- Department of Neuroanatomy, Medical School Homburg/Saar, Institute for Anatomy and Cell Biology, Saarland University, Saarland, Germany
Photoreceptors, the light-sensitive receptor neurons of the retina, receive and transmit a plethora of visual informations from the surrounding world. Photoreceptors capture light and convert this energy into electrical signals that are conveyed to the inner retina. For synaptic communication with the inner retina, photoreceptors make large active zones that are marked by synaptic ribbons. These unique synapses support continuous vesicle exocytosis that is modulated by light-induced, graded changes of membrane potential. Synaptic transmission can be adjusted in an activity-dependent manner, and at the synaptic ribbons, Ca2+- and cGMP-dependent processes appear to play a central role. EF-hand-containing proteins mediate many of these Ca2+- and cGMP-dependent functions. Since continuous signaling of photoreceptors appears to be prone to malfunction, disturbances of Ca2+- and cGMP-mediated signaling in photoreceptors can lead to visual defects, retinal degeneration (rd), and even blindness. This review summarizes aspects of signal transmission at the photoreceptor presynaptic terminals that involve EF-hand-containing Ca2+-binding proteins.
Introduction
Vision belongs to the most important senses of the human body. The light-sensitive retina within our eyes screens the optical world around us and transmits this information to the brain. At the beginning of the complex task of visual perception, photoreceptors physically detect light energy and transmit the information to the inner retina where further processing takes place. The retina employs two different classes of photoreceptors, rod and cones, to begin sorting out different components of light. Rod photoreceptors are specialized to operate at the lowest level of light, single photon detection, and are thus saturated in daylight (Pahlberg and Sampath, 2011). Cone photoreceptors mediate color vision and operate at higher light intensities. In primates, e.g., humans, three different types of cones with long (L)-, medium (M)-, and short (S)- wavelength sensitivities provide color vision; simpler, non-primate mammals, e.g., mice, are dichromatic and possess only two types of cones (L-S-cones, for review, see Abramov and Gordon, 1994).
Mammalian photoreceptors in general are slender, highly polarized neurons with a bipolar morphology (Figure 1). The outer segment (OS) is the distal process that contacts the pigment epithelium and this is where phototransduction takes place. At the molecular level, phototransduction principally occurs via a light-induced transduction cascade that finally leads to closure of cGMP-gated cation channels (CNG-channels; cyclic nucleotide-gated (CNG) channels) which causes the cell to hyperpolarize from about −35 mV to −40 mV in the dark to about −70 mV in very bright light (for review, see Burns and Baylor, 2001; Chen, 2005). At the “opposite” (vitread) end of the photoreceptor, the presynaptic terminal transmits the light information to dendrites of secondary neurons, bipolar, and horizontal cells (Figures 1A,B). The vast array of light information detected by the photoreceptor OS must be transmitted at the first synapse of the visual system, the photoreceptor synapse (for review, see Wässle, 2004; Heidelberger et al., 2005; Schmitz, 2009; Matthews and Fuchs, 2010; Regus-Leidig and Brandstätter, 2011).
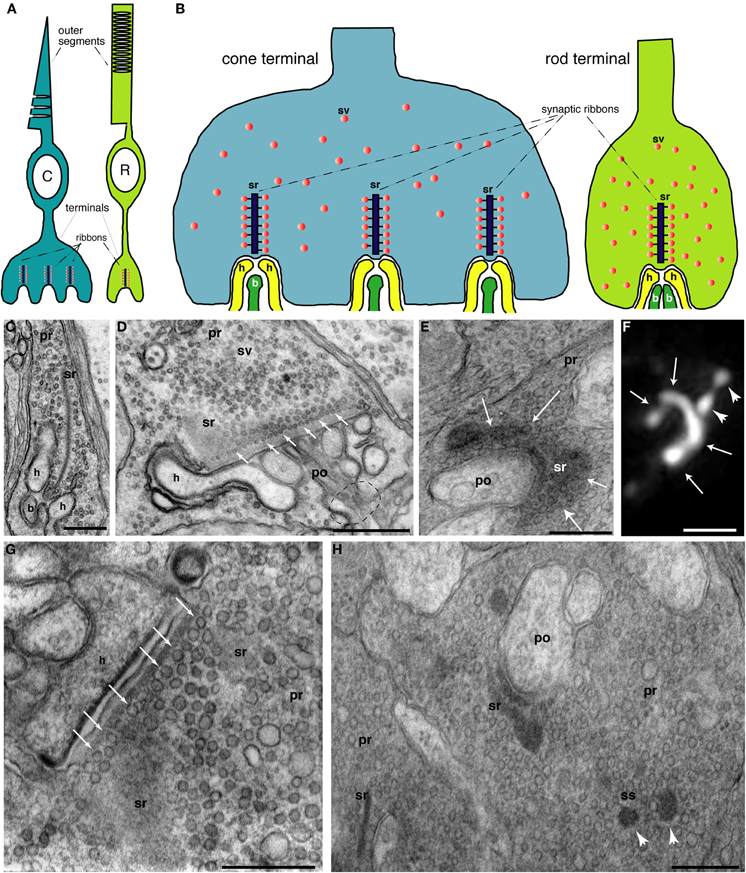
Figure 1. (A) Schematic, simplified drawing of rod (R) and cone (C) photoreceptors. Outer segments (OS) in which phototransduction occurs are depicted as well as the presynaptic terminal where light information is passed from photoreceptors to the secondary neurons, bipolar, and horizontal cells (depicted in yellow and dark green colors in Figure 1B). Subcellular details of photoreceptors including the inner segments were omitted for sake of clarity. (B) Schematic, simplified drawing of rod and cone photoreceptor presynaptic terminals. Rod synapses possess only a single, large active zone with a single synaptic ribbon (sr) whereas cones possess multiple active zones (20–50). Only invaginating ribbon synapses are depicted. Non-invaginating, non-ribbon type synapses (Regus-Leidig and Brandstätter, 2011) are not shown. (C–E, G–H) Electron micrographs of photoreceptor terminals. (C) Shows a cross-sectioned ribbon (sr) with its typical bar-shaped appearance in a rod terminal. The synaptic ribbon is associated with large numbers of synaptic vesicles (sv) (D). The rod photoreceptor in (D) is largely sectioned parallel to the plate-like synaptic ribbon. In the left part, the section passes through the synaptic ribbon (sr); more to the right, the plane of section is parallel, but close to the plate-like synaptic ribbon. Many docked synaptic vesicles can be observed at the base of the synaptic ribbon (small white arrows). The dashed circle indicates the site where the postsynaptic dendrites enter the postsynaptic cavity formed by the invagination of the presynaptic photoreceptor terminal. (E) Also shows a tangential view of the synaptic ribbon. The plate-like character of the ribbon is visible. White arrows denote the ribbon plate which is bended along the presynaptic plasma membrane in a horseshoe-like manner. The horseshoe-shaped appearance of the synaptic ribbon can be also visualized by immunolabeling with anti-RIBEYE antibodies and super-resolution, structured illumination microscopy (SIM) (white arrows in F). White arrowheads in (F) show spherical synaptic spheres (ss), intermediate structures in the assembly and disassembly of plate-shaped synaptic ribbons [see also below; in (H); for review, see Schmitz (2009)]. Figure (G) demonstrates many docked synaptic vesicles at the base of the synaptic ribbon (white arrows) which are probably readily releasable. (H) Electron micrograph of an immature, developing terminal from the early, postnatal mouse retina (postnatal day 6). The ribbon complex is not yet fully assembled. Besides bar-shaped ribbons (sr), spherical precursors of synaptic ribbons, the synaptic spheres (ss), are also present in the presynaptic terminal. Abbreviations: C, cone photoreceptor; R, rod photoreceptor; sr, synaptic ribbon; ss, synaptic spheres; sv, synaptic vesicle; pr, presynaptic terminal; po, postsynaptic dendrite; h, horizontal cell postsynaptic dendrite; b, bipolar cell postsynaptic dendrite. Scale bars: 400 nm (C); 800 nm (D); 320 nm (E); 1 μm (F), 400 nm (G), 500 nm (H).
Structural and Functional Specializations of Photoreceptor Ribbon Synapses: a Synapse Tuned for Phasic and Continuous Release
Both types of photoreceptors, rods, and cones, form ribbon synapses to communicate with their secondary neurons, i.e., bipolar and horizontal cells in the outer plexiform layer of the retina. In mammals, ribbon synapses are also made by retinal bipolar cells, photoreceptor-like neurons in the pineal gland as well as auditory and vestibular hair cells (Schmitz, 2009; Matthews and Fuchs, 2010; Regus-Leidig and Brandstätter, 2011). Ribbon synapses are characterized by large, electron-dense structures, the synaptic ribbons (Figure 1; for review, see Schmitz, 2009). Synaptic ribbons in photoreceptor synapses are plate-like structures which appear bar-shaped in electron micrographs if cross-sectioned (Figure 1; Schmitz, 2009). In rod synapses, typically one synaptic ribbon is contained at a single active zone; in cone synapses 20–50 active zones are present with each usually containing one synaptic ribbon (Wässle, 2004; Regus-Leidig and Brandstätter, 2011). In hair cell ribbon synapses, most synaptic ribbons are spherical in shape (for review, see Matthews and Fuchs, 2010). The synaptic ribbon is associated along its entire surface area with a large number of synaptic vesicles that are filled with the neurotransmitter glutamate. It is anchored at the active zone of the presynaptic plasma membrane; in photoreceptors via the electron-dense arciform density (for review, see Schmitz, 2009; Matthews and Fuchs, 2010; Regus-Leidig and Brandstätter, 2011). RIBEYE is the major component of synaptic ribbons (Schmitz et al., 2000; Magupalli et al., 2008; Schmitz, 2009; Uthaiah and Hudspeth, 2010). It consists of a large and unique aminoterminal A-domain, and a carboxyterminal B-domain which is largely identical with the nuclear co-repressor C-terminal-binding protein 2 (CtBP2). The B-domain/CtBP2 and a related protein, CtBP1, have developed from a family of dehydrogenases and both specifically bind NAD(H) (for review, see Schmitz, 2009).
Typically, ribbon synapses do not respond to bursts of action potentials but are specialized to transmit a large bandwidth of stimulus intensities via fine, graded changes in membrane potential. To report even small changes of receptor potential in response to differing light stimuli, ribbon synapses modulate the rate of tonic vesicle exocytosis (for review, see Heidelberger et al., 2005; Matthews and Fuchs, 2010; Wan and Heidelberger, 2011). Photoreceptor terminals may contain up to several hundred thousands of highly motile synaptic vesicles depending upon the species and type of synapse (for review, see Schmitz, 2009; Matthews and Fuchs, 2010), which support the high basal synaptic vesicle turnover driven by the synaptic ribbon (Figure 1). Various studies, mostly done with fish retinal bipolar cells, indicated that ribbon-associated vesicles are primed and readily-releasable (for review, see Heidelberger et al., 2005; Matthews and Fuchs, 2010; Wan and Heidelberger, 2011). Synaptic ribbons were proposed to capture and prime synaptic vesicles for immediate release. By this way of thinking, the synaptic ribbons would provide a battery of ready-to-go vesicles that could support continuous release for extended periods of time (Jackman et al., 2009). Synaptic ribbons are hot spots of exocytosis as visualized with TIRF-microscopy (Zenisek et al., 2000), and more recently by the analyses of terminals with photodamaged synaptic ribbons that showed strongly depressed release (Snellman et al., 2011). At the base of the synaptic ribbons, voltage-gated L-type calcium channels are highly enriched (tom Dieck et al., 2005). These channels allow voltage-dependent Ca2+-influx at the ribbon synapse which triggers synaptic vesicle release (for review, see Heidelberger et al., 2005; Schmitz, 2009; Striessnig et al., 2010). L-type calcium channels are considered ideally suited to serve the continuously active ribbon synapses (see below). Submicromolar (average) concentrations of Ca2+ are capable of supporting tonic exocytosis in photoreceptors (for review, see Heidelberger et al., 2005). Specific signaling properties of ribbon synapses could require higher Ca2+-concentrations that might be achieved at the base of the synaptic ribbons (Beutner et al., 2001; Choi et al., 2008; Jackman et al., 2009; Jarsky et al., 2010; Graydon et al., 2011). A recent study predicted concentrations up to 100 μM around the presynaptic Ca2+-channels (Graydon et al., 2011), which could support coordinated multivesicular release (Singer et al., 2004; Khimich et al., 2005; Jarsky et al., 2010; Graydon et al., 2011). RIBEYE is involved in the clustering of Ca2+-channels in inner ear hair cells (Sheets et al., 2011), and in agreement with this, several studies found a correlation between the ribbon size and the dimension of Ca2+-microdomains (Johnson et al., 2008; Frank et al., 2009, 2010).
