- 1Cellular and Molecular Neurobiology Unit, Indian Institute of Technology Jodhpur, Jodhpur, India
- 2School of Basic Sciences, Indian Institute of Technology Mandi, Mandi, India
Cells regularly synthesize new proteins to replace old or damaged proteins. Deposition of various aberrant proteins in specific brain regions leads to neurodegeneration and aging. The cellular protein quality control system develop various defense mechanisms against the accumulation of misfolded and aggregated proteins. The mechanisms underlying the selective recognition of specific crucial protein or misfolded proteins are majorly governed by quality control E3 ubiquitin ligases mediated through ubiquitin-proteasome system. Few known E3 ubiquitin ligases have shown prominent neurodevelopmental functions, but their interactions with different developmental proteins play critical roles in neurodevelopmental disorders. Several questions are yet to be understood properly. How E3 ubiquitin ligases determine the specificity and regulate degradation of a particular substrate involved in neuronal proliferation and differentiation is certainly the one, which needs detailed investigations. Another important question is how neurodevelopmental E3 ubiquitin ligases specifically differentiate between their versatile range of substrates and timing of their functional modulations during different phases of development. The premise of this article is to understand how few E3 ubiquitin ligases sense major molecular events, which are crucial for human brain development from its early embryonic stages to throughout adolescence period. A better understanding of these few E3 ubiquitin ligases and their interactions with other potential proteins will provide invaluable insight into disease mechanisms to approach toward therapeutic interventions.
Introduction
Development of brain is a long, self-oriented, tightly regulated, complex molecular process governed by various crucial genes, linked with cell proliferation and differentiation (Gilbert et al., 2005). Previous findings have revealed the role of neural stem cells and several other genes in different neurobiological stages of brain development, such as differentiation of the neural progenitor cells, neural tube formation, neural patterning, neurogenesis to neuronal migration and neuronal myelination (Stiles and Jernigan, 2010; Gage and Temple, 2013). But, how these genes and their relevant end product proteins are stringently regulated under the entire span of neurodevelopment is not well understood. Previous studies suggest that E3 ubiquitin ligases play a pivotal role in different neurodevelopmental stages (Huang, 2010; Stegmuller and Bonni, 2010). Several other genes share and regulate the overall burden of neurogenesis and brain development (Preuss, 2012). Mutations or genetic disturbances in these genes generally affect the cognition and behavior, which may also represent the functional loss of crucial cellular processes, such as, synaptic functions, protein translation, cellular proliferation and differentiation (Ernst, 2016). Continuous cellular and molecular deficits of these neurobiological mechanisms are noticeable, which may result in several neurodevelopmental disorders (Sahin and Sur, 2015).
Eukaryotic cell evolution is one of the greatest landmarks in the history of life, marking the formation of hyper-structures and ultra-specialized cellular systems, which are probably developed from the earlier simpler forms of organisms, i.e., prokaryotes (Norris and Root-Bernstein, 2009; Archibald, 2015). These cells perform a variety of cellular tasks with utmost accuracy; and while doing so, cells need a large number of proteins with varying shapes, sizes, subcellular locations, and functions (Zimmerberg and Kozlov, 2006; Ananthakrishnan and Ehrlicher, 2007). Although cells contain around 20,000 different kinds of protein-coding genes, they still produce and retain only a set of proteins at a time, from all the available sequences, in accordance with the requirements of the cells (Kim et al., 2014; Wilhelm et al., 2014). Furthermore, cells need a tight regulation of synthesis and degradation of proteins, without which successful execution of cellular functions is not possible (Gallastegui and Groll, 2010). Cells comprise a subset of approximately 1400 specialized proteins in their repertoire, which is essentially required to achieve and maintain a functional state of its proteome (Balchin et al., 2016). A large number of chaperones and their cofactors assist newly synthesized linear polypeptide chains in attaining their functional three-dimensional shapes by intercepting unfruitful inter-domain interactions and protect them against various kinds of cytotoxic stresses, in order to prevent unwanted misfolding events inside the crowded cytoplasmic milieu (Ellis, 1987; Hartl and Hayer-Hartl, 2009; Hartl et al., 2011). Defying such kind of tight control, a subset of proteins still remains structurally disordered in the cell, and are further taken care by molecular chaperones (Walter and Buchner, 2002; Dunker et al., 2008).
Age-related changes and continuous stresses cause a significant decline in efficiency of molecular chaperones, which may result in accumulation of proteinaceous aggregates inside the cells (Tyedmers et al., 2010). Such conditions may result in progression of various types of cancers and neurodegenerative diseases (Morimoto, 2008; Kim et al., 2013). To avoid such unwanted deleterious changes, chaperones may also opt to degrade accumulated toxic proteinaceous burden of the cell, in concerted mechanisms, carried out by cellular proteolytic systems, viz., autophagy and UPS (Arndt et al., 2007; Hartl et al., 2011). Protein degradation machinery of the cell facilitates the degradation of cellular proteins, which have greatly varying half-lives, ranging from few minutes to several hours (Bachmair et al., 1986; Belle et al., 2006; Schwartz and Ciechanover, 2009). Both of these pathways recognize small ubiquitin molecules attached to cellular proteins (the process is called ubiquitylation), as the tags of death, and initiate the degradation pathways. Ubiquitin is a very small protein of 76 amino acids in length, and approximately 8.5 kDa of molecular weight (Ciehanover et al., 1978; Glickman and Ciechanover, 2002; Ciechanover, 2005).
Ubiquitylation is a type of post-translational modification of a protein, in which a concerted action of multiple players, lying into a cascade of reactions, attaches a small ubiquitin moiety to a substrate (Hershko et al., 1983, 2000). It may lead either to a functional modulation, or it may also be treated as death signals, depending upon the pattern, the ubiquitin molecules are attached (Woelk et al., 2007; Komander and Rape, 2012). As illustrated in Figure 1, the original discoverers have identified a series of enzymatic reactions, in which, the formation of a thioester bond between C-terminal glycine residue of ubiquitin and a cysteine residue present on E1 ubiquitin activating enzyme activates the ubiquitin in an ATP-dependent manner (Haas and Rose, 1982). Thereafter, the activated ubiquitin is transferred to another cysteine residue, present on a different class of enzymes, called E2, through transesterification (Hershko and Ciechanover, 1992; Ciechanover, 1994). Afterward, transfer of activated ubiquitin to the target protein is mediated by a large group of another set of enzymes, known as E3 ubiquitin ligases (Hershko et al., 1983). Increasing reports suggest that the number of E3 ubiquitin ligases is reaching around thousand, which enables them to provide substrate specificity inside the cells to take control of most of the major and minor cellular pathways (Nakayama and Nakayama, 2006).
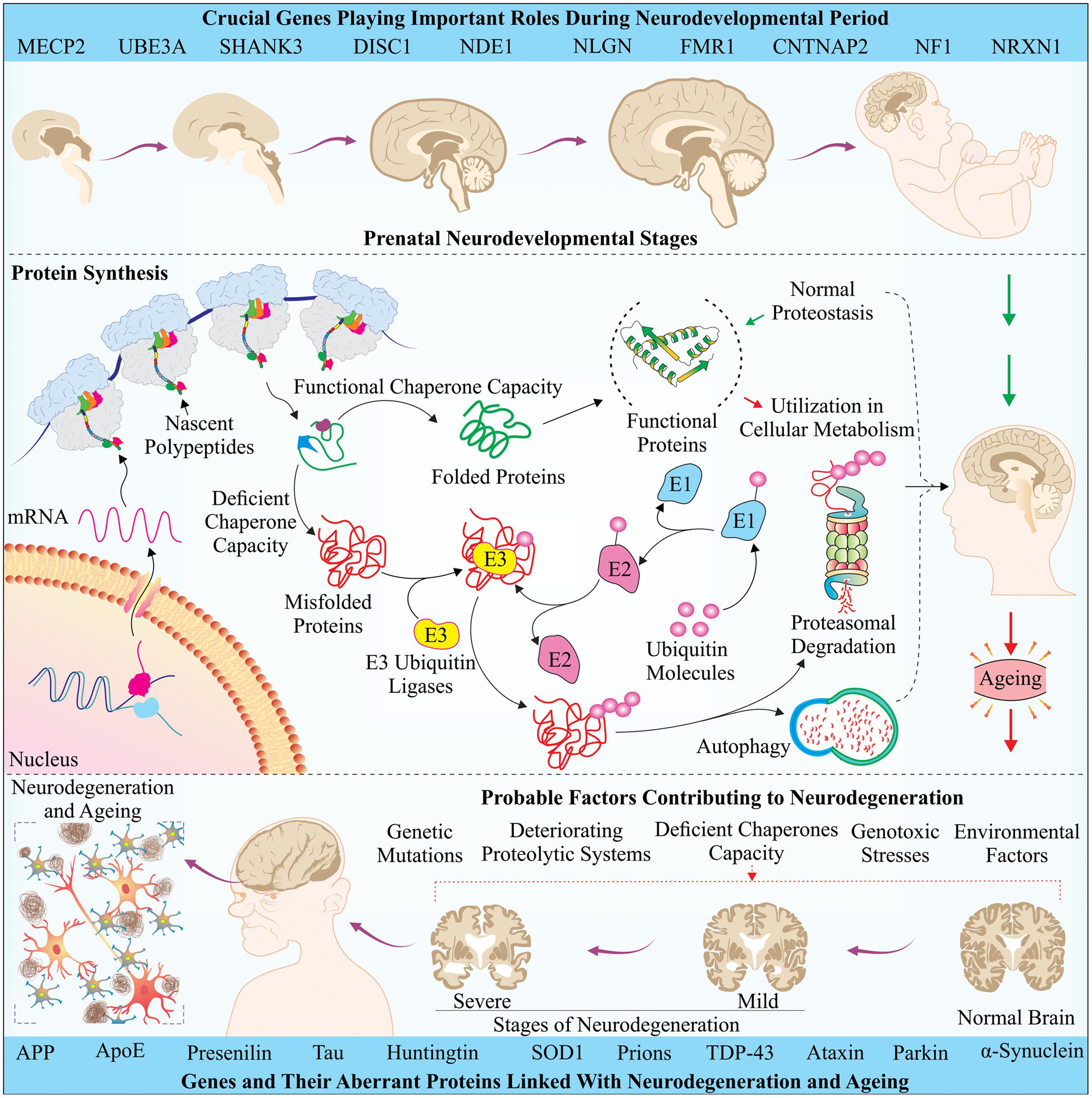
FIGURE 1. An illustration of the orchestration of factors involved in neurodevelopment and neurodegeneration: The upper part of the figure represents how stepwise modifications occur during the prenatal period of brain development. As described in respective sections of the text, few crucially important genes, which play pivotal roles at various stages of brain development, have been mentioned at the top. To establish a better understanding, schematic of cellular translation machinery along with other PQC systems has also been drawn. A state of proteostasis and active control of PQC system regulate the processes of growth, adolescence, and development. However, aging related metabolic changes, including various kinds of stresses and successive deterioration of quality control systems may lead to accumulation of several proteins, causing the formation of inclusion bodies, which results in late-stage neurodegeneration. Few important established protein candidates, involved in various such diseases have been mentioned at the bottom panel; for details, please refer text.
These enzymes attach the ubiquitin molecule to the 𝜀-amino group of an internal lysine residue of the substrate protein, or in other cases, to one of the seven lysine residues present on an already attached ubiquitin molecule (Ciechanover, 1994; Hershko et al., 2000). The way, the number, and the pattern, into which the ubiquitin moieties get attached to a given protein, provide the code, on which other cellular systems act and decide their diverse fates, as they could be sorted, trafficked, functionally or structurally modulated or degraded (Rotin and Kumar, 2009; Rajalingam and Dikic, 2016). After finding a suitable degradatory signal, the proteolytic machinery of the cell, the 26S proteasome degrades the substrate into smaller oligopeptides (Finley, 2009). However, to prevent such degradation signals from being constitutive, cells need to remove these ubiquitin chains immediately from the substrate proteins, once they have been utilized. For this, cells employ another set of approximately a 100 different deubiquitinating (DUBs) enzymes, which by shredding off ubiquitin moieties from proteins, contribute largely to their proper recycling (Komander et al., 2009; Reyes-Turcu et al., 2009). Therefore, similar to kinases and phosphatases, E3 ubiquitin ligases and DUBs also provide a mechanism of reversible modifications of cellular proteins to alter their functions (D’Andrea and Pellman, 1998; Dikic and Robertson, 2012).
Roles of E3 ubiquitin ligases have been recognized in the developing, maintaining, as well as in degrading the components of the cells. Developing roles include those, which are played by E3 ubiquitin ligases in developmental processes, such as, cell division, stem cell differentiation and organogenesis, etc. (Nakayama and Nakayama, 2006; Yokomizo and Dzierzak, 2008; Stegmuller and Bonni, 2010). Maintenance responsibilities include, cell signaling, metabolism, transcriptional control, protein sorting, trafficking, cell to cell communication and modulation of inflammatory responses, etc. (Liu, 2004; Weake and Workman, 2008; Acconcia et al., 2009; Hampton and Garza, 2009; Huang, 2010; Polo, 2012). However, E3 ubiquitin ligases are also profoundly associated with the establishment of cellular proteostasis by regulating the turnover of cellular proteins, using degradatory pathways of UPS and autophagy (Schwartz and Ciechanover, 2009; Kuang et al., 2013). Induction of apoptotic pathways could also be attributed to degradatory functions of the E3 ubiquitin ligases (Vucic et al., 2011). Continuous degradation of intracellular proteins via proteasome or autophagy also facilitates recycling of amino acid pool of the cells for synthesis of new proteins (Lilienbaum, 2013).
It is well accepted that brain development is one of the most complex biological processes, which starts in early gestation period and lasts up to adolescence, and is orchestrated and affected by both genetic and environmental factors (Lenroot and Giedd, 2008; Stiles and Jernigan, 2010). Several billion neurons integrate together to form a network called neural circuitry, which receives and transmits electrochemical signals in order to perform cognitive and behavioral functions with regulatory control (Pakkenberg and Gundersen, 1997; Tau and Peterson, 2010). Therefore, to completely understand the process of neurodevelopment and to address the problems of developmental abnormalities, representation of a comprehensive overview of the majorly associated environmental and genetic factors is needed. We, here, are providing a brief outline of how several external stresses, pollutants, toxic chemicals and consumption of certain compounds may obstruct the successful development.
Regulatory control over a large set of processes makes E3 ubiquitin ligases a putative therapeutic target, with huge potential in the upcoming years of research, in the field of developmental disorders, neurodegeneration, ageing, and cancer (Sun, 2006; Eldridge and O’Brien, 2010; Goru et al., 2016). In this review, a comprehensive description of few important E3 ubiquitin ligases, which are crucial for various kinds of developmental processes, has been provided. We have also given a concise overview of few emerging players, which have been evolved in past few years as crucial regulators of various neurodevelopmental and neurodegenerative disorders, for example, ITCH, E6-AP, MGRN1, and HACE1, which certainly need attention of scientific community for their remedial exploration (Rotin and Kumar, 2009; Upadhyay et al., 2015a).
A large number of E3 ubiquitin ligases form an intricate network, over which regulatory mechanisms of several cellular pathways are built. This is why the UPS is considered to be a highly specific degradation mechanism for intracellular proteins, unlike autophagic and lysosomal degradation pathways. As stated earlier, there is increasing evidence clearly indicating the involvement of E3 ubiquitin ligases in tight regulation of most, if not all, of the cellular processes (Hilt and Wolf, 2000; Joshi et al., 2016). These E3 ubiquitin ligases sense various kinds of changes and stresses quickly inside the cytosol, and mount a simultaneous response by affecting the turnover or functionality of cellular proteins, which play regulatory roles in several cellular processes, e.g., different receptors, tumor suppressors, kinases, and transcriptional regulators, etc. (Mayer et al., 2005; Woelk et al., 2007; Polo, 2012). In the subsequent sections, we will briefly provide a summary of research done so far, in the field of both, proteolytic and non-proteolytic ways of regulation of cellular proteins, mediated by a plethora of E3 ubiquitin ligases.
Neurodevelopmental Processes and Neurodegenerative Diseases are Affected by Environmental and Genetic Factors
Neurodevelopment associated problems have always remained a great challenge to understand for physicians and scientists. Considering research done in last few decades, it would be obvious to say that environment has a deep impact on organism growth, development, evolution, and extinction (Jirtle and Skinner, 2007). Developmental scientists have reported since decades, about how environmental constituents are crucial factors shaping the developmental processes and how environmental stresses and toxicities retard the growth and put challenges before these processes (Knobloch and Pasamanick, 1960). Over the years, it has been observed that prenatal development is a well-programmed process (Huxley et al., 2000) and exposure to various kinds of radiations (Koturbash et al., 2006), xenobiotic chemical compounds (Ho et al., 2006), maternal behavior (Weaver et al., 2004), post-weaning nutrition (Waterland et al., 2006), environmental pollutants (Perera and Herbstman, 2011), and heavy metals (Kampa and Castanas, 2008) have multifactorial deleterious effects on the embryonic health.
Alcohol consumption by the mother during pregnancy may also impair cognitive and behavioral growth (Ornoy and Ergaz, 2010); whereas microcephaly and congenital anomalies are other reported symptoms (Ouellette et al., 1977). Smoking and alcohol intake may also cause ADHD (Mick et al., 2002). On the other hand, increased risks of asthma (Stick et al., 1996) and obesity (Power and Jefferis, 2002) have also been observed in offspring of mothers, who regularly smoke during pregnancy. Regular consumption of marijuana products and drugs like cocaine during gestations period may also have detrimental effects in newly born infants, including lower weight and height (Zuckerman et al., 1989), ADHD and declining cognitive functioning (Huizink and Mulder, 2006). In utero exposure to environmental pollutants, e.g., polycyclic aromatic hydrocarbons (Brown et al., 2007), bisphenol A (Ho et al., 2006), arsenic (Smith et al., 2006), and phthalates (Kang and Lee, 2005) could also exert epigenetic hazards, genotoxicity, high risks of lung cancer, and endocrine disruption, etc. (Perera and Herbstman, 2011). There are several other factors and chemical compounds, e.g., oxidative stress, valproic acid, caffeine, lithium chloride, and retinoic acid, which could have many toxic and teratogenic effects on embryonic development (Dennery, 2007; Selderslaghs et al., 2009). But, adding to all these, genetic alterations and mutations constitute the major factors, lying at the bottom of many developmental disorders.
Notably, an increasing line of evidence has also established the confounding roles of a number of genes on developmental processes. These genes could have multiple direct or indirect influences on various pathways and reactions, which are essential for organism development (Wells et al., 2005; Raff, 2012; Zoghbi and Bear, 2012). A well-regulated expression levels of few genes, e.g., p53, BRCA1, Hox, etc., throughout prenatal period are considered to be a crucial factor for development (Choi and Donehower, 1999; Wells et al., 2005; Schuettengruber et al., 2007), whereas mutations reported in other genes have also shown occurrence of some developmental disorders(Mitchell, 2011). Autism is a heterogeneous condition of the spectrum of communication and developmental disabilities in children; it includes several sets of disorders with varying features and commonly known as ASD (Lord et al., 2000; Frith and Happé, 2005).
More than a 100 different genes have been reported so far to be disrupted, deleted or mutated in ASD, developing several kinds of symptoms and disorders like ID and epilepsy (Geschwind and Levitt, 2007; Betancur, 2011; Miles, 2011). Triplet codon CGG expansion and hypermethylation lead to the suppression of FMR1 gene that is a common cause associated with the fragile X syndrome, a well-known intellectual and emotional disorder (Jin and Warren, 2000). Genetic mutations of X-linked genes neuroligin-3 and -4 (NLGN3 and NLGN4) have also shown similar kinds of symptoms leading to autistic disorders (Jamain et al., 2003). CNTNAP2 (Alarcon et al., 2008), neurexin-1 (NRXN1) (Ching et al., 2010) are two other genes, which have been investigated in recent years for their wider implications in various kinds of developmental processes and association with these diseases. Another autistic condition, neurofibromatosis type 1 (NF1), is an autosomal dominant neurodevelopmental disorder, mainly caused by mutations in gene NF1 (Wallace et al., 1990).
Rett syndrome is an X-linked dominantly inherited, autistic neurodevelopmental disorder leading to mental retardation in early childhood (Rett, 1966; Chahrour and Zoghbi, 2007). Reports suggest that the disease occurs due to mutations in MECP2 (Amir et al., 1999). PMS, a global developmental delay related disorder, is found to be associated with disruption of SHANK3 (Bonaglia et al., 2001). Similarly, DISC1 and 2 are other genes, which have been reported to cause a well-known psychiatric mental disorder SCZ, with reported symptoms of anxiety and depression (Millar et al., 2000). NDE1 plays essential roles in mitosis and microtubule arrangements, so have crucial implication in neurodevelopment (Bradshaw Nicholas et al., 2013). NDE1-null mice have been reported recently to develop smaller brain, with cerebral cortex affected mostly (Alkuraya et al., 2011; Ramalingam et al., 2011); whereas single nucleotide mutations in this gene may also result in SCZ like symptoms (Kimura et al., 2015).
The past decades have seen tremendous advancements in health care leading to better health management and hence people living longer. The increase in longevity has led to increased prevalence of neurodegeneration in the elderly people. The risk factors for neurodegenerative diseases could be both, endogenous or exogenous; but have not been well described (Cannon and Greenamyre, 2011; Kanthasamy et al., 2012). Most of the neurodegenerative diseases occur at a late-age, either because of several genetic mutations, or aging-associated decline in cellular repair mechanisms (Finch and Tanzi, 1997; Niccoli and Partridge, 2012; Yerbury et al., 2016). These detrimental changes include alterations in various components of the cellular PQC system, e.g., declined chaperone capacity, weakening proteolytic functioning of autophagy and UPS, and compromised mitochondrial health and ER proteome balance (Germain, 2008; Koga et al., 2011; Kaushik and Cuervo, 2015). Similar to earlier describe developmental abnormalities, environmental factors and various genotoxic stresses also contribute in neurodegeneration and aging processes (Stokes et al., 1999; Cannon and Greenamyre, 2011; Yegambaram et al., 2015). A major hallmark of neurodegenerative diseases is the abnormal intra- or extracellular accumulation of disease-causing aggregate-prone proteinaceous species, which further hinder with the normal functioning of neuronal cells leading to their death, causing cognitive impairment, dementia, and behavioral abnormalities (Kanazawa, 2001; Ross and Poirier, 2004).
As we have described, several genetic mutations lead to developmental deformities or may also cause embryonic mortality in some cases, it is now easily understandable how important genomic integrity is, for the proper functioning of the organism. Several aging-related changes and late-onset neurodegenerative disorders may also add up to the pathologies generated, either due to some genetic mutations, or structural and functional modifications in disease-associated proteins (Bertram and Tanzi, 2005; Esiri, 2007; Douglas and Dillin, 2010). Most prevalent of these, Alzheimer’s disease, has been reported to be caused due to mutations in genes, like APP (Goate and Chartier-Harlin, 1991), presenilins (Scheuner et al., 1996), tau (Hutton et al., 1998), etc. Apolipoprotein E (ApoE), in recent years, have also been shown as one of the risk factors for the disease (Saunders et al., 1993).
Parkinson’s disease is the second most death-causing neurodegenerative disorder and is also linked with several genes viz. PINK1 (Valente et al., 2004), parkin (Kitada et al., 1998), α-synuclein (Polymeropoulos et al., 1997), as disease-onset factors. Prion diseases are another class of diseases, where genetic mutations alter the structure and function of the cellular prion protein, which may develop into the neurodegenerative changes (Prusiner and DeArmond, 1994). ALS is a disease, related to loss of motor neurons, which is caused by aggregation of protein products of several inclusion-forming proteins, e.g., SOD1 (Wong et al., 1995) and TDP-43 (Iguchi et al., 2013). Several proteins may also attain a property to aggregate due to the expansion of glutamine coding CAG trinucleotide repeats in their gene sequences (La Spada et al., 1991). Aberrant forms of huntingtin (DiFiglia et al., 1997) and ataxin (Orr et al., 1993) are major examples of polyglutamine expansion proteins, which are directly associated with the amyloid-like aggregate formation in different diseases.
Neurodegeneration is not a spontaneous process; exposure to various neurotoxicants present in the environment over a period of time leads to irreparable damages. These neurotoxicants interfere with the metabolism and disturb the homeostasis. Heavy metals, herbicides, pesticides, biogenic metals have been described as potential risk factors for neurodegeneration (Brown et al., 2005; Ritz et al., 2009; Chin-Chan et al., 2015). Essential trace elements (aluminum, zinc, and copper) have been found to be associated with aggregated proteins in Alzheimer’s disease (Bush et al., 1994; White et al., 1999; Bharathi et al., 2008). Lead toxicity is also a well-described phenomenon and exposure of children to lead at early developmental stages might influence the occurrence of neurodegeneration, at later stages (Basha et al., 2005). Rural settings with improper sanitation and industrial waste disposal, agricultural runoff, etc., all carry neurotoxicants and pose threat to human beings.
Neurotoxicants can damage or cross blood brain barrier, gain access through receptors having similar ligands (McCormack and Di Monte, 2003). The damage of neurons affects neurotransmission due to lipid peroxidation, which leads to increased ROS generation and oxidative stress (Nunomura et al., 2001; Aruoma et al., 2006; Reed, 2011). The increased oxidative stress results in the formation of protein aggregates, which in turn activates the glial cells. These cells contribute in enhancing inflammation at the site along with the release of NO, leading to further upregulation in oxidative stress, by the formation of ONOO-peroxynitrite (Vincent et al., 1998). The emerging understanding of pathophysiology of neurodegenerative disorders is helping us to understand the potential risk of environmental factors on human health and also come up with novel strategies for therapeutics. Several E3 ubiquitin ligases in past have been explored for their significant contribution in neurodevelopmental processes and involvement in establishment of cellular proteostasis. In the upcoming section, we are briefly describing few similar quality control (QC) E3 ubiquitin ligases and elaborating on how they appear to be silent for a long time interval in life span and how they suddenly become proactive and play a crucial role at the time of requirement against misfolded and accumulated proteins, linked with neurodegeneration.
Good Programming of UBE3A: How it is Important for Neuronal Development and Neurodegeneration?
E3 ubiquitin ligase E6-AP is encoded by UBE3A gene; mutations and genetic imprinting in this gene result in Angelman syndrome (AS), which can be described by symptoms like frequent laughter, tremor, ataxia, abnormal gait, seizures, and neurological impairments (Kishino et al., 1997). Several studies identified critical neuronal dysfunctions in UBE3A maternal-deficient mice (AS mice model), which include defective synaptic plasticity, neurological deficits, aberrations in LTP, defects in rotarod performance, abnormal dendritic spine morphology, deficits in contextual learning, abnormalities in neocortex maturation, reduced brain weight, cognitive dysfunction and abnormalities in fluid consumption behavior and grip strength (Kuhnle et al., 2013; Silva-Santos et al., 2015). The emerging function of E6-AP were discovered later and is associated with its E3 ubiquitin ligase like capabilities, through which it can play a very important neuroprotective role in cellular QC mechanisms and its functional presence is involved in different neurodegenerative diseases through clearance of several misfolded proteins (Upadhyay et al., 2015a).
Under different stress conditions, E6-AP expression levels are dramatically induced and provide cytoprotection against different proteotoxic insults. E6-AP interacts with Hsp70 and preferentially targets aggregatory proteins for their UPS-dependent clearance. Recruitment of E6-AP with aggresomes at peripheral nuclear regions reveals its capability to recognize large misfolded inclusion-like structures in cells (Mishra et al., 2009b). Considering the efficiency of E6-AP in cellular protein QC mechanisms, it is expected that E6-AP might target neurodegenerative disease-associated proteins. In another study, we have demonstrated that E6-AP facilitates the ubiquitin-mediated degradation of polyglutamine proteins aggregates and alleviates toxicities generated by them in cells. The high expression of E6-AP protects cells against the massive buildup of misfolded proteins that can cause cellular toxicity and finally leads to cell death (Mishra et al., 2008). After understanding the potential of E6-AP against misfolded protein clearance, we again checked its capability in another neurodegenerative disease. We noticed that E6-AP recognizes misfolded SOD1 aggregates and targets them for ubiquitylation and also promotes their degradation via UPS machinery (Mishra et al., 2013).
Previously, we have also found that E6-AP mediates the ubiquitylation and proteasome-dependent degradation of p53 tumor-suppressor protein without E6 oncoprotein (Mishra and Jana, 2008). Lack of E6-AP function results in inefficient elimination of p53, hence may also alter cell cycle progression, and affect the normal physiological functions of different brain regions (Jiang et al., 1998). Interestingly, another study from our group indicates a strong potential of E6-AP in the degradation of cyclin-dependent kinase inhibitor p27. Probably, such type of regulation of p27 and a loss-of-function of E6-AP, both contributes to the molecular pathogenesis of AS (Mishra et al., 2009a). Earlier reports have shown that p27 lack-of-function in knockout mice exhibits increased brain size, which could be possible because of high cellular proliferation rate (Fero et al., 1996; Tarui et al., 2005). E6-AP mediated ubiquitylation and endocytosis of SK2 is implicated in synaptic plasticity and formation of memory and learning (Sun et al., 2015).
E6-associated protein possesses a capacity to regulate cell cycle regulatory proteins, which are vital for cellular proliferation and division. Therefore, E6-AP may contribute significantly in different neurodevelopmental phases, and simultaneously, it can also reduce the accumulation of misfolded proteins in cells and clears the unwanted toxicity generated due to misfolded proteins to avoid neurodegeneration. Interestingly, a finding indicates that AS adults also develop bradykinesia and Parkinsonism symptoms (Harbord, 2001). By truncating or silencing Ube3A-antisense transcript (ATS), improvements in cognition and behavioral functions in AS mice model has been observed (Meng et al., 2013, 2015). Additionally, topoisomerase inhibitor topotecan has also shown to reactivate dormant Ube3a allele having applications in AS therapy (Huang et al., 2011). To summarize and as has been represented in Figure 2A, E6-AP in cells provides a pivotal link between NDDs and neurodegenerative diseases. The essential question in near future would be to look up to the molecular functions of E6-AP and to understand how this E3 ubiquitin ligase is capable of switching its neurodevelopmental responsibilities and its QC functions and how it contributes to the alleviation of the pathobiology of neurodegenerative diseases.
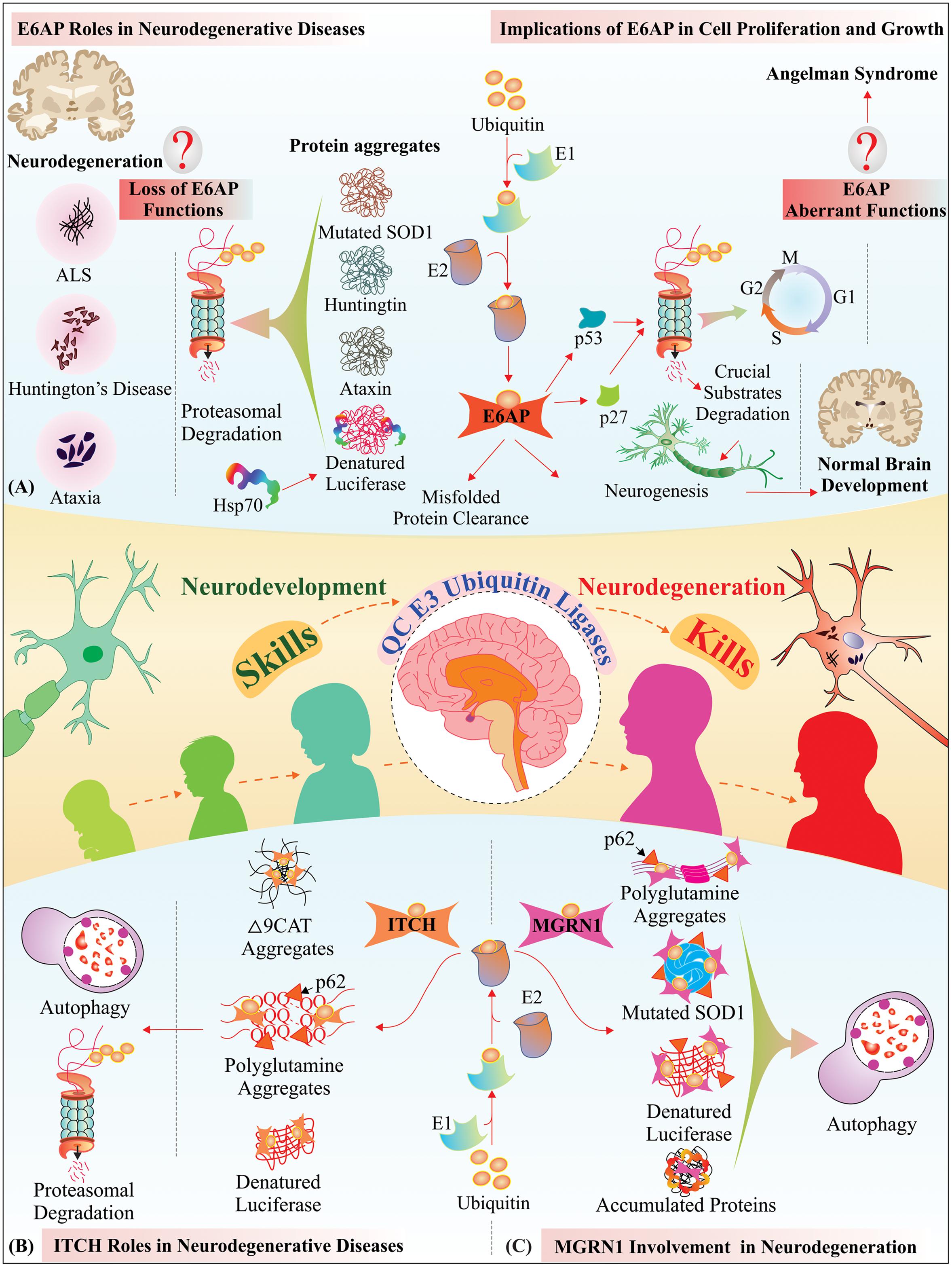
FIGURE 2. Schematic representation of emerging roles of neurobiological QC E3 ubiquitin ligases implicated in neurodevelopment, synaptogenesis, and, their lack of functions contribute to the pathogenesis of neurodegeneration: (A) Genetic defects in UBE3A gene results in defective E6-AP protein, which is responsible for Angelman syndrome neurodevelopmental disorder. Functional E6-AP promotes the ubiquitylation of tumor-suppressor proteins such as p53 and p27 and regulates cellular proliferation and growth. E6-AP also plays a significant role in the clearance of mutant misfolded proteins linked with various neurodegenerative diseases. (B) ITCH specifically ubiquitylates several cytoplasmic protein inclusions for their removal from the dense crowded cellular milieu. The central segment represents overall functional roles of QC E3 ubiquitin ligases due to their crucial involvement in the neurodevelopment and neurodegeneration. (C) Mahogunin functions as a RING finger E3 ubiquitin ligase; and a null mutation in mahogunin is linked with spongiform neurodegeneration. Our studies reveal the potential roles of MGRN1 as a QC E3 ubiquitin ligase involved in the elimination of mutated proteins linked with polyglutamine and ALS neurodegenerative diseases.
Accurate and Wide Spread Functions of AIP4 Gene Product Orients Neuronal Development and Survival
Atrophin-1-interacting protein 4 gene encodes a HECT-containing ITCH E3 ubiquitin ligase. Loss-of-function of this protein exhibits various immunological problems in mice. ITCH a protein of more than 100 kDa catalyzes the degradation of multiple substrates with the help of UPS (Perry et al., 1998). Regulated protein elimination via autophagy and UPS contributes in different molecular pathways of brain development, e.g., axon guidance, axon and dendritic branching, synaptogenesis and building synaptic connections. Numb protein is important for cell fate determination, differentiation of progenitors of cerebellar granule cells, cell migration and also targets the critical substrates for ITCH and help in their ubiquitylation (Cayouette and Raff, 2002; McGill and McGlade, 2003; Klein et al., 2004). Few fundamental questions always appear in mind, such as what is the physiological relevance of these few specific E3 ubiquitin ligases in neurodevelopmental period?
Currently, we are short of understanding how dysfunctions of E3 ubiquitin ligases or aberrant elimination of unwanted proteins contribute to multiple NDDs and neurodegenerative diseases. To challenge such questions, it was important for us to explore the molecular functions of ITCH under various multifactorial stress conditions, where the massive accumulation of aberrant polypeptides can cause failure of QC mechanisms. For the first time, our study established the QC function of ITCH E3 ubiquitin ligase against cytosolic aberrant aggregates, as has been represented in Figure 2B. In our findings, we have noticed that ITCH endogenous levels get elevated after various genotoxic and proteotoxic cellular insults. Sequestosome-1 (SQSTM1) or p62 (early autophagic structures) and 20S proteasome get recruited to the site of the cytoplasmic misfolded aggregate formation with ITCH. Our study also suggests a preferential interactions of ITCH with expanded polyglutamine proteins and further overexpression of this protein reduces aggresomes formation and mitigates the toxic insults generated by expanded polyglutamine proteins (Chhangani et al., 2014b).
Other developmental roles of ITCH are ubiquitylation of JunB for proteasomal degradation to carry out regulation of osteoblasts differentiation from mesenchymal progenitor cells, which may serve as the target of therapies for patients having bone loss (Zhang and Xing, 2013). ITCH also induces Numb-mediated suppression of Hedgehog signaling via ubiquitylation and degradation of transcription factor Gli1 (Di Marcotullio et al., 2006). Deficiency of ITCH has been linked with morphological and developmental abnormalities in addition to multisystem autoimmune disease, as described previously (Lohr et al., 2010). Studies, aiming to understand recognition and interaction of ITCH, may prove to be beneficial in regulating activities of this E3 ubiquitin ligase (Bellomaria et al., 2012; Upadhyay et al., 2015a), which can be further used in studying roles of ITCH in normal metabolism and developing therapeutic interventions against various protein conformational disorders. In the future, it is important to uncover the hidden potential of ITCH E3 ubiquitin ligase, which plays an important role in NDDs as wells as in neurodegenerative diseases. We have to investigate further how the loss-of-function of ITCH contributes to the progression of neurobiological pathomechanism conditions for both NDDs and neurodegeneration. Further, studies should be aimed to solve the problem of aberrant ITCH protein functions and its involvement in neuronal dysfunction.
MGRN1 Ultra-Compact Neuronal Functions Clean-Up Overload of Unwanted Accumulation of Abnormal Proteins, and Implications in Neuronal Developmental Disorders and Neurodegeneration
A recent review explains the neurobiological functions of another RING finger family member, i.e., MGRN1, an E3 ubiquitin ligase; lack of function of this E3 ubiquitin ligase causes developmental defects such as abnormal left-right axis patterning and congenital heart defects (Cota et al., 2006; Upadhyay et al., 2015b). Previous studies suggest that development of neurons and synaptogenesis are the highly controlled molecular mechanism. MGRN1 expression is also high in both human and mouse brain regions. How does the expression of MGRN1 determine neurodevelopmental events, left-right axis formation and how aberrant functions of MGRN1 cause congenital heart defects are not well-understood? The neurobiological functions of MGRN1 are largely unknown. Growing number of findings indicate the role of MGRN1 in neurodegenerative diseases. MGRN1 null mutants bring much more insights into neuronal functions of MGRN1; loss of MGRN1 causes oxidative stress and mitochondrial abnormalities linked with neurodegeneration (Cheng and Cohen, 2007; Sun et al., 2007).
Mahoganoid mutant mice exhibit the problem of spongiform neurodegeneration. Another study also indicates interaction of MGRN1 with both toxic cytosolic form (cyPrP) and transmembrane isoform linked with prion disease (CtmPrP) (He et al., 2003; Chakrabarti and Hegde, 2009). Interestingly, our recent findings represent cellular QC capacity of MGRN1 under various proteotoxic stress conditions and establish a neurobiological role of MGRN1 against toxic misfolded mutants of SOD1 and expanded polyglutamine proteins, which are involved in causing ALS and polyglutamine diseases, respectively (Chhangani et al., 2014a, 2016). As depicted in Figure 2C, recent studies have strongly suggested potential neurobiological roles of MGRN1 in NDDs and neurodegenerative diseases. All the above-summarized studies on MGRN1 show its roles in neurodevelopmental processes and in modulating the QC pathways under different neurodegenerative pathologies (Upadhyay et al., 2015b). These works have paved the way for identifying and characterizing novel E3 ubiquitin ligases, which may act as modulators for neurodevelopmental proteins and probably can also regulate proteostatic pathways, thus would be effectively able to control the occurrence of neurodegenerative disorders. Future research is important to identify the new mechanisms that explore the functions of MGRN1 in brain development, synaptogenesis, synaptic transmission and how the aberrant functioning of this protein contributes to human brain molecular pathophysiology.
HACE1 E3 Ubiquitin Ligase: Efforts Against Neurodevelopmental Disorders and Neurodegenerative Diseases
Another E3 ubiquitin ligase HACE1, which has an essential implication in NDDs and neurodegenerative diseases, also demonstrates an insight into the relationship between QC mechanism and neurobiological functions. HACE1 encodes a HECT domain-containing E3 ubiquitin ligase involved in the regulation of Rac1 and small GTPases. An exome sequencing analysis identified aberrant functional mutations in HACE1, which leads to autosomal recessive neurodevelopmental disorders with ID, spasticity, and abnormal gait (Hollstein et al., 2015). E3 ubiquitin ligase HACE1, along with UBCH7 E2 enzyme recognizes specific proteins for proteasomal degradation. It also targets Rac1 for polyubiquitylation and regulates its activity. Rac1 dysregulation generates neurodevelopmental abnormalities, whereas an increase in its function exhibits anterior cerebellar deficits, therefore Rac1 is important for defining cerebellar morphology patterning in mice (Torrino et al., 2011; Mulherkar et al., 2014).
Another interesting report indicates that HACE1 promotes the synthesis and stabilization of NRF2 protein, while the lack of function generates oxidative stress. HACE1 expression provides neuroprotective response against toxicity generated by mutant huntingtin protein and interestingly HACE1 level was found to be decreased in Huntington’s disease patients striatum (Rotblat et al., 2014). Pam is an E3 ubiquitin ligase that contains C-terminal RING finger domain and reduction of Pam activity in primary neurons causes downregulation of mTOR signaling and stabilization of tuberin. It is homologous to PHR protein family members and is involved in synaptogenesis (D’Souza et al., 2005; Han et al., 2012). How normal functions of Pam occupy a role in neurodevelopmental phases? Whether lack or aberrant functions of Pam can also aggravate the accumulation of misfolded proteins in cells? These are few unanswered questions for future studies linked with neurobiological functions of Pam E3 ubiquitin ligase. Different reports have shown that E3 ubiquitin ligases, like HACE1 and Pam, are suitable candidates, which take part in neurodevelopment, and may also modulate stress responses via different signaling mechanisms (Han et al., 2008; Rotblat et al., 2014; Hollstein et al., 2015). But, future endeavor based on the existing knowledge of these E3 ubiquitin ligases should focus more on how to effectively utilize this understanding in the development of a suitable therapy for treating disorders, which are affected by these signaling mechanisms.
E3 Ubiquitin Ligases are Crucial for Brain Development and their Aberrant Functions are Fatal
Most of the studies on E3 ubiquitin ligases are somewhere related to neurodegeneration and associated disorders, which commonly occur at old age (Chhangani et al., 2012; Upadhyay et al., 2015a). In developing brain, different E3 ubiquitin ligases play crucial roles (Stegmuller and Bonni, 2010). They participate in various processes of neuronal development, such as in synapse formation, neurogenesis, neurite enlargement, dendrite growth, axonal development, neural tube formation, and differentiation (Yan et al., 2009; Kawabe and Brose, 2011). Developmental events of neurons depend on various proteins, whose levels are regulated by their respective E3 ubiquitin ligases. Alterations in the levels of these substrate proteins due to loss-of-function or inactivation of E3 ubiquitin ligases have been shown to cause abnormal phenotypic outcomes and may result in neurodevelopmental disorders (Kuhnle et al., 2013; Morice-Picard et al., 2016). Despite being an interesting target, having multiple substrates with involvements in different signaling mechanisms, E3 ubiquitin ligases seem to be difficult candidates to be exploited for therapeutic applications. For example, ITCH suppresses the aggregation of cytoplasmic misfolded proteins (Chhangani et al., 2014b), while it also negatively regulates Hippo pathway that promotes tumorigenicity (Salah et al., 2011).
However, E3 ubiquitin ligase-mediated ubiquitylation and degradation have gained notable importance as an essential part of the early development of eukaryotes, including both animals and plants (Hellmann and Estelle, 2002; Stegmuller and Bonni, 2010). In Caenorhabditis elegans cullin RING ligases (CRLs) play an important role for the molecular mechanism of self-renewal and differentiation in embryonic cells during early embryogenesis. SKN-1 transcription factor mediates this differentiation process; and CRLs regulate this crucial molecular switching (Du et al., 2015). In the early C. elegans embryo regulation of asymmetry and persistence of the SKN-1 transcription factor also require a defined control, which is provided by a HECT E3 ubiquitin ligase, EEL-1 (Page et al., 2007). Downregulation of katanin, at an early embryogenesis stage of meiosis to mitosis transitions, is a necessity and this crucial step is majorly controlled by CUL-3 (Pintard et al., 2003). In an experimental model of the zebrafish embryo, Lnx-2a, an E3 ubiquitin ligase, balances the differentiation of exocrine cells in the early pancreatic bud, which could be achieved by fine tuning the Notch signaling via destabilization of Numb (Won et al., 2015).
Mammalian E3 ubiquitin ligases are also very crucial for the execution of various developmental processes, with striking control over neurogenesis (Stegmuller and Bonni, 2010). Hematopoietic stem cells highly express DDB1, a component of CUL4-DDB1 E3 ubiquitin ligase complex, which monitors stem cell divisions by ubiquitylation and proteasomal degradation of p53 (Gao et al., 2015). An early development transcription factor SOX-9, is ubiquitinated and degraded by Fbxw7 E3 ubiquitin ligase (Hong et al., 2016). ASB4 and ASB2/Cullin5/Rbx complexes degrade filamin B proteins, and play important roles in myogenic differentiation (Bello et al., 2009; Townley-Tilson et al., 2014); whereas, S-phase kinase-associated protein1-cullin1-F-box protein (SCFSkp2) complex plays important roles in differentiation of the neuronal cells (Boix-Perales et al., 2007). The functions of E3 ubiquitin ligases are required from the initiation of neuronal differentiation and up to the maturation of neurons and formation of synapses (Yi and Ehlers, 2007; Ding and Shen, 2008). Various mutations in E3 ubiquitin ligase genes and UPS dysfunction may lead to different kinds of abnormalities and disorders related to the neuronal development and functioning (Tai and Schuman, 2008; Kawabe and Brose, 2011). In Figure 3, we have schematically represented several reported E3 ubiquitin ligases that significantly contribute in regulation of various processes and pathways of neuronal development.
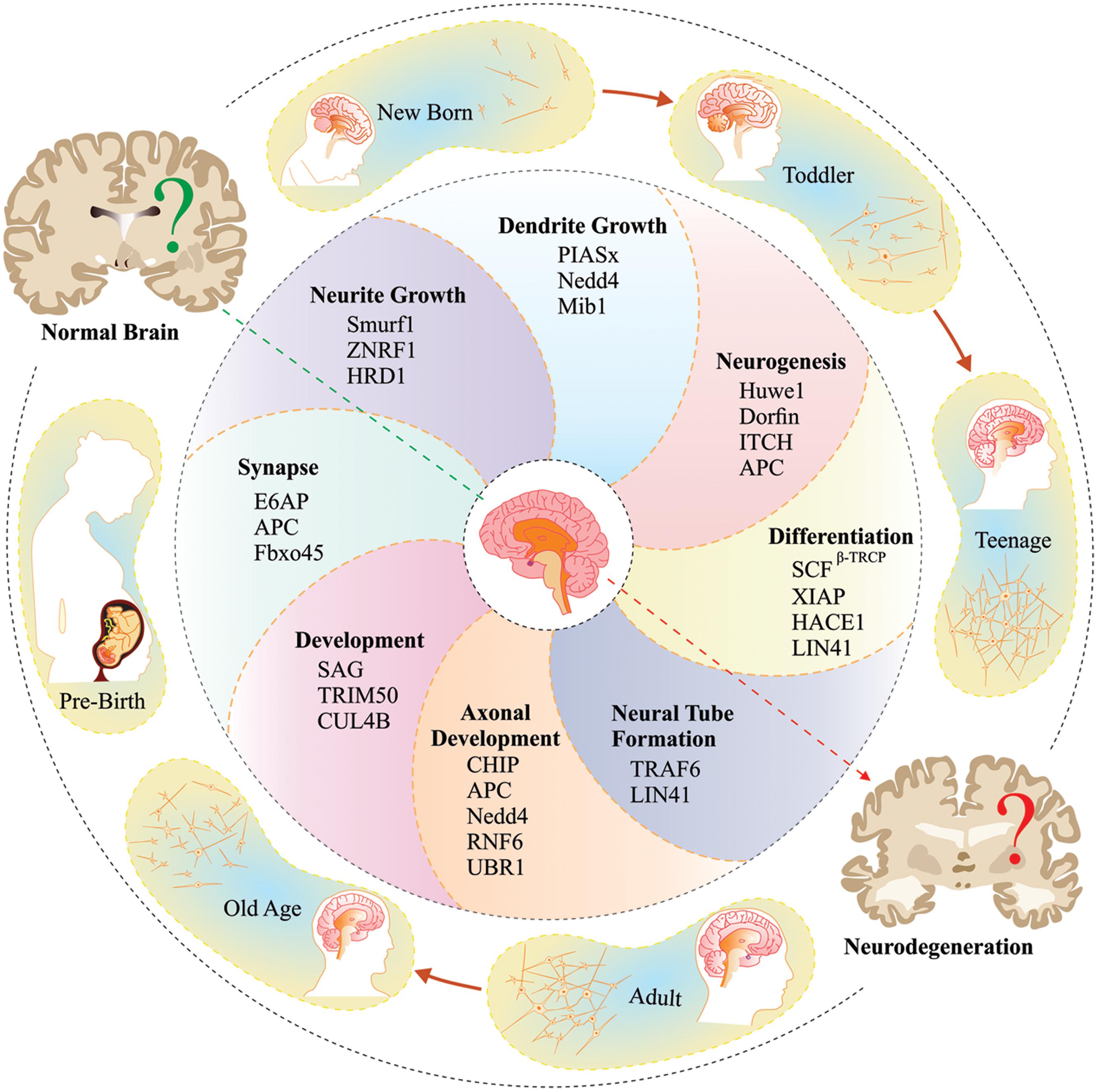
FIGURE 3. Proposed diagrammatic representations comprehend the neurobiological roles of few E3 ubiquitin ligases associated with normal brain development. The complex molecular mechanisms governed by these E3 ubiquitin ligases throughout the entire life span starting from learning skills (neurodevelopment) to kills (neurodegeneration) are still unknown.
Huwe1, an upstream regulator of N-Myc-DLL3-Notch signaling pathway restricts proliferation and initiates differentiation of neuronal stem cells (Zhao et al., 2009). Dorfin also plays an important role in neurogenesis; as reported in a recent study, Dorfin-/- mice show an overall decrease in the ubiquitylation level of various proteins (Park et al., 2015). Another crucial neurodevelopmental E3 ubiquitin ligase, involved in regulation of Notch signaling, is Mib, which ubiquitinate Delta followed by endocytosis (Itoh et al., 2003). XIAP plays regulatory roles in differentiation and neurogenesis, by controlling the formation of axons and dendrites; which is mediated by activation of MEK pathway (Fado et al., 2013). Other E3 ubiquitin ligases, like HACE1, and β-TRCP (SCFβ-TRCP), also have roles in controlling neuronal differentiation, via interaction and degradation of different client proteins (Westbrook et al., 2008; Hollstein et al., 2015). Lin41-mediated terminal differentiation sets the timing of neural tube closure, and mutation of this gene results in embryonic lethality (Maller Schulman et al., 2008). Similarly, deficiency of TRAF6 causes abnormal neural tube closure and may result in exencephaly (Lomaga et al., 2000).
Hrd1, an ER stress E3 ubiquitin ligase, facilitates induced neuronal differentiation and reduced neurite outgrowths (Kawada et al., 2014); whereas, Smurf1 promotes neurite outgrowth by ubiquitylation and proteasomal degradation of RhoA (Bryan et al., 2005). ZNRF1 E3 ubiquitin ligase mediates similar positive effects on neuritogenesis by interacting with tubulin (Yoshida et al., 2009). Mib E3 ubiquitin ligase also has significant control over neuritogenesis (Choe et al., 2007), as well as dendritic morphogenesis (Smrt et al., 2010). A study on granule neurons of cerebral cortex identified a critical role of PIASx, a SUMO E3 ubiquitin ligase, in postsynaptic dendritic differentiation (Shalizi et al., 2007). Ubiquitylation-mediated degradation of Rap2A and PTEN by NEDD4-1 are crucial events in dendritic development (Kawabe et al., 2010) and axonal branching, respectively (Drinjakovic et al., 2010). RNF6 and CHIP are also involved in axonal developmental processes by affecting the degradation of their development-associated substrates, viz., LIMK1 and katenin-p60, respectively (Tursun et al., 2005; Yang et al., 2013). Further, Ubr1-mediated N-end rule protein degradation of some unknown neurite outgrowth inhibitory protein also has critical involvement in neurite outgrowth and axonal regeneration processes (Kavakebi et al., 2005). Neuronal pruning is another crucial step of neurodevelopment, which is influenced by another E3 ubiquitin ligase, i.e., cullin1 based SCF complex (Wong et al., 2013).
Cell cycle regulatory E3 ubiquitin ligase, APC/C, has also been characterized for its determining roles in neuronal morphogenesis and connectivity (Yang et al., 2010). A complex of Cdh1-APC has shown control over axonal growth and patterning (Konishi et al., 2004), while Cdc20-APC E3 ubiquitin ligase has shown regulatory effects over dendritic morphogenesis and presynaptic axonal differentiation, helping synapse formation and development of neuronal circuits (Kim et al., 2009; Yang et al., 2009). Fbxo45, an F-box containing protein, instead of interacting with SCF, forms an E3 ubiquitin ligase complex with PAM, and this complex is important for neuronal migration and synapse formation at neuromuscular junctions (Saiga et al., 2009). SAG, a RING domain protein of SCF complex targets NF1 and modulates Ras-MAPK signaling pathway to affect neuronal development (Tan et al., 2011b).
There are several E3 ubiquitin ligases, whose loss-of-function has prominent roles in one or more developmental abnormalities or disorders. CUL4B mutation may result in X-linked mental retardation (Zou et al., 2007); whereas TRIM family of E3 ubiquitin ligases have been implicated in several developmental disorders, e.g., TRIM50 in Williams–Beuren syndrome (Micale et al., 2008), Midline 1 (MID1) in Opitz syndrome (Quaderi et al., 1997), and TRIM37 in Mulibrey Nanism (Kallijarvi et al., 2002). As discussed above, most of the substrates of E3 ubiquitin ligases are regulatory proteins involved in different signaling pathways, controlling cell cycle progression, cellular metabolism, apoptosis, etc., and hence may impact development and growth of organism (Bielskiene et al., 2015). Thus, disturbances caused in the ubiquitylation mechanism due to impairment in E3 ubiquitin ligases functionalities may lead to different kinds of abnormal conditions, developmental disorders, and embryonic lethality (Huang and Dixit, 2016). Therefore, identification and characterization of methodologies that may have the ability to modulate or stabilize the activities of E3 ubiquitin ligases may prove to be a beneficial strategy in controlling the progression of various disorders (Goldenberg et al., 2010).
The overall development of the brain is affected by multiple factors, including above described environmental factors and genes participating in neurodevelopmental processes; and various disease conditions could originate either due to their dysregulation or non-functional QC system of the cells (van Loo and Martens, 2007; Bale et al., 2010). Once neurodevelopmental period reaches its completion, several internal and external factors start presenting threats and challenges before neurons (Cannon and Greenamyre, 2011; Chin-Chan et al., 2015). A progressive decline in function of cellular defense mechanisms may drive organisms toward aging and result in late-age neurodegeneration (Pettegrew et al., 2000; Crawley, 2006). Age-related neurodegeneration is directly or indirectly associated with various diseases like Alzheimer’s, Parkinson’s, Huntington’s, ALS, and many more (Hung et al., 2010; Niccoli and Partridge, 2012). Roles of E3 ubiquitin ligases and the cellular PQC system have largely been explored and their deficiency has been attributed to several prominent diseases, associated with protein misfolding (Ardley and Robinson, 2004; Chhangani et al., 2012). Interestingly, several reports have shown that a well-regulated proteolysis, mediated by E3 ubiquitin ligases, is an important measure to ensure an error-proof development of organisms, which also holds true for plants (Vierstra, 1996; Sullivan et al., 2003).
There are number of E3 ubiquitin ligases, which have been identified in plants, affecting major developmental pathways, including perception of hormones and their respective signaling (Schwechheimer and Schwager, 2004; Stone and Callis, 2007). Abscisic acid signaling is an important regulator of plant growth and development at various stages, including seed dormancy and germination, embryonic development and reproduction, and vegetative growth, etc. (Wasilewska et al., 2008). Several E3 ubiquitin ligases have been identified for their roles in regulation and maintenance of abscisic acid biosynthesis and signaling pathways (Liu and Stone, 2011). CHIP (Luo et al., 2006), Keep on Going (KEG) (Stone et al., 2006), ABI3-interacting protein 2 (AIP2) (Zhang et al., 2005), and senescence-associated E3 ubiquitin ligase 1 (SAUL1) (Raab et al., 2009) are major regulators of ubiquitination and proteasomal degradation mediated control of the plant development. Auxins are the hormones that are implicated in plants root development. Transport inhibitor response 1 (TIR1), XB3 ortholog 2 in Arabidopsis thaliana (XBAT32), and seven-in-absentia of A. thaliana 5 (SINAT5) E3 ubiquitin ligases actively regulate auxin signaling and formation of lateral roots (Xie et al., 2002; Nodzon et al., 2004; Tan et al., 2007). Several other signaling pathways and mechanisms have also been explored in detail, where E3 ubiquitin ligases are extensively involved in development of plants (Moon et al., 2004; Stone and Callis, 2007; Chen and Hellmann, 2013).
It is now well-known that the environmental toxicants affect both, the processes of neurodevelopment, as well as neurodegeneration; and thus may increase the risk of brain-related disorders multiple folds (Gressens et al., 2001; Landrigan et al., 2005; Aschner and Costa, 2015). So, it is worthy to ask questions of how we can protect our cellular systems from toxicities and damages produced by these external factors. Considering the enormous potential of E3 ubiquitin ligases in the modulation of functions of intracellular proteins and regulation of cellular processes, it is obvious to question if we can modulate these mechanisms and pathways exogenously. To exploit therapeutic potential of E3 ubiquitin ligases, several attempts have been made to devise therapeutic strategies against various diseases.
E3 Ubiquitin Ligases: Possible Emerging Molecular Functions, Targets, and Therapeutic Applications
Past few years of research have documented several efforts toward the development of therapeutic strategies by exploiting druggability of these E3 ubiquitin ligases, by using many natural and synthetic small molecules, having modulating effects over these ligases. As shown in Table 1, we have summarized various available reports so far. Previous studies on natural molecules, like curcumin or diferuloylmethane, extracted from the rhizomes of herb Curcuma longa induces apoptosis in cancerous cells by inhibiting the Cdc27/APC3 component of APC, which is important for its ubiquitylation function (Lee and Langhans, 2012). Trehalose, a disaccharide has been reported to increase levels of CHIP and further induce autophagy in CHIP mutation related ataxia in fibroblast cells (Casarejos et al., 2014). A similar effect on the level of CHIP and autophagy induction has recently been reported for lanosterol, an intermediate compound of cholesterol biosynthesis (Upadhyay et al., 2017). MLN4924, an inhibitor of E3 ubiquitin ligase activity of SAG-SCF, has shown a promising effect in sensitizing leukemia cells toward the effects of retinoic acid (Tan et al., 2011a). It has also been shown that blocking the enzymatic activity of Nedd4, thereby modulating the budding of viruses, filoviruses, arenaviruses. and rhabdoviruses, may help in the development of a novel class of antiviral therapy (Han et al., 2014). A proteasome inhibitor, MG132 has also been shown to increase the level of Ubr1, and this may act as a useful therapeutic strategy in pathologies caused due to reduced amount of Ubr1, like pancreatic dysfunctions and mental abnormalities, characteristics of Johanson-Blizzard syndrome (Zenker et al., 2005). Similar effects of increasing E3 ubiquitin ligase activity have also been demonstrated for ZNRF1, in response to treatment of 6-hydroxydopamine (Wakatsuki et al., 2015).
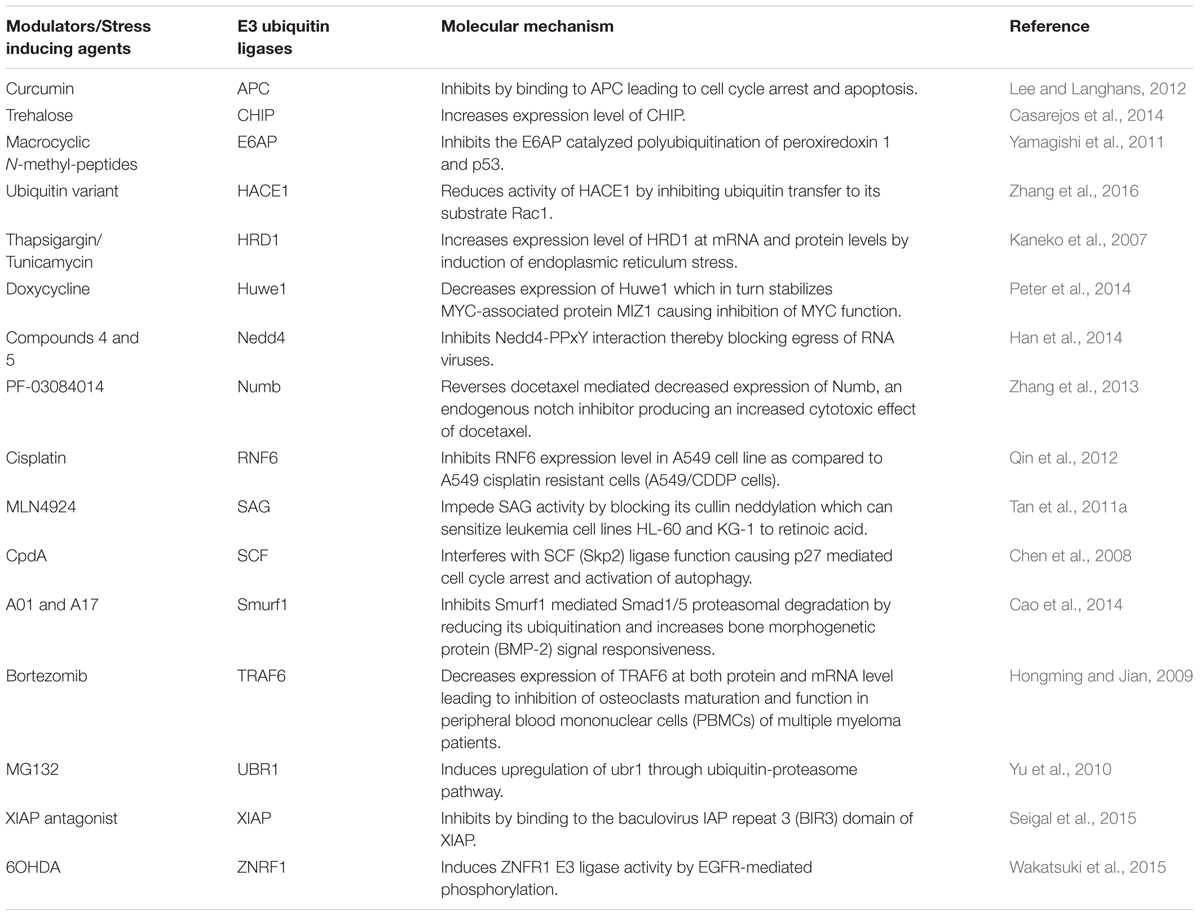
TABLE 1. Summarizes E3 ubiquitin ligases involved in process of neurodevelopment and promising molecules that have been shown to modulate their activity and retain possible therapeutic potential.
To devise the molecular therapeutics that can be used as potential strategies for modulating the functions and activities of various E3 ubiquitin ligases in treating multiple disorders, extensive studies are required, which can characterize these lead molecules for their efficacy, side effects, safety and pharmacological profiles. Although few drugs, such as Nutlin-3, a Mdm2-p53 interaction inhibitor, is used for treating pathology of cancer(Vassilev et al., 2004), but it is still in clinical trials and has also shown few side effects in the treatment of retinoblastoma (Secchiero et al., 2011). Development of ubiquitin variant probes having the abilities to target the ubiquitylation functions of E3 ubiquitin ligase can also prove to be an effective strategy in disease treatment (Zhang et al., 2016). Finding out solutions to these problems will help significantly in bringing these drugs or small molecules from basic research to the level of therapeutic applications.
The need of cost-effective therapies that have minimal side effects and which can reach up to every individual is not yet successfully fulfilled, and thus extensive exploration of natural and synthetic compounds will enable us to devise useful, and effective strategy to prevent and cure such diseases (Tsukamoto, 2016; Collins et al., 2017). The above mentioned small molecules are merely a fraction of research, which could be considered for upcoming strategies to treat fatal neurodegenerative disorders, cancer or other complex diseases, but there still remains a need for more research to be done (Eldridge and O’Brien, 2010; Gurevich and Gurevich, 2014; Skaar et al., 2014). Targeting specific E3 ubiquitin ligase for the treatment of particular disease is not an easy way to exercise in therapeutic applications, as these E3 ubiquitin ligases get involved in multiple pathways simultaneously. Therefore, there remains an immense need of understanding complete functionality and specificity, which these E3 ubiquitin ligases could provide in order to get the maximum beneficial outcome for future drug development strategies.
Key Questions and Future Prospective
All proteins originate through a common mechanism of synthesis, but they largely differ in their degradatory pathways (Ciechanover, 2005; Tai and Schuman, 2008). Neuronal cells, which have unique characteristics with a distinct morphology, critically require a well-regulated system of synthesis and degradation of proteins at the time of development, as well as in later life stages (Yi and Ehlers, 2007; Takano et al., 2015). They maintain a polar shape, in order to develop a functional neuronal circuitry (Tahirovic and Bradke, 2009). It is now evident from multiple studies that cellular proteolytic machinery is exquisitely implicated in the formation of synapses and neuronal circuitry, by maintaining the polarity of neurons (Murphey and Godenschwege, 2002; Bingol and Schuman, 2005). Several crucial E3 ubiquitin ligases, viz., TRIM2, TRIM46, and FBXO31-SCF, have been found to have a regulatory control over migration, polarization and synapse formation (Khazaei et al., 2011; Vadhvani et al., 2013; van Beuningen et al., 2015). A number of reports postulate the decisive involvement of PQC system in the maintenance of cellular proteostasis, and thus in ameliorating the toxicities generated by the accumulation of misfolded proteins in late-age. But, in past many years, indispensable roles of E3 ubiquitin ligases in proteasome-mediated proteolysis of crucial substrate proteins, which play important roles in several pathways of neuronal development, have been investigated in detail (Segref and Hoppe, 2008; Tai and Schuman, 2008; Yamada et al., 2013).
Major neurodevelopmental abnormalities are genetically complex disorders, and are caused by multifactorial aberrations in the developing brain. A very genuine challenge is to understand the precision of molecular pathomechanisms involved in NDDs. The future research on brain disorders must address the importance of QC E3 ubiquitin ligases in regulation of major signaling pathways that could link critical substrates involved in NDDs and neurodegenerative diseases. Dysregulation of protein synthesis, comprising proper folding of proteins, has found to be involved in induction of several stress-response pathways. Accumulation of abnormal proteins may affect the synaptic functions, neuronal connectivity and can also alter normal brain functions. Improper protein translation with a lack of cellular protein QC mechanism is the most prominent factor, which disturbs normal cellular proteostasis and can cause genetic diseases, neurodegeneration, and aging.
So far, we have not understood completely how cellular protein QC machinery contributes to the regulation of those genes, which are important for brain development and normal functions of brain throughout lifespan. Despite many findings in basic human molecular genetics reveal the underlying causes and thus enable us to challenge the occurrence of NDDs and neurodegeneration; still a conclusive solution to modulate the large number of genes and their end product proteins in a favorable manner at early and old age, is a real challenge in development of successful therapeutic strategies. It will be interesting in upcoming research to find out how QC E3 ubiquitin ligases control synapse formation and axon branching during developmental period. How these QC E3 ubiquitin ligases regulate protein synthesis, and prevent accumulation of toxic and non-functional proteins in neurons at later stages of life? Hence, in future, it is important to understand how we can tightly regulate cellular PQC mechanisms during early and later phases of life with the help of known cellular quality control markers such as QC E3 ubiquitin ligases.
Author Contributions
VJ, AU, AA, and RM, executed complete drawing of figures, NA and AP provided critical inputs. AM formulated the entire concept of manuscript and designed the initial draft of figures. All authors reviewed the manuscript.
Funding
AM acknowledges financial support from Extra Mural Research Funding (Individual Centric): Science and Engineering Research Board (SERB) EMR/2016/000716, Department of Science and Technology, Government of India. AP acknowledges financial support from Ramalingaswami Fellowship (BT/RLF/Re-entry/08/2013), Department of Biotechnology, Government of India, New Delhi. AU and VJ were supported by a research fellowship from University Grants Commission, Council for Scientific and Industrial Research, Government of India.
Conflict of Interest Statement
The authors declare that the research was conducted in the absence of any commercial or financial relationships that could be construed as a potential conflict of interest.
Acknowledgments
The authors would like to thank Mr. Bharat Pareek for his technical assistance and entire lab management during the manuscript preparation. We apologize to various authors whose work could not be included due to space limitations.
Abbreviations
ADHD, attention-deficit hyperactivity disorder; AIP4, atrophin-1-interacting protein 4; ALS, amyotrophic lateral sclerosis; APC/C, anaphase-promoting complex/cyclosome; APP, amyloid precursor protein; ASB4, ankyrin repeat and SOCS box containing protein 4; ASD, autism spectrum disorder; β-TRCP, β-transducin repeat-containing protein; CHIP, Carboxy-terminus of Hsc70-interacting protein; CNTNAP2, contactin-associated protein-like 2; CRL, cullin-RING like ubiquitin ligases; DDB1, DNA damage-binding protein 1; DISC1, disrupted-in-schizophrenia 1; E6-AP, E6-associated protein; EEL-1, enhancer of EfL-1 mutant phenotype; Fbxw, F-box and WD40 domain; FMR1, fragile X mental retardation; HACE1, HECT domain and ankyrin repeat containing E3 ubiquitin ligase 1; HECT, homologous to the E6-AP carboxyl terminus; ID, Intellectual disabilities; LIMK-1, LIM domain kinase 1; LIN41, lineage variant 41; Lnx, ligand of Numb protein-X; LTP, long-term potentiation; MAPK, Mitogen-activated protein kinase; MECP2, methyl-CpG-binding protein 2; MEK, MAPK ERK kinase; MGRN1, mahogunin RING finger protein 1; Mib1, mind bomb 1; MID1, midline-1; NDD, neurodevelopmental disorders; NDE1, nuclear distribution E homolog 1; NEDD4, neural precursor cell expressed developmentally down-regulated protein 4; NF-1, Neurofibromatosis type 1; NLGN, neuroligin; NRF2, nuclear factor erythroid 2-related factor 2; PAM, protein associated with Myc; PIAS, protein inhibitor of activated STAT; PINK1, PTEN-induced putative kinase 1; PMS, Phelan-McDermid syndrome; PQC, protein quality control; PTEN, phosphatase and tensin homolog; Rap2A, Ras-related protein 2A; RING, really interesting new gene; RNF, RING finger protein; SAG, sensitive to apoptosis gene; SCF, S-phase kinase-associated protein1 (SKP1)-cullin1 (CUL1)-F-box protein; SCZ, schizophrenia; SHANK3, SH3 and ankyrin domain-containing protein 3; SK2, small-conductance calcium-activated potassium channel 2; SKN-1, skinhead-1; SOD1, superoxide dismutase 1; SOX9, SRY homeobox 9; SUMO, small ubiquitin-like modifier; TDP43, transactive response DNA-binding protein-43; TRAF, TNF receptor associated factor; TRIM, tripartite motif; UBE3A, ubiquitin protein ligase E3A; UPS, ubiquitin-proteasome system; ZNRF1, zinc and ring finger 1.
References
Acconcia, F., Sigismund, S., and Polo, S. (2009). Ubiquitin in trafficking: the network at work. Exp. Cell Res. 315, 1610–1618. doi: 10.1016/j.yexcr.2008.10.014
Alarcon, M., Abrahams, B. S., Stone, J. L., Duvall, J. A., Perederiy, J. V., Bomar, J. M., et al. (2008). Linkage, association, and gene-expression analyses identify CNTNAP2 as an autism-susceptibility gene. Am. J. Hum. Genet. 82, 150–159. doi: 10.1016/j.ajhg.2007.09.005
Alkuraya, F. S., Cai, X., Emery, C., Mochida, G. H., Al-Dosari, M. S., Felie, J. M., et al. (2011). Human mutations in NDE1 cause extreme microcephaly with lissencephaly [corrected]. Am. J. Hum. Genet. 88, 536–547. doi: 10.1016/j.ajhg.2011.04.003
Amir, R. E., Van den Veyver, I. B., Wan, M., Tran, C. Q., Francke, U., and Zoghbi, H. Y. (1999). Rett syndrome is caused by mutations in X-linked MECP2, encoding methyl-CpG-binding protein 2. Nat. Genet. 23, 185–188. doi: 10.1038/13810
Ananthakrishnan, R., and Ehrlicher, A. (2007). The forces behind cell movement. Int. J. Biol. Sci. 3, 303–317. doi: 10.7150/ijbs.3.303
Archibald, J. M. (2015). Endosymbiosis and eukaryotic cell evolution. Curr. Biol. 25, R911–R921. doi: 10.1016/j.cub.2015.07.055
Ardley, H. C., and Robinson, P. A. (2004). The role of ubiquitin-protein ligases in neurodegenerative disease. Neurodegener. Dis. 1, 71–87. doi: 10.1159/000080048
Arndt, V., Rogon, C., and Höhfeld, J. (2007). To be, or not to be–molecular chaperones in protein degradation. Cell. Mol. Life Sci. 64, 2525–2541. doi: 10.1007/s00018-007-7188-6
Aruoma, O. I., Grootveld, M., and Bahorun, T. (2006). Free radicals in biology and medicine: from inflammation to biotechnology. Biofactors 27, 1–3. doi: 10.1002/biof.5520270101
Aschner, M., and Costa, L. G. (2015). Environmental Factors in Neurodevelopmental and Neurodegenerative Disorders. Boston, MA: Academic Press.
