Stress Odorant Sensory Response Dysfunction in Drosophila Fragile X Syndrome Mutants
- 1Department of Pediatrics, University of Alberta, Edmonton, AB, Canada
- 2Neuroscience and Mental Health Institute, University of Alberta, Edmonton, AB, Canada
- 3Department of Medical Genetics, University of Alberta, Edmonton, AB, Canada
Sensory processing dysfunction (SPD) is present in most patients with intellectual disability (ID) and autism spectrum disorder (ASD). Silencing expression of the Fragile X mental retardation 1 (FMR1) gene leads to Fragile X syndrome (FXS), the most common single gene cause of ID and ASD. Drosophila have a highly conserved FMR1 ortholog, dfmr1. dfmr1 mutants display cognitive and social defects reminiscent of symptoms seen in individuals with FXS. We utilized a robust behavioral assay for sensory processing of the Drosophila stress odorant (dSO) to gain a better understanding of the molecular basis of SPD in FXS. Here, we show that dfmr1 mutant flies present significant defects in dSO response. We found that dfmr1 expression in mushroom bodies is required for dSO processing. We also show that cyclic adenosine monophosphate (cAMP) signaling via PKA is activated after exposure to dSO and that several drugs regulating both cAMP and cyclic guanosine monophosphate (cGMP) levels significantly improved defects in dSO processing in dfmr1 mutant flies.
Introduction
Sensory processing dysfunction (SPD) is a key symptom seen in 90% of individuals with intellectual disability (ID) and autism spectrum disorder (ASD) (Marco et al., 2011; Chang et al., 2014) where the response to a given stimulus is different from the typically developing population. The most common clinical features of SPD are under-responsiveness, sensory seeking, auditory filtering, and tactile sensitivity (Tomchek and Dunn, 2007). This reflects that multiple senses are affected, including audition, touch, vision, and oral sensation (Kern et al., 2006). For instance, some individuals with ASD will perceive sound much louder than typically developing individuals and this will affect their behavior. Indeed, they will either block their ears or become increasingly anxious. SPD affects patients with mild to severe ID equally (Engel-Yeger et al., 2011). Sensory processing deficits have also been linked to stereotypical movements and anxiety (Joosten and Bundy, 2010). SPD predicts communication competence and maladaptive behaviors (Lane et al., 2010), which are the drivers of socio-economic impact (Bailey et al., 2012). Importantly, SPD is present in both children and adults (Crane et al., 2009). While brain magnetic resonance imaging (MRI) studies have provided some insights (Owen et al., 2013), the molecular basis and treatment of SPD remain poorly understood.
Fragile X syndrome (FXS) is the most common single gene cause of ID and ASD (Androschuk et al., 2015). FXS is caused by a trinucleotide CGG repeat expansion that leads to the methylation and transcriptional silencing of the Fragile X mental retardation 1 (FMR1) gene. This results in the loss of Fragile X mental retardation protein (FMRP), an mRNA-binding protein that functions in neuronal mRNA metabolism, namely in the translation of neuronal mRNAs involved in synaptic structure and function. Individuals with FXS frequently present with SPD (Goldson, 2001), which has a major impact on their ability to function (Baranek et al., 2002). We reasoned that response to sensory stimulation may serve as endophenotype of the processing of information.
Drosophila have a conserved FMR1 ortholog, dfmr1. dfmr1 mutants present with the circadian, cognitive, and social defects also observed in individuals with FXS. Little is known about the response to sensory signal in dfmr1 mutant flies. Normal shock and olfactory stimuli used for olfactory memory training have not provided a model to study sensory processing. Suh et al. (2004) discovered that Drosophila avoided systematically an environment in which other flies had previously been submitted to mechanical stress. Indeed, the Drosophila stress odorant (dSO) is a signal emitted when flies are subjected to electrical or mechanical stressors, and elicits an innate and robust avoidance behavioral response in wild-type (WT) Drosophila (Suh et al., 2004). Here, we show that Drosophila dfmr1 mutant flies present significant defect in responding to dSO.
Materials and Methods
Drosophila melanogaster Stocks and Crosses
Fly stocks were maintained at 22°C on standard cornmeal-yeast media from Cold Spring Harbor Laboratory. WT stocks were backcrossed to w1118isoCJ1 for 6 generations. dfmr1B55 flies were obtained from Dr. Kendal Broadie (Vanderbilt University). dfmr13 flies and dfmr13 flies containing a WT rescue transgene (dfmr13WTR) were obtained from Dr. Tom Jongens (University of Pennsylvania). Elav-Gal4, OK107-Gal4, C747-Gal4, MB247-Gal4, and Feb170-Gal4 flies were obtained from Dr. Tim Tully. To determine the spatial requirement of FMRP in mediating dSO avoidance, we used RNA interference (RNAi) against FMRP in order to knockdown/reduce expression of FMRP. Using the Gal4-UAS system (Brand and Perrimon, 1993), we generated crosses by mating Elav(Embryonic lethal vision)-Gal4, OK107-Gal4, Feb170-Gal4, MB247-Gal4, and 747-Gal4 virgin females to UAS-dfmr1RNAi1-7 males generated previously in our laboratory (Bolduc et al., 2008). To assess the spatio-temporal requirement of dfmr1, we used Gal80ts; Elav-Gal4 (from Dr. Tom Jongens) to drive the expression UAS-dfmr1RNAi1-7. WT and transgenic flies were tested in parallel. WT and transgenic flies were raised at 18°C (restricting the expression of ELAV-Gal4) and then transferred for 3 days at 30°C allowing its expression, or kept at 18°C, to restrict the expression of ELAV-Gal4, as before for memory experiments in our laboratory (Bolduc et al., 2008).
Behavioral Paradigm
The T maze avoidance assay was conducted, as previously described by Suh et al. (2004), with modifications (Androschuk, 2016). All testing was performed in an environment controlled room which was maintained at 25°C and 70% humidity. To produce dSO a group of 50 flies (mixed sex, termed ‘emitter’ flies) were vortexed (Fisher Vortex Mixer) for 1 min (alternating between 3 s of vortexing followed by 5 s of rest for the entire duration) in a 10 mL Falcon tube sealed with Parafilm (Fisher Scientific 149598) at maximum speed. Emitter flies were then removed from the Falcon tube and the dSO-containing Falcon tube was placed into a T maze. A new dSO-free Falcon tube was placed opposite the dSO-containing tube. Subsequently, 50 naïve flies (termed ‘responder’) were transferred into a new Falcon tube and loaded into the elevator of the T maze. Responder flies were then given 1 min to choose between the dSO-containing and the dSO-free Falcon tubes. Following the 1-min testing period, flies were sequestered and avoidance response was scored. Avoidance was scored as a Performance Index (PI), calculated as follows:
Statistical Analysis
For unplanned comparisons between more than 2 groups, we used one-way ANOVA followed by Tukey’s test. For all analysis between 2 groups, we used a two-tailed Student’s t-test. Analysis was performed using GraphPad (PRISM7).
CO2 Avoidance
Gaseous CO2 was used in place of emitter flies in CO2 avoidance testing. A flow-meter set at 0.2 mL/min or 0.5 mL/min was used to administer CO2 into Falcon tubes for 30 s, which were then momentarily sealed using Parafilm prior to being loaded into the T maze. Responder flies were given 1 min to choose between the CO2-containing and the CO2-free Falcon tubes. Flies were then sequestered and avoidance response was scored as a PI, as above.
Drug Administration
Using previously published feeding protocols for Lithium in the classical olfactory conditioning assay (Choi et al., 2015), we performed dose response curves for the avoidance assay. For drugs not previously tested in our laboratory (IBMX, dipyridamole, 8-CPT), we assessed response at 1 day as well as longer treatment if there was no response after 1 day. The treatments were provided only in post-natal set up to reflect the potential clinical application at this time. For all experiments, only responder flies were treated with vehicle or treatment.