The size and number of synaptic ribbons can vary considerably (Hull et al., 2006; Johnson et al., 2008; Frank et al., 2009, 2010; Regus-Leidig et al., 2010; Liberman et al., 2011; for review, see Vollrath and Spiwoks-Becker, 1996; Schmitz, 2009; Regus-Leidig and Brandstätter, 2011). The plate-shaped synaptic ribbons in photoreceptors appear to assemble and disassemble via spherical intermediates, the synaptic spheres (for review, see Schmitz, 2009; Mercer and Thoreson, 2011b). In the mouse retina, structural changes of synaptic ribbons are activity- (illumination-) dependent; structural changes of fish synaptic ribbons are also strongly influenced by circadian signals (Emran et al., 2010; for review, see Vollrath and Spiwoks-Becker, 1996; Regus-Leidig and Brandstätter, 2011). The activity-dependent plasticity of the synaptic ribbon complex is related to the performance of the visual system also at the systems level (Balkema et al., 2001). At photoreceptor ribbon synapses, postsynaptic dendrites of bipolar and horizontal cells contact the presynaptic release sites in an invagination of the presynaptic terminal (Figure 1). At this site, the released glutamate is detected by the metabotropic glutamate receptor 6 (mGluR6) on the tips of ON-bipolar cells; horizontal cells as well as OFF-bipolar cells employ ionotropic glutamate receptors (Wässle, 2004; DeVries et al., 2006; Morgans et al., 2010).
Recent data revealed that EF-hand-containing proteins play an important role in the activity-dependent adaptational processes at the photoreceptor synapse. These findings suggest that the photoreceptor synaptic apparatus is adjusted during changes in illumination, thus allowing synaptic communication to continue in a senseful manner if background illumination changes over a broad range. The processes in the presynaptic photoreceptor terminals that involve EF-hand-containing proteins, including distinct neuronal Ca2+-sensor (NCS) - proteins and Ca2+-binding proteins (CaBPs), will be summarized in the present review. Postsynaptic activity-dependent signaling is covered by other recent reviews (Burgoyne, 2007; Koike et al., 2010; Morgans et al., 2010).
Ca2+-ions and EF-hand-Containing Ca2+-binding Proteins: Outline
Ca2+-ions are crucial intracellular messengers that have central roles in synaptic transmission ranging from triggering of synaptic vesicle exocytosis, vesicle recruitment, and recovery as well as different aspects of synaptic plasticity (for review, Neher and Sakaba, 2008). Ca2+-binding EF-hand-containing proteins are perfect candidates for participating in photoreceptor signaling. These proteins are characterized by high-affinity Ca2+-binding motifs and consist of a helix-loop-helix motif (Burgoyne, 2007). The loop region, typically 12 residues long, is rich in acidic amino acids that chelate the Ca2+ (as well as Mg2+). The founder molecule is calmodulin, and related to calmodulin are two classes of EF-hand-containing proteins (Figures 2 and 3): (1) the family of neuronal calcium sensor (NCS) proteins that include the guanylate cyclase activating proteins (GCAPs) and (2) the family of calcium-binding proteins (CaBPs) that include calcium-binding protein 4 (CaBP4) (for review, see Haeseleer et al., 2002; Burgoyne, 2007). Furthermore, individual proteins contain EF-hand motifs as important functional parts of their primary structure, e.g., the α1-subunit of L-type voltage-gated Ca2+-channels (VGCCs).
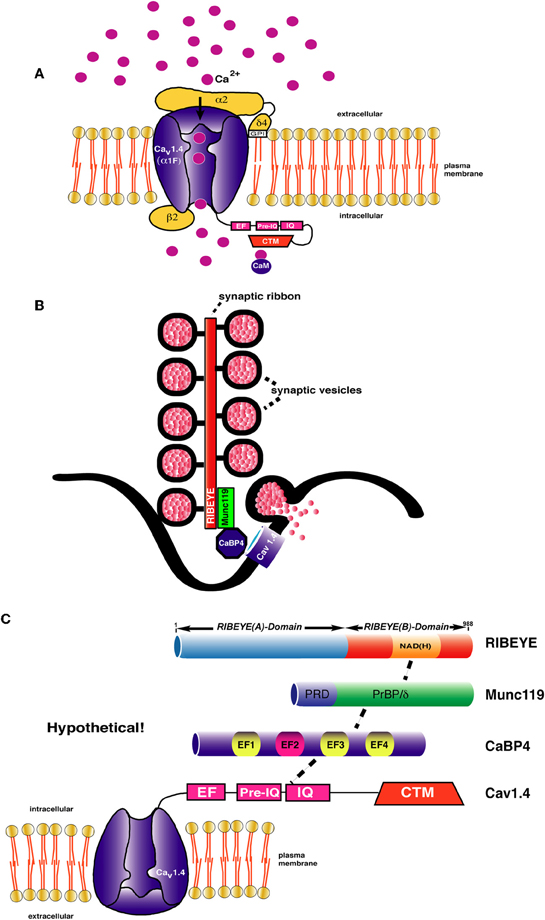
Figure 2. (A) Schematic representation of L-type Ca2+-channel composition of rod photoreceptor synapses [drawn modified based on Lacinova (2005)]. The channels are immobilized at the active zone close to the base of the synaptic ribbon. The α1F-subunit is considered the pore-forming subunit that supports voltage-dependent entry of Ca2+. Ca2+ ions are depicted as pink spheres. The cytoplasmic C-terminus of CaV1.4 α 1-subunit contains an EF-hand, Pre-IQ-, and IQ-domain. In other CaV1 channels, e.g., CaV1.2, these carboxyterminal domains mediate Ca2+ -dependent inactivation [for review, see Striessnig et al. (2010)]. In CaV1.4, CDI is prevented by the additional CTM region that forms an intramolecular interaction with the above mentioned domains [Singh et al. (2006); Wahl-Schott et al. (2006)]. The β2-subunit interacts with the α1-subunit at the cytoplasmic loop connecting domain I with domain II [Catterall (2011)]. The alpha2-delta4 (α2δ 4)-subunit, linked to each other with disulfide-bridges (not shown), complements the channel composition [Wycisk et al. (2006); Mercer et al. (2011a)]. The δ -subunit possesses a single transmembrane segment which is post-translationally cleaved off and replaced by a GPI anchor [Davies et al. (2010)] (B,C) Schematic depiction of the synaptic ribbon. Protein-protein interaction cascades are shown that could link RIBEYE to presynaptic calcium channels. Although all individual interactions (e.g., RIBEYE-Munc119; Munc119-CaBP4; CaBP4-CaV1.4) have been demonstrated [Alpadi et al. (2008); Haeseleer et al. (2004, 2008)], it is not clear whether all shown interactions can occur at the same time. Other interactions that might link the ribbons to presynaptic calcium channels, e.g., via association with RIM-proteins are not shown. Domain structures of the interacting proteins are only schematically depicted. CaBP4 contains 4 EF-hands from which EF2 (depicted in red) is non-functional. EF1, EF3, and EF4 are functional EF-hands (depicted in yellow). Abbreviations: CaM, calmodulin; PrBP/δ, prenyl-binding protein delta homology domain; PRD, proline-rich domain; IQ, IQ-domain; NAD(H), nicotine amide dinucleotide; CTM, C-terminal modulator.
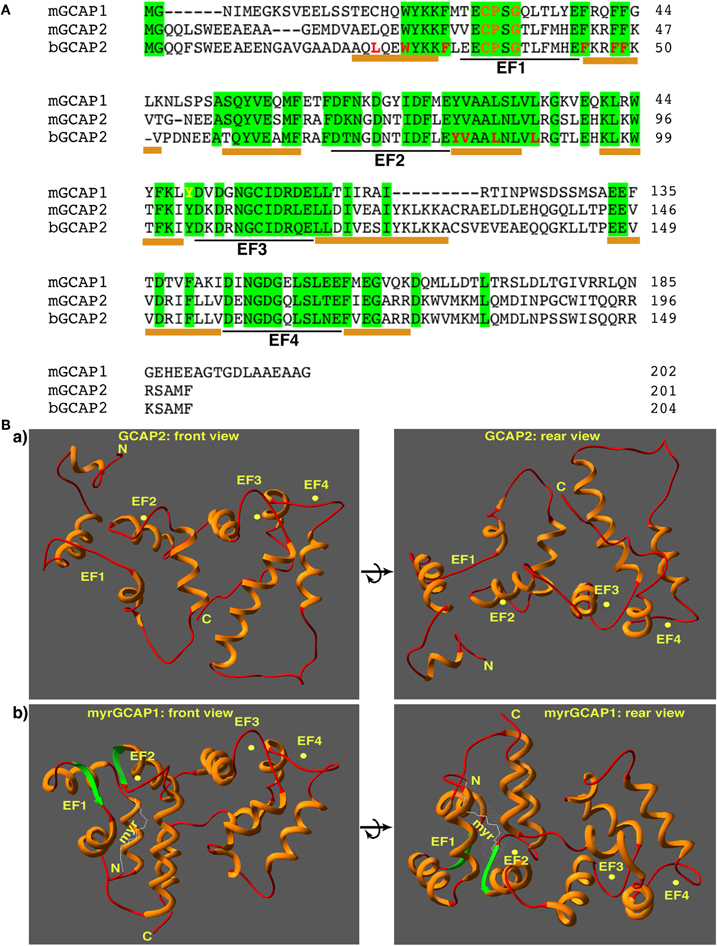
Figure 3. (A) Sequence alignment of GCAP1 and GCAP2 from the indicated species (mGCAP1: NP032215, GI: 40254633; mGCAP2: NP_666191, GI: 22122571; bGCAP2: NP_777211, GI: 27807519). Amino acid residues identical in all three indicated GCAP proteins are highlighted in green. Underlined below the aligned amino acid sequences is the Ca2+-/Mg2+-chelating loop region located between the E- and F- helices of the respective EF-hands. It is flanked on both sides by an α-helix (underlined in amber). The amino acid sequences of the EF-hands of GCAP1 and GCAP2 are highly homologous. Amino acids identical in mGCAP1, mGCAP2, and bGCAP2 are highlighted in green. EF-hands are highly conserved; the C-terminus of GCAP1 of GCAP2 is divergent. The CTR of GCAP2, but not of GCAP1, binds to the NADH-binding sub-domain of RIBEYE(B) [Venkatesan et al. (2010)]. Amino acids in GCAP2 highlighted in red appear to be involved in the interaction with ROS-GCs [Ames et al. (1999)]. Residues in the loop region of EF1 that are incompatible with Ca2+-chelation and also involved in ROS-GC target interaction are shown in orange [Ames et al. (1999); Hwang et al. (2004)]. Abbreviations: mGCAP1, mouse GCAP1; mGCAP2, mouse GCAP2, bGCAP2, bovine GCAP2. (B) Structure of unmyristoylated GCAP2 (a) [Ames et al. (1999); pdb-file: 1jba] and myristoylated GCAP1 (b) [Stephen et al. (2007); pdb-file: 2R2I]. The structure is shown from the front (left) with the Ca2+-chelating loops on top as well as from the back (right) to document the location of the CTR region that binds to RIBEYE(B) in the case of GCAP2 [Venkatesan et al. (2010)]. Ca2+ ions are schematically depicted as yellow spheres.
[Ca2+]i in Presynaptic Photoreceptor Terminals
EF-hand-containing proteins typically bind Ca2+ in the submicromolar range and are regulated by [Ca2+]i. In photoreceptor terminals, presynaptic [Ca2+]i is controlled by various mechanisms. These include [Ca2+]i- influx through calcium-permeable channels in the presynaptic plasma membrane (VGCCs, probably also CNG- and hyperpolarization-activated, cyclic nucleotide-gated (HCN)-channels), Ca2+-buffering systems in the presynaptic terminals, Ca2+-release from the ER (e.g., Ca2+-induced Ca2+-release) as well as extrusion from the cytosol into the ER and the extracellular space (e.g., via plasma membrane Ca2+-ATPase; Na+/Ca2+, K+-exchanger) (Rieke and Schwartz, 1994; Savchenko et al., 1997; Krizaj and Copenhagen, 2002; Suryanarayanan and Slaughter, 2006; Johnson et al., 2007; Knop et al., 2008; Szikra et al., 2008, 2009; Babai et al., 2010; Seeliger et al., 2011). Importantly, Ca2+-concentrations in the presynaptic terminals of photoreceptors have been imaged in-situ using two-photon-microscopy (Choi et al., 2008; Jackman et al., 2009). In the anole lizard (Anolis segrei), 360–600 nm global (average) Ca2+ were measured in cone terminals of dark-adapted retinas; 190–250 nm of global average Ca2+ after bright illumination at physiological extracellular Ca2+-concentrations. At the base of the synaptic ribbon, [Ca2+]i could be much higher than these average values (>4 μM) (Choi et al., 2008; Jackman et al., 2009).
These [Ca2+]i values in the presynaptic terminal differ from [Ca2+]i values in the OS. In the OS of mouse retinas, dark values of 250 nm were measured; down to 23 nm [Ca2+]i were measured in the OS of mice at saturating illumination (Olshevskaya et al., 2002; Woodruff et al., 2002; Koch, 2006; Baehr and Palczewski, 2009). Species-dependent differences in OS [Ca2+]i values have been observed: dark values of ≈700 nm [Ca2+]i were measured in salamander rod OS; many species have dark [Ca2+]i values of ≈500 nm (Olshevskaya et al., 2002; Woodruff et al., 2002; Koch, 2006; Karan et al., 2010). Differences of [Ca2+]i between presynaptic terminals and outer/inner segments could result from the elongated, slender shape of photoreceptors and various Ca2+-extrusion mechanisms between OSs and presynaptic terminals (Krizaj and Copenhagen, 2002). Additionally, signals in the presynaptic terminals are shaped by feedback responses from secondary neurons (Jackman et al., 2010; Regus-Leidig and Brandstätter, 2011).
L-type Voltage-Gated Calcium Channels in Photoreceptor Presynaptic Terminals
The rate of synaptic vesicle exocytosis at ribbon synapses is highly dependent on changes in membrane potential, and the role of voltage-gated calcium channels in this process has been intensively investigated. Synaptic vesicle exocytosis in rod and cone photoreceptor synapses is triggered via Ca2+-influx through L-type voltage-gated calcium channels (LTCCs) at the active zones (for review, see Morgans et al., 2005; Striessnig et al., 2010; Catterall, 2011). The α1-subunit is the largest subunit of LTCCs. CaV1.4 (often also denoted as α1F-subunit (Cacna1f); Catterall et al., 2005) is believed to represent the main pore forming α1-subunit of LTCCs involved in neurotransmitter release at photoreceptor synapses. This assumption is based on several findings: (1) immunocytochemical analyses (Nachman-Clewner et al., 1999; Morgans, 2001; for review, see Morgans et al., 2005); (2) analyses of spontaneous and engineered CaV1.4 mouse knockouts (for review, see Doering et al., 2007; Striessnig et al., 2010). (3) human patients suffering from congenital stationary night blindness (CSNB) show mutations in the CaV1.4 gene (for review, see Doering et al., 2007; Striessnig et al., 2010). Some studies also observed expression of CaV1.3 (also denoted as α1D-subunit (Cacna1d); Catterall et al., 2005) in photoreceptor synapses (Xiao et al., 2007; Kersten et al., 2010). Inner ear hair cell ribbon synapses employ CaV1.3 as pore-forming Ca2+-channel α1-subunit (for review, see Striessnig et al., 2010). But while hearing is severely impaired, vision appears to be normal in CaV1.3 knockout mice (for review, see Striessnig et al., 2010).
CaV1.4 (α1F) is ≈2000 amino acids long and organized into four homologous domains (domain I–IV) (Catterall et al., 2005; Catterall, 2011). Both N- and C-terminus reside in the cytoplasm (Figure 2). The C-terminus (CTR) of CaV1.4 possesses important regulatory functions and consists of a Ca2+-binding EF-hand domain, a pre-IQ and IQ-domain as well as an important regulatory region at the very carboxyterminus, the so-called CTM (C-terminal modulator) or ICDI (inhibitor of CDI) (Singh et al., 2006; Wahl-Schott et al., 2006; Striessnig et al., 2010). The CTM performs functionally important intramolecular interactions with the carboxyterminus of CaV1.4 (see below). The α1-subunit associates with cytoplasmic β-subunits, predominantly at the loop region between domain I and II of CaV1.4 (Dolphin, 2003; Buraei and Yang, 2010). The β2-protein appears to be the main β-channel subunit in photoreceptor LTCCs (Ball et al., 2002, 2011). β-subunits are important for the trafficking of the α1-subunit and for the kinetics of channel opening (Dolphin, 2003; Buraei and Yang, 2010). The CaV1.4 channel is complemented by an α 2δ-subunit, which is the α 2δ 4 protein in photoreceptor synapses (Wycisk et al., 2006; Mercer et al., 2011a).
The properties of CaV1.4 and CaV1.3 can be modulated over a wide range (for review, see Striessnig et al., 2010). In some contexts, CaV1.4 and CaV1.3 open at relatively negative membrane potentials (below –40 mV) which is an important requirement for photoreceptors that vary their membrane potential between –35 and –40 mV (in the dark) to less than –55 mV in the light (see above). Furthermore, for the tonically active photoreceptor synapses it is important that a sufficient Ca2+-concentration is maintained that allows sustained, continuous exocytosis. This could be well accomplished by a calcium channel that does not inactivate or inactivates only very slowly. CaV1.4 shows no Ca2+-dependent inactivation (CDI) and very slow voltage-dependent inactivation (VDI) (Singh et al., 2006; Wahl-Schott et al., 2006; Striessnig et al., 2010). This low degree or lack of inactivation could very well support continuous Ca2+-influx and subsequently tonic exocytosis. Further supplies of Ca2+ that may help maintain sustained release could come from Ca2+-induced Ca2+ release or store-operated Ca2+-entry (Suryanarayanan and Slaughter, 2006; Szikra et al., 2008, 2009; Babai et al., 2010).
The biological purpose of CDI (and VDI), in general, is to provide neurons with a negative feedback mechanism that can protect from Ca2+-overflow and subsequent cell death. CDI is mediated by the EF-hand, the pre-IQ-domain, and the IQ-domain in the CTR of CaV1.4 to which Ca2+/calmodulin can bind (for review, see Doering et al., 2007; Striessnig et al., 2010). In CaV1.4, CDI is absent because of a modulatory domain in the CTR of CaV1.4 that prevents binding of Ca2+-calmodulin to the pre-IQ/IQ-domain. CDI would probably not be compatible with the need of continuous, tonic exocytosis at photoreceptor synapses that also requires tonic Ca2+-influx to drive exocytosis. Mutations in the CaV1.4 gene are associated with incomplete stationary night blindness (CSNB2) (for review, see Striessnig et al., 2010). Inhibition of CDI in inner ear hair cells is mediated by the binding of CaBP4 to the CTR of CaV1.3 (Yang et al., 2006). CaBP4 is an EF-hand-containing protein of the CaBP-family (Haeseleer et al., 2004; Haeseleer, 2008).
In photoreceptor synapses, CaBP4 could have an additional function. Binding of CaBP4 to the IQ-domain of CaV1.4 shifts the activation curve of the channel to more negative values (Haeseleer et al., 2004), thereby extending the operational range of the channel. At –40 mV, the membrane potential in the dark, the depolarized condition, the channel is at the very beginning of its activation curve (for review, see Striessnig et al., 2010). At –50 mV, a membrane potential which is easily achieved during illumination, the CaV1.4 channel would be closed. A CaBP4-induced hyperpolarizing shift of the CaV1.4 activation curve (shift of approximate 10–15 mV) would allow the channel to operate at more negative membrane potentials. It should be kept in mind that many of the biophysical characterizations were obtained from powerful, but simplified, model systems, e.g., transfected HEK cells. Channel regulation in the synapse could be more complex.
Mutations in the CaBP4 gene lead to autosomal recessive CSNB and Leber's congenital amaurosis (LCA)-like phenotype in humans (Zeitz et al., 2006; Aldahmesh et al., 2010); CaBP4 knockout mice have severe disturbances in synaptic transmission emphasizing the physiological importance of this protein. Interestingly, RIBEYE, the main component of synaptic ribbons binds to Munc119 (Alpadi et al., 2008), a protein which has been linked with a cone-rod dystrophy (CORD) (Kobayashi et al., 2000). Munc119, on the other hand, interacts with CaBP4 (Haeseleer, 2008; Alpadi and Schmitz, unpublished data). This multicomponent molecular connection could influence the gating of Ca2+-channels at the active zone of photoreceptors (Figure 2).
The β-subunit of LTCC—together with other channel subunits (i.e., α2δ4; Figure 2) and further channel-associated proteins—plays an important role in the regulation of the kinetics of Ca2+-channel opening, intracellular channel trafficking, and density at the plasma membrane (Dolphin, 2003; Davies et al., 2007; Buraei and Yang, 2010; Striessnig et al., 2010). Deletion of β2-subunit cause similar phenotypes as in CSNB2 patients with CaV1.4 mutations (Ball et al., 2002). β-subunit might be involved in the positional priming of calcium channels and the exocytotic machinery. β-subunits of LTCC bind to the RIM family of active zone proteins (Kiyonaka et al., 2007; Miki et al., 2007; Gebhart et al., 2010) via a carboxyterminal region that includes the C2B-domain of RIMs. RIM proteins are important for vesicle exocytosis, various steps of presynaptic plasticitiy and for the immobilization of Ca2+-channels as shown mostly for conventional synapses (Han et al., 2011; Kaeser et al., 2011). RIMs are also components of the active zone complex of photoreceptors including the synaptic ribbons (Wang et al., 1997). Via the proline-rich region, RIM proteins bind to the RIM-binding proteins (RBPs) which associate with the β-subunit of L-type Ca2+-channels (Hibino et al., 2002). Most interestingly, RIM knockouts lead to loss of Ca2+-channel immobilization in conventional synapses (Han et al., 2011; Kaeser et al., 2011, for review, see Kaeser, 2011). RIM proteins are also important in modulating voltage-gated Ca2+-channels as judged by a mutation in the C2A-domain of RIM1 that causes cone-rod dystrophy (CORD7) (Miki et al., 2007).
In conclusion, modulation of L-type Ca2+-channel properties appears to have a powerful influence on synaptic transmission at the photoreceptor synapse (Striessnig et al., 2010). The plasticity is mediated by the EF-hand/Pre-IQ/IQ-domain-containing carboxyterminal region of the α-channel subunits. Tuning of the Ca2+-channels could be involved in the adjustment of synaptic transmission during different levels of illumination and/or for slower, adaptation of the exocytotic machinery for overall changes of light- and dark-adaptation during day- and night time. Interestingly, L-type calcium channel expression in photoreceptors is likely under circadian control (Ko et al., 2007).
EF-Hand Proteins and Ca2+-/cGMP-Dependent Plasticity at the Synaptic Ribbon
As described above, EF-hand motif-containing proteins are important Ca2+-dependent modulators of presynaptic voltage-gated Ca2+-channel functions. Also the synaptic ribbons are subject to Ca2+-dependent dynamic changes which in turn could feedback on presynaptic Ca2+-levels. Presynaptic Ca2+-channels are anchored at the active zone of photoreceptor synapses by the synaptic ribbons. RIBEYE appears to have a central role in the clustering of Ca2+-channels in inner ear hair cells (Sheets et al., 2011). Ribbon-associated proteins, e.g., the above mentioned RIM proteins or the protein bassoon, could potentially also play an important role (Wang et al., 1997; tom Dieck et al., 2005; Frank et al., 2010; Han et al., 2011; Kaeser et al., 2011). The ribbon-associated protein bassoon anchors synaptic ribbons to the active zone probably via its interaction with RIBEYE (tom Dieck et al., 2005). Bassoon is important for ribbon synapse development and maintaining the stability of the synaptic ribbon complex (Dick et al., 2003; tom Dieck et al., 2005; Regus-Leidig et al., 2010).
Recent studies suggested that activity-dependent structural changes of photoreceptor synaptic ribbons, i.e., assembly and disassembly of synaptic ribbons, are mediated by GCAP2, the guanylate cyclase-activating protein 2 (Venkatesan et al., 2010). GCAP2 belongs to a family of small Ca2+-regulated, EF-hand-containing proteins of the NCS protein family (Koch, 2006; Burgoyne, 2007; Koch et al., 2010; Sharma, 2010). GCAPs are well known to regulate guanylate cyclase (GC) activity in photoreceptor OSs in a Ca2+-dependent manner. How GCAPs could work in the presynaptic photoreceptor terminals to regulate synaptic plasticity is unclear. Current knowledge and ideas about GCAP/GC/cGMP-mediated signaling events in the presynaptic terminals will be summarized in the present review. To elucidate possible similarities between regulatory mechanisms in the OS and synaptic terminals, some key events of OS phototransduction will be also included.