Bachmair, A., Finley, D., and Varshavsky, A. (1986). In vivo half-life of a protein is a function of its amino-terminal residue. Science 234, 179–186. doi: 10.1126/science.3018930
Balchin, D., Hayer-Hartl, M., and Hartl, F. U. (2016). In vivo aspects of protein folding and quality control. Science 353:aac4354. doi: 10.1126/science.aac4354
Bale, T. L., Baram, T. Z., Brown, A. S., Goldstein, J. M., Insel, T. R., McCarthy, M. M., et al. (2010). Early life programming and neurodevelopmental disorders. Biol. Psychiatry 68, 314–319. doi: 10.1016/j.biopsych.2010.05.028
Basha, M. R., Wei, W., Bakheet, S. A., Benitez, N., Siddiqi, H. K., Ge, Y. W., et al. (2005). The fetal basis of amyloidogenesis: exposure to lead and latent overexpression of amyloid precursor protein and beta-amyloid in the aging brain. J. Neurosci. 25, 823–829. doi: 10.1523/JNEUROSCI.4335-04.2005
Belle, A., Tanay, A., Bitincka, L., Shamir, R., and O’Shea, E. K. (2006). Quantification of protein half-lives in the budding yeast proteome. Proc. Natl. Acad. Sci. U.S.A. 103, 13004–13009. doi: 10.1073/pnas.0605420103
Bello, N. F., Lamsoul, I., Heuze, M. L., Metais, A., Moreaux, G., Calderwood, D. A., et al. (2009). The E3 ubiquitin ligase specificity subunit ASB2beta is a novel regulator of muscle differentiation that targets filamin B to proteasomal degradation. Cell Death Differ. 16, 921–932. doi: 10.1038/cdd.2009.27
Bellomaria, A., Barbato, G., Melino, G., Paci, M., and Melino, S. (2012). Recognition mechanism of p63 by the E3 ligase Itch: novel strategy in the study and inhibition of this interaction. Cell Cycle 11, 3638–3648. doi: 10.4161/cc.21918
Bertram, L., and Tanzi, R. E. (2005). The genetic epidemiology of neurodegenerative disease. J. Clin. Invest. 115, 1449–1457. doi: 10.1172/JCI24761
Betancur, C. (2011). Etiological heterogeneity in autism spectrum disorders: more than 100 genetic and genomic disorders and still counting. Brain Res. 1380, 42–77. doi: 10.1016/j.brainres.2010.11.078
Bharathi, Vasudevaraju, P., Govindaraju, M., Palanisamy, A. P., Sambamurti, K., and Rao, K. S. (2008). Molecular toxicity of aluminium in relation to neurodegeneration. Indian J. Med. Res. 128, 545–556.
Bielskiene, K., Bagdoniene, L., Mozuraitiene, J., Kazbariene, B., and Janulionis, E. (2015). E3 ubiquitin ligases as drug targets and prognostic biomarkers in melanoma. Medicina 51, 1–9. doi: 10.1016/j.medici.2015.01.007
Bingol, B., and Schuman, E. M. (2005). Synaptic protein degradation by the ubiquitin proteasome system. Curr. Opin. Neurobiol. 15, 536–541. doi: 10.1016/j.conb.2005.08.016
Boix-Perales, H., Horan, I., Wise, H., Lin, H. R., Chuang, L. C., Yew, P. R., et al. (2007). The E3 ubiquitin ligase skp2 regulates neural differentiation independent from the cell cycle. Neural Dev. 2:27. doi: 10.1186/1749-8104-2-27
Bonaglia, M. C., Giorda, R., Borgatti, R., Felisari, G., Gagliardi, C., Selicorni, A., et al. (2001). Disruption of the ProSAP2 gene in a t(12;22)(q24.1;q13.3) is associated with the 22q13.3 deletion syndrome. Am. J. Hum. Genet. 69, 261–268. doi: 10.1086/321293
Bradshaw Nicholas, J., Hennah, W., and Soares Dinesh, C. (2013). NDE1 and NDEL1: twin neurodevelopmental proteins with similar ‘nature’ but different ‘nurture’. Biomol. Concepts 4, 447–464.
Brown, L. A., Khousbouei, H., Goodwin, J. S., Irvin-Wilson, C. V., Ramesh, A., Sheng, L., et al. (2007). Down-regulation of early ionotrophic glutamate receptor subunit developmental expression as a mechanism for observed plasticity deficits following gestational exposure to benzo(a)pyrene. Neurotoxicology 28, 965–978. doi: 10.1016/j.neuro.2007.05.005
Brown, R. C., Lockwood, A. H., and Sonawane, B. R. (2005). Neurodegenerative diseases: an overview of environmental risk factors. Environ. Health Perspect. 113, 1250–1256. doi: 10.1289/ehp.7567
Bryan, B., Cai, Y., Wrighton, K., Wu, G., Feng, X. H., and Liu, M. (2005). Ubiquitination of RhoA by Smurf1 promotes neurite outgrowth. FEBS Lett. 579, 1015–1019. doi: 10.1016/j.febslet.2004.12.074
Bush, A. I., Pettingell, W. H., Multhaup, G. D., Paradis, M., Vonsattel, J. P., Gusella, J. F., et al. (1994). Rapid induction of Alzheimer A beta amyloid formation by zinc. Science 265, 1464–1467. doi: 10.1126/science.8073293
Cannon, J. R., and Greenamyre, J. T. (2011). The role of environmental exposures in neurodegeneration and neurodegenerative diseases. Toxicol. Sci. 124, 225–250. doi: 10.1093/toxsci/kfr239
Cao, Y., Wang, C., Zhang, X., Xing, G., Lu, K., Gu, Y., et al. (2014). Selective small molecule compounds increase BMP-2 responsiveness by inhibiting Smurf1-mediated Smad1/5 degradation. Sci. Rep. 4:4965. doi: 10.1038/srep04965
Casarejos, M. J., Perucho, J., Lopez-Sendon, J. L., Garcia de Yebenes, J., Bettencourt, C., Gomez, A., et al. (2014). Trehalose improves human fibroblast deficits in a new CHIP-mutation related ataxia. PLoS ONE 9:e106931. doi: 10.1371/journal.pone.0106931
Cayouette, M., and Raff, M. (2002). Asymmetric segregation of Numb: a mechanism for neural specification from Drosophila to mammals. Nat. Neurosci. 5, 1265–1269. doi: 10.1038/nn1202-1265
Chahrour, M., and Zoghbi, H. Y. (2007). The story of Rett syndrome: from clinic to neurobiology. Neuron 56, 422–437. doi: 10.1016/j.neuron.2007.10.001
Chakrabarti, O., and Hegde, R. S. (2009). Functional depletion of mahogunin by cytosolically exposed prion protein contributes to neurodegeneration. Cell 137, 1136–1147. doi: 10.1016/j.cell.2009.03.042
Chen, L., and Hellmann, H. (2013). Plant E3 ligases: flexible enzymes in a sessile world. Mol. Plant 6, 1388–1404. doi: 10.1093/mp/sst005
Chen, Q., Xie, W., Kuhn, D. J., Voorhees, P. M., Lopez-Girona, A., Mendy, D., et al. (2008). Targeting the p27 E3 ligase SCF(Skp2) results in p27- and Skp2-mediated cell-cycle arrest and activation of autophagy. Blood 111, 4690–4699. doi: 10.1182/blood-2007-09-112904
Cheng, T. H., and Cohen, S. N. (2007). Human MDM2 isoforms translated differentially on constitutive versus p53-regulated transcripts have distinct functions in the p53/MDM2 and TSG101/MDM2 feedback control loops. Mol. Cell. Biol. 27, 111–119. doi: 10.1128/MCB.00235-06
Chhangani, D., Endo, F., Amanullah, A., Upadhyay, A., Watanabe, S., Mishra, R., et al. (2016). Mahogunin ring finger 1 confers cytoprotection against mutant SOD1 aggresomes and is defective in an ALS mouse model. Neurobiol. Dis. 86, 16–28. doi: 10.1016/j.nbd.2015.11.017
Chhangani, D., Joshi, A. P., and Mishra, A. (2012). E3 ubiquitin ligases in protein quality control mechanism. Mol. Neurobiol. 45, 571–585. doi: 10.1007/s12035-012-8273-x
Chhangani, D., Nukina, N., Kurosawa, M., Amanullah, A., Joshi, V., Upadhyay, A., et al. (2014a). Mahogunin ring finger 1 suppresses misfolded polyglutamine aggregation and cytotoxicity. Biochim. Biophys. Acta 1842, 1472–1484. doi: 10.1016/j.bbadis.2014.04.014
Chhangani, D., Upadhyay, A., Amanullah, A., Joshi, V., and Mishra, A. (2014b). Ubiquitin ligase ITCH recruitment suppresses the aggregation and cellular toxicity of cytoplasmic misfolded proteins. Sci. Rep. 4:5077. doi: 10.1038/srep05077
Chin-Chan, M., Navarro-Yepes, J., and Quintanilla-Vega, B. (2015). Environmental pollutants as risk factors for neurodegenerative disorders: Alzheimer and Parkinson diseases. Front. Cell Neurosci. 9:124. doi: 10.3389/fncel.2015.00124
Ching, M. S., Shen, Y., Tan, W. H., Jeste, S. S., Morrow, E. M., Chen, X., et al. (2010). Deletions of NRXN1 (neurexin-1) predispose to a wide spectrum of developmental disorders. Am. J. Med. Genet. B Neuropsychiatr. Genet. 153B, 937–947. doi: 10.1002/ajmg.b.31063
Choe, E. A., Liao, L., Zhou, J. Y., Cheng, D., Duong, D. M., Jin, P., et al. (2007). Neuronal morphogenesis is regulated by the interplay between cyclin-dependent kinase 5 and the ubiquitin ligase mind bomb 1. J. Neurosci. 27, 9503–9512. doi: 10.1523/JNEUROSCI.1408-07.2007
Choi, J., and Donehower, L. A. (1999). p53 in embryonic development: maintaining a fine balance. Cell. Mol. Life Sci. 55, 38–47. doi: 10.1007/s000180050268
Ciechanover, A. (1994). The ubiquitin-proteasome proteolytic pathway. Cell 79, 13–22. doi: 10.1016/0092-8674(94)90396-4
Ciechanover, A. (2005). Proteolysis: from the lysosome to ubiquitin and the proteasome. Nat. Rev. Mol. Cell Biol. 6, 79–87. doi: 10.1038/nrm1552
Ciehanover, A., Hod, Y., and Hershko, A. (1978). A heat-stable polypeptide component of an ATP-dependent proteolytic system from reticulocytes. Biochem. Biophys. Res. Commun. 81, 1100–1105. doi: 10.1016/0006-291X(78)91249-4
Collins, I., Wang, H., Caldwell, J. J., and Chopra, R. (2017). Chemical approaches to targeted protein degradation through modulation of the ubiquitin-proteasome pathway. Biochem. J. 474, 1127–1147. doi: 10.1042/BCJ20160762
Cota, C. D., Bagher, P., Pelc, P., Smith, C. O., Bodner, C. R., and Gunn, T. M. (2006). Mice with mutations in Mahogunin ring finger-1 (Mgrn1) exhibit abnormal patterning of the left-right axis. Dev. Dyn. 235, 3438–3447. doi: 10.1002/dvdy.20992
Crawley, J. N. (2006). “Neurodevelopment and neurodegeneration,” in What’s Wrong With My Mouse?: Behavioral Phenotyping of Transgenic and Knockout Mice, ed. J. N. Crawley (Hoboken, NJ: John Wiley & Sons, Inc.), 290–320.
D’Andrea, A., and Pellman, D. (1998). Deubiquitinating enzymes: a new class of biological regulators. Crit. Rev. Biochem. Mol. Biol. 33, 337–352. doi: 10.1080/10409239891204251
Dennery, P. A. (2007). Effects of oxidative stress on embryonic development. Birth Defects Res. C Embryo Today 81, 155–162. doi: 10.1002/bdrc.20098
Di Marcotullio, L., Ferretti, E., Greco, A., De Smaele, E., Po, A., Sico, M. A., et al. (2006). Numb is a suppressor of Hedgehog signalling and targets Gli1 for Itch-dependent ubiquitination. Nat. Cell Biol. 8, 1415–1423. doi: 10.1038/ncb1510
DiFiglia, M., Sapp, E., Chase, K. O., Davies, S. W., Bates, G. P., Vonsattel, J., et al. (1997). Aggregation of huntingtin in neuronal intranuclear inclusions and dystrophic neurites in brain. Science 277, 1990–1993. doi: 10.1126/science.277.5334.1990
Dikic, I., and Robertson, M. (2012). Ubiquitin ligases and beyond. BMC Biol. 10:22. doi: 10.1186/1741-7007-10-22
Ding, M., and Shen, K. (2008). The role of the ubiquitin proteasome system in synapse remodeling and neurodegenerative diseases. Bioessays 30, 1075–1083. doi: 10.1002/bies.20843
Douglas, P. M., and Dillin, A. (2010). Protein homeostasis and aging in neurodegeneration. J. Cell Biol. 190, 719–729. doi: 10.1083/jcb.201005144
Drinjakovic, J., Jung, H., Campbell, D. S., Strochlic, L., Dwivedy, A., and Holt, C. E. (2010). E3 ligase Nedd4 promotes axon branching by downregulating PTEN. Neuron 65, 341–357. doi: 10.1016/j.neuron.2010.01.017
D’Souza, J., Hendricks, M., Le Guyader, S., Subburaju, S., Grunewald, B., Scholich, K., et al. (2005). Formation of the retinotectal projection requires Esrom, an ortholog of PAM (protein associated with Myc). Development 132, 247–256. doi: 10.1242/dev.01578
Du, Z., He, F., Yu, Z., Bowerman, B., and Bao, Z. (2015). E3 ubiquitin ligases promote progression of differentiation during C. elegans embryogenesis. Dev. Biol. 398, 267–279. doi: 10.1016/j.ydbio.2014.12.009
Dunker, A. K., Silman, I., Uversky, V. N., and Sussman, J. L. (2008). Function and structure of inherently disordered proteins. Curr. Opin. Struct. Biol. 18, 756–764. doi: 10.1016/j.sbi.2008.10.002
Eldridge, A. G., and O’Brien, T. (2010). Therapeutic strategies within the ubiquitin proteasome system. Cell Death Differ. 17, 4–13. doi: 10.1038/cdd.2009.82
Ernst, C. (2016). Proliferation and differentiation deficits are a major convergence point for neurodevelopmental disorders. Trends Neurosci. 39, 290–299. doi: 10.1016/j.tins.2016.03.001
Fado, R., Moubarak, R. S., Minano-Molina, A. J., Barneda-Zahonero, B., Valero, J., Saura, C. A., et al. (2013). X-linked inhibitor of apoptosis protein negatively regulates neuronal differentiation through interaction with cRAF and Trk. Sci. Rep. 3:2397. doi: 10.1038/srep02397
Fero, M. L., Rivkin, M., Tasch, M., Porter, P., Carow, C. E., Firpo, E., et al. (1996). A syndrome of multiorgan hyperplasia with features of gigantism, tumorigenesis, and female sterility in p27(Kip1)-deficient mice. Cell 85, 733–744. doi: 10.1016/S0092-8674(00)81239-8
Finch, C. E., and Tanzi, R. E. (1997). Genetics of aging. Science 278, 407–411. doi: 10.1126/science.278.5337.407
Finley, D. (2009). Recognition and processing of ubiquitin-protein conjugates by the proteasome. Annu. Rev. Biochem. 78, 477–513. doi: 10.1146/annurev.biochem.78.081507.101607
Frith, U., and Happé, F. (2005). Autism spectrum disorder. Curr. Biol. 15, R786–R790. doi: 10.1016/j.cub.2005.09.033
Gage, F. H., and Temple, S. (2013). Neural stem cells: generating and regenerating the brain. Neuron 80, 588–601. doi: 10.1016/j.neuron.2013.10.037
Gallastegui, N., and Groll, M. (2010). The 26S proteasome: assembly and function of a destructive machine. Trends Biochem. Sci. 35, 634–642. doi: 10.1016/j.tibs.2010.05.005
Gao, J., Buckley, S. M., Cimmino, L., Guillamot, M., Strikoudis, A., Cang, Y., et al. (2015). The CUL4-DDB1 ubiquitin ligase complex controls adult and embryonic stem cell differentiation and homeostasis. eLife 4:e07539. doi: 10.7554/eLife.07539
Germain, D. (2008). Ubiquitin-dependent and-independent mitochondrial protein quality controls: implications in ageing and neurodegenerative diseases. Mol. Microbiol. 70, 1334–1341. doi: 10.1111/j.1365-2958.2008.06502.x
Geschwind, D. H., and Levitt, P. (2007). Autism spectrum disorders: developmental disconnection syndromes. Curr. Opin. Neurobiol. 17, 103–111. doi: 10.1016/j.conb.2007.01.009
Gilbert, S. L., Dobyns, W. B., and Lahn, B. T. (2005). Genetic links between brain development and brain evolution. Nat. Rev. Genet. 6, 581–590. doi: 10.1038/nrg1634
Glickman, M. H., and Ciechanover, A. (2002). The ubiquitin-proteasome proteolytic pathway: destruction for the sake of construction. Physiol. Rev. 82, 373–428. doi: 10.1152/physrev.00027.2001
Goate, A., and Chartier-Harlin, M.-C. (1991). Segregation of a missense mutation in the amyloid precursor protein gene with familial Alzheimer’s disease. Nature 349, 704–706. doi: 10.1038/349704a0
Goldenberg, S. J., Marblestone, J. G., Mattern, M. R., and Nicholson, B. (2010). Strategies for the identification of ubiquitin ligase inhibitors. Biochem. Soc. Trans. 38, 132–136. doi: 10.1042/BST0380132
Goru, S. K., Pandey, A., and Gaikwad, A. B. (2016). E3 ubiquitin ligases as novel targets for inflammatory diseases. Pharmacol. Res. 106, 1–9. doi: 10.1016/j.phrs.2016.02.006
Gressens, P., Mesples, B., Sahir, N., Marret, S., and Sola, A. (2001). Environmental factors and disturbances of brain development. Semin. Neonatol. 6:185–194. doi: 10.1053/siny.2001.0048
Gurevich, E. V., and Gurevich, V. V. (2014). Therapeutic potential of small molecules and engineered proteins. Handb. Exp. Pharmacol. 219, 1–12. doi: 10.1007/978-3-642-41199-1_1
Haas, A. L., and Rose, I. A. (1982). The mechanism of ubiquitin activating enzyme. A kinetic and equilibrium analysis. J. Biol. Chem. 257, 10329–10337.
Hampton, R. Y., and Garza, R. M. (2009). Protein quality control as a strategy for cellular regulation: lessons from ubiquitin-mediated regulation of the sterol pathway. Chem. Rev. 109, 1561–1574. doi: 10.1021/cr800544v
Han, S., Kim, S., Bahl, S., Li, L., Burande, C. F., Smith, N., et al. (2012). The E3 ubiquitin ligase protein associated with Myc (Pam) regulates mammalian/mechanistic target of rapamycin complex 1 (mTORC1) signaling in vivo through N- and C-terminal domains. J. Biol. Chem. 287, 30063–30072. doi: 10.1074/jbc.M112.353987
Han, S., Witt, R. M., Santos, T. M., Polizzano, C., Sabatini, B. L., and Ramesh, V. (2008). Pam (Protein associated with Myc) functions as an E3 ubiquitin ligase and regulates TSC/mTOR signaling. Cell. Signal. 20, 1084–1091. doi: 10.1016/j.cellsig.2008.01.020
Han, Z., Lu, J., Liu, Y., Davis, B., Lee, M. S., Olson, M. A., et al. (2014). Small-molecule probes targeting the viral PPxY-host Nedd4 interface block egress of a broad range of RNA viruses. J. Virol. 88, 7294–7306. doi: 10.1128/JVI.00591-14
Harbord, M. (2001). Levodopa responsive Parkinsonism in adults with Angelman Syndrome. J. Clin. Neurosci. 8, 421–422. doi: 10.1054/jocn.2000.0753
Hartl, F. U., Bracher, A., and Hayer-Hartl, M. (2011). Molecular chaperones in protein folding and proteostasis. Nature 475, 324–332. doi: 10.1038/nature10317
Hartl, F. U., and Hayer-Hartl, M. (2009). Converging concepts of protein folding in vitro and in vivo. Nat. Struct. Mol. Biol. 16, 574–581. doi: 10.1038/nsmb.1591
He, L., Lu, X. Y., Jolly, A. F., Eldridge, A. G., Watson, S. J., Jackson, P. K., et al. (2003). Spongiform degeneration in mahoganoid mutant mice. Science 299, 710–712. doi: 10.1126/science.1079694
Hellmann, H., and Estelle, M. (2002). Plant development: regulation by protein degradation. Science 297, 793–797. doi: 10.1126/science.1072831
Hershko, A., and Ciechanover, A. (1992). The ubiquitin system for protein degradation. Annu. Rev. Biochem. 61, 761–807. doi: 10.1146/annurev.bi.61.070192.003553
Hershko, A., Ciechanover, A., and Varshavsky, A. (2000). The ubiquitin system. Nat. Med. 6, 1073–1081. doi: 10.1038/80384
Hershko, A., Heller, H., Elias, S., and Ciechanover, A. (1983). Components of ubiquitin-protein ligase system. Resolution, affinity purification, and role in protein breakdown. J. Biol. Chem. 258, 8206–8214.
Hilt, W., and Wolf, D. H. (2000). Proteasomes: The World of Regulatory Proteolysis. Austin, TX: Landes Bioscience.
Ho, S. M., Tang, W. Y., Belmonte de Frausto, J., and Prins, G. S. (2006). Developmental exposure to estradiol and bisphenol A increases susceptibility to prostate carcinogenesis and epigenetically regulates phosphodiesterase type 4 variant 4. Cancer Res. 66, 5624–5632. doi: 10.1158/0008-5472.CAN-06-0516
Hollstein, R., Parry, D. A., Nalbach, L., Logan, C. V., Strom, T. M., Hartill, V. L., et al. (2015). HACE1 deficiency causes an autosomal recessive neurodevelopmental syndrome. J. Med. Genet. 52, 797–803. doi: 10.1136/jmedgenet-2015-103344
Hong, X., Liu, W., Song, R., Shah, J. J., Feng, X., Tsang, C. K., et al. (2016). SOX9 is targeted for proteasomal degradation by the E3 ligase FBW7 in response to DNA damage. Nucleic Acids Res. 44, 8855–8869. doi: 10.1093/nar/gkw748
Hongming, H., and Jian, H. (2009). Bortezomib inhibits maturation and function of osteoclasts from PBMCs of patients with multiple myeloma by downregulating TRAF6. Leuk. Res. 33, 115–122. doi: 10.1016/j.leukres.2008.07.028
Huang, C. (2010). Roles of E3 ubiquitin ligases in cell adhesion and migration. Cell Adh. Migr. 4, 10–18. doi: 10.4161/cam.4.1.9834
Huang, H. S., Allen, J. A., Mabb, A. M., King, I. F., Miriyala, J., Taylor-Blake, B., et al. (2011). Topoisomerase inhibitors unsilence the dormant allele of Ube3a in neurons. Nature 481, 185–189. doi: 10.1038/nature10726
Huang, X., and Dixit, V. M. (2016). Drugging the undruggables: exploring the ubiquitin system for drug development. Cell Res. 26, 484–498. doi: 10.1038/cr.2016.31
Huizink, A. C., and Mulder, E. J. (2006). Maternal smoking, drinking or cannabis use during pregnancy and neurobehavioral and cognitive functioning in human offspring. Neurosci. Biobehav. Rev. 30, 24–41. doi: 10.1016/j.neubiorev.2005.04.005
Hung, C. W., Chen, Y. C., Hsieh, W. L., Chiou, S. H., and Kao, C. L. (2010). Ageing and neurodegenerative diseases. Ageing Res. Rev. 9(Suppl. 1), S36–S46. doi: 10.1016/j.arr.2010.08.006
Hutton, M., Lendon, C. L., Rizzu, P., Baker, M., Froelich, S., Houlden, H., et al. (1998). Association of missense and 5’-splice-site mutations in tau with the inherited dementia FTDP-17. Nature 393, 702–705. doi: 10.1038/31508
Huxley, R. R., Shiell, A. W., and Law, C. M. (2000). The role of size at birth and postnatal catch-up growth in determining systolic blood pressure: a systematic review of the literature. J. Hypertens. 18, 815–831. doi: 10.1097/00004872-200018070-00002
Iguchi, Y., Katsuno, M., Niwa, J.-I., Takagi, S., Ishigaki, S., Ikenaka, K., et al. (2013). Loss of TDP-43 causes age-dependent progressive motor neuron degeneration. Brain 136, 1371–1382. doi: 10.1093/brain/awt029
Itoh, M., Kim, C. H., Palardy, G., Oda, T., Jiang, Y. J., Maust, D., et al. (2003). Mind bomb is a ubiquitin ligase that is essential for efficient activation of Notch signaling by Delta. Dev. Cell 4, 67–82. doi: 10.1016/S1534-5807(02)00409-4
Jamain, S., Quach, H., Betancur, C., Rastam, M., Colineaux, C., Gillberg, I. C., et al. (2003). Mutations of the X-linked genes encoding neuroligins NLGN3 and NLGN4 are associated with autism. Nat. Genet. 34, 27–29. doi: 10.1038/ng1136
Jiang, Y. H., Armstrong, D., Albrecht, U., Atkins, C. M., Noebels, J. L., Eichele, G., et al. (1998). Mutation of the Angelman ubiquitin ligase in mice causes increased cytoplasmic p53 and deficits of contextual learning and long-term potentiation. Neuron 21, 799–811. doi: 10.1016/S0896-6273(00)80596-6
Jin, P., and Warren, S. T. (2000). Understanding the molecular basis of fragile X syndrome. Hum. Mol. Genet. 9, 901–908. doi: 10.1093/hmg/9.6.901
Jirtle, R. L., and Skinner, M. K. (2007). Environmental epigenomics and disease susceptibility. Nat. Rev. Genet. 8, 253–262. doi: 10.1038/nrg2045
Joshi, V., Amanullah, A., Upadhyay, A., Mishra, R., Kumar, A., and Mishra, A. (2016). A decade of boon or burden: What has the CHIP ever done for cellular protein quality control mechanism implicated in neurodegeneration and aging? Front. Mol. Neurosci. 9:93. doi: 10.3389/fnmol.2016.00093
Kallijarvi, J., Avela, K., Lipsanen-Nyman, M., Ulmanen, I., and Lehesjoki, A. E. (2002). The TRIM37 gene encodes a peroxisomal RING-B-box-coiled-coil protein: classification of mulibrey nanism as a new peroxisomal disorder. Am. J. Hum. Genet. 70, 1215–1228. doi: 10.1086/340256
Kampa, M., and Castanas, E. (2008). Human health effects of air pollution. Environ. Pollut. 151, 362–367. doi: 10.1016/j.envpol.2007.06.012
Kanazawa, I. (2001). How do neurons die in neurodegenerative diseases? Trends Mol. Med. 7, 339–344. doi: 10.1016/S1471-4914(01)02017-2
Kaneko, M., Yasui, S., Niinuma, Y., Arai, K., Omura, T., Okuma, Y., et al. (2007). A different pathway in the endoplasmic reticulum stress-induced expression of human HRD1 and SEL1 genes. FEBS Lett. 581, 5355–5360. doi: 10.1016/j.febslet.2007.10.033
Kang, S. C., and Lee, B. M. (2005). DNA methylation of estrogen receptor alpha gene by phthalates. J. Toxicol. Environ. Health A 68, 1995–2003. doi: 10.1080/15287390491008913
Kanthasamy, A., Jin, H., Anantharam, V., Sondarva, G., Rangasamy, V., Rana, A., et al. (2012). Emerging neurotoxic mechanisms in environmental factors-induced neurodegeneration. Neurotoxicology 33, 833–837. doi: 10.1016/j.neuro.2012.01.011
Kaushik, S., and Cuervo, A. M. (2015). Proteostasis and aging. Nat. Med. 21, 1406–1415. doi: 10.1038/nm.4001
Kavakebi, P., Hausott, B., Tomasino, A., Ingorokva, S., and Klimaschewski, L. (2005). The N-end rule ubiquitin-conjugating enzyme, HR6B, is up-regulated by nerve growth factor and required for neurite outgrowth. Mol. Cell. Neurosci. 29, 559–568. doi: 10.1016/j.mcn.2005.04.008
Kawabe, H., and Brose, N. (2011). The role of ubiquitylation in nerve cell development. Nat. Rev. Neurosci. 12, 251–268. doi: 10.1038/nrn3009
Kawabe, H., Neeb, A., Dimova, K., Young, S. M. Jr., Takeda, M., Katsurabayashi, S., et al. (2010). Regulation of Rap2A by the ubiquitin ligase Nedd4-1 controls neurite development. Neuron 65, 358–372. doi: 10.1016/j.neuron.2010.01.007
Kawada, K., Iekumo, T., Saito, R., Kaneko, M., Mimori, S., Nomura, Y., et al. (2014). Aberrant neuronal differentiation and inhibition of dendrite outgrowth resulting from endoplasmic reticulum stress. J. Neurosci. Res. 92, 1122–1133. doi: 10.1002/jnr.23389
Khazaei, M. R., Bunk, E. C., Hillje, A. L., Jahn, H. M., Riegler, E. M., Knoblich, J. A., et al. (2011). The E3-ubiquitin ligase TRIM2 regulates neuronal polarization. J. Neurochem. 117, 29–37. doi: 10.1111/j.1471-4159.2010.06971.x
Kim, A. H., Puram, S. V., Bilimoria, P. M., Ikeuchi, Y., Keough, S., Wong, M., et al. (2009). A centrosomal Cdc20-APC pathway controls dendrite morphogenesis in postmitotic neurons. Cell 136, 322–336. doi: 10.1016/j.cell.2008.11.050
Kim, M. S., Pinto, S. M., Getnet, D., Nirujogi, R. S., Manda, S. S., Chaerkady, R., et al. (2014). A draft map of the human proteome. Nature 509, 575–581. doi: 10.1038/nature13302
Kim, Y. E., Hipp, M. S., Bracher, A., Hayer-Hartl, M., and Ulrich Hartl, F. (2013). Molecular chaperone functions in protein folding and proteostasis. Annu. Rev. Biochem. 82, 323–355. doi: 10.1146/annurev-biochem-060208-092442
Kimura, H., Tsuboi, D., Wang, C., Kushima, I., Koide, T., Ikeda, M., et al. (2015). Identification of Rare, single-nucleotide mutations in NDE1 and their contributions to schizophrenia susceptibility. Schizophr. Bull. 41, 744–753. doi: 10.1093/schbul/sbu147
Kishino, T., Lalande, M., and Wagstaff, J. (1997). UBE3A/E6-AP mutations cause Angelman syndrome. Nat. Genet. 15, 70–73. doi: 10.1038/ng0197-70
Kitada, T., Asakawa, S., Hattori, N., Matsumine, H., Yamamura, Y., Minoshima, S., et al. (1998). Mutations in the parkin gene cause autosomal recessive juvenile parkinsonism. Nature 392, 605–608. doi: 10.1038/33416
Klein, A. L., Zilian, O., Suter, U., and Taylor, V. (2004). Murine numb regulates granule cell maturation in the cerebellum. Dev. Biol. 266, 161–177. doi: 10.1016/j.ydbio.2003.10.017
Knobloch, H., and Pasamanick, B. (1960). Environmental factors affecting human development, before and after birth. Pediatrics 26, 210–218.
Koga, H., Kaushik, S., and Cuervo, A. M. (2011). Protein homeostasis and aging: the importance of exquisite quality control. Ageing Res. Rev. 10, 205–215. doi: 10.1016/j.arr.2010.02.001
Komander, D., Clague, M. J., and Urbé, S. (2009). Breaking the chains: structure and function of the deubiquitinases. Nat. Rev. Mol. Cell Biol. 10, 550–563. doi: 10.1038/nrm2731
Komander, D., and Rape, M. (2012). The ubiquitin code. Annu. Rev. Biochem. 81, 203–229. doi: 10.1146/annurev-biochem-060310-170328
Konishi, Y., Stegmuller, J., Matsuda, T., Bonni, S., and Bonni, A. (2004). Cdh1-APC controls axonal growth and patterning in the mammalian brain. Science 303, 1026–1030. doi: 10.1126/science.1093712
Koturbash, I., Baker, M., Loree, J., Kutanzi, K., Hudson, D., Pogribny, I., et al. (2006). Epigenetic dysregulation underlies radiation-induced transgenerational genome instability in vivo. Int. J. Radiat. Oncol. Biol. Phys. 66, 327–330. doi: 10.1016/j.ijrobp.2006.06.012
Kuang, E., Qi, J., and Ronai, Z. (2013). Emerging roles of E3 ubiquitin ligases in autophagy. Trends Biochem. Sci. 38, 453–460. doi: 10.1016/j.tibs.2013.06.008
Kuhnle, S., Mothes, B., Matentzoglu, K., and Scheffner, M. (2013). Role of the ubiquitin ligase E6AP/UBE3A in controlling levels of the synaptic protein Arc. Proc. Natl. Acad. Sci. U.S.A. 110, 8888–8893. doi: 10.1073/pnas.1302792110
La Spada, A. R., Wilson, E. M., Lubahn, D. B., Harding, A. E., and Fischbeck, K. H. (1991). Androgen receptor gene mutations in X-linked spinal and bulbar muscular atrophy. Nature 352, 77–79. doi: 10.1038/352077a0
Landrigan, P. J., Sonawane, B., Butler, R. N., Trasande, L., Callan, R., and Droller, D. (2005). Early environmental origins of neurodegenerative disease in later life. Environ. Health Perspect. 113 1230–1233. doi: 10.1289/ehp.7571
Lee, S. J., and Langhans, S. A. (2012). Anaphase-promoting complex/cyclosome protein Cdc27 is a target for curcumin-induced cell cycle arrest and apoptosis. BMC Cancer 12:44. doi: 10.1186/1471-2407-12-44
Lenroot, R. K., and Giedd, J. N. (2008). The changing impact of genes and environment on brain development during childhood and adolescence: initial findings from a neuroimaging study of pediatric twins. Dev. Psychopathol. 20, 1161–1175. doi: 10.1017/S0954579408000552
Lilienbaum, A. (2013). Relationship between the proteasomal system and autophagy. Int. J. Biochem. Mol. Biol. 4, 1–26.
Liu, H., and Stone, S. L. (2011). E3 ubiquitin ligases and abscisic acid signaling. Plant Signal. Behav. 6, 344–348. doi: 10.4161/psb.6.3.13914
Liu, Y. C. (2004). Ubiquitin ligases and the immune response. Annu. Rev. Immunol. 22, 81–127. doi: 10.1146/annurev.immunol.22.012703.104813
Lohr, N. J., Molleston, J. P., Strauss, K. A., Torres-Martinez, W., Sherman, E. A., Squires, R. H., et al. (2010). Human ITCH E3 ubiquitin ligase deficiency causes syndromic multisystem autoimmune disease. Am. J. Hum. Genet. 86, 447–453. doi: 10.1016/j.ajhg.2010.01.028
Lomaga, M. A., Henderson, J. T., Elia, A. J., Robertson, J., Noyce, R. S., Yeh, W. C., et al. (2000). Tumor necrosis factor receptor-associated factor 6 (TRAF6) deficiency results in exencephaly and is required for apoptosis within the developing CNS. J. Neurosci. 20, 7384–7393.
Lord, C., Cook, E. H., Leventhal, B. L., and Amaral, D. G. (2000). Autism spectrum disorders. Neuron 28, 355–363. doi: 10.1016/S0896-6273(00)00115-X
Luo, J., Shen, G., Yan, J., He, C., and Zhang, H. (2006). AtCHIP functions as an E3 ubiquitin ligase of protein phosphatase 2A subunits and alters plant response to abscisic acid treatment. Plant J. 46, 649–657. doi: 10.1111/j.1365-313X.2006.02730.x
Maller Schulman, B. R., Liang, X., Stahlhut, C., DelConte, C., Stefani, G., and Slack, F. J. (2008). The let-7 microRNA target gene, Mlin41/Trim71 is required for mouse embryonic survival and neural tube closure. Cell Cycle 7, 3935–3942. doi: 10.4161/cc.7.24.7397
Mayer, R. J., Ciechanover, A. J., and Rechsteiner, M. (2005). Protein Degradation: Ubiquitin and the Chemistry of Life. Weinheim: Wiley.
McCormack, A. L., and Di Monte, D. A. (2003). Effects of L-dopa and other amino acids against paraquat-induced nigrostriatal degeneration. J. Neurochem. 85, 82–86. doi: 10.1046/j.1471-4159.2003.01621.x
McGill, M. A., and McGlade, C. J. (2003). Mammalian numb proteins promote Notch1 receptor ubiquitination and degradation of the Notch1 intracellular domain. J. Biol. Chem. 278, 23196–23203. doi: 10.1074/jbc.M302827200
Meng, L., Person, R. E., Huang, W., Zhu, P. J., Costa-Mattioli, M., and Beaudet, A. L. (2013). Truncation of Ube3a-ATS unsilences paternal Ube3a and ameliorates behavioral defects in the Angelman syndrome mouse model. PLoS Genet. 9:e1004039. doi: 10.1371/journal.pgen.1004039
Meng, L., Ward, A. J., Chun, S., Bennett, C. F., Beaudet, A. L., and Rigo, F. (2015). Towards a therapy for Angelman syndrome by targeting a long non-coding RNA. Nature 518, 409–412. doi: 10.1038/nature13975
Micale, L., Fusco, C., Augello, B., Napolitano, L. M., Dermitzakis, E. T., Meroni, G., et al. (2008). Williams-Beuren syndrome TRIM50 encodes an E3 ubiquitin ligase. Eur. J. Hum. Genet. 16, 1038–1049. doi: 10.1038/ejhg.2008.68
Mick, E., Biederman, J., Faraone, S. V., Sayer, J., and Kleinman, S. (2002). Case-control study of attention-deficit hyperactivity disorder and maternal smoking, alcohol use, and drug use during pregnancy. J. Am. Acad. Child Adolesc. Psychiatry 41, 378–385. doi: 10.1097/00004583-200204000-00009
Miles, J. H. (2011). Autism spectrum disorders—a genetics review. Genet. Med. 13, 278–294. doi: 10.1097/GIM.0b013e3181ff67ba
Millar, J. K., Wilson-Annan, J. C., Anderson, S., Christie, S., Taylor, M. S., Semple, C. A., et al. (2000). Disruption of two novel genes by a translocation co-segregating with schizophrenia. Hum. Mol. Genet. 9, 1415–1423. doi: 10.1093/hmg/9.9.1415
Mishra, A., Dikshit, P., Purkayastha, S., Sharma, J., Nukina, N., and Jana, N. R. (2008). E6-AP promotes misfolded polyglutamine proteins for proteasomal degradation and suppresses polyglutamine protein aggregation and toxicity. J. Biol. Chem. 283, 7648–7656. doi: 10.1074/jbc.M706620200
Mishra, A., Godavarthi, S. K., and Jana, N. R. (2009a). UBE3A/E6-AP regulates cell proliferation by promoting proteasomal degradation of p27. Neurobiol. Dis. 36, 26–34. doi: 10.1016/j.nbd.2009.06.010
Mishra, A., Godavarthi, S. K., Maheshwari, M., Goswami, A., and Jana, N. R. (2009b). The ubiquitin ligase E6-AP is induced and recruited to aggresomes in response to proteasome inhibition and may be involved in the ubiquitination of Hsp70-bound misfolded proteins. J. Biol. Chem. 284, 10537–10545. doi: 10.1074/jbc.M806804200
Mishra, A., and Jana, N. R. (2008). Regulation of turnover of tumor suppressor p53 and cell growth by E6-AP, a ubiquitin protein ligase mutated in Angelman mental retardation syndrome. Cell. Mol. Life Sci. 65, 656–666. doi: 10.1007/s00018-007-7476-1
Mishra, A., Maheshwari, M., Chhangani, D., Fujimori-Tonou, N., Endo, F., Joshi, A. P., et al. (2013). E6-AP association promotes SOD1 aggresomes degradation and suppresses toxicity. Neurobiol. Aging 34, 1310.e11–1310.e23. doi: 10.1016/j.neurobiolaging.2012.08.016
Mitchell, K. J. (2011). The genetics of neurodevelopmental disease. Curr. Opin. Neurobiol. 21, 197–203. doi: 10.1016/j.conb.2010.08.009
Moon, J., Parry, G., and Estelle, M. (2004). The ubiquitin-proteasome pathway and plant development. Plant Cell 16, 3181–3195. doi: 10.1105/tpc.104.161220
Morice-Picard, F., Benard, G., Rezvani, H. R., Lasseaux, E., Simon, D., Moutton, S., et al. (2016). Complete loss of function of the ubiquitin ligase HERC2 causes a severe neurodevelopmental phenotype. Eur. J. Hum. Genet. 25, 52–58. doi: 10.1038/ejhg.2016.139
Morimoto, R. I. (2008). Proteotoxic stress and inducible chaperone networks in neurodegenerative disease and aging. Genes Dev. 22, 1427–1438. doi: 10.1101/gad.1657108
Mulherkar, S., Uddin, M. D., Couvillon, A. D., Sillitoe, R. V., and Tolias, K. F. (2014). The small GTPases RhoA and Rac1 regulate cerebellar development by controlling cell morphogenesis, migration and foliation. Dev. Biol. 394, 39–53. doi: 10.1016/j.ydbio.2014.08.004
Murphey, R. K., and Godenschwege, T. A. (2002). New roles for ubiquitin in the assembly and function of neuronal circuits. Neuron 36, 5–8. doi: 10.1016/S0896-6273(02)00943-1
Nakayama, K. I., and Nakayama, K. (2006). Ubiquitin ligases: cell-cycle control and cancer. Nat. Rev. Cancer 6, 369–381. doi: 10.1038/nrc1881
Niccoli, T., and Partridge, L. (2012). Ageing as a risk factor for disease. Curr. Biol. 22, R741–R752. doi: 10.1016/j.cub.2012.07.024
Nodzon, L. A., Xu, W. H., Wang, Y., Pi, L. Y., Chakrabarty, P. K., and Song, W. Y. (2004). The ubiquitin ligase XBAT32 regulates lateral root development in Arabidopsis. Plant J. 40, 996–1006. doi: 10.1111/j.1365-313X.2004.02266.x
Norris, V., and Root-Bernstein, R. (2009). The eukaryotic cell originated in the integration and redistribution of hyperstructures from communities of prokaryotic cells based on molecular complementarity. Int. J. Mol. Sci. 10, 2611–2632. doi: 10.3390/ijms10062611
Nunomura, A., Perry, G., Aliev, G., Hirai, K., Takeda, A., Balraj, E. K., et al. (2001). Oxidative damage is the earliest event in Alzheimer disease. J. Neuropathol. Exp. Neurol. 60, 759–767. doi: 10.1093/jnen/60.8.759
Ornoy, A., and Ergaz, Z. (2010). Alcohol abuse in pregnant women: effects on the fetus and newborn, mode of action and maternal treatment. Int. J. Environ. Res. Public Health 7, 364–379. doi: 10.3390/ijerph7020364
Orr, H. T., Chung, M.-Y., Banfi, S., Kwiatkowski, T. J., Servadio, A., Beaudet, A. L., et al. (1993). Expansion of an unstable trinucleotide CAG repeat in spinocerebellar ataxia type 1. Nat. Genet. 4, 221–226. doi: 10.1038/ng0793-221
Ouellette, E. M., Rosett, H. L., Rosman, N. P., and Weiner, L. (1977). Adverse effects on offspring of maternal alcohol abuse during pregnancy. N. Engl. J. Med. 297, 528–530. doi: 10.1056/NEJM197709082971003
Page, B. D., Diede, S. J., Tenlen, J. R., and Ferguson, E. L. (2007). EEL-1, a Hect E3 ubiquitin ligase, controls asymmetry and persistence of the SKN-1 transcription factor in the early C. elegans embryo. Development 134, 2303–2314. doi: 10.1242/dev.02855
Pakkenberg, B., and Gundersen, H. J. (1997). Neocortical neuron number in humans: effect of sex and age. J. Comp. Neurol. 384, 312–320. doi: 10.1002/(SICI)1096-9861(19970728)384:2<312::AID-CNE10>3.0.CO;2-K
Park, H., Yang, J., Kim, R., Li, Y., Lee, Y., Lee, C., et al. (2015). Mice lacking the PSD-95-interacting E3 ligase, Dorfin/Rnf19a, display reduced adult neurogenesis, enhanced long-term potentiation, and impaired contextual fear conditioning. Sci. Rep. 5:16410. doi: 10.1038/srep16410
Perera, F., and Herbstman, J. (2011). Prenatal environmental exposures, epigenetics, and disease. Reprod. Toxicol. 31, 363–373. doi: 10.1016/j.reprotox.2010.12.055
Perry, W. L., Hustad, C. M., Swing, D. A., O’Sullivan, T. N., Jenkins, N. A., and Copeland, N. G. (1998). The itchy locus encodes a novel ubiquitin protein ligase that is disrupted in a18H mice. Nat. Genet. 18, 143–146. doi: 10.1038/ng0298-143
Peter, S., Bultinck, J., Myant, K., Jaenicke, L. A., Walz, S., Muller, J., et al. (2014). Tumor cell-specific inhibition of MYC function using small molecule inhibitors of the HUWE1 ubiquitin ligase. EMBO Mol. Med. 6, 1525–1541. doi: 10.15252/emmm.201403927
Pettegrew, J., Klunk, W., Panchalingam, K., McClure, R., and Stanley, J. (2000). Molecular insights into neurodevelopmental and neurodegenerative diseases. Brain Res. Bull. 53, 455–469. doi: 10.1016/S0361-9230(00)00376-2
Pintard, L., Kurz, T., Glaser, S., Willis, J. H., Peter, M., and Bowerman, B. (2003). Neddylation and deneddylation of CUL-3 is required to target MEI-1/Katanin for degradation at the meiosis-to-mitosis transition in C. elegans. Curr. Biol. 13, 911–921. doi: 10.1016/S0960-9822(03)00336-1
Polo, S. (2012). Signaling-mediated control of ubiquitin ligases in endocytosis. BMC Biol. 10:25. doi: 10.1186/1741-7007-10-25
Polymeropoulos, M. H., Lavedan, C., Leroy, E., Ide, S. E., Dehejia, A., Dutra, A., et al. (1997). Mutation in the α-synuclein gene identified in families with Parkinson’s disease. Science 276, 2045–2047. doi: 10.1126/science.276.5321.2045
Power, C., and Jefferis, B. J. (2002). Fetal environment and subsequent obesity: a study of maternal smoking. Int. J. Epidemiol. 31, 413–419. doi: 10.1093/intjepid/31.2.413
Preuss, T. M. (2012). Human brain evolution: from gene discovery to phenotype discovery. Proc. Natl. Acad. Sci. U.S.A. 109(Suppl. 1), 10709–10716. doi: 10.1073/pnas.1201894109
Prusiner, S. B., and DeArmond, S. J. (1994). Prion diseases and neurodegeneration. Annu. Rev. Neurosci. 17, 311–339. doi: 10.1146/annurev.ne.17.030194.001523
Qin, X., Chen, S., Qiu, Z., Zhang, Y., and Qiu, F. (2012). Proteomic analysis of ubiquitination-associated proteins in a cisplatin-resistant human lung adenocarcinoma cell line. Int. J. Mol. Med. 29, 791–800.
Quaderi, N. A., Schweiger, S., Gaudenz, K., Franco, B., Rugarli, E. I., Berger, W., et al. (1997). Opitz G/BBB syndrome, a defect of midline development, is due to mutations in a new RING finger gene on Xp22. Nat. Genet. 17, 285–291. doi: 10.1038/ng1197-285
Raab, S., Drechsel, G., Zarepour, M., Hartung, W., Koshiba, T., Bittner, F., et al. (2009). Identification of a novel E3 ubiquitin ligase that is required for suppression of premature senescence in Arabidopsis. Plant J. 59, 39–51. doi: 10.1111/j.1365-313X.2009.03846.x
Raff, R. A. (2012). The Shape of Life: Genes, Development, and the Evolution of Animal Form. Chicago, IL: University of Chicago Press.
Rajalingam, K., and Dikic, I. (2016). SnapShot: expanding the ubiquitin code. Cell 164, 1074–1074.e1. doi: 10.1016/j.cell.2016.02.019
Ramalingam, A., Zhou, X. G., Fiedler, S. D., Brawner, S. J., Joyce, J. M., Liu, H. Y., et al. (2011). 16p13.11 duplication is a risk factor for a wide spectrum of neuropsychiatric disorders. J. Hum. Genet. 56, 541–544. doi: 10.1038/jhg.2011.42
Reed, T. T. (2011). Lipid peroxidation and neurodegenerative disease. Free Radic. Biol. Med. 51, 1302–1319. doi: 10.1016/j.freeradbiomed.2011.06.027
Rett, A. (1966). On a unusual brain atrophy syndrome in hyperammonemia in childhood. Wien. Med. Wochenschr. 116, 723–726.
Reyes-Turcu, F. E., Ventii, K. H., and Wilkinson, K. D. (2009). Regulation and cellular roles of ubiquitin-specific deubiquitinating enzymes. Annu. Rev. Biochem. 78, 363–397. doi: 10.1146/annurev.biochem.78.082307.091526
Ritz, B. R., Manthripragada, A. D., Costello, S., Lincoln, S. J., Farrer, M. J., Cockburn, M., et al. (2009). Dopamine transporter genetic variants and pesticides in Parkinson’s disease. Environ. Health Perspect. 117, 964–969. doi: 10.1289/ehp.0800277
Ross, C. A., and Poirier, M. A. (2004). Protein aggregation and neurodegenerative disease. Nat. Med. 10(Suppl.), S10–S17. doi: 10.1038/nm1066
Rotblat, B., Southwell, A. L., Ehrnhoefer, D. E., Skotte, N. H., Metzler, M., Franciosi, S., et al. (2014). HACE1 reduces oxidative stress and mutant Huntingtin toxicity by promoting the NRF2 response. Proc. Natl. Acad. Sci. U.S.A. 111, 3032–3037. doi: 10.1073/pnas.1314421111
Rotin, D., and Kumar, S. (2009). Physiological functions of the HECT family of ubiquitin ligases. Nat. Rev. Mol. Cell Biol. 10, 398–409. doi: 10.1038/nrm2690
Sahin, M., and Sur, M. (2015). Genes, circuits, and precision therapies for autism and related neurodevelopmental disorders. Science 350:aab3897. doi: 10.1126/science.aab3897
Saiga, T., Fukuda, T., Matsumoto, M., Tada, H., Okano, H. J., Okano, H., et al. (2009). Fbxo45 forms a novel ubiquitin ligase complex and is required for neuronal development. Mol. Cell. Biol. 29, 3529–3543. doi: 10.1128/MCB.00364-09
Salah, Z., Melino, G., and Aqeilan, R. I. (2011). Negative regulation of the Hippo pathway by E3 ubiquitin ligase ITCH is sufficient to promote tumorigenicity. Cancer Res. 71, 2010–2020. doi: 10.1158/0008-5472.CAN-10-3516
Saunders, A. M., Strittmatter, W. J., Schmechel, D., George-Hyslop, P. S., Pericak-Vance, M. A., Joo, S., et al. (1993). Association of apolipoprotein E allele €4 with late-onset familial and sporadic Alzheimer’s disease. Neurology 43, 1467–1467. doi: 10.1212/WNL.43.8.1467
Scheuner, D., Eckman, C., Jensen, M., Song, X., Citron, M., Suzuki, N., et al. (1996). Secreted amyloid β–protein similar to that in the senile plaques of Alzheimer’s disease is increased in vivo by the presenilin 1 and 2 and APP mutations linked to familial Alzheimer’s disease. Nat. Med. 2, 864–870. doi: 10.1038/nm0896-864
Schuettengruber, B., Chourrout, D., Vervoort, M., Leblanc, B., and Cavalli, G. (2007). Genome regulation by polycomb and trithorax proteins. Cell 128, 735–745. doi: 10.1016/j.cell.2007.02.009
Schwartz, A. L., and Ciechanover, A. (2009). Targeting proteins for destruction by the ubiquitin system: implications for human pathobiology. Annu. Rev. Pharmacol. Toxicol. 49, 73–96. doi: 10.1146/annurev.pharmtox.051208.165340
Schwechheimer, C., and Schwager, K. (2004). Regulated proteolysis and plant development. Plant Cell Rep. 23, 353–364. doi: 10.1007/s00299-004-0858-z
Secchiero, P., Bosco, R., Celeghini, C., and Zauli, G. (2011). Recent advances in the therapeutic perspectives of Nutlin-3. Curr. Pharm. Des. 17, 569–577. doi: 10.2174/138161211795222586
Segref, A., and Hoppe, T. (2008). Think locally: control of ubiquitin-dependent protein degradation in neurons. EMBO Rep. 10, 44–50. doi: 10.1038/embor.2008.229
Seigal, B. A., Connors, W. H., Fraley, A., Borzilleri, R. M., Carter, P. H., Emanuel, S. L., et al. (2015). The discovery of macrocyclic XIAP antagonists from a DNA-programmed chemistry library, and their optimization to give lead compounds with in vivo antitumor activity. J. Med. Chem. 58, 2855–2861. doi: 10.1021/jm501892g
Selderslaghs, I. W., Van Rompay, A. R., De Coen, W., and Witters, H. E. (2009). Development of a screening assay to identify teratogenic and embryotoxic chemicals using the zebrafish embryo. Reprod. Toxicol. 28, 308–320. doi: 10.1016/j.reprotox.2009.05.004
Shalizi, A., Bilimoria, P. M., Stegmuller, J., Gaudilliere, B., Yang, Y., Shuai, K., et al. (2007). PIASx is a MEF2 SUMO E3 ligase that promotes postsynaptic dendritic morphogenesis. J. Neurosci. 27, 10037–10046. doi: 10.1523/JNEUROSCI.0361-07.2007
Silva-Santos, S., van Woerden, G. M., Bruinsma, C. F., Mientjes, E., Jolfaei, M. A., Distel, B., et al. (2015). Ube3a reinstatement identifies distinct developmental windows in a murine Angelman syndrome model. J. Clin. Invest. 125, 2069–2076. doi: 10.1172/JCI80554
Skaar, J. R., Pagan, J. K., and Pagano, M. (2014). SCF ubiquitin ligase-targeted therapies. Nat. Rev. Drug Discov. 13, 889–903. doi: 10.1038/nrd4432
Smith, A. H., Marshall, G., Yuan, Y., Ferreccio, C., Liaw, J., von Ehrenstein, O., et al. (2006). Increased mortality from lung cancer and bronchiectasis in young adults after exposure to arsenic in utero and in early childhood. Environ. Health Perspect. 114, 1293–1296. doi: 10.1289/ehp.8832
Smrt, R. D., Szulwach, K. E., Pfeiffer, R. L., Li, X., Guo, W., Pathania, M., et al. (2010). MicroRNA miR-137 regulates neuronal maturation by targeting ubiquitin ligase mind bomb-1. Stem Cells 28, 1060–1070. doi: 10.1002/stem.431
Stegmuller, J., and Bonni, A. (2010). Destroy to create: E3 ubiquitin ligases in neurogenesis. F1000 Biol. Rep. 2:38 doi: 10.3410/B2-38
Stick, S. M., Burton, P. R., Gurrin, L., Sly, P. D., and LeSouëf, P. N. (1996). Effects of maternal smoking during pregnancy and a family history of asthma on respiratory function in newborn infants. Lancet 348, 1060–1064. doi: 10.1016/S0140-6736(96)04446-7
Stiles, J., and Jernigan, T. L. (2010). The basics of brain development. Neuropsychol. Rev. 20, 327–348. doi: 10.1007/s11065-010-9148-4
Stokes, A. H., Hastings, T. G., and Vrana, K. E. (1999). Cytotoxic and genotoxic potential of dopamine. J. Neurosci. Res. 55, 659–665. doi: 10.1002/(SICI)1097-4547(19990315)55:6<659::AID-JNR1>3.0.CO;2-C
Stone, S. L., and Callis, J. (2007). Ubiquitin ligases mediate growth and development by promoting protein death. Curr. Opin. Plant Biol. 10, 624–632. doi: 10.1016/j.pbi.2007.07.010
Stone, S. L., Williams, L. A., Farmer, L. M., Vierstra, R. D., and Callis, J. (2006). KEEP ON GOING, a RING E3 ligase essential for Arabidopsis growth and development, is involved in abscisic acid signaling. Plant Cell 18, 3415–3428. doi: 10.1105/tpc.106.046532
Sullivan, J. A., Shirasu, K., and Deng, X. W. (2003). The diverse roles of ubiquitin and the 26S proteasome in the life of plants. Nat. Rev. Genet. 4, 948–958. doi: 10.1038/nrg1228
Sun, J., Zhu, G., Liu, Y., Standley, S., Ji, A., Tunuguntla, R., et al. (2015). UBE3A regulates synaptic plasticity and learning and memory by controlling SK2 channel endocytosis. Cell Rep. 12, 449–461. doi: 10.1016/j.celrep.2015.06.023
Sun, K., Johnson, B. S., and Gunn, T. M. (2007). Mitochondrial dysfunction precedes neurodegeneration in mahogunin (Mgrn1) mutant mice. Neurobiol. Aging 28, 1840–1852. doi: 10.1016/j.neurobiolaging.2007.07.012
Sun, Y. (2006). E3 ubiquitin ligases as cancer targets and biomarkers. Neoplasia 8, 645–654. doi: 10.1593/neo.06376
Tahirovic, S., and Bradke, F. (2009). Neuronal polarity. Cold Spring Harb. Perspect. Biol. 1:a001644. doi: 10.1101/cshperspect.a001644
Tai, H. C., and Schuman, E. M. (2008). Ubiquitin, the proteasome and protein degradation in neuronal function and dysfunction. Nat. Rev. Neurosci. 9, 826–838. doi: 10.1038/nrn2499
Takano, T., Xu, C., Funahashi, Y., Namba, T., and Kaibuchi, K. (2015). Neuronal polarization. Development 142, 2088–2093. doi: 10.1242/dev.114454
Tan, M., Li, Y., Yang, R., Xi, N., and Sun, Y. (2011a). Inactivation of SAG E3 ubiquitin ligase blocks embryonic stem cell differentiation and sensitizes leukemia cells to retinoid acid. PLoS ONE 6:e27726. doi: 10.1371/journal.pone.0027726
Tan, M., Zhao, Y., Kim, S. J., Liu, M., Jia, L., Saunders, T. L., et al. (2011b). SAG/RBX2/ROC2 E3 ubiquitin ligase is essential for vascular and neural development by targeting NF1 for degradation. Dev. Cell 21, 1062–1076. doi: 10.1016/j.devcel.2011.09.014
Tan, X., Calderon-Villalobos, L. I., Sharon, M., Zheng, C., Robinson, C. V., Estelle, M., et al. (2007). Mechanism of auxin perception by the TIR1 ubiquitin ligase. Nature 446, 640–645. doi: 10.1038/nature05731
Tarui, T., Takahashi, T., Nowakowski, R. S., Hayes, N. L., Bhide, P. G., and Caviness, V. S. (2005). Overexpression of p27 Kip 1, probability of cell cycle exit, and laminar destination of neocortical neurons. Cereb. Cortex 15, 1343–1355. doi: 10.1093/cercor/bhi017
Tau, G. Z., and Peterson, B. S. (2010). Normal development of brain circuits. Neuropsychopharmacology 35, 147–168. doi: 10.1038/npp.2009.115
Torrino, S., Visvikis, O., Doye, A., Boyer, L., Stefani, C., Munro, P., et al. (2011). The E3 ubiquitin-ligase HACE1 catalyzes the ubiquitylation of active Rac1. Dev. Cell 21, 959–965. doi: 10.1016/j.devcel.2011.08.015
Townley-Tilson, W. H., Wu, Y., Ferguson, J. E. III, and Patterson, C. (2014). The ubiquitin ligase ASB4 promotes trophoblast differentiation through the degradation of ID2. PLoS ONE 9:e89451. doi: 10.1371/journal.pone.0089451
Tsukamoto, S. (2016). Search for inhibitors of the ubiquitin-proteasome system from natural sources for cancer therapy. Chem. Pharm. Bull. 64, 112–118. doi: 10.1248/cpb.c15-00768
Tursun, B., Schluter, A., Peters, M. A., Viehweger, B., Ostendorff, H. P., Soosairajah, J., et al. (2005). The ubiquitin ligase Rnf6 regulates local LIM kinase 1 levels in axonal growth cones. Genes Dev. 19, 2307–2319. doi: 10.1101/gad.1340605
Tyedmers, J., Mogk, A., and Bukau, B. (2010). Cellular strategies for controlling protein aggregation. Nat. Rev. Mol. Cell Biol. 11, 777–788. doi: 10.1038/nrm2993
Upadhyay, A., Amanullah, A., Chhangani, D., Mishra, R., and Mishra, A. (2015a). Selective multifaceted E3 ubiquitin ligases barricade extreme defense: potential therapeutic targets for neurodegeneration and ageing. Ageing Res. Rev. 24, 138–159. doi: 10.1016/j.arr.2015.07.009
Upadhyay, A., Amanullah, A., Chhangani, D., Mishra, R., Prasad, A., and Mishra, A. (2015b). Mahogunin ring finger-1 (MGRN1), a multifaceted ubiquitin ligase: recent unraveling of neurobiological mechanisms. Mol. Neurobiol. 53, 4484–4496. doi: 10.1007/s12035-015-9379-8
Upadhyay, A., Amanullah, A., Mishra, R., Kumar, A., and Mishra, A. (2017). Lanosterol suppresses the aggregation and cytotoxicity of misfolded proteins linked with neurodegenerative diseases. Mol. Neurobiol. doi: 10.1007/s12035-016-0377-2 [Epub ahead of print].
Vadhvani, M., Schwedhelm-Domeyer, N., Mukherjee, C., and Stegmüller, J. (2013). The centrosomal e3 ubiquitin ligase FBXO31-SCF regulates neuronal morphogenesis and migration. PLoS ONE 8:e57530. doi: 10.1371/journal.pone.0057530
Valente, E. M., Abou-Sleiman, P. M., Caputo, V., Muqit, M. M., Harvey, K., Gispert, S., et al. (2004). Hereditary early-onset Parkinson’s disease caused by mutations in PINK1. Science 304, 1158–1160. doi: 10.1126/science.1096284
van Beuningen, S. F., Will, L., Harterink, M., Chazeau, A., van Battum, E. Y., Frias, C. P., et al. (2015). TRIM46 controls neuronal polarity and axon specification by driving the formation of parallel microtubule arrays. Neuron 88, 1208–1226. doi: 10.1016/j.neuron.2015.11.012
van Loo, K. M., and Martens, G. J. (2007). Genetic and environmental factors in complex neurodevelopmental disorders. Curr. Genomics 8, 429–444. doi: 10.2174/138920207783591717
Vassilev, L. T., Vu, B. T., Graves, B., Carvajal, D., Podlaski, F., Filipovic, Z., et al. (2004). In vivo activation of the p53 pathway by small-molecule antagonists of MDM2. Science 303, 844–848. doi: 10.1126/science.1092472
Vierstra, R. D. (1996). Proteolysis in plants: mechanisms and functions. Plant Mol. Biol. 32, 275–302. doi: 10.1007/BF00039386
Vincent, V., Tilders, F., and Van Dam, A. (1998). Production, regulation and role of nitric oxide in glial cells. Mediators Inflamm. 7, 239–255. doi: 10.1080/09629359890929
Vucic, D., Dixit, V. M., and Wertz, I. E. (2011). Ubiquitylation in apoptosis: a post-translational modification at the edge of life and death. Nat. Rev. Mol. Cell Biol. 12, 439–452. doi: 10.1038/nrm3143
Wakatsuki, S., Furuno, A., Ohshima, M., and Araki, T. (2015). Oxidative stress-dependent phosphorylation activates ZNRF1 to induce neuronal/axonal degeneration. J. Cell Biol. 211, 881–896. doi: 10.1083/jcb.201506102
Wallace, M. R., Marchuk, D. A., Andersen, L. B., Letcher, R., Odeh, H. M., Saulino, A. M., et al. (1990). Type 1 neurofibromatosis gene: identification of a large transcript disrupted in three NF1 patients. Science 249, 181–187. doi: 10.1126/science.2134734
Walter, S., and Buchner, J. (2002). Molecular chaperones—cellular machines for protein folding. Angew. Chem. Int. Ed. Engl. 41, 1098–1113. doi: 10.1002/1521-3773(20020402)41:7<1098::AID-ANIE1098>3.0.CO;2-9
Wasilewska, A., Vlad, F., Sirichandra, C., Redko, Y., Jammes, F., Valon, C., et al. (2008). An update on abscisic acid signaling in plants and more. Mol. Plant 1, 198–217. doi: 10.1093/mp/ssm022
Waterland, R. A., Lin, J. R., Smith, C. A., and Jirtle, R. L. (2006). Post-weaning diet affects genomic imprinting at the insulin-like growth factor 2 (Igf2) locus. Hum. Mol. Genet. 15, 705–716. doi: 10.1093/hmg/ddi484
Weake, V. M., and Workman, J. L. (2008). Histone ubiquitination: triggering gene activity. Mol. Cell. 29, 653–663. doi: 10.1016/j.molcel.2008.02.014
Weaver, I. C., Cervoni, N., Champagne, F. A., D’Alessio, A. C., Sharma, S., Seckl, J. R., et al. (2004). Epigenetic programming by maternal behavior. Nat. Neurosci. 7, 847–854. doi: 10.1038/nn1276
Wells, D., Bermudez, M., Steuerwald, N., Thornhill, A., Walker, D., Malter, H., et al. (2005). Expression of genes regulating chromosome segregation, the cell cycle and apoptosis during human preimplantation development. Hum. Reprod. 20, 1339–1348. doi: 10.1093/humrep/deh778
Westbrook, T. F., Hu, G., Ang, X. L., Mulligan, P., Pavlova, N. N., Liang, A., et al. (2008). SCFbeta-TRCP controls oncogenic transformation and neural differentiation through REST degradation. Nature 452, 370–374. doi: 10.1038/nature06780
White, A. R., Reyes, R., Mercer, J. F., Camakaris, J., Zheng, H., Bush, A. I., et al. (1999). Copper levels are increased in the cerebral cortex and liver of APP and APLP2 knockout mice. Brain Res. 842, 439–444. doi: 10.1016/S0006-8993(99)01861-2
Wilhelm, M., Schlegl, J., Hahne, H., Gholami, A. M., Lieberenz, M., Savitski, M. M., et al. (2014). Mass-spectrometry-based draft of the human proteome. Nature 509, 582–587. doi: 10.1038/nature13319
Woelk, T., Sigismund, S., Penengo, L., and Polo, S. (2007). The ubiquitination code: a signalling problem. Cell Div. 2:11. doi: 10.1186/1747-1028-2-11
Won, M., Ro, H., and Dawid, I. B. (2015). Lnx2 ubiquitin ligase is essential for exocrine cell differentiation in the early zebrafish pancreas. Proc. Natl. Acad. Sci. U.S.A. 112, 12426–12431. doi: 10.1073/pnas.1517033112
Wong, J. J., Li, S., Lim, E. K., Wang, Y., Wang, C., Zhang, H., et al. (2013). A Cullin1-based SCF E3 ubiquitin ligase targets the InR/PI3K/TOR pathway to regulate neuronal pruning. PLoS Biol. 11:e1001657. doi: 10.1371/journal.pbio.1001657
Wong, P. C., Pardo, C. A., Borchelt, D. R., Lee, M. K., Copeland, N. G., Jenkins, N. A., et al. (1995). An adverse property of a familial ALS-linked SOD1 mutation causes motor neuron disease characterized by vacuolar degeneration of mitochondria. Neuron 14, 1105–1116. doi: 10.1016/0896-6273(95)90259-7
Xie, Q., Guo, H. S., Dallman, G., Fang, S., Weissman, A. M., and Chua, N. H. (2002). SINAT5 promotes ubiquitin-related degradation of NAC1 to attenuate auxin signals. Nature 419, 167–170. doi: 10.1038/nature00998
Yamada, T., Yang, Y., and Bonni, A. (2013). Spatial organization of ubiquitin ligase pathways orchestrates neuronal connectivity. Trends Neurosci. 36, 218–226. doi: 10.1016/j.tins.2012.12.004
Yamagishi, Y., Shoji, I., Miyagawa, S., Kawakami, T., Katoh, T., Goto, Y., et al. (2011). Natural product-like macrocyclic N-methyl-peptide inhibitors against a ubiquitin ligase uncovered from a ribosome-expressed de novo library. Chem. Biol. 18, 1562–1570. doi: 10.1016/j.chembiol.2011.09.013
Yan, D., Wu, Z., Chisholm, A. D., and Jin, Y. (2009). The DLK-1 kinase promotes mRNA stability and local translation in C. elegans synapses and axon regeneration. Cell 138, 1005–1018. doi: 10.1016/j.cell.2009.06.023
Yang, S. W., Oh, K. H., Park, E., Chang, H. M., Park, J. M., Seong, M. W., et al. (2013). USP47 and C terminus of Hsp70-interacting protein (CHIP) antagonistically regulate katanin-p60-mediated axonal growth. J. Neurosci. 33, 12728–12738. doi: 10.1523/JNEUROSCI.0698-13.2013
Yang, Y., Kim, A. H., and Bonni, A. (2010). The dynamic ubiquitin ligase duo: Cdh1-APC and Cdc20-APC regulate neuronal morphogenesis and connectivity. Curr. Opin. Neurobiol. 20, 92–99. doi: 10.1016/j.conb.2009.12.004
Yang, Y., Kim, A. H., Yamada, T., Wu, B., Bilimoria, P. M., Ikeuchi, Y., et al. (2009). A Cdc20-APC ubiquitin signaling pathway regulates presynaptic differentiation. Science 326, 575–578. doi: 10.1126/science.1177087
Yegambaram, M., Manivannan, B., Beach, T. G., and Halden, R. U. (2015). Role of environmental contaminants in the etiology of Alzheimer’s disease: a review. Curr. Alzheimer Res. 12, 116–146. doi: 10.2174/1567205012666150204121719
Yerbury, J. J., Ooi, L., Dillin, A., Saunders, D. N., Hatters, D. M., Beart, P. M., et al. (2016). Walking the tightrope: proteostasis and neurodegenerative disease. J. Neurochem. 137, 489–505. doi: 10.1111/jnc.13575
Yi, J. J., and Ehlers, M. D. (2007). Emerging roles for ubiquitin and protein degradation in neuronal function. Pharmacol. Rev. 59, 14–39. doi: 10.1124/pr.59.1.4
Yokomizo, T., and Dzierzak, E. (2008). Fine-tuning of hematopoietic stem cell homeostasis: novel role for ubiquitin ligase. Genes Dev. 22, 960–963. doi: 10.1101/gad.1669908
Yoshida, K., Watanabe, M., and Hatakeyama, S. (2009). ZNRF1 interacts with tubulin and regulates cell morphogenesis. Biochem. Biophys. Res. Commun. 389, 506–511. doi: 10.1016/j.bbrc.2009.09.011
Yu, X., Robinson, J. F., Sidhu, J. S., Hong, S., and Faustman, E. M. (2010). A system-based comparison of gene expression reveals alterations in oxidative stress, disruption of ubiquitin-proteasome system and altered cell cycle regulation after exposure to cadmium and methylmercury in mouse embryonic fibroblast. Toxicol. Sci. 114, 356–377. doi: 10.1093/toxsci/kfq003
Zenker, M., Mayerle, J., Lerch, M. M., Tagariello, A., Zerres, K., Durie, P. R., et al. (2005). Deficiency of UBR1, a ubiquitin ligase of the N-end rule pathway, causes pancreatic dysfunction, malformations and mental retardation (Johanson-Blizzard syndrome). Nat. Genet. 37, 1345–1350. doi: 10.1038/ng1681
Zhang, C. C., Yan, Z., Zong, Q., Fang, D. D., Painter, C., Zhang, Q., et al. (2013). Synergistic effect of the gamma-secretase inhibitor PF-03084014 and docetaxel in breast cancer models. Stem Cells Transl. Med. 2, 233–242. doi: 10.5966/sctm.2012-0096
Zhang, H., and Xing, L. (2013). Ubiquitin e3 ligase itch negatively regulates osteoblast differentiation from mesenchymal progenitor cells. Stem Cells 31, 1574–1583. doi: 10.1002/stem.1395
Zhang, W., Wu, K. P., Sartori, M. A., Kamadurai, H. B., Ordureau, A., Jiang, C., et al. (2016). System-wide modulation of HECT E3 ligases with selective ubiquitin variant probes. Mol. Cell. 62, 121–136. doi: 10.1016/j.molcel.2016.02.005
Zhang, X., Garreton, V., and Chua, N. H. (2005). The AIP2 E3 ligase acts as a novel negative regulator of ABA signaling by promoting ABI3 degradation. Genes Dev. 19, 1532–1543. doi: 10.1101/gad.1318705
Zhao, X., D’ Arca, D., Lim, W. K., Brahmachary, M., Carro, M. S., Ludwig, T., et al. (2009). The N-Myc-DLL3 cascade is suppressed by the ubiquitin ligase Huwe1 to inhibit proliferation and promote neurogenesis in the developing brain. Dev. Cell 17, 210–221. doi: 10.1016/j.devcel.2009.07.009
Zimmerberg, J., and Kozlov, M. M. (2006). How proteins produce cellular membrane curvature. Nat. Rev. Mol. Cell Biol. 7, 9–19. doi: 10.1038/nrm1784
Zoghbi, H. Y., and Bear, M. F. (2012). Synaptic dysfunction in neurodevelopmental disorders associated with autism and intellectual disabilities. Cold Spring Harb. Perspect. Biol. 4:a009886. doi: 10.1101/cshperspect.a009886
Zou, Y., Liu, Q., Chen, B., Zhang, X., Guo, C., Zhou, H., et al. (2007). Mutation in CUL4B, which encodes a member of cullin-RING ubiquitin ligase complex, causes X-linked mental retardation. Am. J. Hum. Genet. 80, 561–566. doi: 10.1086/512489
Keywords: E3 ubiquitin ligases, neurodevelopmental disorders, neurodegenerative diseases, neurons, aging
Citation: Upadhyay A, Joshi V, Amanullah A, Mishra R, Arora N, Prasad A and Mishra A (2017) E3 Ubiquitin Ligases Neurobiological Mechanisms: Development to Degeneration. Front. Mol. Neurosci. 10:151. doi: 10.3389/fnmol.2017.00151
Received: 20 February 2017; Accepted: 04 May 2017;
Published: 19 May 2017.
Edited by:
Hans-Georg Breitinger, German University in Cairo, EgyptReviewed by:
Michael Telias, University of California, Berkeley, United StatesPrasad Kasturi, Max Planck Institute of Biochemistry, Germany
Copyright © 2017 Upadhyay, Joshi, Amanullah, Mishra, Arora, Prasad and Mishra. This is an open-access article distributed under the terms of the Creative Commons Attribution License (CC BY). The use, distribution or reproduction in other forums is permitted, provided the original author(s) or licensor are credited and that the original publication in this journal is cited, in accordance with accepted academic practice. No use, distribution or reproduction is permitted which does not comply with these terms.
*Correspondence: Amit Mishra, YW1pdEBpaXRqLmFjLmlu