IBMX
The 3-isobutyl-1-methylxanthine (IBMX; Sigma I7018) was added to standard food media for drug administration. The 1-day-old flies were placed in food bottles containing 0.05 mg/mL IBMX or the food alone for 4 days and transferred to food vials containing 0.05 mg/mL IMBX or the food alone the day prior to testing (Androschuk, 2016).
8-CPT
The 8-(4-Chlorophenylthio)adenosine 3′,5′-cyclic monophosphate sodium salt (8-CPT; Sigma C3912) was administered to flies on 2.1-cm Whatman filter paper (Fisher WHT1540321). The 1-day-old flies were placed in vials containing 225 μL of 8-CPT with 5% sucrose or 5% sucrose only and treated for 5 days prior to testing. Flies were transferred daily to new vials containing fresh 8-CPT with sucrose or sucrose alone (Androschuk, 2016).
LiCl
Lithium chloride (LiCl; Sigma L9650) was added directly to the standard food media for drug administration. The 1-day-old flies were set up in food bottles containing 10 mM LiCl or the food alone for 4 days and transferred to food vials containing 10 mM LiCl or the food alone the day prior to testing (Androschuk, 2016).
Dipyridamole
The 0.8 mM dipyridamole (Sigma D9766) was added directly to standard food media for drug administration with 0.8% DMSO. The 1-day-old flies were placed for 24 h in food bottles containing either dipyridamole or vehicle.
Immunohistochemistry
After 1 min exposure to dSO, naïve responder flies avoiding the dSO were placed on ice for 2 min and heads of female responder flies were removed and placed in cold PBS for dissection. Unexposed flies were placed in a dSO-free 10 mL Falcon tube sealed with Parafilm for 1 min. Then flies were processed blind in parallel. Flies were then placed on ice for 2 min and heads of female flies were removed and placed in cold PBS for dissection. Fly heads were dissected as before (Bolduc et al., 2008). Protein kinase A (PKA) was identified with 1:1000 α-PKA catalytic subunit (phospho T198) (Abcam ab118531).
Following overnight incubation with the secondary antibody (1:200 Cy3 α-Rabbit Jackson ImmunoResearch 111-165-003) and 1% PBS triton (PBST) with 0.25% NGS, brains were washed three times with 1% PBST and mounted using FocusClear (Cedarlane FC-101). Imaging was completed using a Zeiss LSM 700 Confocal Microscope and images were quantified using ImageJ (Androschuk, 2016). Gain was set the same for both groups.
Pathway Analysis
In silico pathway analyses were performed with Ingenuity Pathway Analysis (IPA, Qiagen) to identify interactions with cAMP and cGMP by genes associated with ASD from the SFARI Gene database (https://www.sfari.org/) and genes implicated in ID from published literature (Gilissen et al., 2014).
Results
dfmr1 Is Required for dSO Response
In order to determine the role of FMRP in the processing and modulation of dSO avoidance behavior in Drosophila, we utilized the two null alleles, dfmr13 and dfmr1B55, known to have olfactory and courtship memory defects, as well as social interaction defect (McBride et al., 2005; Bolduc et al., 2008; Bolduc et al., 2010). We found that dfmr13 and dfmr1B55 flies exhibited a significant decrease in dSO avoidance compared to flies with the appropriate genetic control (dfrm13 with a genomic rescue fragment, FMR13WTR, and WT flies) (Figure 1A). Similarly, transheterozygous FMRB55/FMR13 mutants exhibited a significant decrease in dSO avoidance behavior compared to WT flies (Figure 1B). Next, we tested if FMRP was involved in dSO emission or dSO response. We conducted avoidance trials in which WT flies were utilized as the emitter or responder and tested with the dfmr1 mutant flies. WT flies exhibited normal avoidance in response to dSO emitted by FMRB55 and FMR13 (Figure 1C). FMRB55 and FMR13 flies exhibited decreased avoidance as compared to their genetic controls when WT flies were utilized as emitter flies (Figure 1D). Considering the normal avoidance of WT flies when using dfmr1 flies as emitters, we considered that FMRP is involved in sensory processing and not emission of dSO.
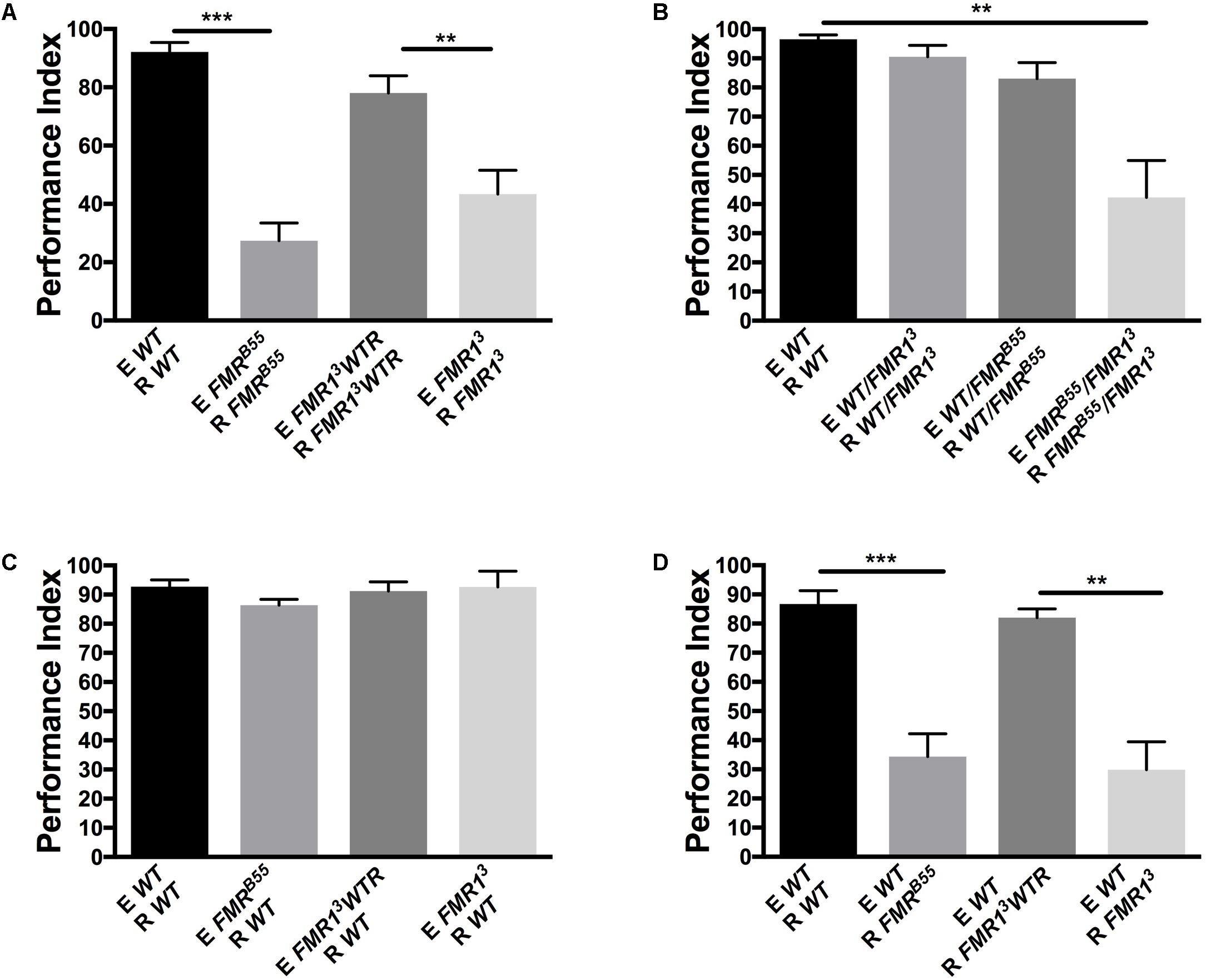
FIGURE 1. Fragile X mental retardation protein (FMRP) is required for avoidance of Drosophila melanogaster stress odorant (dSO). For all figures, the flies emitting the dSO (E) are submitted to the vortexing protocol. The flies tested for their response to tubes exposed to dSO or not are considered the responders (R) of dSO signaling. (A) FMRB55 mutants exhibit a significantly lower avoidance in response to dSO compared to WT flies (Student’s t-test P < 0.0001; N = 8); avoidance is quantified as Performance Index (PI). FMR13 exhibit decreased avoidance compared to FMR13WTR flies, the avoidance of which is rescued genetically through the addition of a genomic dfmr13 fragment (Student’s t-test P = 0.0049; N = 8). dSO avoidance behavior is scored as PI. (B) FMRB55/FMR13 flies exhibit decreased avoidance compared to WT flies (Tukey’s test P = 0.0001; N = 7). Avoidance behavior is genetically rescued in FMRB55/WT (Tukey P = 0.9348; N = 7) and FMR13/WT (ANOVA P = 0.5638; N = 7) flies. FMRB55/FMR13 flies exhibit decrease avoidance behavior compared to FMR13/WT (Tukey’s test P = 0.0004; N = 7) and FMRB55/WT (Tukey’s test P = 0.0028; N = 7) flies. (C) WT flies did not exhibit decreased avoidance behavior to dSO emitted by FMRB55, (Student’s t-test P = 0.0988; N = 5), FMR13 (Student’s t-test P = 0.9897; N = 5), and FMR13WTR flies (Student’s t-test P = 0.7153; N = 5). (D) FMRB55 flies exhibit decreased avoidance behavior to WT dSO (Student’s t-test P < 0.0001; N = 12). FMR13 also flies exhibit diminished avoidance behavior to WT dSO as compared to FMR13WTR flies (Student’s t-test P = 0.0018; N = 12). ∗∗P < 0.01, ∗∗∗P < 0.001.
dfmr1 Is Required in Mushroom Bodies (MB) for dSO Processing
We first used the pan-neuronal driver ELAV-Gal4 and UAS-FMR responder with RNAi to knockdown FMRP in neurons. Pan-neuronal knockdown of FMRP resulted in a significant decrease in dSO avoidance response, which we confirmed causes a dSO processing defect and not emission deficiency from knockdown of FMRP (Figures 2A,B). Next, we asked whether loss of FMRP in two higher-order processing centers, the mushroom bodies (MB) and the central complex, are involved in dSO avoidance. We showed previously that FMRP was required in MB for olfactory memory (Bolduc et al., 2008). Bräcker et al. (2013) showed that MB were required for CO2 avoidance response in the context of food deprivation or food-related odors. Knockdown of FMRP using the MB-specific driver OK107 resulted in a significantly decreased avoidance response compared to WT flies (Figures 2C,D). To confirm the requirement of FMRP in the MB in mediating dSO avoidance behavior, we utilized the MB-specific driver MB247 to knockdown FMRP, which resulted in a significant defect in dSO avoidance (Figures 2E,F). Unlike the significant decrease in dSO avoidance that resulted from using the OK107-Gal4 and MB247-Gal4 driver lines to knockdown FMRP in the MB, use of the C747-Gal4 driver line did not result in a significant decrease in dSO avoidance (results not shown). These differences are likely due to regional specificity and strength of expression of each individual driver within the MB. The OK107-Gal4 and MB247-Gal4 driver lines strongly target expression in α, β, and γ Kenyon cells, while C747-Gal4 expression is weaker in γ Kenyon cells (Aso et al., 2009). Knockdown of FMRP in the central complex using FEB170-Gal4 did not result in any significant changes in dSO avoidance (Supplementary Figures 1A,B). In addition, we did not observe significant defects in the avoidance after post-natal variation in FMRP levels [using Gal80ts; ELAV-gal4 with UAS-dfmr1RNAi1-7 to lower FMRP level as before (Bolduc et al., 2008)], which is different to what was observed in long-term olfactory memory defects in dfmr1 mutants previously and more similar to short-term memory (Figure 2G; Bolduc et al., 2008).
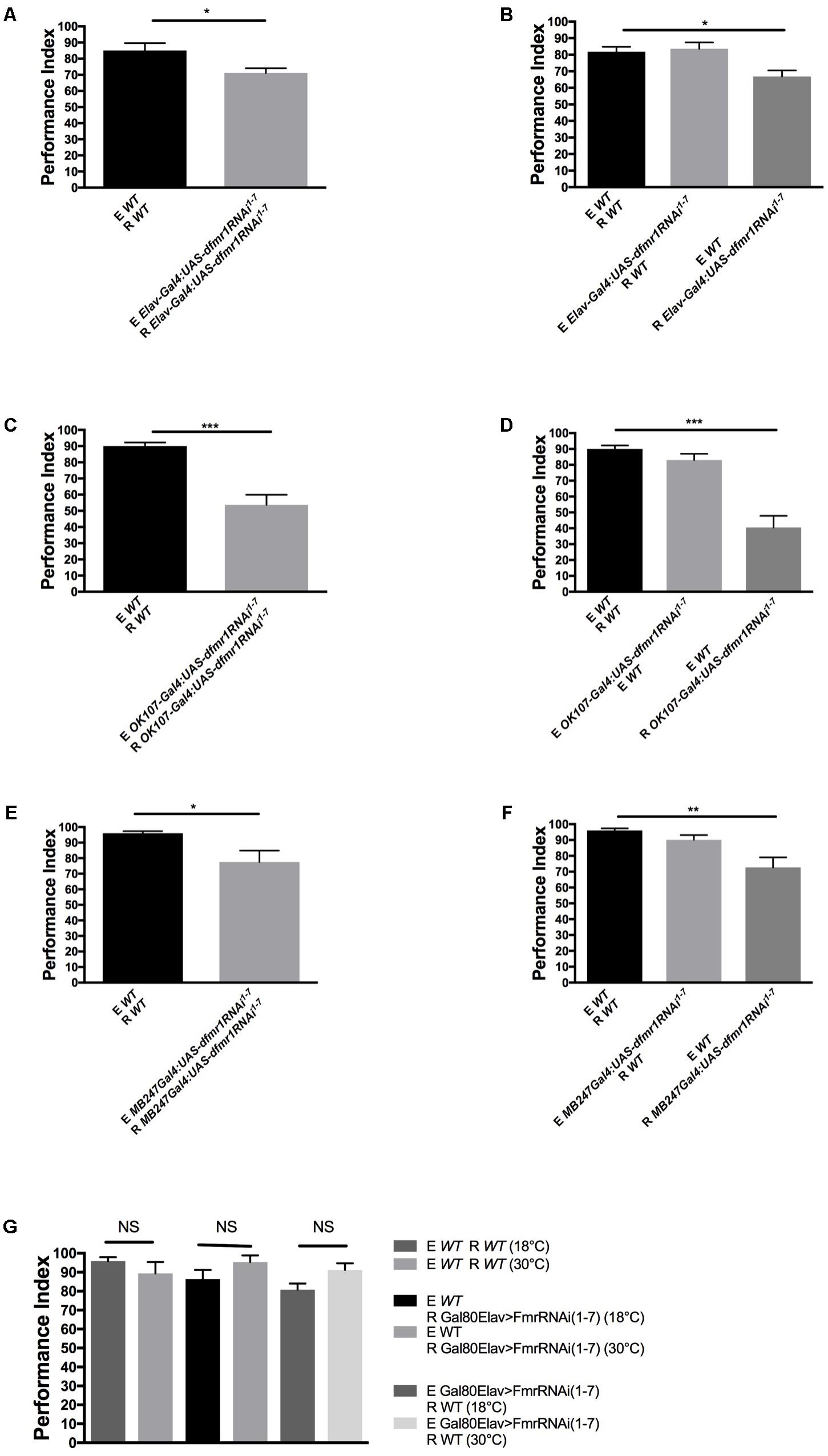
FIGURE 2. FMRP expression in mushroom bodies and glia is required for dSO avoidance. (A) Pan-neuronal knockdown of FMRP by Elav-Gal4:UAS-dfmr1RNAi1-7, results in decreased avoidance to dSO compared to WT flies (Student’s t-test P = 0.0409; N = 20). (B) WT flies did not exhibit any significant decrease in avoidance behavior to dSO emitted by Elav-Gal4:UAS-dfmr1RNAi1-7 flies (Student’s t-test P = 0.7653; N = 10). Elav-Gal4:UAS-dfmr1RNAi1-7 flies exhibit decreased avoidance behavior to WT dSO as compared to WT flies (Student’s t-test P = 0.00285; N = 12). (C) OK107-Gal4:UAS-dfmr1RNAi1-7 flies exhibit significantly decreased avoidance to dSO compared to WT flies (Student’s t-test P < 0.0001; N = 12). (D) OK107-Gal4:UAS-dfmr1RNAi1-7 flies exhibit a significantly decreased avoidance response when tested against dSO emitted by WT flies (Student’s t-test P < 0.0001; N = 8). WT flies exhibited normal avoidance behavior when tested against dSO emitted by OK107 > FmrRNAi(1-7) flies (Student’s t-test P = 0.1240; N = 8). (E) MB247Gal4;UAS-dfmr1RNAi1-7 flies exhibited diminished avoidance behavior as compared to WT flies (Student’s t-test P = 0.0239; N = 10) when tested with same genotype pairs. (F) MB247Gal4;UAS-dfmr1RNAi1-7 flies exhibit a significantly decreased avoidance response when tested against dSO emitted by WT flies (Student’s t-test P = 0.0016; N = 8). WT flies exhibited normal avoidance behavior when tested against dSO emitted by MB247Gal4;UAS-dfmr1RNAi1-7 flies (Student’s t-test P = 0.0707; N = 8). (G) WT (Student’s t-test P = 0.27; N = 5) and Gal80ts;ELAV-Gal4 > UAS-dfmr1RNAi1-7 flies present no significant defect in avoidance performance comparing their performance at restrictive (18°C) versus permissive (30°C) either as responder to dSO (R) (Student’s t-test P = 0.1689; N = 5 PI per group) or as emitter of dSO (E) (Student’s t-test P = 0.059; N = 5 PI per group). NS, not significant. ∗P < 0.05, ∗∗P < 0.01, ∗∗∗P < 0.001.
Targeting cAMP/cGMP Signaling Pharmacologically in Adult Flies Rescues dSO Response in dfmr1 Mutants
Next, we explored if pharmacological intervention could improve dfmr1 mutant avoidance response and help decipher the molecular mechanism related to the dSO defects in dfmr1 mutant flies. We first considered the seminal report from Suh et al. (2004) who showed that CO2 was a key component of the dSO. Lin et al. (2013) further showed that CO2 olfactory information was conveyed by 2 types of projection neurons depending on the concentration of CO2 present in the environment (Lin et al., 2013). We therefore tested response to CO2 for dfmr1 mutants and found that dfmr13 and dfmr1B55 had significant response deficits to CO2 at 0.2 mL/min and 0.5 mL/min (Supplementary Figures 1C,D). As cAMP signaling is required for CO2 sensing (Klengel et al., 2005) and cAMP signaling dysregulation is linked to FXS early on in human (Berry-Kravis et al., 1984) and in Drosophila (Kanellopoulos et al., 2012), we investigated if cAMP regulation could be involved in the defective dSO response in dfmr1 mutants. Activity dependent reactivity of cAMP is abnormal in FXS (Berry-Kravis et al., 1995). Moreover, FMRP binds to adenylyl cyclase (AC) and phosphodiesterase (PDE) mRNAs (Darnell et al., 2011). Importantly, PDE4 inhibitors Rolipram and Lithium, which lead to increased cAMP levels, have been found to rescue memory and long-term depression (LTD) defects in FXS mice and flies (Choi et al., 2015, 2016).
We assessed if dSO exposure was associated with activation of the cAMP pathway using brain immunohistochemistry first. Activation of cAMP leads to concomitant activation of cAMP-dependent protein kinase A (PKA). Using confocal imaging, we examined the relative levels of the phosphorylated catalytic subunit of PKA in WT fly brains in response to dSO exposure by utilizing a free catalytic subunit-specific PKA (phospho T198) antibody. PKA catalytic subunit mRNA and protein have been shown to be expressed throughout the brain with increased signal in the MB Kenyon cells especially in the dorsal aspect (Skoulakis et al., 1993). PKA is activated when cAMP binds to regulatory subunits, resulting in the disassociation of catalytic subunits. The catalytic-PKA phosphorylation levels were significantly elevated overall in WT brains following dSO exposure compared to naïve, unexposed WT flies, suggesting that cAMP signaling participates in modulating dSO avoidance behavior (Figures 3A,B). Interestingly, high expression was noted in cells located dorsally in the brain in the region corresponding to the Kenyon cells of the MB, similar to the previous report (Skoulakis et al., 1993). Nonetheless, further confirmation with a functional PKA activity assay and measurement of constituents of the cAMP pathway or downstream targets (CREB for instance) will be important to conduct in the future to measure treatment efficacy and could be used as biomarkers.
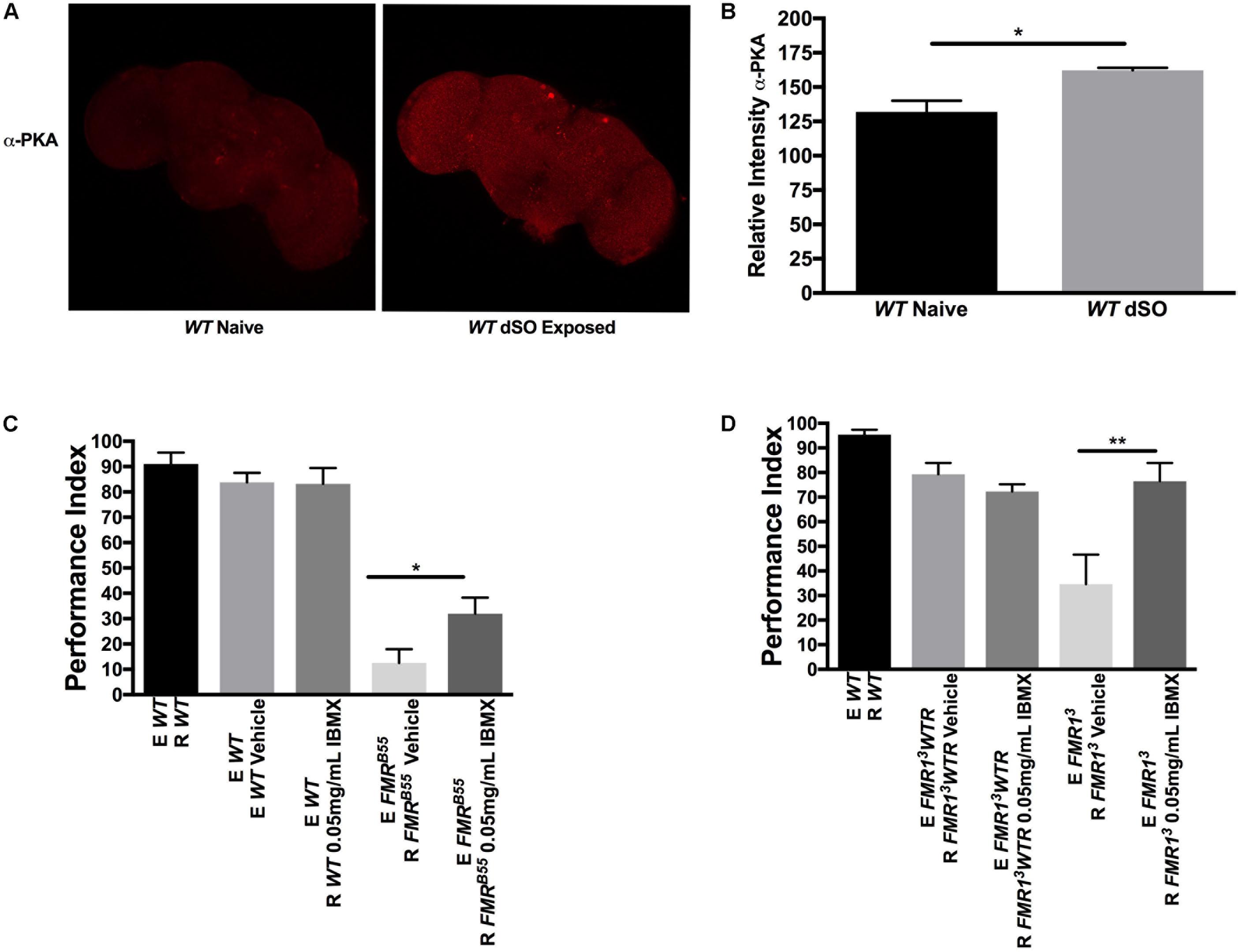
FIGURE 3. Pharmacological intervention targeting cyclic adenosine monophosphate (cAMP) rescues dSO avoidance in Fragile X syndrome flies. (A) Confocal imaging of WT flies catalytic subunit PKA (phospho T198) levels in dSO exposed and unexposed WT fly brains processed in parallel and imaged with same gain. (B) dSO exposure results in an overall significant increase in PKA catalytic subunit (phospho T198) levels in WT brains compared to unexposed control (Student’s t-test P = 0.0226; N = 3). All graphs depict mean ± SEM. (C) 5-day treatment of FMRB55 flies with 0.05 mg/mL IBMX results in significantly increased avoidance compared to FMRB55 on vehicle (Student’s t-test P = 0.0282; N = 14). No significant difference in avoidance behavior observed in WT flies following 5-day treatment with 0.05 mg/mL IBMX as compared to vehicle (Student’s t-test P = 0.9379; N = 14). (D) 5-day treatment of FMR13 flies with 0.05 mg/mL IBMX resulted in a significantly increase in avoidance compared to FMR13 fed vehicle (Student’s t-test P = 0.0068; N = 13). No significant difference in avoidance behavior observed in FMR13WTR flies following 5-day treatment with 0.05 mg/mL IBMX as compared to vehicle (Student’s t-test P = 0.02077; N = 13). ∗P < 0.05, ∗∗P < 0.01.
We wanted to determine whether dSO avoidance behavior could be rescued through pharmacological intervention targeting the cAMP and/or cGMP signaling pathway restricted to the post-natal period as this is closer to potential clinical interventions in individuals with FXS. We first asked whether IBMX, a non-specific cAMP and cGMP PDE inhibitor, could rescue avoidance behavior in FXS flies. IBMX administration for 5 days resulted in a significant increase in avoidance behavior in FMRB55 and FMR13 flies (Figures 3C,D). Interestingly, Rolipram, a selective PDE4 (cAMP specific) inhibitor shown to improve olfactory and courtship memory (Choi et al., 2015, 2016), did not lead to significant improvement in avoidance (data not shown). This may suggest that both cAMP and cGMP need to be modulated for rescue of avoidance behavior. We reasoned that other PDEs may be required for dSO rescue in dfmr1 mutants. Therefore, we used 8-(4 Chlorophenylthio) adenosine 3′,5′-cyclic monophosphate sodium (8-CPT) which is an activator of cAMP-dependent PKA and inhibitor of cGMP dependent PDE. Administration for 5 days of 8-CPT resulted in a significant rescue of dSO avoidance in FMRB55 and FMR13 flies (Figures 4A,B). Then, we tested dipyridamole, a United States Food and Drug Administration (FDA) approved drug which increases cAMP levels via both inhibition of PDE-dependent cGMP degradation and adenosine-dependent cAMP synthesis. Dipyrididamole is an inhibitor of PDE 6 which inhibits cGMP and PDE 11 which inhibits both cAMP and cGMP degradation. We observed a significant improvement of FMRB55 (Figure 4C) mutants’ avoidance response after 1 day of treatment. We also tested another drug already FDA approved, Lithium, with effect on cAMP signaling and shown to improve learning and memory in FMRB55, FMR13 flies and FMR1 KO mice (Choi et al., 2015, 2016). We observed significant rescue of avoidance response in FMRB55 (Figure 4D) mutants with Lithium administration after 5 days of treatment (no effect was seen after 24 h treatment – not shown). Together, our pharmacological results strenghten the previous molecular work in FMR1 KO mice showing that FXS may involve both production and degradation of cAMP considering that FMRP binds to mRNAs for PDE regulating cAMP (PDE4B, PDE4DIP, PDE8B), but also cAMP and cGMP (PDE2A) (Darnell et al., 2011).
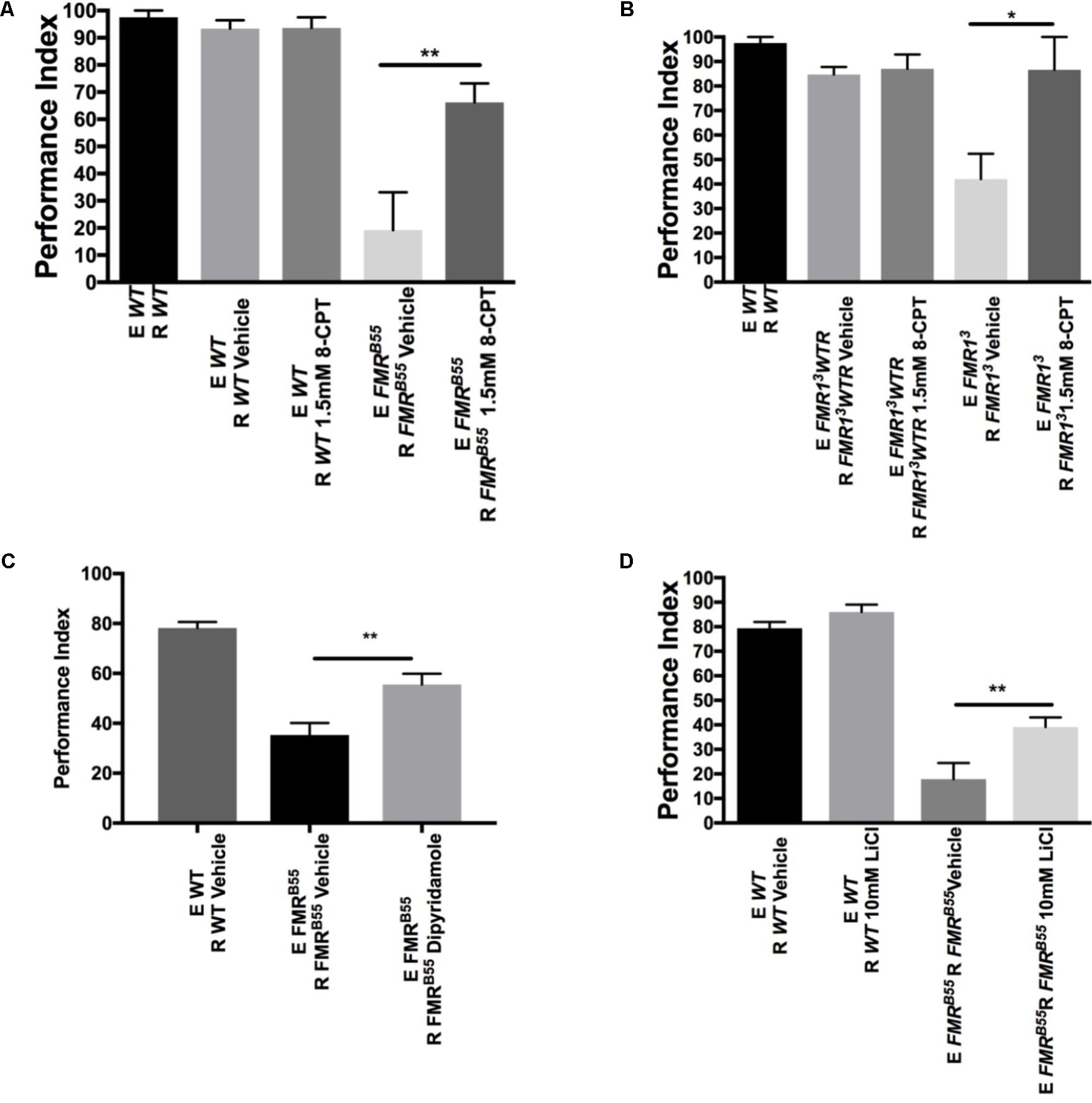
FIGURE 4. Pharmacological rescue of dSO avoidance with PDE antagonists in dfmr1 mutant flies. (A) FMRB55 flies treated for 5 days with 1.5 mM 8-CPT exhibited significantly increased avoidance behavior as compared to vehicle (Student’s t-test P = 0.0073; N = 5). 5-day treatment of WT flies with 1.5 mM 8-CPT did not result in any significant difference in avoidance behavior as compared to vehicle (Student’s t-test P = 0.9688; N = 5). (B) FMR13 flies treated for 5 days with 1.5 mM 8-CPT exhibited significantly increased avoidance behavior as compared to vehicle (Student’s t-test P = 0.0252; N = 6). 5-day treatment of FMR13WTR flies with 1.5 mM 8-CPT did not result in any significant difference in avoidance behavior as compared to vehicle (Student’s t-test P = 0.7334; N = 6). (C) FMRB55 flies treated for 1 day with 0.8 mM Dipyridamole exhibited significantly increased avoidance as compared to vehicle (Student’s t-test P = 0.0064; N = 8). (D) FMRB55 flies treated for 5 days with 10 mM LiCl exhibited significantly increased avoidance behavior as compared to vehicle (Student’s t-test P = 0.0094; N = 15). 5-day treatment of WT flies with 10 mM LiCl did not result in any significant difference in avoidance behavior as compared to vehicle (Student’s t-test P = 0.99; N = 15). All graphs depict mean ± SEM. ∗P < 0.05, ∗∗P < 0.01.
cAMP and cGMP Are Linked to Several ID and ASD Genes
Based on the recent report of interaction between FMR1 and several novel ASD candidate genes, we asked if other ID and ASD genes were linked to cAMP/cGMP signaling (Iossifov et al., 2014; Ronemus et al., 2014). This is important as treatment identified for FXS may then be tried in priority with other ID/ASD genes related molecularly. Using an in silico gene pathway analysis approach, we identified both ID and ASD genes interacting with cAMP (Figures 5A,C) and to a lesser extent cGMP (Figures 5B,D).
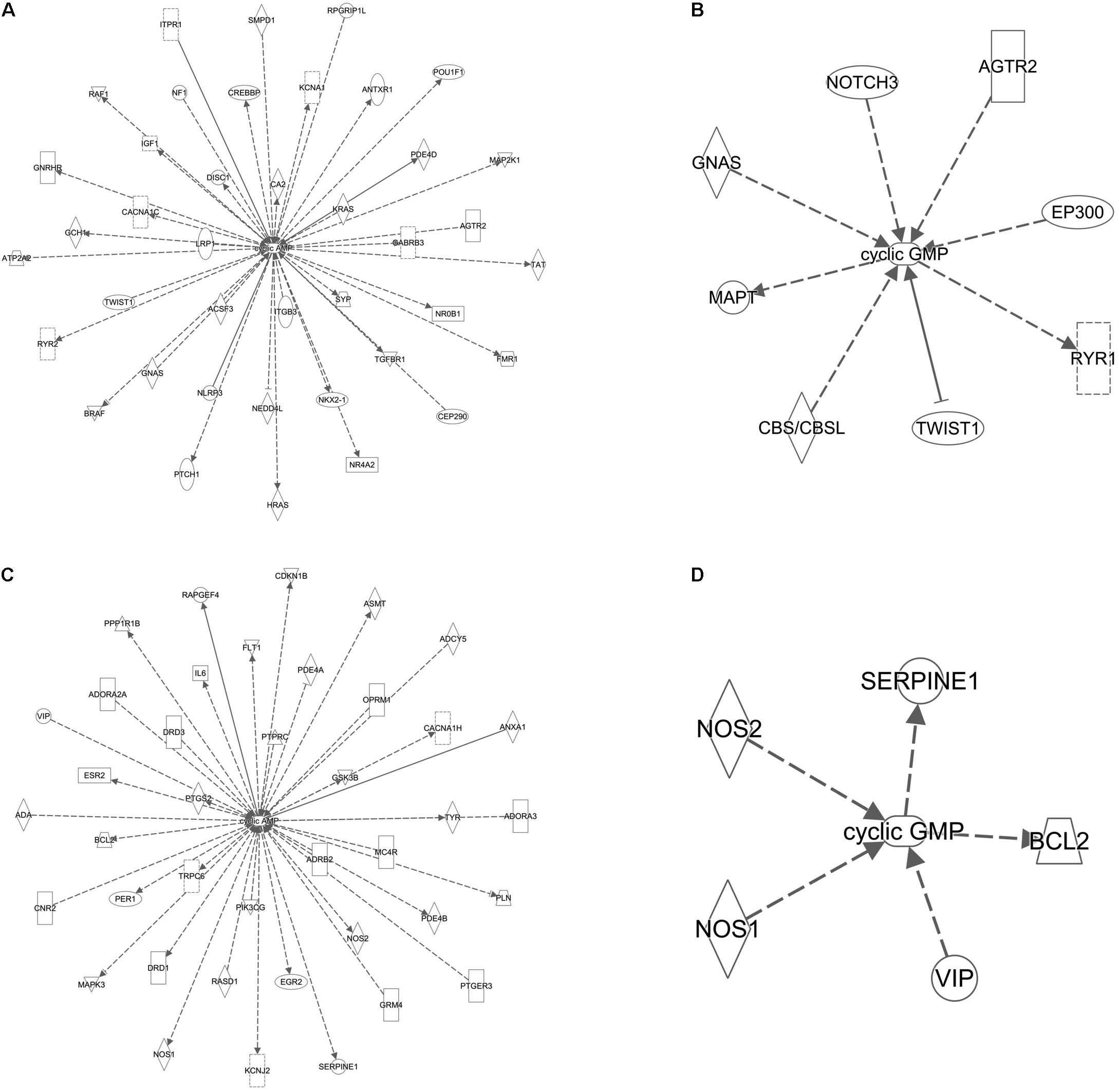
FIGURE 5. cAMP and cGMP are linked to several ID and ASD genes. (A) Gene network of ID genes and cAMP. (B) Gene network of ID genes and cGMP. (C) Gene network of ASD genes and cAMP. (D) Gene network of ASD genes and cGMP. Solid lines indicate direct experimental relationships; dotted lines indicate indirect experimental relationships. Arrows indicate an effect on the target molecule and line arrowheads indicate inhibition.
Discussion
Our work provides a novel application of dSO avoidance response assay as an endophenotype model to study sensory response behavior in Drosophila models of FXS and possibly other ID and autism causes. We show that sensory response required developmental dfmr1 expression while emission of the sensory cue (dSO) did not. Our results illustrate the importance of dfmr1 expression in the MB for typical dSO response. This parallels our previous finding showing that dfmr1 expression in MB was required for learning and memory (Bolduc et al., 2008) although the developmental but also acute expression of FMRP was linked to memory formation.
To our knowledge, this is the first time that dSO defects are rescued pharmacologically in a post-natal setting in dfmr1 mutants. This is a promising avenue for individuals with FXS suffering of SPD as both Lithium and dipyridamole are FDA approved drugs. As there is pre-clinical evidence showing a conserved deficit of cAMP across species in FXS (Kelley et al., 2007) and recent evidence of improvement of cognitive symptoms in fly and mouse models of FXS (Choi et al., 2015, 2016) with PDE4 inhibitors, our results underline the importance of a symptom specific approach in ID and ASD pharmacological intervention testing. Moreover, PDE-specific inhibitors are currently undergoing clinical trial for behavioral defects in FXS and it may be interesting to assess improvement in SPD. PDEs are well-conserved in flies and include highly conserved critical domains compared to human PDEs (Day et al., 2005). In Drosophila, there are seven genes encoding PDEs. The most studied is dunce which encodes a PDE4 ortholog and is required for olfactory learning and memory (Kauvar, 1982). More recently, orthologs for PDE1, PDE5 PDE6, PDE8, PDE9, and PDE11 were identified. Our results with IBMX show a strong effect and indicate that multiple signaling cascades may be impacted in FXS. Pharmacologically, IBMX is a complex drug. It inhibits PDE1, PDE2, PDE3, PDE4, PDE5, PDE7, and PDE 11, while PDE8 and PDE9 are insensitive to IBMX. In addition though, apart from its inhibitory effects on PDEs, IBMX has been shown in rat adipocytes to block the inhibitory regulatory protein, Gi, thereby stimulating AC and increasing intracellular cAMP levels (Parsons et al., 1988). IBMX and other xanthine-derived PDE inhibitors are also well-known adenosine receptor antagonists, consequently increasing cAMP production, which could also be a mode of action as it is “hypoactive” in FXS (Daly et al., 1981; Morgan et al., 1993). Indeed, our results and previous molecular evidence showing that FMRP binds to mRNA of PDEs regulating cGMP suggest that both cAMP and cGMP need to be considered in FXS. As cGMP has been shown to modulate cholinergic and dopaminergic signaling, it is possible that sensory processing requires a tight balance of both cAMP and cGMP (Moody et al., 1981). Maurin et al. (2018) recently showed the importance of PDE2a in FMR1KO mice which has been shown to regulate both cAMP and cGMP. Thus, further molecular dissection studies, for instance using neurons derived from induced pluripotent cells from FXS patients, with more specific PDE inhibitors and AC activators will be required prior to clinical trials.
In addition, our genetic manipulation of FMRP suggests that the defect in avoidance is routed in developmental defects. Importantly though, despite the absence of a clear effect in modulation of FMRP level in adult fly brain on avoidance response, pharmacological treatment of adult dfmr1 mutants can still improve avoidance performance defects. This implies a potential developmental origin of cognitive dysfunction, but also illustrates that pharmacological treatment should be considered even in absence of acute effect of the target gene on behavior. This raises the possibility that downstream consequences of the absence of dfmr1 during development, such as dysregulation in epigenetic marks (Korb et al., 2017) and/or structural defects (spine or neuronal network) (Comery et al., 1997; Mansilla et al., 2017) affecting cAMP equilibrium, established during development may be key to the avoidance defects and not the level of FMRP itself. This may be an important consideration when assessing the potential benefit of post-natal treatment in animal models of neurodevelopmental disability.
Finally, treatment targeting cAMP and cGMP may be of benefit to other individuals with neurodevelopmental disorders considering how ID and ASD genes are linked to cAMP-cGMP signaling in silico. This raises the need for high-throughput, but clinically relevant systems, to test not only multiple candidate drugs, but several genes.
Author Contributions
AA co-designed the experiments, performed most of the experiments, analyzed the results, and co-wrote the manuscript. RH performed the in silico pathway analyses and assisted in manuscript preparation. SW performed some of the behavioral experiments. CR assisted with the drug preparation and advice on the experimental design. FB co-designed the experiments, analyzed the results, and co-wrote the paper.
Funding
This work was supported by the Canadian Institute of Health Research (CIHR) (FB). FB is supported by the Career Development Award from the Canadian Child Health Clinician-Scientist Program.
Conflict of Interest Statement
The authors declare that the research was conducted in the absence of any commercial or financial relationships that could be construed as a potential conflict of interest.
Acknowledgments
We would like to thank the reviewers for their constructive comments. We would like to thank Ms. Kerri Whitlock for her comments on the manuscript. Some of the results were obtained as part of the Master in Medical Science in Pediatrics to AA. We would like to thank Dr. Sarah Hughes (University of Alberta) for the generous use of the Zeiss LSM 700 Confocal Microscope. We would also like to thank Dr. Tim Tully (Dart Neuroscience) for the WTw118-202U, Elav-Gal4, OK107-Gal4, 247-Gal4, and 747-Gal4 flies, Dr. Tom Jongens (University of Pennsylvania) for the dfmr13 and WTR and ELAV-Gal80ts flies, and Dr. Kendal Broadie (Vanderbilt University) for the dfmr1B55 flies.
Supplementary Material
The Supplementary Material for this article can be found online at: https://www.frontiersin.org/articles/10.3389/fnmol.2018.00242/full#supplementary-material
FIGURE 1. Spatial requirement and CO2 response in dfmr1 mutants. (A) Feb170-Gal4:UAS-dfmr1RNAi1-7 flies did not exhibit any defect in avoidance response compared to WT flies (Student’s t-test P = 0.8973; N = 10). (B) Feb170-Gal4:UAS-dfmr1RNAi1-7 flies did not exhibit any defect in avoidance when tested against WT dSO (Student’s t-test P = 0.2119; N = 10). (C) FMRB55 (Student’s t-test P < 0.0001; N = 6) and FMR13 (Student’s t-test P = 0.0013; N = 6) flies exhibited significantly decreased avoidance to CO2(g) at a concentration of 0.2 mL/min compared to WT flies. (D) FMRB55 (Student’s t-test P < 0.0001; N = 10) and FMR13 (Student’s t-test P = 0.0009; N = 13) flies exhibited significantly decreased avoidance to CO2(g) at a concentration of 0.5 mL/min compared to WT flies. All graphs depict mean ± SEM. ∗P < 0.05, ∗∗P < 0.01, ∗∗∗P < 0.001.
References
Androschuk, A. (2016). Cyclic Adenosine Monophosphate (cAMP) and Fragile X Mental Retardation Protein (FMRP) Mediate Avoidance Behaviour in Drosophila. Master thesis, University of Alberta, Edmonton, AB.
Androschuk, A., Al-Jabri, B., and Bolduc, F. V. (2015). From learning to memory: what flies can tell us about intellectual disability treatment. Front. Psychiatry 6:85. doi: 10.3389/fpsyt.2015.00085
Aso, Y., Grübel, K., Busch, S., Friedrich, A. B., Siwanowicz, I., and Tanimoto, H. (2009). The mushroom body of adult Drosophila characterized by GAL4 drivers. J. Neurogenet. 23, 156–172. doi: 10.1080/01677060802471718
Bailey, D. B. Jr., Raspa, M., Bishop, E., Mitra, D., Martin, S., Wheeler, A., et al. (2012). Health and economic consequences of fragile X syndrome for caregivers. J. Dev. Behav. Pediatr. 33, 705–712. doi: 10.1097/DBP.0b013e318272dcbc
Baranek, G. T., Chin, Y. H., Hess, L. M., Yankee, J. G., Hatton, D. D., and Hooper, S. R. (2002). Sensory processing correlates of occupational performance in children with fragile X syndrome: preliminary findings. Am. J. Occup. Ther. 56, 538–546. doi: 10.5014/ajot.56.5.538
Berry-Kravis, E., Freedman, S. B., and Dawson, G. (1984). Specific receptor-mediated inhibition of cyclic AMP synthesis by dopamine in a neuroblastoma X brain hybrid cell line NCB-20. J. Neurochem. 43, 413–420. doi: 10.1111/j.1471-4159.1984.tb00917.x
Berry-Kravis, E., Hicar, M., and Ciurlionis, R. (1995). Reduced cyclic AMP production in fragile X syndrome: cytogenetic and molecular correlations. Pediatr. Res. 38, 638–643. doi: 10.1203/00006450-199511000-00002
Bolduc, F. V., Bell, K., Cox, H., Broadie, K. S., and Tully, T. (2008). Excess protein synthesis in Drosophila fragile X mutants impairs long-term memory. Nat. Neurosci. 11, 1143–1145. doi: 10.1038/nn.2175
Bolduc, F. V., Valente, D., Nguyen, A. T., Mitra, P. P., and Tully, T. (2010). An assay for social interaction in Drosophila fragile X mutants. Fly 4, 216–225. doi: 10.4161/fly.4.3.12280
Bräcker, L. B., Siju, K. P., Varela, N., Aso, Y., Zhang, M., Hein, I., et al. (2013). Essential role of the mushroom body in context-dependent CO2 avoidance in Drosophila. Curr. Biol. 23, 1228–1234. doi: 10.1016/j.cub.2013.05.029
Brand, A. H., and Perrimon, N. (1993). Targeted gene expression as a means of altering cell fates and generating dominant phenotypes. Development 118, 401–415.
Chang, Y. S., Owen, J. P., Desai, S. S., Hill, S. S., Arnett, A. B., Harris, J., et al. (2014). Autism and sensory processing disorders: shared white matter disruption in sensory pathways but divergent connectivity in social-emotional pathways. PLoS One 9:e103038. doi: 10.1371/journal.pone.0103038
Choi, C. H., Schoenfeld, B. P., Bell, A. J., Hinchey, J., Rosenfelt, C., Gertner, M. J., et al. (2016). Multiple drug treatments that increase camp signaling restore long-term memory and aberrant signaling in fragile X syndrome models. Front. Behav. Neurosci. 10:136. doi: 10.3389/fnbeh.2016.00136
Choi, C. H., Schoenfeld, B. P., Weisz, E. D., Bell, A. J., Chambers, D. B., Hinchey, J., et al. (2015). PDE-4 inhibition rescues aberrant synaptic plasticity in Drosophila and mouse models of fragile X syndrome. J. Neurosci. 35, 396–408. doi: 10.1523/JNEUROSCI.1356-12.2015
Comery, T. A., Harris, J. B., Willems, P. J., Oostra, B. A., Irwin, S. A., Weiler, I. J., et al. (1997). Abnormal dendritic spines in fragile X knockout mice: maturation and pruning deficits. Proc. Natl. Acad. Sci. U.S.A. 94, 5401–5404. doi: 10.1073/pnas.94.10.5401
Crane, L., Goddard, L., and Pring, L. (2009). Sensory processing in adults with autism spectrum disorders. Autism 13, 215–228. doi: 10.1177/1362361309103794
Daly, J. W., Bruns, R. F., and Snyder, S. H. (1981). Adenosine receptors in the central nervous system: relationship to the central actions of methylxanthines. Life Sci. 28, 2083–2097. doi: 10.1016/0024-3205(81)90614-7
Darnell, J. C., Van Driesche, S. J., Zhang, C., Hung, K. Y., Mele, A., Fraser, C. E., et al. (2011). FMRP stalls ribosomal translocation on mRNAs linked to synaptic function and autism. Cell 146, 247–261. doi: 10.1016/j.cell.2011.06.013
Day, J. P., Dow, J. A., Houslay, M. D., and Davies, S. A. (2005). Cyclic nucleotide phosphodiesterases in Drosophila melanogaster. Biochem. J. 388, 333–342. doi: 10.1042/BJ20050057
Engel-Yeger, B., Hardal-Nasser, R., and Gal, E. (2011). Sensory processing dysfunctions as expressed among children with different severities of intellectual developmental disabilities. Res. Dev. Disabil. 32, 1770–1775. doi: 10.1016/j.ridd.2011.03.005
Gilissen, C., Hehir-Kwa, J. Y., Thung, D. T., van de Vorst, M., van Bon, B. W., Willemsen, M. H., et al. (2014). Genome sequencing identifies major causes of severe intellectual disability. Nature 511, 344–347. doi: 10.1038/nature13394
Iossifov, I., O’Roak, B. J., Sanders, S. J., Ronemus, M., Krumm, N., Levy, D., et al. (2014). The contribution of de novo coding mutations to autism spectrum disorder. Nature 515, 216–221. doi: 10.1038/nature13908
Joosten, A. V., and Bundy, A. C. (2010). Sensory processing and stereotypical and repetitive behaviour in children with autism and intellectual disability. Aust. Occup. Ther. J. 57, 366–372. doi: 10.1111/j.1440-1630.2009.00835.x
Kanellopoulos, A. K., Semelidou, O., Kotini, A. G., Anezaki, M., and Skoulakis, E. M. (2012). Learning and memory deficits consequent to reduction of the fragile X mental retardation protein result from metabotropic glutamate receptor-mediated inhibition of cAMP signaling in Drosophila. J. Neurosci. 32, 13111–13124. doi: 10.1523/JNEUROSCI.1347-12.2012
Kauvar, L. M. (1982). Defective cyclic adenosine 3′:5′-monophosphate phosphodiesterase in the Drosophila memory mutant dunce. J. Neurosci. 2, 1347–1358. doi: 10.1523/JNEUROSCI.02-10-01347.1982
Kelley, D. J., Davidson, R. J., Elliott, J. L., Lahvis, G. P., Yin, J. C., and Bhattacharyya, A. (2007). The cyclic AMP cascade is altered in the fragile X nervous system. PLoS One 2:e931. doi: 10.1371/journal.pone.0000931
Kern, J. K., Trivedi, M. H., Garver, C. R., Grannemann, B. D., Andrews, A. A., Savla, J. S., et al. (2006). The pattern of sensory processing abnormalities in autism. Autism 10, 480–494. doi: 10.1177/1362361306066564
Klengel, T., Liang, W. J., Chaloupka, J., Ruoff, C., Schröppel, K., Naglik, J. R., et al. (2005). Fungal adenylyl cyclase integrates CO2 sensing with cAMP signaling and virulence. Curr. