control group (Figure 2A, exposed). This trend was further substantiated in the combined data analysis (Figure 2B), where the proportion of recording sites with high CF significantly decreased (25–40 kHz, p = 0.00109; 50–64 kHz, p = 0.00264; two-tailed Mann–Whitney U test). In contrast, the proportion of recording sites with low-frequency CF significantly increased in the noise-exposed group (3.2–5.0 kHz: p = 0.0162; 6.4–10 kHz: p = 0.00295). Conversely, no significant difference was observed in the total number of recording sites with CF between the control and the noise-exposed groups (49.9 ± 10.6 vs. 40.8 ± 10.1; p = 0.0991, two-tailed Mann–Whitney U test).
To assess acoustic trauma-induced disorganization of CF maps, CF maps from multiple animals were aligned and pooled using a reference point to create a group CF map (Figure 2C). In accordance with our previous studies (Takahashi et al., 2005a, 2011; Funamizu et al., 2013), the reference point was determined at the activation focus of click-evoked LFPs by employing a quintic polynomial surface approximation for each animal (Figures 2A,C, indicated by cross marks).
To identify the areas affected by noise exposure, CF maps were pooled on 400-μm grids and the intergroup differences were examined. Within each grid, corresponding to each square measuring 400 × 400 μm2, CFs values were gathered and compared between the groups. Significantly different CF values between the groups (two-tailed Mann–Whitney U test, p < 0.05) were typically observed within CF regions ranging from 10 and 30 kHz (Figure 2C, shaded areas), possibly corresponding to the “edge frequency” of the exposed tones. These shaded areas were regarded as the affected region.
To determine whether tonotopic disorganization within the auditory cortex is indicative of tinnitus symptoms (Muhlnickel et al., 1998; Adjamian et al., 2009; Engineer et al., 2011) or not (Langers et al., 2012; Elgoyhen et al., 2015; Koops et al., 2020), the correlation between the tonotopic disorganization degree and the behavioral index of tinnitus was explored. The tonotopic disorganization degree in each animal was defined as the deviation from the pooled CF map of the control group. In this evaluation, each animal’s CF within the affected region was compared to the median CF within a 200-μm radius of the corresponding test site on the pooled CF map of the control group. The median of these CF differences, expressed in octaves, was used to establish the degree of tonotopic disorganization for each animal, which was then plotted against the post-exposure GPIs at 32 kHz, serving as behavioral index of tinnitus at 32 kHz (Figure 2D). The analysis revealed a significant correlation between the degree of tonotopic disorganization and the behavioral GPI index (R = 0.5679; t-test, p = 0.0217). In contrast, no significant correlation was observed between the tonotopic disorganization degree and the behavioral metrics of hyperacusis (PPI or ΔPPI) at any prepulse frequency (Supplementary Figure S2). These results indicate that a pronounced disorganization of the tonotopic map is associated with severe tinnitus symptoms, but not with hyperacusis.
3.3 Cortical recruitment and neural gain
The examination focused on how the fourth layer of the auditory cortex was recruited for the neural representation of tones at the levels of MUA and LFP. These characteristics were analyzed for each test tone with varying frequencies and SPL (Figure 3A). For each recording site, FRA values were calculated for MUA and LFP and then converted into binary values to classify whether a given recording site was active (1) or inactive (0) in response to each test stimulus (Figure 3B). The threshold for binarizing FRA of MUA was determined as the inflection point of a smoothed z-score histogram of MUA (Guo et al., 2012; Noda and Takahashi, 2015). The threshold for the bFRA of LFP was set at 1.2 times the baseline fluctuations within 400–500 ms after the stimulus onset (Liu et al., 2015). Subsequently, by averaging bFRA across recording sites, the population bFRAs, or the CRF (Kilgard and Merzenich, 1998; Takahashi et al., 2011), were obtained for both MUA and LFP. These CRFs served as a measure of the activation level of the entire auditory cortex.
Figure 4 presents a comparison of group averages of CRFs at the MUA and LFP levels, denoted as CRFMUA and CRFLFP, between the control and noise-exposed groups. The control auditory cortex demonstrated the highest recruitment in response to tones around 16 kHz (Figure 4A, CRFMUA), whereas the auditory cortex in the noise-exposed group exhibited the largest recruitment to tones around 5 kHz and reduced recruitment to high-frequency tones compared to the control cortex (Figure 4B, CRFMUA). Group comparison revealed significant effects of acoustic trauma: increased recruitment to low-frequency, high-SPL tones in CRFMUA (white asterisks in Figure 4B; p < 0.05, separate two-tailed Mann–Whitney U tests for each frequency and SPL level without multiple comparison adjustments) and decreased recruitment to high-frequency tones in CRFMUA and CRFLFP (black asterisks). Thus, the increase in CRF, a potential indicator of hyperacusis, was observed at the MUA level but not at the LFP level, suggesting that intracellular amplification from LFP to MUA at the thalamocortical recipient layer plays a critical role in hyperacusis. The acoustic trauma-induced CRF increases at moderate SPL in our results align with clinical observations that patients with hyperacusis perceive moderate-intensity sounds as intolerably loud, aversive, or painful (Anari et al., 1999; Baguley, 2003; Auerbach et al., 2014; Pienkowski, 2017, 2019; Eggermont, 2017a,b; Auerbach et al., 2019).
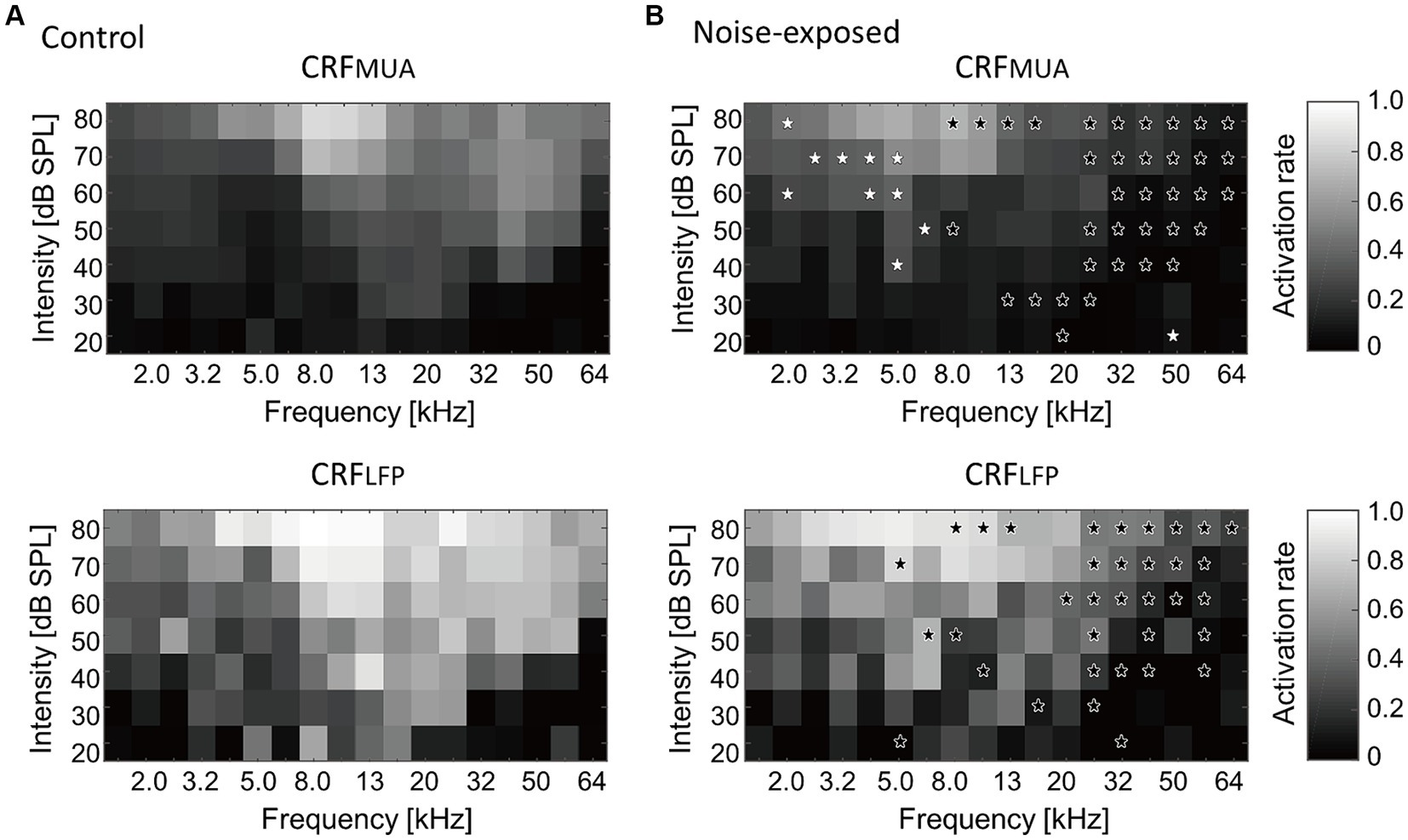
Figure 4. Cortical recruitment functions (CRFs) at the level of MUA and LFP (i.e., CRFMUA and CRFLFP) in the control (A) and the exposed (B) groups. Black asterisks indicate decreased recruitment after the noise exposure, while white asterisks indicate increased recruitment (p < 0.05, separate two-tailed Mann–Whitney U tests for each frequency and SPL level without multiple comparison adjustments).
To address the hypothesis that the above reorganizations of the map and CRF were associated with weakened lateral inhibition, which makes growth function of evoked activities against test intensity monotonic, the monotonicity index (MI) for each individual recording site was examined (Figure 5A). The MI was defined as the ratio of the firing rate at the loudest SPL used (i.e., 80 dB SPL; red rectangles in Figure 5A) to the maximum firing rate at the optimal SPL (i.e., blue rectangles in Figure 5A) (Zhou and Wang, 2010). MI takes a value ranging from 0 to 1, with higher values indicating greater monotonicity. Figure 5B characterizes MIs in either low (1.6–5.0 kHz), middle (6.4–20 kHz), or high (25–64 kHz) CF sites. MIs at the middle frequency region tended to show decreased proportion of moderately nonmonotonic neurons after noise exposure (Figure 5C; 0.5 < MI < 1.0; Mann–Whitney U test, p = 0.0531), exhibiting significant differences in distribution between pre- and post-exposure groups (Kolmogorov–Smirnov test: p = 8.3e-04), suggesting that the lateral inhibition in the middle frequency weakened after the traumatic noise exposure. This weakened lateral inhibition may underlie the increased neural recruitment to low-frequency tones, which may be associated with hyperacusis symptoms.
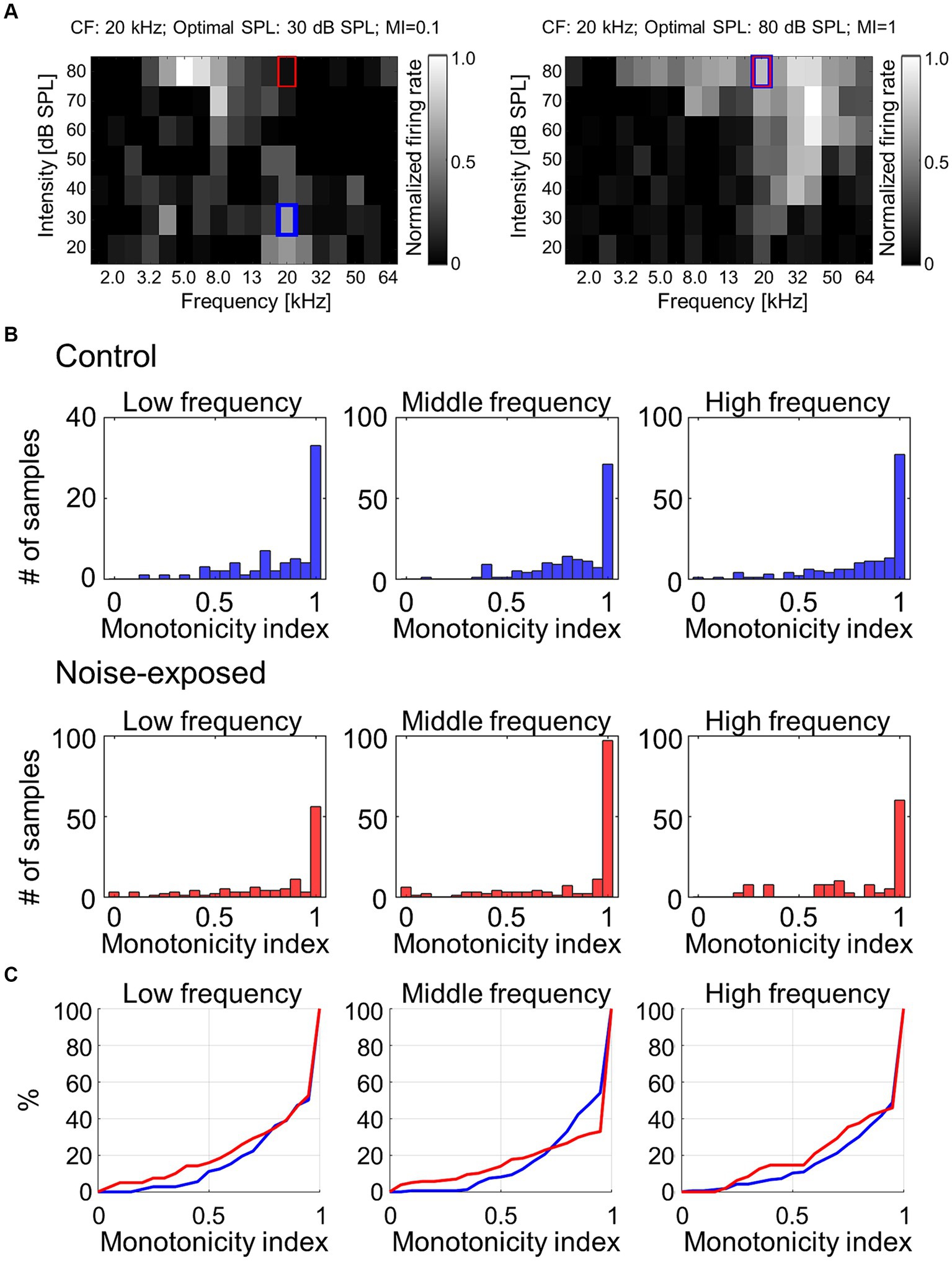
Figure 5. Monotonicity index (MI) of MUA. (A) Two examples of MI calculated as the ratio of the firing rate at the loudest SPL used (i.e., 80 dB SPL; red rectangles) to the maximum firing rate at the optimal SPL (i.e., blue rectangles). (B) Histograms of MI at low-, mid-, and high-frequency sites in the control and exposed groups. (C) Cumulative distribution of MI.
The increased recruitment at the level of MUA was hypothesized to contribute to hearing sensitivity in the noise-exposed animals and that the neurophysiological measure of CRFMUA could predict the behavioral measure of hyperacusis, including ΔPPI at 4, 8, 16, and 32 kHz tones. To calculate CRFMUA of each noise-exposed animal, the deviation from the group average of CRFMUA in control animals was quantified and defined as ΔCRFMUA. At 4, 8, 16, and 32 kHz, the median of ΔCRFMUA among 60–80 dB SPLs, or ΔCRFMUA 60–80 dB SPL was quantified, since hyperacusis was commonly observed at moderate to high-SPL tones (Chen et al., 2014, 2015; Radziwon et al., 2019). Consequently, ΔCRFMUA 60–80 dB SPL exhibited a significant positive correlation with ΔPPI (Figure 6; R = −0.39; t-test, p = 0.038; y = 1.56x + 0.26), supporting our hypothesis. ΔCRFMUA 60–80 dB SPL did not exhibited a significant correlation with the behavioral symptoms of tinnitus (ΔGPI at 32 kHz; Supplementary Figure S3; R = −0.3454, p = 0.4480), suggesting that ΔCRFMUA 60–80 dB SPL is neural correlates of hyperacusis, but not those of tinnitus.
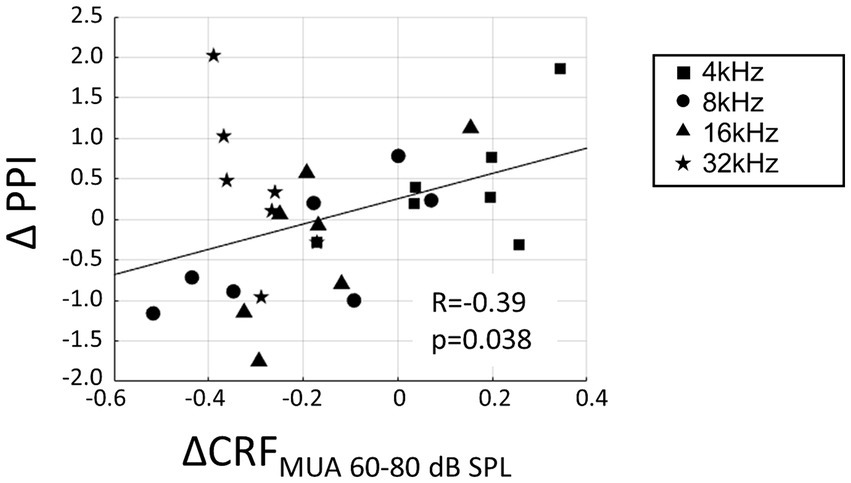
Figure 6. Correlation between hyperacusis symptoms (ΔPPI) and acoustic trauma-induced increase of CRFMUA at moderate SPL (ΔCRFMUA 60–80 dB SPL). ΔPPI plotted against ΔCRFMUA 60–80 dB SPL for tones at 4, 8, 16, and 32 kHz in the exposed group. A linear regression line is presented (y = 1.56x + 0.26).
As a measure of neural gain from LFP to MUA, the magnitude of MUA relative to LFP in the auditory cortex was characterized. Both LFP and MUA were normalized to each tone at each electrode concerning the maximum LFP and MUA (Figure 7A), resulting in normalized LFP and MUA values ranging between 0 and 1. Then, for each animal, the ratio of MUA to LFP (MUA/LFP) for a tone frequency (f [kHz]) and SPL [dB SPL] was quantified as follows in Equation (2):
where ‘mean’ represented the average across electrodes that were active in the bFRA of LFP (Figure 7B). Figure 7C illustrates MUA/LFP as a function of SPL by averaging MUA/LFP(f, SPL) across frequencies for each animal. While MUA/LFP decreased with SPL both the control and noise-exposed groups, indicating that the gain from LFP to MUA was high for low-SPL tones, this tendency was weaker in the noise-exposed group, especially below 60 dB SPL (Figure 7C; p = 0.048 for 30 dB SPL and p = 0.0499 for 40 dB SPL; Mann–Whitney U test without multiple-testing correction), suggesting that the dynamic range of the gain had narrowed in the noise-exposed group.
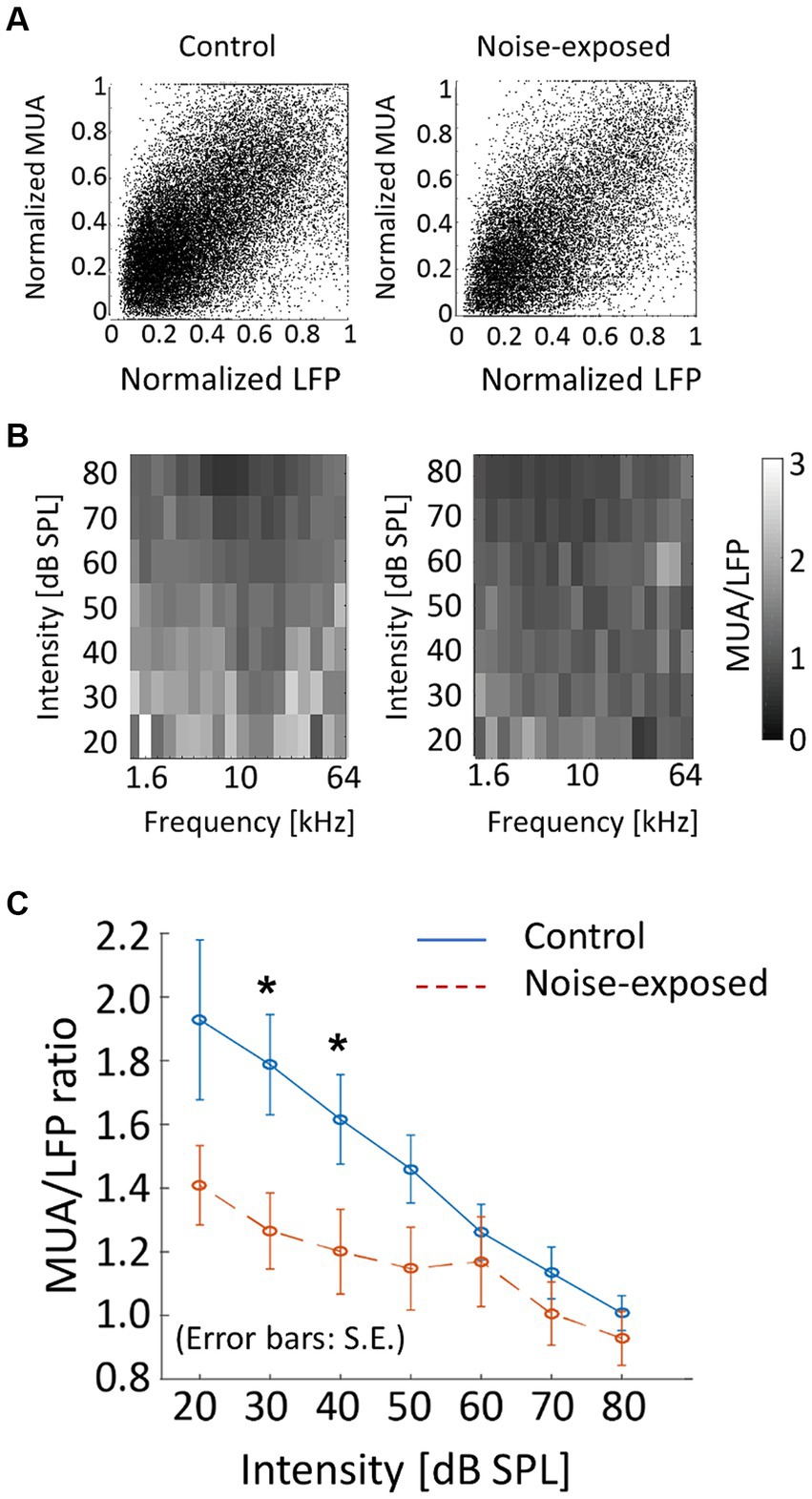
Figure 7. Neural gain from LFP to MUA (MUA/LFP) in the control and exposed groups. (A) Normalized MUA was plotted against normalized LFP. Each dot indicates a neural response to a tone. (B) MUA/LFP for each frequency-intensity condition. When the bFRA of LFP were inactive, MUA/LFP ratio was considered zero. (C) MUA/LFP as a function of intensity. Plots and error bars represent the average and errors. Asterisks represent statistical difference between groups (p < 0.05, Mann–Whitney U test).
4 Discussion
We initially confirmed, through behavioral experiments, that rats exhibited different characteristics of PPI and GPI indices after being exposed to a traumatic 10-kHz tone. This observation suggests that the putative hyperacusis frequency differs from the tinnitus frequency. Although not reaching sufficient statistical levels due to wide variance across subjects, the U-shaped profile of ΔPPI indicated that the hearing impairment frequency was approximately between 8 and 16 kHz, while the possible hyperacusis frequency lay either below or above this hearing impairment frequency. Additionally, a reduction in GPI indicated that the subject had tinnitus in the high-frequency range, specifically approximately 32 kHz. Secondly, auditory cortex mapping revealed a significant correlation between GPI, a behavioral measure of tinnitus symptoms, and the extent of tonotopic map disorganization. Thirdly, ΔPPI, a behavioral index of hyperacusis symptoms, showed a correlation with the recruitment function at the MUA level in response to moderate- and high-SPL tones. However, this correlation was not observed at the LFP level. This suggests that hyperacusis was most prominent with high-SPL, low-frequency tones. The enhancement of MUA recruitment function was likely a result of increased gains in thalamocortical transmission, where LFP were considered as inputs and MUA as outputs. This gain modification was associated with the loss of monotonicity, a phenomenon in which inhibitory synapses played a crucial role.
4.1 Comparison with human studies
Our behavioral and electrophysiological findings suggest that exposure to a traumatic 10-kHz tone results in hearing loss with the 8–16 kHz range, tinnitus at a high frequency of approximately 32 kHz, and hyperacusis in response to moderate- and high-SPL tones at frequencies below 8 kHz. These findings align with human studies, where tinnitus pitch was most frequently observed at or above the frequency of the noise exposure (Atherley et al., 2005; Loeb and Smith, 2005; Sereda et al., 2011). However, it is worth noting that tinnitus frequencies have varied in previous animal models, sometimes falling below the noise exposure frequency (Turner et al., 2006; Engineer et al., 2011) or above it (Wang et al., 2009; Holt et al., 2010; Longenecker and Galazyuk, 2011; Turner et al., 2012; Li et al., 2013).
Nonetheless, our work may not be directly comparable to clinical studies. Firstly, human subjects with hyperacusis have shown reduced cortical activation in response to tones at the tinnitus frequency compared to those without hyperacusis (Koops and van Dijk, 2021). In our animal models, we did not observe a consistent trend between the putative tinnitus and hyperacusis frequency ranges. Secondly, the allocation of attention, wherein increased attention to hyperacusis frequency reduces attention to the tinnitus frequency, has been proposed as an underlying mechanism in the interaction between tinnitus and hyperacusis (Krumbholz et al., 2007; Paltoglou et al., 2011). However, our present study suggests an attention-free mechanism for tinnitus and hyperacusis. Our tonotopic map and cortical recruitment function, observed under anesthesia, demonstrated that acoustic trauma selectively reduced activation in high-frequency regions, including the tinnitus frequency, and conversely, induced hyperactivation in low-frequency regions at moderate and high intensities. Nevertheless, it is important to consider that acoustic startle responses are regulated by the reticular formation, i.e., the arousal system, which receives inputs from the auditory system and the amygdala (Kandler and Herbert, 1991; Koch et al., 1992; Carlson and Willott, 1998; Paus, 2000). Furthermore, the stress experienced by rats while being held in a confined chamber during GPI and PPI measurements may also affect their startle behavior (Eggermont, 2017a,b; Guercio et al., 2019). These emotional and conscious experiences should be taken into account. Therefore, further investigations are still required to validate our methods as translational studies of tinnitus and hyperacusis.
Clinically, noise-induced tinnitus can be acute or chronic. Acute tinnitus may last from a few minutes to several weeks after noise exposure (Snow, 2004; Han et al., 2009), while tinnitus that persists for several months is considered chronic (Mazurek et al., 2022) and tinnitus that persists for years is considered permanent and irreversible (Snow, 2004). Animal studies also showed that early signs of tinnitus and hyperacusis were sometimes reversible, while chronic signs developed over weeks (Turner et al., 2012; Hayes et al., 2014). Specifically, temporal elevation of auditory thresholds up to 30 dB was typically observed in the acute phase (Kujawa and Liberman, 2009; Middleton et al., 2011; Turner et al., 2012), which might be observed as the decreased PPI for BBN (Figure 1A) and have confounding effects in PPI and GPI interpretation. Therefore, our behavioral and electrophysiological experiments conducted shortly after noise exposure (2–8 days) are limited to the acute effects of noise exposure. Further studies are still required to investigate the neural correlates of chronic tinnitus and hyperacusis.
4.2 Neural correlates
Conceptual models of tinnitus and hyperacusis have been developed based on pioneering functional imaging studies in humans (Llinas et al., 1999; Weisz et al., 2005; Auer, 2008; Gu et al., 2010; Moazami-Goudarzi et al., 2010; Leaver et al., 2012; Maudoux et al., 2012; Husain and Schmidt, 2014). These studies have identified increased central gain, aberrant functional connectivity, and abnormal oscillations as the neural correlates (Weisz et al., 2007; Sereda et al., 2011; Henry et al., 2014). In addition to the group-level correlations between neural hyperactivity and hyperacusis-like behavior (Sun et al., 2009; Chen et al., 2014; Hickox and Liberman, 2014), we sought to demonstrate that examining inter-subject variability provides more robust evidence. This approach helps to illustrate how the loudness growth measured behaviorally is correlated with gain enhancement in the auditory cortex (Auerbach et al., 2019).
Our data suggests that tinnitus is linked to the disorganization of the tonotopic map. Tonotopic map disorganizations in subjects with tinnitus has been previously reported in humans (Muhlnickel et al., 1998; Adjamian et al., 2009) and in animals (Engineer et al., 2011), although these findings have remained controversial (Langers et al., 2012; Elgoyhen et al., 2015; Koops et al., 2020). We believe that these discrepancies arise because human studies on tonotopic mapping often used moderate-intensity tones to evoke distinct cortical activation, while in animal studies, CFs in tonotopic maps were defined based on the lowest intensity tones that activated the test neuron. Our data align with previous research indicating that the hyperactivity induced by salicylate and trauma in the auditory cortex is associated with a FRA shift toward the mid-frequency region (Norena et al., 2010; Stolzberg et al., 2011; Chen et al., 2012, 2013, 2014). The central noise hypothesis of tinnitus is consistent with the disorganization of the tonotopic map characterized with low-intensity tones, which evoke responses with relatively low S/N ratios. In this context, map plasticity characterized by low-intensity tones may be the potential neural correlates of tinnitus but not hyperacusis.
The perceived loudness depends on both the intensity and the bandwidth of the test stimulus, which is related to the number of activated frequency channels (Zwicker et al., 1957; Hawley et al., 2005; Gu et al., 2010). Therefore, the recruitment function, which quantifies the number of activated neurons in response to a given test tone, serves as a suitable predictor of how loudly the test tone is perceived. Neural recruitment typically corresponds to the activation of a neural population. Activation of the primary auditory cortex increases with intensity in both individuals with normal and impaired hearing (Hall et al., 2001; Langers et al., 2007; Behler and Uppenkamp, 2016). Likewise, hyperacusis, in both human and animals, is commonly associated with hyperactivity in higher-order subcortical nuclei and the auditory cortex (Harms and Melcher, 2002; Sigalovsky and Melcher, 2006; Gu et al., 2010; Knipper et al., 2013; Ruttiger et al., 2013; Zeng, 2013; Chen et al., 2015; Auerbach et al., 2019), rather than with broadening of cortical tuning (Koops and van Dijk, 2021). This hyperactivity has been observed over a wide range of test frequencies beyond regions of hearing loss (Noreña and Chery-Croze, 2007; Diehl and Schaette, 2015; Sheldrake et al., 2015). Additionally, earplugging and acoustic enhancements also induce adaptive gain control mechanisms across frequency channels (Formby et al., 2003; Noreña and Chery-Croze, 2007; Munro et al., 2014).
Our results indicate that hearing loss led to reduced recruitment of neural populations in response to high-frequency tones, but increased recruitment in response to high-SPL, low-frequency tones, specifically at the MUA level, but not at the LFP level (Figure 4). Since the first negative deflection of LFP reflects synaptic inputs to the thalamocortical layer (Einevoll et al., 2013; Mazzoni et al., 2015; Haider et al., 2016), while MUA reflects outputs resulting from nonlinear intracellular processing in the cortex, our findings suggest that the neural correlate of hyperacusis is associated with the output rather than the input of the thalamocortical input layer. Consequently, hyperacusis was likely to manifest in response to high-SPL, low-frequency tones, which is consistent with previous research indicating that sound-evoked hyperactivity is not limited to frequencies affected by hearing loss in patients with hyperacusis (Koops and van Dijk, 2021) and in animal models (McGill et al., 2022). Figure 6 also supported our hypothesis in that the sign of hyperacusis (ΔPPI) was correlated with increased recruitment of MUA to high SPL tones (ΔCRFMUA 60–80 dB SPL); however, this was true for 4–16 kHz tones, but not for 32 kHz tone. Map plasticity was also frequency dependent, increasing in low CF regions and decreasing in high CF regions (Figure 2). These data suggest that additional CF-dependent mechanisms, which cannot be captured by ΔCRFMUA 60–80 dB SPL, underlie the compensatory gain control and behavioral symptom of hyperacusis.
We showed that comparison of CRF between LFP and MUA served as a possible measure to characterize how efficiently the thalamo-cortical synaptic inputs (LFP) were converted into cortical discharges (MUA), i.e., the neural gain from the LFP to MUA. Thalamocortical transmissions in the noise-exposed group were characterized by narrower dynamic ranges of input/output ratios compared to those in the control group (Figure 7). These results align with previous studies demonstrating that cochlear damage reduces sound-evoked neural responses at the auditory nerve (Wake et al., 1993; Heinz and Young, 2004; Heinz et al., 2005), leading to an enhanced gain, characterized by a steep increase in neural response with sound intensity, at the level of the auditory cortex (Popelar et al., 1987; Syka et al., 1994; Qiu et al., 2000; Norena et al., 2003; Seki and Eggermont, 2003; Chambers et al., 2016; Jiang et al., 2017; Resnik and Polley, 2017; Asokan et al., 2018; Resnik and Polley, 2021; McGill et al., 2022; Parameshwarappa et al., 2022).
Our analyses were made possible because both auditory-evoked LFP and MUA were distinct at layer 4 in the auditory cortex. However, we cannot definitively conclude that our findings are specific to the thalamocortical layer. Similar analyses could be employed to investigate whether and how the gain from synaptic inputs to neuronal discharges varies among auditory subcortical nuclei and different layers of the auditory cortex following hearing loss. In previous studies, tinnitus and hyperacusis have been linked to increased auditory-evoked LFP and fMRI responses in the IC, MGB and A1. These findings were considered evidence of central gain enhancement following hearing loss, possibly through homeostatic plasticity (Salvi et al., 1990; Qiu et al., 2000; Gu et al., 2010; Auerbach et al., 2014; Chen et al., 2015). Central noise, thought to underlie tinnitus but not hyperacusis, may also increase with central gain in some models (Norena, 2011) but not in others (Zeng, 2013). Due to these discrepancies, hyperacusis may not always be associated with tinnitus (Baguley, 2003). The hyperactivity in hyperacusis gradually develops through the auditory brainstem pathway in a sequential manner and is most consistently observed at the level of the auditory cortex (Qiu et al., 2000; Schaette and McAlpine, 2011; Chen et al., 2015). These cumulative effects may result from nonlinear processing from LFP to MUA at each nucleus, as demonstrated at the level of the thalamocortical recipient layer in our current study.
4.3 Neural mechanisms
The gain control mechanisms underlying tinnitus and hyperacusis are likely triggered by partial impairment of the peripheral auditory pathway. For instance, rats exhibited clearer evidence of tinnitus after exposure to noise at 110 dB SPL compared to exposure at 116 dB SPL or higher (Turner and Larsen, 2016). In mice, spontaneous activities in the IC increased more following a 2-h noise exposure at 100 dB SPL than at 105 dB SPL. In our study, we utilized a unilateral hearing loss model, known for its effectiveness in inducing tinnitus and hyperacusis (Isaacson and Vora, 2003; Jahn and Polley, 2023). This model may be accompanied by neural plasticity at various auditory processing centers, including the cochlear nucleus (Rubio, 2006), lateral superior olive (Kotak and Sanes, 1995), IC (Vale and Sanes, 2000), and the auditory cortex (Kotak et al., 2008; Sarro et al., 2008). Severe bilateral hearing loss could deprive the higher-order auditory system of effective gain control.
The changes in gain are associated with increased gene expression of glutamate receptors and decreased expression of GABA receptors in the auditory cortex (Sarro et al., 2008; Balaram et al., 2019). This suggests hypersensitization and disinhibition, respectively. Particularly, parvalbumin-expressing interneurons play a crucial role in triggering the hyperactivity of cortical pyramidal neurons (Resnik and Polley, 2017; Masri et al., 2021; Resnik and Polley, 2021).
We have demonstrated that hearing loss significantly reduces the monotonicity of tone-evoked activities, suggesting that a loss of inhibition underlies the modulation of neural recruitment induced by acoustic trauma. Auditory-evoked potentials in the primary auditory cortex exhibit a linear response to the rate of pressure change (in Pa/s) when stimulated with CF tone but a nonlinear response to non-CF tones. This suggests that inhibition plays a role in the nonlinearity characteristics of loudness perception (Takahashi et al., 2004, 2005b). The loss of inhibition, or disinhibition, is the most likely mechanism behind central gain enhancement (Norena, 2011; Auerbach et al., 2014; Chen et al., 2014). Disinhibition resulting from acoustic trauma aligns with findings that acoustic trauma reduces tuning to CF tones (Scholl and Wehr, 2008) and broadens the FRA in auditory cortex neurons, extending far beyond the CF (Wang et al., 1996; Salvi et al., 2000). This suggests that individual neurons receive a wide range of inhibition. Similarly, high-dose treatment with sodium salicylate induces tinnitus and hyperacusis and enhances gain in the central auditory system (Auerbach et al., 2014; Hayes et al., 2014; Jiang et al., 2017; Auerbach et al., 2019), most notably in the auditory cortex (Yang et al., 2007; Norena et al., 2010; Zhang et al., 2011), along with hyperactivity in non-auditory systems (Chen et al., 2014, 2015, 2016, 2017).
Both noise trauma and salicylate treatment are likely to result in synaptic disinhibition (Milbrandt et al., 2000; Sun et al., 2009; Lu et al., 2011; Wang et al., 2011; Yang et al., 2011). Salicylate suppresses GABA-mediated inhibition and enhances excitability (Xu et al., 2005; Gong et al., 2008), and systemic salicylate application induces hyperactivity in the auditory cortex, leading to symptoms of tinnitus and hyperacusis (Sun et al., 2009; Chen et al., 2012), which can be ameliorated by enhancing GABA-mediated inhibition (Brozoski et al., 2007; Sun et al., 2009; Lu et al., 2011). The compensatory plasticity resulting from the loss of inhibition is already observed at the level of the cochlear nucleus (Salvi et al., 2000; Ngodup et al., 2015; Fang et al., 2016). Our results align with the observation that hyperacusis is often experienced over a broad frequency range, including lower frequencies with normal hearing thresholds (Anari et al., 1999; Noreña and Chery-Croze, 2007; Sheldrake et al., 2015). These properties of hyperacusis are attributed to the loss of lateral inhibition, which has been observed from the region of central hearing loss to lower frequency regions (Auerbach et al., 2014). Without lateral inhibition, frequencies near the lesion edge could become “over-represented” in the central auditory system, leading to the recruitment of excess neurons in the auditory cortex (Eggermont, 2017a,b). Our findings suggest that the negative effects of the loss of lateral inhibition are more pronounced at moderate and high intensities than at lower intensities. It is important to note that the neural activities in our study were characterized under anesthesia, which could have significant effects on neural gain and sound-evoked activities (Thornton and Sharpe, 1998; Szalda and Burkard, 2005; Yang et al., 2007; Noda and Takahashi, 2015; Ros et al., 2017). Since isoflurane increases inhibitory tones, the effects of disinhibition may be more pronounced in awake conditions than those characterized in our study.
Our findings were based on the collective activities of neurons. Imaging with cellular resolution provided additional insights, revealing that changes in gain following acoustic trauma varied among pyramidal neurons at cortical layer 2/3. Specifically, the gain remained stable in pyramidal neurons with low spontaneous activity and nonmonotonic intensity tuning, likely due to strong inhibition (Wu et al., 2006; Tan et al., 2007). Conversely, non-homeostatic gain control was typically observed in neurons with high spontaneous activity and monotonic tuning (McGill et al., 2022). At the synaptic level, the balance between excitatory and inhibitory inputs underlies the frequency tuning of auditory cortical neurons (Wehr and Zador, 2003; Zhang et al., 2003). The loss of inhibition may lead to CF upshifts in low-CF neurons and CF downshifts in high-CF neurons (Wang and Salvi, 2002; Scholl et al., 2008). These observations are consistent with the map plasticity induced by acoustic trauma in the auditory cortex, which includes an expanded representation in the 3.2–6.4 kHz regions and reduced representation in the 25–40 kHz regions.
Tinnitus and hyperacusis are likely to co-occur, possibly because the auditory cortex plays a central role in the tinnitus-hyperacusis network, which includes connections with the amygdala, the reticular formation, the hippocampus, striatum, and the cerebellum (Carlson and Willott, 1998; Paus, 2000; De Ridder et al., 2006; Ulanovsky and Moss, 2008; Gu et al., 2010; Zeng, 2013; Chen et al., 2014; Hayes et al., 2014; Chen et al., 2015; Salvi et al., 2021). This interconnected network may lead to a dissociation between cortical and subcortical neural activities (Boyen et al., 2014). Tinnitus is associated with altered functional networks extending beyond the auditory system (Llinas et al., 1999; Leaver et al., 2012; Husain and Schmidt, 2014; Chen et al., 2015). Specifically, increased functional connectivity between the auditory cortex and the amygdala, along with hyperactivity in the amygdala, are commonly observed in both patients with tinnitus and animal models (Hazell and Jastreboff, 1990; van Veen et al., 1998; Wallhäusser-Franke et al., 2003; Chen et al., 2012; Kim et al., 2012; Chen et al., 2013; Jüris et al., 2013; Chen et al., 2016; Aazh et al., 2018). This plasticity is likely driven by homeostatic plasticity, through which the auditory system overcompensates for the reduced output at the peripheral cochlear level (Norena, 2011; Auerbach et al., 2014).
In conclusion, our study in the auditory cortex has revealed a correlation between the disorganization of the tonotopic map and tinnitus-like symptoms, as well as an association between increased unit activity and hyperacusis. These findings indicate that neural correlates of tinnitus can be identified in population firing responses to low-intensity tones, while those of hyperacusis are linked to high-intensity tones. These results support the central noise hypothesis in tinnitus and the maladaptive gain control hypothesis in hyperacusis. To the best of our knowledge, this study is the first to disentangle the neural correlates of tinnitus and hyperacusis within the auditory cortex. We believe that our research offers a novel perspective on the neural foundations of tinnitus and hyperacusis resulting from noise-induced hearing loss.
Data availability statement
The raw data supporting the conclusions of this article will be made available by the authors, without undue reservation.
Ethics statement
The animal study was approved by the Committee on the Ethics of Animal Experiments at the Research Center for Advanced Science and Technology, The University of Tokyo (RAC170005). The study was conducted in accordance with the local legislation and institutional requirements.
Author contributions
NW: Data curation, Formal analysis, Funding acquisition, Investigation, Methodology, Visualization, Writing – original draft. TS: Funding acquisition, Methodology, Validation, Writing – review & editing. HT: Conceptualization, Funding acquisition, Project administration, Supervision, Writing – review & editing.
Funding
The author(s) declare that financial support was received for the research, authorship, and/or publication of this article. This study was supported by JSPS KAKENHI (23H03023, 23H04336, 23H03465, and 24H01544), JST (JPMJMS2296 and JPMJPR22S8), AMED (JP23dm0307009), the Asahi Glass Foundation, and the Secom Science and Technology Foundation.
Conflict of interest
The authors declare that the research was conducted in the absence of any commercial or financial relationships that could be construed as a potential conflict of interest.
Publisher’s note
All claims expressed in this article are solely those of the authors and do not necessarily represent those of their affiliated organizations, or those of the publisher, the editors and the reviewers. Any product that may be evaluated in this article, or claim that may be made by its manufacturer, is not guaranteed or endorsed by the publisher.
Supplementary material
The Supplementary material for this article can be found online at: https://www.frontiersin.org/articles/10.3389/fnins.2024.1385942/full#supplementary-material
Footnotes
References
Aazh, H., Knipper, M., Danesh, A. A., Cavanna, A. E., Andersson, L., Paulin, J., et al. (2018). Insights from the third international conference on hyperacusis: causes, evaluation, diagnosis, and treatment. Noise Health 20, 162–170. doi: 10.4103/nah.NAH_2_18
Adams, B., Sereda, M., Casey, A., Byrom, P., Stockdale, D., and Hoare, D. J. (2021). A Delphi survey to determine a definition and description of hyperacusis by clinician consensus. Int. J. Audiol. 60, 607–613. doi: 10.1080/14992027.2020.1855370
Adjamian, P., Sereda, M., and Hall, D. A. (2009). The mechanisms of tinnitus: perspectives from human functional neuroimaging. Hear. Res. 253, 15–31. doi: 10.1016/j.heares.2009.04.001
Anari, M., Axelsson, A., Eliasson, A., and Magnusson, L. (1999). Hypersensitivity to sound: questionnaire data, audiometry and classification. Scand. Audiol. 28, 219–230. doi: 10.1080/010503999424653
Asokan, M. M., Williamson, R. S., Hancock, K. E., and Polley, D. B. (2018). Sensory overamplification in layer 5 auditory corticofugal projection neurons following cochlear nerve synaptic damage. Nat. Commun. 9:2468. doi: 10.1038/s41467-018-04852-y
Atherley, G. R. C., Hempstock, T. I., and Noble, W. G. (2005). Study of tinnitus induced temporarily by noise. J. Acoust. Soc. Am. 44, 1503–1506. doi: 10.1121/1.1911288
Auer, D. P. (2008). Spontaneous low-frequency blood oxygenation level-dependent fluctuations and functional connectivity analysis of the ‘resting’brain. Magn. Reson. Imaging 26, 1055–1064. doi: 10.1016/j.mri.2008.05.008
Auerbach, B. D., and Gritton, H. J. (2022). Hearing in complex environments: auditory gain control, attention, and hearing loss. Front. Neurosci. 16:787. doi: 10.3389/fnins.2022.799787
Auerbach, B. D., Radziwon, K., and Salvi, R. (2019). Testing the central gain model: loudness growth correlates with central auditory gain enhancement in a rodent model of Hyperacusis. Neuroscience 407, 93–107. doi: 10.1016/j.neuroscience.2018.09.036
Auerbach, B. D., Rodrigues, P. V., and Salvi, R. J. (2014). Central gain control in tinnitus and Hyperacusis. Front. Neurol. 5:206. doi: 10.3389/fneur.2014.00206
Balaram, P., Hackett, T., and Polley, D. (2019). Synergistic transcriptional changes in AMPA and GABAA receptor genes support compensatory plasticity following unilateral hearing loss. Neuroscience 407, 108–119. doi: 10.1016/j.neuroscience.2018.08.023
Basura, G. J., Koehler, S. D., and Shore, S. E. (2015). Bimodal stimulus timing-dependent plasticity in primary auditory cortex is altered after noise exposure with and without tinnitus. J. Neurophysiol. 114, 3064–3075. doi: 10.1152/jn.00319.2015
Bauer, C. A., Turner, J. G., Caspary, D. M., Myers, K. S., and Brozoski, T. J. (2008). Tinnitus and inferior colliculus activity in chinchillas related to three distinct patterns of cochlear trauma. J. Neurosci. Res. 86, 2564–2578. doi: 10.1002/jnr.21699
Behler, O., and Uppenkamp, S. (2016). “Auditory fMRI of sound intensity and loudness for unilateral stimulation” in Physiology, psychoacoustics and cognition in Normal and impaired hearing. eds. P. VanDijk, D. Baskent, E. Gaudrain, E. DeKleine, A. Wagner, and C. Lanting, 165–174. doi: 10.1007/978-3-319-25474-6_18
Bigras, C., Villatte, B., Duda, V., and Hebert, S. (2023). The electrophysiological markers of hyperacusis: a scoping review. Int. J. Audiol. 62, 489–499. doi: 10.1080/14992027.2022.2070083
Boyen, K., de Kleine, E., van Dijk, P., and Langers, D. R. M. (2014). Tinnitus-related dissociation between cortical and subcortical neural activity in humans with mild to moderate sensorineural hearing loss. Hear. Res. 312, 48–59. doi: 10.1016/j.heares.2014.03.001
Brozoski, T. J., Spires, T. J. D., and Bauer, C. A. (2007). Vigabatrin, a GABA transaminase inhibitor, reversibly eliminates tinnitus in an animal model. J. Assoc. Res. Otolaryngol. 8, 105–118. doi: 10.1007/s10162-006-0067-2
Cai, S., Ma, W.-L. D., and Young, E. D. (2009). Encoding intensity in ventral cochlear nucleus following acoustic trauma: implications for loudness recruitment. J. Assoc. Res. Otolaryngol. 10, 5–22. doi: 10.1007/s10162-008-0142-y
Carlson, S., and Willott, J. F. (1998). Caudal pontine reticular formation of C57BL/6J mice: responses to startle stimuli, inhibition by tones, and plasticity. J. Neurophysiol. 79, 2603–2614. doi: 10.1152/jn.1998.79.5.2603
Cederroth, C. R., Lugo, A., Edvall, N. K., Lazar, A., Lopez-Escamez, J.-A., Bulla, J., et al. (2020). Association between hyperacusis and tinnitus. J. Clin. Med. 9:2412. doi: 10.3390/jcm9082412
Chambers, A. R., Resnik, J., Yuan, Y., Whitton, J. P., Edge, A. S., Liberman, M. C., et al. (2016). Central gain restores auditory processing following near-complete cochlear denervation. Neuron 89, 867–879. doi: 10.1016/j.neuron.2015.12.041
Chen, Y. C., Chen, G. D., Auerbach, B. D., Manohar, S., Radziwon, K., and Salvi, R. (2017). Tinnitus and hyperacusis: contributions of paraflocculus, reticular formation and stress. Hear. Res. 349, 208–222. doi: 10.1016/j.heares.2017.03.005
Chen, Y. C., Li, X. W., Liu, L. J., Wang, J., Lu, C. Q., Yang, M., et al. (2015). Tinnitus and hyperacusis involve hyperactivity and enhanced connectivity in auditory-limbic-arousal-cerebellar network. eLife 4:e06576. doi: 10.7554/eLife.06576
Chen, G. D., Manohar, S., and Salvi, R. (2012). Amygdala hyperactivity and tonotopic shift after salicylate exposure. Brain Res. 1485, 63–76. doi: 10.1016/j.brainres.2012.03.016
Chen, G.-D., Radziwon, K. E., Kashanian, N., Manohar, S., and Salvi, R. (2014). Salicylate-induced auditory perceptual disorders and plastic changes in nonclassical auditory centers in rats. Neural Plast. 2014, 1–18. doi: 10.1155/2014/658741
Chen, G. D., Sheppard, A., and Salvi, R. (2016). Noise trauma induced plastic changes in brain regions outside the classical auditory pathway. Neuroscience 315, 228–245. doi: 10.1016/J.neuroscience.2015.12.005
Chen, G. D., Stolzberg, D., Lobarinas, E., Sun, W., Ding, D. L., and Salvi, R. (2013). Salicylate-induced cochlear impairments, cortical hyperactivity and re-tuning, and tinnitus. Hear. Res. 295, 100–113. doi: 10.1016/j.heares.2012.11.016
Coomber, B., Berger, J. I., Kowalkowski, V. L., Shackleton, T. M., Palmer, A. R., and Wallace, M. N. (2014). Neural changes accompanying tinnitus following unilateral acoustic trauma in the guinea pig. Eur. J. Neurosci. 40, 2427–2441. doi: 10.1111/ejn.12580
Dauman, R., and Bouscau-Faure, F. (2005). Assessment and amelioration of hyperacusis in tinnitus patients. Acta Otolaryngol. 125, 503–509. doi: 10.1080/00016480510027565
De Ridder, D., Fransen, H., Francois, O., Sunaert, S., Kovacs, S., and Van De Heyning, P. (2006). Amygdalohippocampal involvement in tinnitus and auditory memory. Acta Otolaryngol. 126, 50–53. doi: 10.1080/03655230600895580
Diehl, P. U., and Schaette, R. (2015). Abnormal auditory gain in hyperacusis: investigation with a computational model. Front. Neurol. 6:157. doi: 10.3389/fneur.2015.00157
Eggermont, J. J. (2017a). Acquired hearing loss and brain plasticity. Hear. Res. 343, 176–190. doi: 10.1016/j.heares.2016.05.008
Eggermont, J. J. (2017b). Animal models of stress and tinnitus. Tinnitus and Stress: An Interdisciplinary Companion for Healthcare Professionals, 77–94.
Eggermont, J. J., and Roberts, L. E. (2004). The neuroscience of tinnitus. Trends Neurosci. 27, 676–682. doi: 10.1016/j.tins.2004.08.010
Einevoll, G. T., Kayser, C., Logothetis, N. K., and Panzeri, S. (2013). Modelling and analysis of local field potentials for studying the function of cortical circuits. Nat. Rev. Neurosci. 14, 770–785. doi: 10.1038/nrn3599
Elgoyhen, A. B., Langguth, B., De Ridder, D., and Vanneste, S. (2015). Tinnitus: perspectives from human neuroimaging. Nat. Rev. Neurosci. 16, 632–642. doi: 10.1038/nrn4003
Engineer, N. D., Riley, J. R., Seale, J. D., Vrana, W. A., Shetake, J. A., Sudanagunta, S. P., et al. (2011). Reversing pathological neural activity using targeted plasticity. Nature 470, 101–104. doi: 10.1038/nature09656
Fang, L., Fu, Y., and Zhang, T.-Y. (2016). Salicylate-induced hearing loss trigger structural synaptic modifications in the ventral cochlear nucleus of rats via medial olivocochlear (MOC) feedback circuit. Neurochem. Res. 41, 1343–1353. doi: 10.1007/s11064-016-1836-x
Formby, C., Sherlock, L. P., and Gold, S. L. (2003). Adaptive plasticity of loudness induced by chronic attenuation and enhancement of the acoustic background (L). J. Acoust. Soc. Am. 114, 55–58. doi: 10.1121/1.1582860
Funamizu, A., Kanzaki, R., and Takahashi, H. (2013). Pre-attentive, context-specific representation of fear memory in the auditory cortex of rat. PLoS One 8:e63655. doi: 10.1371/journal.pone.0063655
Gong, N., Zhang, M., Zhang, X.-B., Chen, L., Sun, G.-C., and Xu, T.-L. (2008). The aspirin metabolite salicylate enhances neuronal excitation in rat hippocampal CA1 area through reducing GABAergic inhibition. Neuropharmacology 54, 454–463. doi: 10.1016/j.neuropharm.2007.10.017
Gu, J. W., Halpin, C. F., Nam, E.-C., Levine, R. A., and Melcher, J. R. (2010). Tinnitus, diminished sound-level tolerance, and elevated auditory activity in humans with clinically normal hearing sensitivity. J. Neurophysiol. 104, 3361–3370. doi: 10.1152/jn.00226.2010
Guercio, G., Thomas, M., Cisneros-Franco, J., Voss, P., Panizzutti, R., and de Villers-Sidani, E. (2019). Improving cognitive training for schizophrenia using neuroplasticity enhancers: lessons from decades of basic and clinical research. Schizophr. Res. 207, 80–92. doi: 10.1016/j.schres.2018.04.028
Guo, W., Chambers, A. R., Darrow, K. N., Hancock, K. E., Shinn-Cunningham, B. G., and Polley, D. B. (2012). Robustness of cortical topography across fields, laminae, anesthetic states, and neurophysiological signal types. J. Neurosci. 32, 9159–9172. doi: 10.1523/jneurosci.0065-12.2012
Haider, B., Schulz, D. P. A., Häusser, M., and Carandini, M. (2016). Millisecond coupling of local field potentials to synaptic currents in the awake visual cortex. Neuron 90, 35–42. doi: 10.1016/j.neuron.2016.02.034
Hall, D. A., Haggard, M. P., Summerfield, A. Q., Akeroyd, M. A., Palmer, A. R., and Bowtell, R. W. (2001). Functional magnetic resonance imaging measurements of sound-level encoding in the absence of background scanner noise. J. Acoust. Soc. Am. 109, 1559–1570. doi: 10.1121/1.1345697
Han, B. I., Lee, H. W., Kim, T. Y., Lim, J. S., and Shin, K. S. (2009). Tinnitus: characteristics, causes, mechanisms, and treatments. J. Clin. Neurol. 5, 11–19. doi: 10.3988/jcn.2009.5.1.11
Harms, M. P., and Melcher, J. R. (2002). Sound repetition rate in the human auditory pathway: representations in the waveshape and amplitude of fMRI activation. J. Neurophysiol. 88, 1433–1450. doi: 10.1152/jn.2002.88.3.1433
Hawley, M. L., Melcher, J. R., and Fullerton, B. C. (2005). Effects of sound bandwidth on fMRI activation in human auditory brainstem nuclei. Hear. Res. 204, 101–110. doi: 10.1016/j.heares.2005.01.005
Hayes, S. H., Radziwon, K. E., Stolzberg, D. J., and Salvi, R. J. (2014). Behavioral models of tinnitus and Hyperacusis in animals. Front. Neurol. 5:179. doi: 10.3389/fneur.2014.00179
Hazell, J. W. P., and Jastreboff, P. J. (1990). Tinnitus. I: auditory mechanisms: a model for tinnitus and hearing impairment. J. Otolaryngol. 19, 1–5.
Heinz, M. G., Issa, J. B., and Young, E. D. (2005). Auditory-nerve rate responses are inconsistent with common hypotheses for the neural correlates of loudness recruitment. J. Assoc. Res. Otolaryngol. 6, 91–105. doi: 10.1007/s10162-004-5043-0
Heinz, M. G., and Young, E. D. (2004). Response growth with sound level in auditory-nerve fibers after noise-induced hearing loss. J. Neurophysiol. 91, 784–795. doi: 10.1152/jn.00776.2003
Henry, J. A., Roberts, L. E., Caspary, D. M., Theodoroff, S. M., and Salvi, R. J. (2014). Underlying mechanisms of tinnitus: review and clinical implications. J. Am. Acad. Audiol. 25, 005–022. doi: 10.3766/jaaa.25.1.2
Herrmann, B., and Butler, B. E. (2021). Hearing loss and brain plasticity: the hyperactivity phenomenon. Brain Struct. Funct. 226, 2019–2039. doi: 10.1007/s00429-021-02313-9
Hesse, L. L., Bakay, W., Ong, H.-C., Anderson, L., Ashmore, J., McAlpine, D., et al. (2016). Non-monotonic relation between noise exposure severity and neuronal hyperactivity in the auditory midbrain. Front. Neurol. 7:133. doi: 10.3389/fneur.2016.00133
Hickox, A. E., and Liberman, M. C. (2014). Is noise-induced cochlear neuropathy key to the generation of hyperacusis or tinnitus? J. Neurophysiol. 111, 552–564. doi: 10.1152/jn.00184.2013
Hofmeier, B., Wolpert, S., Aldamer, E. S., Walter, M., Thiericke, J., Braun, C., et al. (2018). Reduced sound-evoked and resting-state BOLD fMRI connectivity in tinnitus. Neuroimage Clin 20, 637–649. doi: 10.1016/j.nicl.2018.08.029
Holt, A. G., Bissig, D., Mirza, N., Rajah, G., and Berkowitz, B. (2010). Evidence of key tinnitus-related brain regions documented by a unique combination of manganese-enhanced MRI and acoustic startle reflex testing. PLoS One 5:e14260. doi: 10.1371/journal.pone.0014260
Husain, F. T., and Schmidt, S. A. (2014). Using resting state functional connectivity to unravel networks of tinnitus. Hear. Res. 307, 153–162. doi: 10.1016/j.heares.2013.07.010
Hwang, J.-H., Chou, P.-H., Wu, C.-W., Chen, J.-H., and Liu, T.-C. (2009). Brain activation in patients with idiopathic hyperacusis. Am. J. Otolaryngol. 30, 432–434. doi: 10.1016/j.amjoto.2008.08.005
Isaacson, J. E., and Vora, N. M. (2003). Differential diagnosis and treatment of hearing loss. Am. Fam. Physician 68, 1125–1132.
Ison, J. R., Hammond, G. R., and Krauter, E. E. (1973). Effects of experience on stimulus-produced reflex inhibition in the rat. Journal of Comparative and Physiological Psychology 83, 324–336. doi: 10.1037/h0034423
Jahn, K. N., and Polley, D. B. (2023). Asymmetric hearing thresholds are associated with Hyperacusis in a Large clinical population. Hear. Res. 437:108854. doi: 10.1016/j.heares.2023.108854
Jiang, C., Luo, B., Manohar, S., Chen, G.-D., and Salvi, R. (2017). Plastic changes along auditory pathway during salicylate-induced ototoxicity: hyperactivity and CF shifts. Hear. Res. 347, 28–40. doi: 10.1016/j.heares.2016.10.021
Jüris, L., Andersson, G., Larsen, H. C., and Ekselius, L. (2013). Psychiatric comorbidity and personality traits in patients with hyperacusis. Int. J. Audiol. 52, 230–235. doi: 10.3109/14992027.2012.743043
Kaltenbach, J. A., and Afman, C. E. (2000). Hyperactivity in the dorsal cochlear nucleus after intense sound exposure and its resemblance to tone-evoked activity: a physiological model for tinnitus. Hear. Res. 140, 165–172. doi: 10.1016/S0378-5955(99)00197-5
Kaltenbach, J. A., and McCaslin, D. L. (1996). Increases in spontaneous activity in the dorsal cochlear nucleus following exposure to high intensity sound: a possible neural correlate of tinnitus. Audit. Neurosci. 3, 57–78.
Kaltenbach, J. A., Zhang, J., and Afman, C. E. (2000). Plasticity of spontaneous neural activity in the dorsal cochlear nucleus after intense sound exposure. Hear. Res. 147, 282–292. doi: 10.1016/S0378-5955(00)00138-6
Kandler, K., and Herbert, H. (1991). Auditory projections from the cochlear nucleus to pontine and mesencephalic reticular nuclei in the rat. Brain Res. 562, 230–242. doi: 10.1016/0006-8993(91)90626-7
Kiang, N., Moxon, E., and Levine, R. (1970). Auditory-nerve activity in cats with normal and abnormal cochleas, Ciba foundation symposium-sensorineural hearing loss. London: Wiley Online Library, 241–273.
Kilgard, M. P., and Merzenich, M. M. (1998). Cortical map reorganization enabled by nucleus basalis activity. Science 279, 1714–1718.
Kim, J. Y., Kim, Y. H., Lee, S., Seo, J. H., Song, H. J., Cho, J. H., et al. (2012). Alteration of functional connectivity in tinnitus brain revealed by resting-state fMRI?: a pilot study. Int. J. Audiol. 51, 413–417. doi: 10.3109/14992027.2011.652677
Knipper, L., Van Dijk, P., Nunes, I., Ruttiger, L., and Zimmermann, U. (2013). Advances in the neurobiology of hearing disorders: recent developments regarding the basis of tinnitus and hyperacusis. Prog. Neurobiol. 111, 17–33. doi: 10.1016/j.pneurobio.2013.08.002
Koch, M., Lingenhöhl, K., and Pilz, P. K. D. (1992). Loss of the acoustic startle response following neurotoxic lesions of the caudal pontine reticular formation: possible role of giant neurons. Neuroscience 49, 617–625. doi: 10.1016/0306-4522(92)90231-P
Komiya, H., and Eggermont, J. J. (2000). Spontaneous firing activity of cortical neurons in adult cats with reorganized tonotopic map following pure-tone trauma. Acta Otolaryngol. 120, 750–756. doi: 10.1080/000164800750000298
Koops, E. A., Renken, R. J., Lanting, C. P., and Van Dijk, P. (2020). Cortical Tonotopic map changes in humans are larger in hearing loss than in additional tinnitus. J. Neurosci. 40, 3178–3185. doi: 10.1523/jneurosci.2083-19.2020
Koops, E. A., and van Dijk, P. (2021). Hyperacusis in tinnitus patients relates to enlarged subcortical and cortical responses to sound except at the tinnitus frequency. Hear. Res. 401:108158. doi: 10.1016/j.heares.2020.108158
Kotak, V. C., Fujisawa, S., Lee, F. A., Karthikeyan, O., Aoki, C., and Sanes, D. H. (2005). Hearing loss raises excitability in the auditory cortex. J. Neurosci. 25, 3908–3918. doi: 10.1523/JNEUROSCI.5169-04.2005
Kotak, V. C., and Sanes, D. (1995). Synaptically evoked prolonged depolarizations in the developing auditory system. J. Neurophysiol. 74, 1611–1620. doi: 10.1152/jn.1995.74.4.1611
Kotak, V. C., Takesian, A. E., and Sanes, D. H. (2008). Hearing loss prevents the maturation of GABAergic transmission in the auditory cortex. Cereb. Cortex 18, 2098–2108. doi: 10.1093/cercor/bhm233
Krumbholz, K., Eickhoff, S. B., and Fink, G. R. (2007). Feature-and object-based attentional modulation in the human auditory “where” pathway. J. Cogn. Neurosci. 19, 1721–1733. doi: 10.1162/jocn.2007.19.10.1721
Kujawa, S. G., and Liberman, M. C. (2009). Adding insult to injury: cochlear nerve degeneration after "temporary" noise-induced hearing loss. J. Neurosci. 29, 14077–14085. doi: 10.1523/jneurosci.2845-09.2009
Langers, D. R., Kleine, E., and Dijk, P. (2012). Tinnitus does not require macroscopic tonotopic map reorganization. Front. Syst. Neurosci. 6:2. doi: 10.3389/fnsys.2012.00002
Langers, D. R., van Dijk, P., Schoenmaker, E. S., and Backes, W. H. (2007). fMRI activation in relation to sound intensity and loudness. Neuroimage 35, 709–718. doi: 10.1016/j.neuroimage.2006.12.013
Lanting, C., De Kleine, E., Bartels, H., and Van Dijk, P. (2008). Functional imaging of unilateral tinnitus using fMRI. Acta Otolaryngol. 128, 415–421. doi: 10.1080/00016480701793743
Leaver, A. M., Seydell-Greenwald, A., Turesky, T. K., Morgan, S., Kim, H. J., and Rauschecker, J. P. (2012). Cortico-limbic morphology separates tinnitus from tinnitus distress. Front. Syst. Neurosci. 6:21. doi: 10.3389/fnsys.2012.00021
Li, S., Choi, V., and Tzounopoulos, T. (2013). Pathogenic plasticity of Kv7. 2/3 channel activity is essential for the induction of tinnitus. Proc. Natl. Acad. Sci. 110, 9980–9985. doi: 10.1073/pnas.1302770110
Li, S., Kalappa, B. I., and Tzounopoulos, T. (2015). Noise-induced plasticity of KCNQ2/3 and HCN channels underlies vulnerability and resilience to tinnitus. eLife 4:e07242. doi: 10.7554/eLife.07242
Liu, X. P., Zhou, L. R., Ding, F. C., Wang, Y. H., and Yan, J. (2015). Local field potentials are local events in the mouse auditory cortex. Eur. J. Neurosci. 42, 2289–2297. doi: 10.1111/ejn.13003
Llinas, R., Ribary, U., Jeanmonod, D., Kronberg, E., and Mitra, P. (1999). Thalamocortical dysrhythmia, a neurological and neuropsychiatric syndrome characterized by magnetoencephalography. Proc. Natl. Acad. Sci. U. S. A. 96, 15222–15227.
Lockwood, A. H., Salvi, R., Coad, M., Towsley, M., Wack, D., and Murphy, B. (1998). The functional neuroanatomy of tinnitus: evidence for limbic system links and neural plasticity. Neurology 50, 114–120. doi: 10.1212/WNL.50.1.114
Loeb, M., and Smith, R. P. (2005). Relation of induced tinnitus to physical characteristics of the inducing stimuli. J. Acoust. Soc. Am. 42, 453–455. doi: 10.1121/1.1910600
Longenecker, R. J., and Galazyuk, A. V. (2011). Development of tinnitus in CBA/CaJ mice following sound exposure. J. Assoc. Res. Otolaryngol. 12, 647–658. doi: 10.1007/s10162-011-0276-1
Longenecker, R. J., and Galazyuk, A. V. (2016). Variable effects of acoustic trauma on behavioral and neural correlates of tinnitus in individual animals. Front. Behav. Neurosci. 10:207. doi: 10.3389/fnbeh.2016.00207
Lu, J., Lobarinas, E., Deng, A., Goodey, R., Stolzberg, D., Salvi, R. J., et al. (2011). GABAergic neural activity involved in salicylate-induced auditory cortex gain enhancement. Neuroscience 189, 187–198. doi: 10.1016/j.neuroscience.2011.04.073
Masri, S., Chan, N., Marsh, T., Zinsmaier, A., Schaub, D., Zhang, L., et al. (2021). Chemogenetic activation of cortical Parvalbumin-positive interneurons reverses noise-induced impairments in gap detection. J. Neurosci. 41, 8848–8857. doi: 10.1523/jneurosci.2687-19.2021
Maudoux, A., Lefebvre, P., Cabay, J. E., Demertzi, A., Vanhaudenhuyse, A., Laureys, S., et al. (2012). Connectivity graph analysis of the auditory resting state network in tinnitus. Brain Res. 1485, 10–21. doi: 10.1016/j.brainres.2012.05.006
Mazurek, B., Hesse, G., Dobel, C., Kratzsch, V., Lahmann, C., and Sattel, H. (2022). Chronic tinnitus. Dtsch. Arztebl. Int. 119, 219–225. doi: 10.3238/arztebl.m2022.0135
Mazzoni, A., Lindén, H., Cuntz, H., Lansner, A., Panzeri, S., and Einevoll, G. T. (2015). Computing the local field potential (LFP) from integrate-and-fire network models. PLoS Comput. Biol. 11:e1004584. doi: 10.1371/journal.pcbi.1004584
McGill, M., Hight, A. E., Watanabe, Y. L., Parthasarathy, A., Cai, D., Clayton, K., et al. (2022). Neural signatures of auditory hypersensitivity following acoustic trauma. eLife 11:e80015. doi: 10.7554/eLife.80015
Melcher, J. R., Levine, R. A., Bergevin, C., and Norris, B. (2009). The auditory midbrain of people with tinnitus: abnormal sound-evoked activity revisited. Hear. Res. 257, 63–74. doi: 10.1016/j.heares.2009.08.005
Middleton, J. W., Kiritani, T., Pedersen, C., Turner, J. G., Shepherd, G. M. G., and Tzounopoulos, T. (2011). Mice with behavioral evidence of tinnitus exhibit dorsal cochlear nucleus hyperactivity because of decreased GABAergic inhibition. Proc. Natl. Acad. Sci. 108, 7601–7606. doi: 10.1073/pnas.1100223108
Milbrandt, J., Holder, T., Wilson, M., Salvi, R., and Caspary, D. (2000). GAD levels and muscimol binding in rat inferior colliculus following acoustic trauma. Hear. Res. 147, 251–260. doi: 10.1016/S0378-5955(00)00135-0
Moazami-Goudarzi, M., Michels, L., Weisz, N., and Jeanmonod, D. (2010). Temporo-insular enhancement of EEG low and high frequencies in patients with chronic tinnitus. QEEG study of chronic tinnitus patients. BMC Neurosci. 11, 1–12. doi: 10.1186/1471-2202-11-40
Muhlnickel, W., Elbert, T., Taub, E., and Flor, H. (1998). Reorganization of auditory cortex in tinnitus. Proc. Natl. Acad. Sci. U. S. A. 95, 10340–10343.
Mulders, W., and Robertson, D. (2009). Hyperactivity in the auditory midbrain after acoustic trauma: dependence on cochlear activity. Neuroscience 164, 733–746. doi: 10.1016/j.neuroscience.2009.08.036
Munro, K. J., Turtle, C., and Schaette, R. (2014). Plasticity and modified loudness following short-term unilateral deprivation: evidence of multiple gain mechanisms within the auditory system. J. Acoust. Soc. Am. 135, 315–322. doi: 10.1121/1.4835715
Nahmani, M., and Turrigiano, G. G. (2014). Adult cortical plasticity following injury: recapitulation of critical period mechanisms? Neuroscience 283, 4–16. doi: 10.1016/j.neuroscience.2014.04.029
Ngodup, T., Goetz, J. A., McGuire, B. C., Sun, W., Lauer, A. M., and Xu-Friedman, M. A. (2015). Activity-dependent, homeostatic regulation of neurotransmitter release from auditory nerve fibers. Proc. Natl. Acad. Sci. 112, 6479–6484. doi: 10.1073/pnas.1420885112
Noda, T., and Takahashi, H. (2015). Anesthetic effects of isoflurane on the tonotopic map and neuronal population activity in the rat auditory cortex. Eur. J. Neurosci. 42, 2298–2311. doi: 10.1111/ejn.13007
Norena, A. J. (2011). An integrative model of tinnitus based on a central gain controlling neural sensitivity. Neurosci. Biobehav. Rev. 35, 1089–1109. doi: 10.1016/j.neubiorev.2010.11.003
Noreña, A. J., and Chery-Croze, S. (2007). Enriched acoustic environment rescales auditory sensitivity. Neuroreport 18, 1251–1255. doi: 10.1097/WNR.0b013e3282202c35
Norena, A., and Eggermont, J. (2003). Changes in spontaneous neural activity immediately after an acoustic trauma: implications for neural correlates of tinnitus. Hear. Res. 183, 137–153. doi: 10.1016/S0378-5955(03)00225-9
Norena, A., Moffat, G., Blanc, J., Pezard, L., and Cazals, Y. (2010). Neural changes in the auditory cortex of awake guinea pigs after two tinnitus inducers: salicylate and acoustic trauma. Neuroscience 166, 1194–1209. doi: 10.1016/j.neuroscience.2009.12.063
Norena, A. J., Tomita, M., and Eggermont, J. J. (2003). Neural changes in cat auditory cortex after a transient pure-tone trauma. J. Neurophysiol. 90, 2387–2401. doi: 10.1152/jn.00139.2003
Paltoglou, A. E., Sumner, C. J., and Hall, D. A. (2011). Mapping feature-sensitivity and attentional modulation in human auditory cortex with functional magnetic resonance imaging. Eur. J. Neurosci. 33, 1733–1741. doi: 10.1111/j.1460-9568.2011.07656.x
Parameshwarappa, V., Pezard, L., and Norena, A. J. (2022). Changes in the spatiotemporal pattern of spontaneous activity across a cortical column after noise trauma. J. Neurophysiol. 127, 239–254. doi: 10.1152/jn.00262.2021
Paus, T. (2000). Functional anatomy of arousal and attention systems in the human brain, Progress in brain research. Prog. Brain Res. 2000, 65–77. doi: 10.1016/S0079-6123(00)26007-X
Pienkowski, M. (2017). On the etiology of listening difficulties in noise despite clinically Normal audiograms. Ear Hear. 38, 135–148. doi: 10.1097/AUD.0000000000000388
Pienkowski, M. (2019). Rationale and efficacy of sound therapies for tinnitus and Hyperacusis. Neuroscience 407, 120–134. doi: 10.1016/j.neuroscience.2018.09.012
Pilati, N., Ison, M. J., Barker, M., Mulheran, M., Large, C. H., Forsythe, I. D., et al. (2012). Mechanisms contributing to central excitability changes during hearing loss. Proc. Natl. Acad. Sci. 109, 8292–8297. doi: 10.1073/pnas.1116981109
Popelar, J., Syka, J., and Berndt, H. (1987). Effect of noise on auditory evoked responses in awake guina pigs. Hear. Res. 26, 239–247. doi: 10.1016/0378-5955(87)90060-8
Qiu, C., Salvi, R., Ding, D., and Burkard, R. (2000). Inner hair cell loss leads to enhanced response amplitudes in auditory cortex of unanesthetized chinchillas: evidence for increased system gain. Hear. Res. 139, 153–171. doi: 10.1016/S0378-5955(99)00171-9
Radziwon, K., Auerbach, B. D., Ding, D. L., Liu, X. P., Chen, G. D., and Salvi, R. (2019). Noise-induced loudness recruitment and hyperacusis: insufficient central gain in auditory cortex and amygdala. Neuroscience 422, 212–227. doi: 10.1016/j.neuroscience.2019.09.010
Resnik, J., and Polley, D. B. (2017). Fast-spiking GABA circuit dynamics in the auditory cortex predict recovery of sensory processing following peripheral nerve damage. eLife 6:e21452. doi: 10.7554/eLife.21452
Resnik, J., and Polley, D. B. (2021). Cochlear neural degeneration disrupts hearing in background noise by increasing auditory cortex internal noise. Neuron 109, 984–996.e4. doi: 10.1016/j.neuron.2021.01.015
Roberts, L. E., Eggermont, J. J., Caspary, D. M., Shore, S. E., Melcher, J. R., and Kaltenbach, J. A. (2010). Ringing ears: the neuroscience of tinnitus. J. Neurosci. 30, 14972–14979. doi: 10.1523/jneurosci.4028-10.2010
Robinson, B. L., and McAlpine, D. (2009). Gain control mechanisms in the auditory pathway. Curr. Opin. Neurobiol. 19, 402–407. doi: 10.1016/j.conb.2009.07.006
Ropp, T. J., Tiedemann, K. L., Young, E. D., and May, B. J. (2014). Effects of unilateral acoustic trauma on tinnitus-related spontaneous activity in the inferior colliculus. J. Assoc. Res. Otolaryngol. 15, 1007–1022. doi: 10.1007/s10162-014-0488-2
Ros, C., Soler, C., and de Carellán Mateo, A. G. (2017). Comparison of the brainstem auditory evoked responses during sevoflurane or alfaxalone anaesthesia in adult cats. Vet. Anaesth. Analg. 44, 1085–1090. doi: 10.1016/j.vaa.2016.11.007
Rubio, M. E. (2006). Redistribution of synaptic AMPA receptors at glutamatergic synapses in the dorsal cochlear nucleus as an early response to cochlear ablation in rats. Hear. Res. 216-217, 154–167. doi: 10.1016/j.heares.2006.03.007
Ruttiger, L., Singer, W., Panford-Walsh, R., Matsumoto, M., Lee, S. C., Zuccotti, A., et al. (2013). The reduced Cochlear output and the failure to adapt the central auditory response causes tinnitus in noise exposed rats. PLoS One 8:e57247. doi: 10.1371/journal.pone.0057247
Salvi, R., Radziwon, K., Manohar, S., Ben, A., Ding, D. L., Liu, X. P., et al. (2021). Neural mechanisms of tinnitus and Hyperacusis in acute drug-induced ototoxicity. Am. J. Audiol. 30, 901–915. doi: 10.1044/2020_aja-20-00023
Salvi, R., Saunders, S., Gratton, M., Arehole, S., and Powers, N. (1990). Enhanced evoked response amplitudes in the inferior colliculus of the chinchilla following acoustic trauma. Hear. Res. 50, 245–257. doi: 10.1016/0378-5955(90)90049-U
Salvi, R. J., Wang, J., and Ding, D. (2000). Auditory plasticity and hyperactivity following cochlear damage. Hear. Res. 147, 261–274. doi: 10.1016/S0378-5955(00)00136-2
Sanes, D. H., and Kotak, V. C. (2011). Developmental plasticity of auditory cortical inhibitory synapses. Hear. Res. 279, 140–148. doi: 10.1016/j.heares.2011.03.015
Sarro, E. C., Kotak, V. C., Sanes, D. H., and Aoki, C. (2008). Hearing loss alters the subcellular distribution of presynaptic GAD and postsynaptic GABAA receptors in the auditory cortex. Cereb. Cortex 18, 2855–2867. doi: 10.1093/cercor/bhn044
Schaette, R., and Kempter, R. (2006). Development of tinnitus-related neuronal hyperactivity through homeostatic plasticity after hearing loss: a computational model. Eur. J. Neurosci. 23, 3124–3138. doi: 10.1111/j.1460-9568.2006.04774.x
Schaette, R., and McAlpine, D. (2011). Tinnitus with a Normal audiogram: physiological evidence for hidden hearing loss and computational model. J. Neurosci. 31, 13452–13457. doi: 10.1523/jneurosci.2156-11.2011
Schecklmann, M., Landgrebe, M., and Langguth, B.the TRI Database Study Group (2014). Phenotypic characteristics of hyperacusis in tinnitus. PLoS One 9:e86944. doi: 10.1371/journal.pone.0086944
Scholl, B., Gao, X., and Wehr, M. (2008). Level dependence of contextual modulation in auditory cortex. J. Neurophysiol. 99, 1616–1627. doi: 10.1152/jn.01172.2007
Scholl, B., and Wehr, M. (2008). Disruption of balanced cortical excitation and inhibition by acoustic trauma. J. Neurophysiol. 100, 646–656. doi: 10.1152/jn.90406.2008
Seki, S., and Eggermont, J. J. (2003). Changes in spontaneous firing rate and neural synchrony in cat primary auditory cortex after localized tone-induced hearing loss. Hear. Res. 180, 28–38. doi: 10.1016/S0378-5955(03)00074-1
Sereda, M., Hall, D. A., Bosnyak, D. J., Edmondson-Jones, M., Roberts, L. E., Adjamian, P., et al. (2011). Re-examining the relationship between audiometric profile and tinnitus pitch. Int. J. Audiol. 50, 303–312. doi: 10.3109/14992027.2010.551221
Sheldrake, J., Diehl, P. U., and Schaette, R. (2015). Audiometric characteristics of hyperacusis patients. Front. Neurol. 6:105. doi: 10.3389/fneur.2015.00105
Shiramatsu, T. I., Noda, T., Akutsu, K., and Takahashi, H. (2016). Tonotopic and field-specific representation of long-lasting sustained activity in rat auditory cortex. Front Neural Circuit 10:59. doi: 10.3389/fncir.2016.00059
Shore, S. E., Roberts, L. E., and Langguth, B. (2016). Maladaptive plasticity in tinnitus—triggers, mechanisms and treatment. Nat. Rev. Neurol. 12, 150–160. doi: 10.1038/nrneurol.2016.12
Sigalovsky, I. S., and Melcher, J. R. (2006). Effects of sound level on fMRI activation in human brainstem, thalamic and cortical centers. Hear. Res. 215, 67–76. doi: 10.1016/j.heares.2006.03.002
Stolzberg, D., Chen, G.-D., Allman, B. L., and Salvi, R. (2011). Salicylate-induced peripheral auditory changes and tonotopic reorganization of auditory cortex. Neuroscience 180, 157–164. doi: 10.1016/j.neuroscience.2011.02.005
Sturm, J. J., Zhang-Hooks, Y. X., Roos, H., Nguyen, T., and Kandler, K. (2017). Noise trauma-induced behavioral gap detection deficits correlate with reorganization of excitatory and inhibitory local circuits in the inferior colliculus and are prevented by acoustic enrichment. J. Neurosci. 37, 6314–6330. doi: 10.1523/jneurosci.0602-17.2017
Sun, W., Deng, A. C., Jayaram, A., and Gibson, B. (2012). Noise exposure enhances auditory cortex responses related to hyperacusis behavior. Brain Res. 1485, 108–116. doi: 10.1016/j.brainres.2012.02.008
Sun, W., Lu, J., Stolzberg, D., Gray, L., Deng, A., Lobarinas, E., et al. (2009). Salicylate increases the gain of the central auditory system. Neuroscience 159, 325–334. doi: 10.1016/j.neuroscience.2008.12.024
Sun, W., Manohar, S., Jayaram, A., Kumaraguru, A., Fu, Q., Li, J., et al. (2011). Early age conductive hearing loss causes audiogenic seizure and hyperacusis behavior. Hear. Res. 282, 178–183. doi: 10.1016/j.heares.2011.08.004
Syka, J., Rybalko, N., and Popelar, J. (1994). Enhancement of the auditory cortex evoked responses in awake guinea pigs after noise exposure. Hear. Res. 78, 158–168. doi: 10.1016/0378-5955(94)90021-3
Szalda, K., and Burkard, R. (2005). The effects of nembutal anesthesia on the auditory steady-state response (ASSR) from the inferior colliculus and auditory cortex of the chinchilla. Hear. Res. 203, 32–44. doi: 10.1016/j.heares.2004.11.014
Sztuka, A., Pospiech, L., Gawron, W., and Dudek, K. (2010). DPOAE in estimation of the function of the cochlea in tinnitus patients with normal hearing. Auris Nasus Larynx 37, 55–60. doi: 10.1016/j.anl.2009.05.001
Takahashi, H., Nakao, M., and Kaga, K. (2004). Distributed representation of sound intensity in the rat auditory cortex. Neuroreport 15, 2061–2065. doi: 10.1097/00001756-200409150-00013
Takahashi, H., Nakao, M., and Kaga, K. (2005a). Interfield differences in intensity and frequency representation of evoked potentials in rat auditory cortex. Hear. Res. 210, 9–23. doi: 10.1016/j.heares.2005.05.014
Takahashi, H., Nakao, M., and Kaga, K. (2005b). Spatial and temporal strategy to analyze steady-state sound intensity in cortex. Neuroreport 16, 137–140. doi: 10.1097/00001756-200502080-00013
Takahashi, H., Yokota, R., Funamizu, A., Kose, H., and Kanzaki, R. (2011). Learning-stage-dependent, field-specific, map plasticity in the rat auditory cortex during appetitive operant conditioning. Neuroscience 199, 243–258. doi: 10.1016/j.neuroscience.2011.09.046
Tan, A. Y., Atencio, C. A., Polley, D. B., Merzenich, M. M., and Schreiner, C. E. (2007). Unbalanced synaptic inhibition can create intensity-tuned auditory cortex neurons. Neuroscience 146, 449–462. doi: 10.1016/j.neuroscience.2007.01.019
Thornton, C., and Sharpe, R. (1998). Evoked responses in anaesthesia. Br. J. Anaesth. 81, 771–781. doi: 10.1093/bja/81.5.771
Turner, J. G., Brozoski, T. J., Bauer, C. A., Parrish, J. L., Myers, K., Hughes, L. F., et al. (2006). Gap detection deficits in rats with tinnitus: a potential novel screening tool. Behav. Neurosci. 120, 188–195. doi: 10.1037/0735-7044.120.1.188
Turner, J. G., and Larsen, D. (2016). Effects of noise exposure on development of tinnitus and hyperacusis: prevalence rates 12 months after exposure in middle-aged rats. Hear. Res. 334, 30–36. doi: 10.1016/j.heares.2015.11.004
Turner, J., Larsen, D., Hughes, L., Moechars, D., and Shore, S. (2012). Time course of tinnitus development following noise exposure in mice. J. Neurosci. Res. 90, 1480–1488. doi: 10.1002/jnr.22827
Turrigiano, G. (2012). Homeostatic synaptic plasticity: local and global mechanisms for stabilizing neuronal function. Cold Spring Harb. Perspect. Biol. 4:a005736. doi: 10.1101/cshperspect.a005736
Turrigiano, G. G., and Nelson, S. B. (2004). Homeostatic plasticity in the developing nervous system. Nat. Rev. Neurosci. 5, 97–107. doi: 10.1038/nrn1327
Tziridis, K., Ahlf, S., and Schulze, H. (2012). A Low Cost Setup for Behavioral Audiometry in Rodents. J. Vis. Exp. 68, e4433. doi: 10.3791/4433
Ulanovsky, N., and Moss, C. F. (2008). What the bat's voice tells the bat's brain. Proc. Natl. Acad. Sci. 105, 8491–8498. doi: 10.1073/pnas.0703550105
Vale, C., and Sanes, D. H. (2000). Afferent regulation of inhibitory synaptic transmission in the developing auditory midbrain. J. Neurosci. 20, 1912–1921. doi: 10.1523/JNEUROSCI.20-05-01912.2000
van Veen, E. D., Jacobs, J. B., and Bensing, J. M. (1998). Assessment of distress associated with tinnitus. J. Laryngol. Otol. 112, 258–263. doi: 10.1017/S002221510015830X
Wake, N., Ishizu, K., Abe, T., and Takahashi, H. (2021). Prepulse inhibition predicts subjective hearing in rats. Sci. Rep. 11:18902. doi: 10.1038/s41598-021-98167-6
Wake, N., Shiramatsu, T. I., and Takahashi, H. (2019). Tone frequency representation beyond the tonotopic map: cross-correlation between ongoing activity in the rat auditory cortex. Neuroscience 409, 35–42. doi: 10.1016/j.neuroscience.2019.04.026
Wake, M., Takeno, S., Ibrahim, D., Harrison, R., and Mount, R. (1993). Carboplatin ototoxicity: an animal model. J. Laryngol. Otol. 107, 585–589. doi: 10.1017/S0022215100123771
Wallhäusser-Franke, E., Mahlke, C., Oliva, R., Braun, S., Wenz, G., and Langner, G. (2003). Expression of c-fos in auditory and non-auditory brain regions of the gerbil after manipulations that induce tinnitus. Exp. Brain Res. 153, 649–654. doi: 10.1007/s00221-003-1614-2
Wang, H., Brozoski, T. J., and Caspary, D. M. (2011). Inhibitory neurotransmission in animal models of tinnitus: maladaptive plasticity. Hear. Res. 279, 111–117. doi: 10.1016/j.heares.2011.04.004
Wang, H., Brozoski, T. J., Turner, J. G., Ling, L., Parrish, J. L., Hughes, L. F., et al. (2009). Plasticity at glycinergic synapses in dorsal cochlear nucleus of rats with behavioral evidence of tinnitus. Neuroscience 164, 747–759. doi: 10.1016/j.neuroscience.2009.08.026
Wang, J., Powers, N., Hofstetter, P., Trautwein, P., Ding, D., and Salvi, R. (1997). Effects of selective inner hair cell loss on auditory nerve fiber threshold, tuning and spontaneous and driven discharge rate. Hear. Res. 107, 67–82. doi: 10.1016/S0378-5955(97)00020-8
Wang, J., and Salvi, R. (2002). Gamma-aminobutyric acid circuits shape response properties of auditory cortex neurons. Brain Res. 944, 219–231. doi: 10.1016/S0006-8993(02)02926-8
Wang, J., Salvi, R. J., and Powers, N. (1996). Plasticity of response properties of inferior colliculus neurons following acute cochlear damage. J. Neurophysiol. 75, 171–183. doi: 10.1152/jn.1996.75.1.171
Wehr, M., and Zador, A. (2003). Balanced inhibition underlies tuning and sharpnes spike timing in auditory cortex. Nature 426, 442–446. doi: 10.1038/nature02116
Weisz, N., Moratti, S., Meinzer, M., Dohrmann, K., and Elbert, T. (2005). Tinnitus perception and distress is related to abnormal spontaneous brain activity as measured by magnetoencephalography. PLoS Med. 2:e153. doi: 10.1371/journal.pmed.0020153
Weisz, N., Muller, S., Schlee, W., Dohrmann, K., Hartmann, T., and Elbert, T. (2007). The neural code of auditory phantom perception. J. Neurosci. 27, 1479–1484. doi: 10.1523/JNEUROSCI.3711-06.2007
Wong, E. D., Radziwon, K., Chen, G. D., Liu, X. P., Manno, F. A. M., Manno, S. H. C., et al. (2020). Functional magnetic resonance imaging of enhanced central auditory gain and electrophysiological correlates in a behavioral model of hyperacusis. Hear. Res. 389:107908. doi: 10.1016/j.heares.2020.107908
Wu, G. K., Li, P., Tao, H. W., and Zhang, L. I. (2006). Nonmonotonic synaptic excitation and imbalanced inhibition underlying cortical intensity tuning. Neuron 52, 705–715. doi: 10.1016/j.neuron.2006.10.009
Wu, C., Martel, D. T., and Shore, S. E. (2016). Increased synchrony and bursting of dorsal cochlear nucleus fusiform cells correlate with tinnitus. J. Neurosci. 36, 2068–2073. doi: 10.1523/JNEUROSCI.3960-15.2016
Xiong, B., Alkharabsheh, A., Manohar, S., Chen, G. D., Yu, N., Zhao, X., et al. (2017). Hyperexcitability of inferior colliculus and acoustic startle reflex with age-related hearing loss. Hear. Res. 350, 32–42. doi: 10.1016/j.heares.2017.03.011
Xu, H., Gong, N., Chen, L., and Xu, T.-L. (2005). Sodium salicylate reduces gamma aminobutyric acid-induced current in rat spinal dorsal horn neurons. Neuroreport 16, 813–816. doi: 10.1097/00001756-200505310-00007
Yang, G., Lobarinas, E., Zhang, L., Turner, J., Stolzberg, D., Salvi, R., et al. (2007). Salicylate induced tinnitus: behavioral measures and neural activity in auditory cortex of awake rats. Hear. Res. 226, 244–253. doi: 10.1016/j.heares.2006.06.013
Yang, S., Su, W., and Bao, S. (2012). Long-term, but not transient, threshold shifts alter the morphology and increase the excitability of cortical pyramidal neurons. J. Neurophysiol. 108, 1567–1574. doi: 10.1152/jn.00371.2012
Yang, S., Weiner, B. D., Zhang, L. S., Cho, S.-J., and Bao, S. (2011). Homeostatic plasticity drives tinnitus perception in an animal model. Proc. Natl. Acad. Sci. 108, 14974–14979. doi: 10.1073/pnas.1107998108
Zeng, F.-G. (2013). An active loudness model suggesting tinnitus as increased central noise and hyperacusis as increased nonlinear gain. Hear. Res. 295, 172–179. doi: 10.1016/j.heares.2012.05.009
Zeng, F.-G. (2020). Tinnitus and hyperacusis: central noise, gain and variance. Curr. Opin. Physio. 18, 123–129. doi: 10.1016/j.cophys.2020.10.009
Zhang, L. I., Tan, A. Y., Schreiner, C. E., and Merzenich, M. M. (2003). Topography and synaptic shaping of direction selectivity in primary auditory cortex. Nature 424, 201–205. doi: 10.1038/nature01796
Zhang, X., Yang, P., Cao, Y., Qin, L., and Sato, Y. (2011). Salicylate induced neural changes in the primary auditory cortex of awake cats. Neuroscience 172, 232–245. doi: 10.1016/j.neuroscience.2010.10.073
Zhou, Y., and Wang, X. (2010). Cortical processing of dynamic sound envelope transitions. J. Neurosci. 30, 16741–16754. doi: 10.1523/JNEUROSCI.2016-10.2010
Keywords: tinnitus, hyperacusis, auditory cortex, rat, hearing loss, acoustic trauma, plasticity
Citation: Wake N, Shiramatsu TI and Takahashi H (2024) Map plasticity following noise exposure in auditory cortex of rats: implications for disentangling neural correlates of tinnitus and hyperacusis. Front. Neurosci. 18:1385942. doi: 10.3389/fnins.2024.1385942
Edited by:
Alice Lisa Burghard, UCONN Health, United StatesReviewed by:
Adam Hockley, University of Salamanca, SpainJoel I. Berger, The University of Iowa, United States
Copyright © 2024 Wake, Shiramatsu and Takahashi. This is an open-access article distributed under the terms of the Creative Commons Attribution License (CC BY). The use, distribution or reproduction in other forums is permitted, provided the original author(s) and the copyright owner(s) are credited and that the original publication in this journal is cited, in accordance with accepted academic practice. No use, distribution or reproduction is permitted which does not comply with these terms.
*Correspondence: Hirokazu Takahashi, takahashi@i.u-tokyo.ac.jp