Guanylate Cyclase-Activating Proteins (GCAPs) in Photoreceptors
Guanalyte cyclase-activating proteins (GCAPs) are small, EF-hand-containing Ca2+-binding proteins of ≈24 kDa (Figure 3). GCAPs belong to the subfamily of NCS proteins (Koch, 2006; Burgoyne, 2007). They contain four EF-hands, and the first EF-hand in GCAPs is non-functional due to exchanges of critical amino acids in the Ca2+-binding loop (Figure 3). Instead, EF1 provides a binding interface for the membrane-bound photoreceptor guanylate cyclases (ROS-GCs; Ermilov et al., 2001; see below). EF2–4 are functionally active and bind Ca2+ (as well as Mg2+). In the OSs, the free intracellular Mg2+-concentration is largely constant (at ≈1 mM) and not affected by changes in illumination (Chen, 2005; Peshenko et al., 2011a). In contrast, free intracellular Ca2+ levels change strongly upon illumination as described above. If Ca2+ (and cGMP) is high (in the dark), Ca2+ will replace the bound Mg2+ at the EF-hands of GCAPs (Stephen et al., 2008; Dizhoor et al., 2010; Peshenko et al., 2011a). The replacement of Mg2+ by Ca2+ at the EF-hands of GCAPs is functionally important because this changes the character of interaction with important effector proteins, the guanylate cyclases (GC, see below). GCAP proteins are myristoylated at their N-terminus (for review, see Palczewski et al., 2004; Koch, 2006; Baehr and Palczewski, 2007, 2009). In contrast to the recoverin-like NCS proteins, GCAPs do not perform a Ca2+-dependent myristoyl-switch (Stephen et al., 2007; Ames and Lim, 2011). Irrespective whether Ca2+ is bound or not, the myristoyl chain remains buried inside the molecule and is not involved in Ca2+-dependent membrane anchoring (Figure 3). Instead, the myristoyl residue has been suggested to stabilize the conformation of the protein (Stephen et al., 2007).
Three GCAP isoforms (GCAP1, GCAP2, and GCAP3) are expressed in mammalian retinas with species-dependent differences (Palczewski et al., 2004; Koch, 2006; Baehr and Palczewski, 2007, 2009; Dizhoor et al., 2010). In rod photoreceptors of mouse retinas, both GCAP1 and GCAP2 are expressed. GCAP1 appears to be the predominant isoform in cones (Palczewski et al., 2004; Koch, 2006; Baehr and Palczewski, 2007, 2009). Consistently, mutations of the GCAP1 gene lead to cone-dominated dystrophies in the human retina as well as in the respective mouse models (Jiang et al., 2005; Buch et al., 2011). GCAP3 expression is restricted to cone photoreceptors in the human retina; in the mouse retina GCAP3 is not expressed arguing that GCAP3 is probably dispensable for vision in mice (for review, see Baehr and Palczewski, 2007, 2009). Despite strong sequence similarities, biophysical and biochemical properties of GCAP proteins differ (e.g., Ca2+-affinities, dimerization properties, and activation of GCs; Ermilov et al., 2001; Olshevskaya et al., 2002; Koch et al., 2010). In photoreceptor outer segments (OS), GCAPs constitutively associate with membranes via interaction with ROS-GCs (Olshevskaya et al., 2002; Stephen et al., 2007; Ames and Lim, 2011). Mice with a deletion of GCAP1 and GCAP2 genes showed increased amplitudes of single photon responses and a delayed recovery phase (for review, see Palczewski et al., 2004; Baehr and Palczewski, 2007, 2009).
GCAP Effector Proteins in Photoreceptor Outer Segments
In photoreceptor OSs, GCAP effector proteins have been extensively characterized (Karan et al., 2010; Hunt et al., 2010; Koch et al., 2010). Main effectors of GCAP proteins are the ≈115 kDa membrane-bound rod outer segment-guanylate cyclases (ROS-GCs). Two ROS-GCs are found in mammalian photoreceptors: ROS-GC1 (retGC1, GC-E) and ROS-GC2 (retGC2, GCF) (for review, see Olshevskaya et al., 2002; Potter, 2011). ROS-GCs are large, type 1 transmembrane proteins (≈1100 aa; Figure 4) with an extracellular domain, a transmembrane domain, and a cytoplasmic domain that consists of a short juxtamembrane domain (JMD), a kinase homology domain (KHD), a dimerization domain (DD), a catalytic domain (CCD) that converts GTP into cGMP and C-terminal extension (CTE). Both ROS-GC1 and ROS-GC2 are expressed in rods; ROS-GC2 appears to be absent from mouse cone photoreceptors (Haire et al., 2006; Karan et al., 2010). ROS-GCs play a crucial role in photoreceptor OS phototransduction. A light-induced conformational change of rhodopsin leads to a transducin-mediated activation of phosphodiesterase 6 (PDE6) and subsequently reduced levels of cGMP (Burns and Baylor, 2001). Thus, light generates a drop in cGMP levels in the OSs and subsequent closure of cGMP-gated CNG-channels (Biel and Michalakis, 2009). As a result of light-induced closure of CNG channels intracellular Ca2+ levels drop in the OS from about 250nM (dark) to less than <50 nM (light) in the mouse retina. Light-induced decreased levels of cGMP need to be replenished in order to be able to detect the next flash of light. Recovery of cGMP levels is accomplished by a Ca2+-dependent feedback mechanism mediated by GCAP proteins. After illumination (at low Ca2+), GCAPs are in the Mg2+-bound state and stimulate GC activity. In contrast, in the Ca2+-bound state (at high Ca2+ in the dark) GCAPs inhibit GC activity (Koch, 2006; Sharma, 2010; Sakurai et al., 2011). Thus, GCAPs work as bimodal regulators of GCs: as an inhibitor of GC activity function (if Ca2+ is bound) and as an activator of GC function (and cGMP synthesis) if Mg2+ is bound. At low Ca2+ levels (light), GCAPs activate GCs and thus raise cGMP levels to restore pre-flash cGMP levels. These fundamental properties of GCAP proteins are crucial for the Ca2+-dependent feedback of the phototransduction cascade. This is necessary to make the OS responsive to new flashes of light and to reset the sensitivity of the phototransduction cascade to different levels of illumination. Particularly EF-hand 3 (EF3) emerged as key region that determines whether GCAPs act as an activator or inhibitor of GCs (Olshevskaya et al., 2002; Baehr and Palczewski, 2007, 2009).
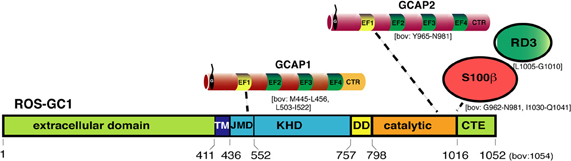
Figure 4. Schematic representation of ROS-GC1 and ROS-GC1-interaction partners in photoreceptors. ROS-GC1 contains an aminoterminal extracellular domain, transmembrane domain (TM), kinase homology domain (KHD), dimerization domain (DD), and the catalytic domain that converts GTP into cGMP. The aminoterminal portion of the KHD is also referred to as juxtamembrane domain (JMD) [Lange et al. (1999)]. The borders of the respective domains are schematically depicted in the amino acid sequence of human ROS-GC1 (NP_000171, GI: 4504217). Numbers indicated correspond to the mature ROS-GC1 protein (without leader sequence). The borders of the individual domains were determined by the analyses of various ROS-GC1 constructs; the precise structure of photoreceptor ROS-GC1 (e.g., X-ray-structure) is not yet available. At the intracellular domains of ROS-GC1, different NCS proteins bind at different locations. GCAP1 binds to the JMD, the aminoterminal portion of the kinase homology domain of ROS-GC1 probably via its aminoterminal EF1 hand. In contrast, S100β and GCAP2 bind close to each other to the catalytic domain. The binding of GCAPs appears to compete with the binding of the retinal degeneration protein 3 (RD3). While GCAPs inhibit mostly ROS-GC1 activity at high Ca2+ -concentrations, S100β stimulates ROS-GC1 activity at high Ca2+. The Ca2+-concentrations needed by S100β to stimulate ROS-GC1 activity is high but could be achieved at the active zone of photoreceptors close to presynaptic Ca2+-channels. The numbers below the schematic depiction of ROS-GC1 domains depict the respective borders in human ROS-GC1 sequence. Most of the mapping of the ROS-GC1 interacting proteins has been done with bovine ROS-GC1 reviewed in Sharma (2010). For some interactions (e.g., GCAP1), multiple interaction sites were reported. GCAP1 was also reported to bind to the catalytic domain though with lower affinity than at the KHD [for review, Sharma (2002, 2010)]. The respective amino acid regions of bovine ROS-GC1 involved in the interaction with the indicated proteins are indicated in square brackets. Non-photoreceptor-interacting proteins of ROS-GC1 [Sharma, (2010)] are not depicted. Abbreviations: TM, transmembrane domain; JMD, juxtamembrane domain; DD, dimerization domain; CTE, carboxyterminal extension; RD3, retinal degeneration 3. Proteins and protein domains are only schematically depicted and not drawn in scale.
GCAP1 binds to the juxtamembrane KHD of ROS-GCs (for review, see Koch et al., 2010). GCAP2 binds directly to the catalytic domain of ROS-GCs. Despite high sequence similarities, GCAPs are not functionally equivalent; many regulatory properties differ (for review, see Koch, 2006; Dizhoor et al., 2010; Koch et al., 2010). GCAP2 has a higher affinity for Ca2+ than GCAP1 (for review, see Koch, 2006; Dizhoor et al., 2010). Different Ca2+-affinities of GCAPs could enhance the operational range of Ca2+-regulation of GCs and give rise to the Ca2+-relay model of GC activation/inhibition in the OS (for review, see Koch, 2006; Burgoyne, 2007). At intermediate levels, Ca2+ is still bound to GCAP2 whereas GCAP1 is already Ca2+-free (Mg2+-bound version). As a consequence, GCAP1 would stimulate GC activity at these intermediate concentrations, whereas GCAP2 would still be inhibitory. Recently, it was found that the RD3 protein, which is associated with LCA, also binds to the carboxyterminal of ROS-GC and inhibits GC activity by an allosteric mechanism (Azadi et al., 2010; Peshenko et al., 2011b). RD3 binding to ROS-GCs promotes dissociation of GCAPs from the ROS-GC complex.
GCAPs in Photoreceptor Presynaptic Terminals and their Involvement in Activity-Dependent Changes of Synaptic Ribbons
Various studies demonstrated the presence of GCAP proteins in photoreceptor presynaptic terminals (Otto-Bruc et al., 1997; Kachi et al., 1999; Cuenca et al., 1998; Pennesi et al., 2003; Makino et al., 2008; Venkatesan et al., 2010). But the significance of GCAP proteins in the presynaptic terminals is not well understood. One function of GCAP-mediated signaling appears to mediate the Ca2+-dependent regulation of synaptic ribbon plasticity (Venkatesan et al., 2010). Synaptic ribbons are dynamic structures (for review, see Vollrath and Spiwoks-Becker, 1996; Schmitz, 2009). The synaptic ribbon undergoes activity- (illumination-) dependent changes. Illumination leads to smaller and less numerable synaptic ribbons in the mouse retina (Spiwoks-Becker et al., 2004). The dynamics of these structures is known to be dependent upon Ca2+ and cGMP (Vollrath and Spiwoks-Becker, 1996). Chelating intracellular Ca2+ leads to a disassembly of synaptic ribbons at the electron microscopic level (Spiwoks-Becker et al., 2004; Regus-Leidig et al., 2010). Immunocytochemical analyses of these effects revealed a sequential process (Regus-Leidig et al., 2010). First, synaptic ribbon components, such as RIBEYE, piccolo and RIM1, were removed, in parallel to the disassembly of synaptic ribbons at the ultrastructural level. In a second step, bassoon, an important mediator of synaptic ribbon stability and organizer of the active zone (Dick et al., 2003; for review, see Joselevitch and Zenisek, 2010; Regus-Leidig and Brandstätter, 2011), is removed from the active zone (Regus-Leidig et al., 2010). Venkatesan et al. (2010) demonstrated that RIBEYE, the main component of synaptic ribbons, binds to the carboxyterminal region of GCAP2 in a NAD(H)-dependent manner (Figure 5). Overexpression of GCAP2 in the presynaptic terminals of photoreceptors leads to disassembly of synaptic ribbons and a reduction in their number (Venkatesan et al., 2010). Therefore, one function of GCAP2 could be to regulate the assembly and disassembly of synaptic ribbons. The molecular mechanisms, how this could be achieved are currently unknown.
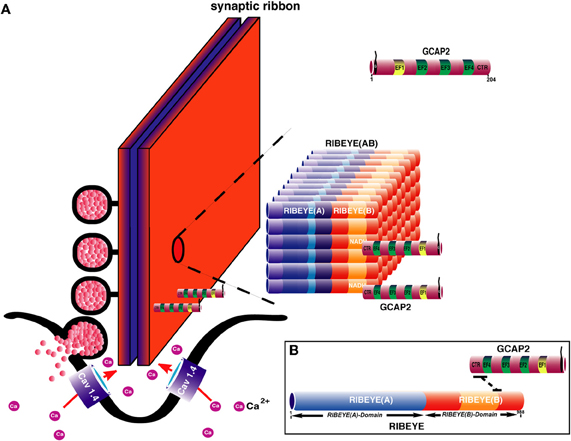
Figure 5. (A) Hypothetical model for the assembly of the synaptic ribbon: the scaffold of the synaptic ribbon is built by RIBEYE proteins, the major, and unique component of synaptic ribbons via multiple RIBEYE-RIBEYE interactions [Magupalli et al. (2008); Schmitz (2009)]. In this model, the A-domain is located in the center of the ribbon to build the core of the synaptic ribbon. The B-domain faces the cytoplasmic side of the synaptic ribbon where it interacts with various proteins, e.g., Munc119 (see also Figure 2) and with the GCAP2. Interaction with GCAP2 could regulate assembly and disassembly of synaptic ribbons which is known to be Ca2+-dependent [Vollrath and Spiwoks-Becker (1996); Schmitz (2009)]. Overexpression of GCAP2 leads to ribbon disassembly. The recruitment of GCAP2 by RIBEYE could influence Ca2+-buffering at the synaptic ribbon thus also influencing synaptic signaling. The differently colored portions in RIBEYE(A)-domain represent RIBEYE-RIBEYE interaction sites [Magupalli et al. (2008)]. How GCAP2 regulates ribbon assembly and disassembly is unknown but could involve GCAP effectors, e.g., ROS-GCs (Figure 4) which were reported to be present in the presynaptic terminals. (B) Molecular dissection of RIBEYE-GCAP2 interaction: the carboxyterminal region (CTR) of GCAP2 interacts with the hinge 2 region of RIBEYE(B) [Venkatesan et al. (2010)].
Which GCAP effectors in the synapse might execute its synaptic functions? ROS-GC1, the GCAP effector in the OS, has been localized to the photoreceptor synapses by immunoperoxidase methods and other sensitive techniques (Liu et al., 1994; Cooper et al., 1995; Duda et al., 2002). Conventional immunofluorescence microscopic analyses using mouse retina failed to detect ROS-GC1 in photoreceptor synapses (Azadi et al., 2010; Karan et al., 2010). This might be attributed to the lower sensitivity of immunofluorescence microscopy in comparison to immunoperoxidase techniques. Possibly, ROS-GC1 might be masked in the presynaptic matrix, not accessible to antibodies or the amount is close to the detection limits. Different antibodies with different affinities or species differences might also contribute to the different levels of immunoreactivities of ROS-GC1 in synaptic terminals. In the bovine retina, a strong ROS-GC1 was observed in photoreceptor terminals (Venkataraman et al., 2003). GC activity was demonstrated also histochemically in photoreceptor terminals (Rambotti et al., 2002). Biochemical data supported the presence of ROS-GC1 in photoreceptor synapses (Duda et al., 2002; Venkataraman et al., 2003). In contrast to the photoreceptor OS, ROS-GC1 in photoreceptor synaptic terminals is stimulated, not inhibited, by the presence of high concentrations of intracellular Ca2+ (Duda et al., 2002; Venkataraman et al., 2003; for review, see Sharma, 2002, 2010; Koch, 2006). The Ca2+-stimulated ROS-GC1 activity is mediated by the EF-hand protein S100β (previously also called CD-GCAP) that has been localized to the presynaptic photoreceptor terminal (Duda et al., 2002; Venkataraman et al., 2003; Sharma, 2010). S100β binds to the catalytic domain of ROS-GC and subsequently enhances ROS-GC1 activity at high Ca2+-concentrations. These Ca2+-concentrations could be achieved close to the synaptic ribbon (Choi et al., 2008; Jackman et al., 2009; Graydon et al., 2011). Thus, S100β binds to ROS-GC1 at the catalytic domain, similar to GCAP2 (Duda et al., 2002, 2005; Sharma, 2002, 2010). It is possible that S100β competes with GCAP2 for binding to ROS-GC1.
The regulation of cGMP levels could be the key in the regulation of activity-dependent synaptic ribbon plasticity. cGMP was reported to stabilize synaptic ribbons in the pineal gland (Seidel et al., 1990; Spessert et al., 1992). cGMP-dependent protein kinases could be effectors that might mediate the stabilizing effect of cGMP on synaptic ribbons. cGMP-dependent kinases have been localized to photoreceptor synapses (Feil et al., 2005). But the involvement of these kinases in ribbon dynamics has not yet been elucidated. Interestingly, the RD3 protein, which blocks binding of GCAP2 to ROS-GC1, is present in the presynaptic terminals (Azadi et al., 2010; Peshenko et al., 2011b). Thus, a complex interplay of several proteins that compete for binding to ROS-GCs modulates cGMP-dependent signaling in the photoreceptor synapse in a complex manner. The recruitment of GCAP2 to synaptic ribbons and the subsequent disassembly of synaptic ribbons could be due to changes in cGMP levels that induce further downstream effects or due to increased GCAP2-mediated Ca2+-buffering. Future investigations have to discriminate between these possibilities. The importance of cGMP and cGMP-dependent protein kinases for synaptic ribbon dynamics is supported by a recent study that showed a synaptic ribbon-protective effect of cGMP in an inner ear trauma model (Jaumann et al., 2012). In this study, the authors demonstrated that inhibition of cGMP-hydrolyzing PDE5 leads to stabilization of synaptic ribbons in a cGMP-regulated protein kinase 1-dependent manner in inner hair cells. Analyses of GCAP1/2 double knockout mice also pointed to a synaptic function of GCAPs proteins at the photoreceptor synapse (Okawa et al., 2010). GCAP1/2 knockout mice show disturbed signal processing at the synapse: although the single-photon-responses in OS of GCAP knockout mice were much larger than in wildtype mice, the synaptic processing of this information, as measured by recordings from postsynaptic bipolar cells, was more inefficient. A main synaptic function of GCAPs appears to improve the signal-to-noise ratio of synaptic transmission (Okawa et al., 2010). The underlying molecular mechanisms are still unknown but could involve structural changes of the synapse.
cGMP is an Important Modulator of Synaptic Plasticity in Photoreceptor Terminals
Various other aspects of plasticity in photoreceptor presynaptic terminals are mediated by cGMP (Rieke and Schwartz, 1994; Vollrath and Spiwoks-Becker, 1996; Savchenko et al., 1997; Zhang and Townes-Anderson, 2002; Zhang et al., 2005). The group of Townes-Anderson showed that outgrowth of neurites in rods and cones photoreceptor depends upon influx of Ca2+ (for review, see Townes-Anderson and Zhang, 2006). In cones, Ca2+ enters the presynaptic terminal through cGMP-gated Ca2+-channels to mediate this type of synaptic plasticity. Hyperpolarization-activated, cyclic nucleotide-gated (HCN) channels could be further effectors of presynaptic cGMP. HCN1 channels have been demonstrated in presynaptic photoreceptor terminals (Müller et al., 2003; Knop et al., 2008; Seeliger et al., 2011; Tanimoto et al., 2012). cGMP-regulated channels could extend the range of synaptic transmission e.g., at very negative membrane potentials at which L-type calcium channels might already be closed (Rieke and Schwartz, 1994; Savchenko et al., 1997). Soluble GCs could also contribute to the generation of cGMP. Several studies suggest that this source of cGMP production could play a role in neurotransmitter release and structural plasticity in photoreceptor terminals (Savchenko et al., 1997; Kourennyi et al., 2004; Zhang et al., 2005; Blom et al., 2009; Sato et al., 2011).
Imbalance of cGMP and Ca2+-Homeostasis in Photoreceptors Leads to Disease
As described above, cGMP and Ca2+ homeostasis are intimately related and possess a central role for phototransduction and light-adaptation. Tight control of cGMP and Ca2+-levels are of central importance for the survival of photoreceptors (Hunt et al., 2010). Various severe neurodegenerative diseases of the retina are associated with disturbances of the cGMP/Ca2+-homeostasis (Fain, 2006; Barabas et al., 2010; Paquet-Durand et al., 2011). These include Retinitis pigmentosa (RP), LCA, and distinct forms of cone and rod dystrophies (Baehr and Palczewski, 2009; Jiang and Baehr, 2010; Paquet-Durand et al., 2011). Mutations in the ROS-GC1 gene can lead to LCA, a devastating degeneration leading to childhood blindness, or a cone-rod-dystrophy (CORD 6) (for review, see Hunt et al., 2010). Diseases associated with ROS-GC2 are not known. The gene for GCAP1 has been associated with a form of cone-rod dystrophy, CORD3 (for review, see Jiang and Baehr, 2010). Missense mutations in GCAP1 cause loss of photoreceptors, particularly cones. Many of the disease-causing mutations are located in EF3 and EF4 or indirectly affect the structure of these EF-hands. The disease mutants lead to a decrease in Ca2+-sensitivity thus making these mutants to constitutive, Ca2+-insensitive activators of GCs. As a result, cGMP and Ca2+ levels are pathologically increased leading to photoreceptor cell death (Baehr and Palczewski, 2009; Jiang and Baehr, 2010; Paquet-Durand et al., 2011). The retinal degeneration 1 (rd1) mouse is characterized by a loss-of-function mutation in the gene encoding for the β-subunit of the photoreceptor-specific PDE6 (for a recent review, see Barabas et al., 2010). Consequently, rd1 mice have low PDE6 activity and high levels of cGMP which lead to photoreceptor cell death, predominantly in rods. Also the proteins discussed above, i.e., CaV1.4, Munc119, RIM, and CaBP4, have high clinical relevance; mutations in the respective genes cause various severe degenerative diseases of the retina, as described above.
Open Questions/Perspectives
Activity-dependent, adaptative signaling in photoreceptor presynaptic terminals is just at the beginning of being understood. Currently, knowledge about these processes in the synapse lags behind to what is known about dynamic processes in the OS. Ca2+, cGMP, and EF-hand-containing proteins likely play numerous roles in signaling at the photoreceptor synapse and activity-dependent synaptic changes. Dynamics of synaptic ribbons at a molecular level may involve control of RIBEYE-RIBEYE interactions. How these interactions are controlled at a molecular level is currently not known. The involved effector molecules and molecular pathways need to be elucidated. Differences between rod and cone dynamic signaling need to be worked out since the purpose of synaptic transmission at these two different types of photoreceptor synapses is different (although related). Are there differences in adaptative signaling in cone and rod synapses and eventually also between the different active zones present in cone synapses? Recent Ca2+-imaging analyses strongly argue that this is the case (Johnson et al., 2007; Sheng et al., 2007). Most of our current knowledge about the physiology of retinal ribbon synapses was obtained from goldfish bipolar cells and salamander photoreceptors. The mouse retina with its powerful genetic possibilities just entered the stage. Mouse knockout models as well as the possibility of manipulating the mouse retina with recombinant viruses can be expected to provide further important insights into signal processing at the photoreceptor synapse.
Conflict of Interest Statement
The authors declare that the research was conducted in the absence of any commercial or financial relationships that could be construed as a potential conflict of interest.
Acknowledgments
Work by the authors is supported by the German Research Community (DFG) SFB894 TPA7, GRK1326 and the Human Frontiers Science Organization HFSP. Thanks to Dr. Jutta Schmitz-Kraemer for critically reading the manuscript. The authors apologize that not all relevant original papers could be mentioned due to limitations in space.
Abbreviations
NCS, neuronal Ca2+-sensor proteins; ROS-GC rod outer segment guanylate cyclase; GC, guanylate cyclase; OS, outer segments; IS, inner segments; OPL, outer plexiform layer (containing photoreceptor ribbon synapses); PDE6, cGMP phosphodiesterase 6; CNG, cyclic nucleotide-gated; CNG channel, cyclic nucleotide-gated channel; HCN channel, hyperpolarization-activated, cyclic nucleotide-gated channel; LTCC, L-type calcium channels; VGCC, voltage-gated calcium channels; CSNB, congenital stationary night blindness; GCAP, guanylate cyclase-activating protein; [Ca2+]i cytoplasmic concentration of free Ca2+; ER, endoplasmic reticulum; CDI, calcium-dependent inactivation; VDI, voltage-dependent inactivation; KHD, kinase homology domain; CTR, carboxy-terminal region; LCA, Leber congenital amaurosis; CORD, cone-rod dystrophy; ON-bipolar cells, bipolar cells that depolarize in response to illumination; OFF-bipolar cells, bipolar cells that hyperpolarize in response to illumination; ERG, electroretinogram; KO, knockout; SIM, structured illumination microscopy.
References
Abramov, I., and Gordon, J. (1994). Color appearance: on seeing red- or yellow, or green, or blue. Ann. Rev. Psychol. 45, 451–485.
Aldahmesh, M. A., Al-Owain, M., Alqahtani, F., Hazzaa, S., and Alkurya, F. S. (2010). A null mutation in CaBP4 causes Leber's congenital amaurosis-like phenotype. Mol. Vis. 16, 207–212.
Alpadi, K., Magupalli, V. G., Käppel, S., Köblitz, L., Schwarz, K., Seigel, G. M., Sung, C. H., and Schmitz, F. (2008). RIBEYE recruits Munc119, the mammalian ortholog of the Caenorhabditis elegans protein unc119 to synaptic ribbons of photoreceptor synapses. J. Biol. Chem. 283, 26461–26467.
Ames, J. B., Dizhoor, A. M., Ikura, M., Palczewski, K., and Stryer, L. (1999). Three-dimensional structure of guanylyl cyclase activating protein-2, a calcium-sensitive modulator of photoreceptor guanylyl cyclases. J. Biol. Chem. 274, 19329–19337.
Ames, J. B., and Lim, S. (2011). Molecular structure and target recognition of neuronal calcium sensor proteins Biochim. Biophys. Acta [Epub ahead of print].
Azadi, S., Molday, L. L., and Molday, R. S. (2010). RD3, the protein associated with Leber congenital amaurosis type 12, is required for guanylate cyclase trafficking in photoreceptor cells. Proc. Natl. Acad. Sci. U.S.A. 107, 21158–21163.
Babai, N., Morgans, C. W., and Thoreson, W. B. (2010). Calcium-induced calcium release contributes to synaptic release from mouse rod photoreceptors. Neuroscience 165, 1447–1456.
Baehr, W., and Palczewski, K. (2007). Guanylate-cyclase-activating proteins and retina disease. Subcell. Biochem. 45, 71–91.
Baehr, W., and Palczewski, K. (2009). Focus on molecules: guanylate cyclase-activating proteins (GCAPs). Exp. Eye Res. 89, 2–3.
Balkema, G. W., Cusick, K., and Nguyen, T. H. (2001). Diurnal variation in synaptic ribbon length and visual threshold. Vis. Neurosci. 18, 789–792.
Ball, S. L., McEnery, M. W., Yunker, A. M., Shin, H. S., and Gregg, R. G. (2011). Distribution of voltage gated calcium channel β subunits in the mouse retina. Brain Res. 1412, 1–8.
Ball, S. L., Powers, P. A., Shin, H. S., Morgans, C. W., Peachey, N. S., and Gregg, R. G. (2002). Role of the β2 subunit of voltage-dependent Ca2+ channels in the retinal outer plexiform layer. Invest. Ophthalmol. Vis. Sci. 43, 1595–1603.
Barabas, P., Peck, C. C., and Krizaj, D. (2010). Do calcium channel blockers rescue dying photoreceptors in the Pde6brd1 mouse? Adv. Exp. Med. Biol. 664, 491–499.
Beutner, D., Voets, T., Neher, E., and Moser, T. (2001). Calcium dependence of exocytosis and endocytosis at the cochlear inner hair cell afferent synapse. Neuron 29, 681–690.
Biel, M., and Michalakis, S. (2009). Cyclic nucleotide-gated channels. Handb. Exp. Pharamacol. 191, 111–136.
Blom, J. J., Blute, T. A., and Eldred, W. D. (2009). Functional localization of the nitric oxide/cGMP pathway in the salamander retina. Vis. Neurosci. 26, 275–286.
Buch, P. K., Mihelec, M., Cottrill, P., Wilkie, S. E., Pearson, R. A., Duran, Y., West, E. L., Michaelides, M., and Hunt, D. M. (2011). Dominant cone-rod dystrophy: a mouse model generated by gene targeting of the GCAP1/Guca1a gene. Plos One 3, 18089. doi: 10.1371/journal.pone.0018089
Burgoyne, R. D. (2007). Neuronal calcium sensor proteins: generating diversity in neuronal Ca2+-signalling. Nat. Rev. Neurosci. 8, 182–193.
Burns, M. E., and Baylor, D. A. (2001). Activation, deactivation, and adaptation in vertebrate photoreceptor cells. Ann. Rev. Neurosci. 24, 779–805.
Catterall, W. A., Perez-Reyes, E., Snutch, T. P., and Striessnig, J. (2005). International union of pharmacology. XLVIII. Nomenclature and structure-function relationships of voltage-gated calcium channels. Pharmacol. Rev. 57, 411–425.
Chen, K. (2005). The vertebrate phototransduction cascade: amplification and termination mechanisms. Rev. Physiol. Biochem. Pharmacol. 154, 101–121.
Choi, S. Y., Jackman, S., Thoreson, W. B., and Kramer, R. H. (2008). Light regulation of Ca2+ in the cone photoreceptor synaptic terminal. Vis. Neurosci. 25, 693–700.
Cooper, N., Liu, N., Yoshida, A., Pozdnyakov, N., Margulies, A., and Sitaramayya, A. (1995). The bovine rod outer segment guanylate cyclase, ROS-GC, is present in both outer segment and synaptic layers of the retina. J. Mol. Neurosci. 6, 211–222.
Cuenca, N., Lopez, S., Howes, K., and Kolb, H. (1998). The localization of guanylyl cyclase-activating proteins in the mammalian retina. Invest. Ophthalmol. Vis. Sci. 39, 1249–1250.
Davies, A., Hendrich, J., Van Minh, A. T., Wratten, J., Douglas, L., and Dolphin, A. C. (2007). Functional biology of the alpha(2)delta subunits of voltage-gated calcium channels. Trends Pharmacol. Sci. 28, 220–228.
Davies, A., Kadurin, I., Alvarez-Laviada, A., Douglas, L., Nieto-Rostro, M., Bauer, C. S., Pratt, W. S., and Dolphin, A. C. (2010). The α2δ subunits of voltage-gated calcium channels form GPI-anchored proteins, a posttranslational modification essential for function. Proc. Natl. Acad. Sci. U.S.A. 107, 1654–1659.
DeVries, S. H., Li, W., and Saszik, S. (2006). Parallel processing in two transmitter microenvironments at the cone photoreceptor synapse. Neuron 50, 735–748.
Dick, O., tom Dieck, S., Altrock, W. D., Ammermüller, J., Weiler, R., Garner, C. C., Gundelfinger, E. D., and Brandstätter, J. H. (2003). The presynaptic active zone protein bassoon is essential for photoreceptor ribbon synapse formation in the retina. Neuron 37, 775–786.
Dizhoor, A. M., Olshevskaya, E. V., and Peshenko, I. V. (2010). Mg2+/Ca2+ cation binding cycle of guanylyl cyclase activating proteins (GCAPs): role in the regulation of photoreceptor guanylyl cyclase. Mol. Cell. Biochem. 334, 117–124.
Dolphin, A. C. (2003). β subunits of voltage-gated calcium channels. J. Bioenerg. Biomembr. 35, 599–620.
Duda, T., Fik-Rymarkiewicz, E., Venkataraman, V., Krishnan, R., Koch, K. W., and Sharma, R. K. (2005). The calcium-sensor guanylate cyclase activating protein type 2 specific site in rod outer segment membrane guanylate cyclase type 1. Biochemistry 44, 7336–7345.
Duda, T., Koch, K. W., Venkataraman, V., Lange, C., Beyermann, M., and Sharma, R. K. (2002). Ca2+-sensor S100β-modulated sites of membrane guanylate cyclase in the photoreceptor-bopolar synapse. EMBO J. 21, 2547–2556.
Emran, F., Rihel, J., Adolph, A. R., and Dowling, J. E. (2010). Zebrafish larvae lose vision at night. Proc. Natl. Acad. Sci. U.S.A. 107, 6034–6039.
Ermilov, A. N., Olshevskaya, E. V., and Dizhoor, A. M. (2001). Instead of binding calcium, one of the EF-hand structures in guanylyl cyclase activating protein 2 is required for targeting photoreceptor guanylyl cyclase. J. Biol. Chem. 51, 48143–48148.
Feil, S., Zimmermann, A., Knorn, A., Brummer, S., Schlossmann, J., Hofmann, F., and Feil, R. (2005). Distribution of cGMP-dependent protein kinase type I and its isoform in the mouse brain and retina. Neuroscience 135, 863–868.
Frank, T., Khimich, D., Neef, A., and Moser, T. (2009). Mechanisms contributing to synaptic Ca2+ signals and their heterogeneity in hair cells. Proc. Natl. Acad. Sci. U.S.A. 106, 4483–4488.
Frank, T., Rutherford, M. A., Strenzke, N., Neef, A., Pangrsic, T., Khimich, D., Fejtova, D., Gundelfinger, E. D., Liberman, M. C., Harke, B., Bryan, K. E., Lee, A., Egner, A., Riedel, D., and Moser, T. (2010). Bassoon and the synaptic ribbon organize Ca2+-channels and vesicles to add release sites and promote refilling. Neuron 68, 724–738.
Gebhart, M., Juhasz-Vedres, G., Zuccotti, A., Brandt, N., Engel, J., Trockenbacher, A., Kaur, G., Obermair, G. J., Knipper, M., Koschak, A., and Striessnig, J. (2010). Modulation of CaV1.3 channel gating by Rab3 interacting molecule. Mol. Cell. Neurosci. 44, 246–259.
Graydon, C. W., Cho, S., Li, G. L., Kachar, B., and von Gersdorff, H. (2011). Sharp Ca2+ nanodomains beneath the ribbon promote highly synchrous multivesicular release at hair cell synapses. J. Neurosci1. 31, 16637–16650.
Haeseleer, F., Imanishi, Y., Maeda, T., Possin, D. E., Maeda, A., Lee, A., Rieke, F., and Palczewski, K. (2004). Essential role of Ca2+-binding protein 4, a CaV1.4 channel regulator, in photoreceptor synaptic function. Nat. Neurosci. 7, 1079–1087.
Haeseleer, F., Imanishi, Y., Sokal, I., Filipek, S., and Palzewski, K. (2002). Calcium-binding proteins: intracellular sensors from the calmodulin superfamily. Biochem. Biophys. Res. Commun. 290, 615–623.
Haeseleer, F. (2008). Interaction and colocalization of CaBP4 and unc119 (MRG4) in photoreceptors. Invest. Ophthalmol. Vis. Sci. 49, 2366–2375.
Haire, S. E., Pang, J., Boye, S. L., Sokal, I., Craft, C. M., Palczewski, K., Hauswirth, M. M., and Semple-Rowland, S. L. (2006). Light-driven cone arrestin translocation in cones of postnatal guanylate cyclase-1 knockout mouse retina treated with AAV-GC1. Invest. Ophthalmol. Vis. Sci. 47, 3745–3753.
Han, Y., Kaeser, P. S., Südhof, T. C., and Schneggenburger, R. (2011). RIM determines Ca2+ channel density and vesicle docking at the presynaptic active zone. Neuron 69, 304–316.
Heidelberger, R., Thoreson, W. B., and Witkovsky, P. (2005). Synaptic transmission at retinal ribbon synapses. Prog. Retin. Eye Res. 24, 682–720.
Hibino, H., Pironkova, R., Onwumere, O., Vologodskaia, M., Hudspeth, A. J., and Lesage, F. (2002). RIM binding proteins (RBPs) couple Rab3-interacting molecules (RIMs) to voltage-gated Ca2+-channels. Neuron 34, 411–423.
Hull, C., Studholme, K., Yazulla, S., and von Gersdorff, H. (2006). Diurnal changes in exocytosis and the number of synaptic ribbons at active zones of an ON-type bipolar cell terminal. J. Neurophysiol. 96, 2025–2033.
Hunt, D. M., Buch, P., and Michaelidis, M. (2010). Guanylate cyclases and associated activator proteins in retinal disease. Mol. Cell. Biochem. 334, 157–168.
Hwang, J.-Y., Schlesinger, R., and Koch, K. W. (2004). Irregular dimerization of guanylate cyclase-activating protein 1 mutants causes loss of target activation. Eur. J. Biochem. 271, 3785–3793.
Jackman, S. L., Babai, N., Chambers, J. J., Thoreson, W. B., and Kramer, R. H. (2010). A positive feedback synapse from horizontal cells to cone photoreceptors. Plos Biol. 9, e1001057. doi: 10.1371/journal.pbio.1001057
Jackman, S. L., Choi, S. Y., Thoreson, W. B., Rabl, K., Bartoletti, T. M., and Kramer, R. H. (2009). Role of the synaptic ribbon in transmitting the cone light response. Nat. Neurosci. 12, 303–310.
Jarsky, T., Tian, M., and Singer, J. H. (2010). Nanodomain control of exocytosis is responsible for the signalling capability of a retinal ribbon synapse. J. Neurosci. 30, 11885–11895.
Jaumann, M., Dettling, J., Gubelt, M., Zimmermann, U., Gerling, A., Paquet-Durand, F., Feil, S., Wolpert, S., Franz, C., Varakina, K., Xiong, H., Brandt, N., Kuhn, S., Geisler, H. S., Rohbock, K., Ruth, P., Schlossmann, J., Hütter, J., Sandner, P., Feil, R., Engel, J., Knipper, M., and Rüttiger, L. (2012). cGMP-Prgk1 signaling and Pde5 inhibition shelter cochlear hair cells and hearing function. Nat. Med. 18, 252–259.
Jiang, L., and Baehr, W. (2010). GCAP1 mutations associated with autosomal dominant cone dystrophy. Adv. Exp. Med. Biol. 664, 273–282.
Jiang, L., Katz, B. J., Yang, Z., Zhao, Y., Faulkner, N., Hu, J., Baird, J., Baehr, W., Creel, D. J., and Zhang, K. (2005). Autosomal dominant cone dystrophy caused by a novel mutation in the GCAP1 gene (Guca1A). Mol. Vis. 11, 143–151.
Johnson, J. E. Jr., Perkins, G. A., Anand Giddabasappa, C. S., Chaney, S., Xiao, W., White, A. D., Brown, J. M., Waggoner, J., Ellisman, M. H., and Fox, D. A. (2007). Spatiotemporal regulation of ATP and Ca2+ dynamics in vertebrate rod and cone ribbon synapses. Mol. Vis. 13, 887–919.
Johnson, S. L., Forge, A., Knipper, M., Münkner, S., and Marcotti, W. (2008). Tonotopic variation in the calcium dependence of neurotransmitter release and vesicle pool replenishment at mammalian auditory ribbon synapses. J. Neurosci. 28, 7670–7678.
Joselevitch, C., and Zenisek, D. (2010). The cytomatrix protein bassoon contributes to fast transmission at conventional and ribbon synapses. Neuron 68, 604–606.
Kachi, S., Nishizawa, Y., Olshevskaya, E., Yamazaki, A., Miyake, Y., Wakabashi, T., Dizhoor, A. M., and Usukura, J. (1999). Detailed localization of photoreceptor guanylate cyclase activating protein-1 and -2 in mammalian retinas using light and electron microscopy. Exp. Eye Res. 68, 465–473.
Kaeser, P. S., Deng, L., Wang, Y., Dulubova, I., Liu, X., Rizo, J., and Südhof, T. C. (2011).RIM tethers Ca2+ channels to presynaptic active zones via a direct PDZ-domain interaction. Cell 144, 282–295.
Karan, S., Frederick, J. M., and Baehr, W. (2010). Novel functions of photoreceptor guanylate cyclases revealed by targeted deletion. Mol. Cell. Biochem. 334, 141–155.
Kersten, F. J. K., van Wijk, E., van Reenwijk, J., van der Zwaag, B., Märker, T., Peters, T. A., Katsanis, N., Wolfrum, U., Keunen, J. E. E., Roepman, R., and Kremer, H. (2010). Association of whirlin with CaV1.3(α1D) channels in photoreceptors, defining a novel member of the Usher protein network. Invest. Ophthalmol. Vis. Sci. 51, 2338–2346.
Khimich, D., Nouvian, R., Pujol, R., tom Dieck, S., Egner, A., Gundelfinger, E. D., and Moser, T. (2005). Hair cell synaptic ribbons are essential for synchronous auditory signalling. Nature 434, 889–894.
Kiyonaka, S., Wakamori, M., Miki, T., Uriu, Y., Nonaka, M., Bito, H., Beedle, A. M., Mori, E., Hara, Y., De Waard, M., Kanagawa, M., Itakura, M., Takahashi, M., Campbell, K. P., and Mori, Y. (2007). RIM1 confers sustained activity and neurotransmitter vesicle anchoring to presynaptic Ca2+-channels. Nat. Neurosci. 10, 691–701.
Knop, G. C., Seeliger, M. W., Thiel, F., Mataruga, A., Kaupp, U. B., Friedburg, C., Tanimoto, N., and Kaupp, U. B. (2008). Light responses in the mouse retina are prolonged upon targeted deletion of the HCN1 channel gene. Eur. J. Neurosci. 28, 2221–2230.
Ko, M. L., Liu, Y., Dryer, S. E., and Ko, G. Y. (2007). The expression of L-type voltage-gated calcium channels in retinal photoreceptors is under circadian control. J. Neurochem. 103, 784–792.
Kobayashi, A., Higashide, T., Hamasaki, D., Kubota, S., Sakuma, A., An, W., Fujimaki, T., McLaren, M. J., Weleber, R. G., and Inana, G. (2000). HRG4 (UNC119) mutation found in cone-rod dystrophy causes retinal degeneration in a transgenic model. Invest. Ophthalmol. Vis. Sci. 41, 3268–3277.
Koch, K. W. (2006). GCAPs, the classical neuronal calcium sensors in the retina. In: Calcium binding proteins. Landes Biosci. 1, 3–6.
Koch, K. W., Duda, T., and Sharma, R. K. (2010). Ca2+-modulated vision-linked ROS-GC guanylate cyclase transduction machinery. Mol. Cell. Biochem. 334, 105–115.
Koike, C., Numata, T., Ueda, H., Mori, Y., and Furukawa, T. (2010), TRPM1: a vertebrate TRP channel responsible for retinal ON bipolar function. Cell Calcium 48, 95–101.
Kourennyi, D. E., Liu, X. D., Hart, J., Mahmud, F., Baldridge, W. H., and Barnes, S. (2004). Reciprocal modulation of calcium dynamics at rod and cone photoreceptor synapses by nitric oxide. J. Neurophysiol. 92, 477–483.
Krizaj, D., and Copenhagen, D. R. (2002). Calcium regulation in photoreceptors. Front. Biosci. 7, 2023–2044.
Lange, C., Duda, T., Beyermann, M., Sharma, R. K., and Koch, K.-W. (1999). Regions in vertebrate photoreceptor guanylyl cyclase ROS-GC1 involved in Ca2+-dependent regulation by guanylyl cyclase-activating protein GCAP-1. FEBS Lett. 460, 27–31.
Liberman, L. D., Wang, H., and Liberman, M. C. (2011). Opposing gradients of ribbon size and AMPA receptor expression underlie sensitivity differences among cochlear-nerve/hair-cell synapses. J. Neurosci. 31, 801–808.
Liu, X., Seno, K., Nishizawa, Y., Hayashi, F., Yamazaki, A., Matsumoto, H., Wakabayashi, T., and Usukura, J. (1994). Ultrastructural localization of retinal guanylate cyclase in human and monkey retina. Exp. Eye Res. 59, 761–768.
Magupalli, V. G., Schwarz, K., Alpadi, K., Natarajan, S., Seigel, G. M., and Schmitz, F. (2008). Multiple RIBEYE – RIBEYE interactions create a dynamic scaffold for the formation of synaptic ribbons. J. Neurosci. 28, 7954–7967.
Makino, C. L., Peshenko, I. V., Wen, X. H., Olshevskaya, E. V., Barrett, R., and Dizhoor, A. M. (2008). A role for GCAP2 in regulating the photoreceptor response: guanylyl cyclase activation and rod physiology in GUCA1B knockout mice. J. Biol. Chem. 283, 29135–29143.
Matthews, G., and Fuchs, P. (2010). The diverse roles of ribbon synapses in sensory neurotransmission. Nat. Rev. Neurosci. 11, 812–822.
Mercer, A. J., Chen, M., and Thoreson, W. B. (2011a). Lateral mobility of presynaptic L-type calcium channels at photoreceptor ribbon synapses. J. Neurosci. 31, 4397–4406.
Mercer, A. J., and Thoreson, W. B. (2011b). The dynamic architecture of photoreceptor ribbon synapses: cytoskeletal, extracellular matrix, and intramembrane proteins. Vis. Neurosci. 28, 453–471.
Miki, T., Kiyonaka, S., Uriu, Y., deWaard, M., Wakamori, M., Beedle, A. M., Campbell, K., and Mori, Y. (2007). Mutation associated with an autosomal dominant cone-rod dystrophy CORD7 modifies RIM1-mediated modulation of voltage-gated Ca2+-channels. Channels 1, 144–147.
Morgans, C. W. (2001). Localization of the α1F calcium channel subunit in the rat retina. Invest. Ophthalmol. Vis. Sci. 42, 2414–2418.
Morgans, C. W., Bayley, P. R., Oesch, N., Ren, G., Akileswaran, L., and Taylor, W. R. (2005). Photoreceptor calcium channels: insight from night blindness. Vis. Neurosci. 22, 561–568.
Morgans, C. W., Brown, R. L., and Duvoisin, R. M. (2010). TRPM1: an endpoint of the mGluR6 signal transduction cascade in retinal ON-bipolar cells. Bioesssays 32, 609–614.
Müller, F., Scholten, A., Ivanova, E., Haverkamp, S., Kremmer, E., and Kaupp, U. B. (2003). HCN channels are expressed differentially in retinal bipolar cells and concentrated at synaptic terminals. Eur. J. Cell Biol. 17, 2084–2096.
Nachman-Clewner, M., St Jules, R., and Townes-Anderson, E. (1999). L-type calcium channels in the photoreceptor synapse: localization and role in synaptic plasticity. J. Comp. Neurol. 415, 1–16.
Neher, E., and Sakaba, T. (2008). Multiple roles of calcium ions in the regulation of neurotransmitter release. Neuron 59, 861–872.
Okawa, H., Miyagishima, J., Arman, A. C., Hurley, J. B., Field, G. F., and Sampath, A. P. (2010). Optimal processing of photoreceptor signals is required to maximize behavioural sensitivity. J. Physiol. 588, 1947–1960.
Olshevskaya, E. V., Ermilov, A. N., and Dizhoor, A. M. (2002). Factors that affect regulation of cGMP synthesis in vertebrate photoreceptors and their genetic link to human retinal degeneration. Mol. Cell. Biochem. 230, 139–147.
Otto-Bruc, A., Fariss, R. N., Haeseleer, F., Huang, J., Buczylko, J., Surgocheva, I., Baehr, W., Milam, A. H., and Palczewski, K. (1997). Localization of guanylate cyclase-activating protein 2 in mammalian retina. Proc. Natl. Acad. Sci. U.S.A. 94, 4727–4732.
Pahlberg, J., and Sampath, A. P. (2011). Visual thresholds is set by linear and nonlinear mechanisms: how neural circuits in the retina improve the signal-to-noise ratio of the single photon response. Bioessays 33, 438–447.
Paquet-Durand, F., Beck, S., Michalakis, S., Goldmann, T., Huber, S., Mühlfriedel, R., Trifunovic, D., Fischer, M. D., Fahl, E., Duetsch, G., Becirovic, E., Wolfrum, U., van Veen, T., Biel, M., Tanimoto, N., and Seeliger, M. W. (2011). A key role for cyclic nucleotide gated (CNG) channels in cGMP related retinitis pigmentosa. Hum. Mol. Genet. 20, 941–947.
Palczewski, K., Sokal, I., and Baehr, W. (2004). Guanylate cyclase-activating proteins: structure, function and diversity. Biochem. Biophys. Res. Comm. 322, 1123–1130.
Pennesi, M. E., Howes, K. A., Baehr, W., and Wu, S. M. (2003). Guanylate cyclase activating protein (GCAP1) 1 rescues cone recovery kinetics in GCAP1/GCAP2 knockout mice. Proc. Natl. Acad. Sci. U.S.A. 100, 6783–6788.
Peshenko, I. V., Olshevskaya, E. V., Savchenko, A. B., Karan, S., Palczewski, K., Baehr, W., and Dizhoor, A. M. (2011a). Enzymatic properties and regulation of the native isozymes of retinal membrane guanylyl cyclase (RetGC) from mouse photoreceptors. Biochemistry 50, 5590–5600.
Peshenko, I. V., Olshevskaya, E. V., Azadi, S., Molday, L. L., Molday, R. S., and Dizhoor, A. M. (2011b). Retinal degeneration 3 (RD3) protein inhibits catalytic activity of retinal membrane guanylyl cyclase (RetGC) and its stimulation by activating proteins. Biochemistry 50, 9511–9519.
Potter, L. R. (2011). Guanylyl cyclase structure, function and regulation. Cell Signal. 23, 1921–1926.
Rambotti, M. G., Spreca, A., Giambanco, I., Sorci, G., and Donato, R. (2002). Ultracytochemistry as a tool for the study of the cellular and subcellular localization of membrane-bound guanylate cyclases (GC) activity. Applicability to both receptor-activated and receptor-independent GC activity. Mol. Cell. Biochem. 230, 85–96.
Regus-Leidig, H., and Brandstätter, J. H. (2011). Structure and function of a complex sensory synapse. Acta Physiol. (Oxf) [Epub ahead of print].
Regus-Leidig, H., Specht, D., tom Dieck, S., and Brandstätter, J. H. (2010). Stability of active zone components at the photoreceptor ribbon complex. Mol. Vis. 16, 2690–2770.
Rieke, F., and Schwartz, E. A. (1994). A cGMP-gated current can control exocytosis at cone synapses. Neuron 13, 863–873.
Sakurai, K., Chen, J., and Kefalov, V. J. (2011). Role of guanylyl cyclase modulation in mouse cone phototransduction. J. Neurosci. 22, 7991–8000.
Sato, M., Ohtsuka, T., and Stell, W. K. (2011). Endogenous nitric oxide enhances the light-response of cones during light-adaptation in the rat retina. Vis. Res. 51, 131–137.
Savchenko, A., Barnes, S., and Kramer, R. H. (1997). Cyclic-nucleotide-gated channels mediate synaptic feedback by nitric oxide. Nature 390, 694–698.
Schmitz, F. (2009). The making of synaptic ribbons: how they are built and what they do. Neuroscientist 15, 611–624.
Schmitz, F., Königstorfer, A., and Südhof, T. C. (2000). RIBEYE, a component of synaptic ribbons: a protein's journey through evolution provides insights into synaptic ribbon function. Neuron 28, 857–872.
Seeliger, M. W., Brombas, A., Weiler, R., Humphries, P., Knop, G., Tanimoto, N., and Müller, F. (2011). Modulation of rod photoreceptor output by HCN1 channels is essential for regular mesopic cone vision. Nat. Commun. 2, 532.
Seidel, A., Kantarjian, A., and Vollrath, L. (1990). A possible role for cyclic guanosine monophosphate in the rat pineal gland. Neurosci. Lett. 110, 227–231.
Sharma, R. K. (2002). Evolution of the membrane guanylate cyclase transduction system. Mol. Cell. Biochem. 230, 3–30.
Sharma, R. K. (2010). Membrane guanylate cyclase is a beautiful signal transduction machinery. Mol. Cell. Biochem. 334, 3–36.
Sheets, L., Trapani, J. G., Mo, W., Obholzer, N., and Nicolson, T. (2011). RIBEYE is required for presynaptic Ca(V)1.3 channel localization and afferent innervation of sensory hair cells. Development 138, 1309–1319.
Sheng, Z., Choi, S. Y., Dharia, A., Li, J., Sterling, P., and Kramer, R. H. (2007). Synaptic Ca2+ in darkness is lower in rods than in cones, causing slower tonic release of vesicles. J. Neurosci. 27, 5033–5042.
Singer, J. H., Lassová, L., Vardi, N., and Singer, J. S. (2004). Coordinated multivesicular release at a mammalian ribbon synapse. Nat. Neurosci. 7, 826–833.
Singh, A., Hamedinger, D., Hoda, J. C., Gebhart, M., Koschak, A., Romanin, C., and Striessnig, J. (2006). C-terminal modulator controls Ca2+-dependent gating of Ca(v)1.4 L-type Ca2+ channels. Nat. Neurosci. 9, 1108–1116.
Snellman, J., Mehta, B., Babai, N., Bartoletti, T. M., Akmentin, W., Francis, A., Matthews, G., Thoreson, W. B., and Zenisek, D. (2011). Acute destruction of the synaptic ribbon reveals a role for the ribbon in vesicle priming. Nat. Neurosci. 14, 1135–1141.
Spessert, R., Gupta, B. B. P., Seidel, A., Maitra, S. K., and Vollrath, L. (1992). Involvement of cyclic guanosine monophosphate (cGMP) and cytosolic guanylate cyclase in the regulation of synaptic ribbon numbers in the rat pineal gland. Brain Res. 570, 231–236.
Spiwoks-Becker, I., Glas, M., Lasarzik, I., and Vollrath, L. (2004). Synaptic ribbons lose and regain material in response to illumination changes. Eur. J. Neurosci. 19, 1559–1571.
Stephen, R., Bereta, G., Golczak, M., Palczewski, K., and Souza, M. C. (2007). Stabilizing function for myristoyl group revealed by the crystal structure of a neuronal calcium sensor, guanylate cyclase-activating protein 1. Structure 15, 1392–1402.
Stephen, R., Filipek, S., Palczewski, K., and Souza, M. C. (2008). Ca2+-dependent regulation of phototransduction. Photochem. Photobiol. 84, 903–910.
Striessnig, J., Bolz, H. J., and Koschak, A. (2010). Channelopathies in CaV 1.1, CaV1.3, and CaV1.4 voltage-gated L-type Ca2+-channels. Pflugers Arch. 460, 361–374.
Suryanarayanan, A., and Slaughter, M. M. (2006). Synaptic transmission mediated by internal calcium stores in rod photoreceptors. J. Neurosci. 26, 1759–1766.
Szikra, T., Barabas, P., Bartoletti, T. M., Huang, W., Akopian, A., Thoreson, W. B., and Krizaj, D. (2009). Calcium homeostasis and cone signalling are regulated by interactions between calcium stores and plasma membrane ion channels. Plos One 4, e6723. doi: 10.1371/journal.pone.0006723
Szikra, T., Cusato, K., Thoreson, W. B., Barabas, P., Bartoletti, T. M., and Krizaj, D. (2008). Depletion of calcium stores regulates calcium influx and signal transmission in rod photoreceptors. J. Physiol. 586, 4859–4875.
Tanimoto, N., Brombas, A., Müller, F., and Seeliger, M. W. (2012). HCN1 channels significantly shape retinal photoresponses. Adv. Exp. Med. Biol. 723, 807–812.
tom Dieck, S., Altrock, W. D., Kessels, M. M., Qualmann, B., Regus, H., Brauner, D., Fejtova, A., Bracko, O., Gundelfinger, E. D., and Brandstätter, J. H. (2005). Molecular dissection of the photoreceptor ribbon synapse: physical interaction of bassoon and Ribeye is essential for the assembly of the ribbon complex. J. Cell Biol. 168, 825–836.
Townes-Anderson, E., and Zhang, N. (2006). “Synaptic plasticity and structural remodelling of rod and cone cells,” in Plasticity of the Visual System: From Genes to Circuits, eds R. Pinaud, L. A. Tremere, and P. DeWeerds, (Berlin, Heidelberg, NY: Springer Science), 13–31.
Uthaiah, R. C., and Hudspeth, A. J. (2010). Molecular anatomy of the hair cell's ribbon synapse. J. Neurosci. 30, 12387–12399.
Venkataraman, V., Duda, T., Vardi, N., Koch, K. W., and Sharma, R. K. (2003). Calcium-modulated guanylate cyclase transduction machinery in the photoreceptor-bipolar synaptic region. Biochemistry 42, 5640–5648.
Venkatesan, J. K., Natarajan, S., Schwarz, K., Mayer, S. I., Alpadi, K., Magupalli, V. G., Sung, C. H., and Schmitz, F. (2010). Nicotinamide adenine dinucleotide-dependent binding of the neuronal Ca2+ sensor protein GCAP2 to photoreceptor synaptic ribbons. J. Neurosci. 30, 6559–6567.
Vollrath, L., and Spiwoks-Becker, I. (1996). Plasticity of retinal ribbon synapses. Microsc. Res. Tech. 35, 472–487.
Wahl-Schott, C., Baumann, L., Cuny, H., Eckert, C., Griessmaier, K., and Biel, M. (2006). Switching off calcium-dependent inactivation in L-type calcium channels by an autoinhibitory domain. Proc. Natl. Acad. Sci. U.S.A. 103, 15657–15662.
Wan, Q. F., and Heidelberger, R. (2011). Synaptic release at mammalian bipolar cell terminals. Vis. Neurosci. 28, 109–119.
Wang, Y., Okamoto, M., Schmitz, F., Hofmann, K., and Südhof, T. C. (1997). RIM is a putative effector in regulating synaptic-vesicle fusion. Nature 388, 593–598.
Woodruff, M. L., Sampath, A. P., Matthews, H. R., Krasnoperova, N. V., Lem, J., and Fain, G. L. (2002). Measurement of cytoplasmic calcium concentration in the rods of wild-type and transducin knock-out mice. J. Physiol. 542, 843–854.
Wycisk, K. A., Budde, B., Feil, S., Skosyrski, S., Buzzi, F., Neidhardt, J., Glaus, E., Nürnberg, P., Rüther, K., and Berger, W. (2006). Structural and functional abnormalities of retinal ribbon synapses due to Cacna2d4 mutation. Invest. Ophthalmol. Vis. Sci. 47, 3523–3530.
Xiao, H., Chen, X., and Steele, Jr. E. C. (2007). Abundant L-type calcium channel CaV1.3 (alpha1D) subunit mRNA is detected in rod photoreceptors of the mouse retina via in-situ hybridization. Mol. Vis. 13, 764–771.
Yang, P. S., Alseikhan, B. A., Hiel, H., Grant, L., Mori, M. X., Yang, W., Fuchs, P. A., and Yue, D. T. (2006). Switching of the Ca2+-dependent inactivation of CaV1.3-channels by calcium binding proteins of auditory hair cells. J. Neurosci. 26, 10677–10689.
Zeitz, C., Kloeckener-Gruissem, B., Forster, U., Kohl, S., Magyar, I., Wissinger, B., Mátyás, G., Borruat, F. X., Schorderet, D. F., Zrenner, E., Munier, F. L., and Berger, W. (2006). Mutations in CABP4, the gene encoding the Ca2+-binding protein 4, cause autosomal recessive night blindness. Am. J. Hum. Genet. 79, 657–667.
Zenisek, D., Steyer, J. A., and Almers, W. (2000). Transport, capture and exocytosis of synaptic vesicles at active zones. Nature 406, 849–854.
Zhang, N., and Townes-Anderson, E. (2002). Regulation of structural plasticity by different channel types in rod and cone photoreceptors. J. Neurosci. 22, 7065–7079.
Keywords: photoreceptor, ribbon synapse, synaptic ribbon, GCAP, RIBEYE, CaBP4, CaV1.4 calcium channel, EF-hands
Citation: Schmitz F, Natarajan S, Venkatesan JK, Wahl S, Schwarz K and Grabner CP (2012) EF hand-mediated Ca2+- and cGMP-signaling in photoreceptor synaptic terminals. Front. Mol. Neurosci. 5:26. doi: 10.3389/fnmol.2012.00026
Received: 09 January 2012; Paper pending published: 25 January 2012;
Accepted: 15 February 2012; Published online: 29 February 2012.
Edited by:
Karl-Wilhelm Koch, Carl von Ossietzky University Oldenburg, GermanyReviewed by:
Karl-Wilhelm Koch, Carl von Ossietzky University Oldenburg, GermanyFlorentina Soto, Washington University in St. Louis, USA
Copyright: © 2012 Schmitz, Natarajan, Venkatesan, Wahl, Schwarz and Grabner. This is an open-access article distributed under the terms of the Creative Commons Attribution Non Commercial License, which permits non-commercial use, distribution, and reproduction in other forums, provided the original authors and source are credited.
*Correspondence: Frank Schmitz and Chad P. Grabner, Department of Neuroanatomy, Medical School Homburg/Saar, Institute for Anatomy and Cell Biology, Saarland University, Kirrbergerstrasse, University Campus, 66421 Homburg/Saar, Germany. e-mail:ZnJhbmsuc2NobWl0ekB1a3MuZXU=;Y2hhZGdyYWJuZXJAZ21haWwuY29t
†Present Address: Department of Experimental Orthopedics, Saarland University, University Hospital of Orthopedics, Homburg/Saar, Germany.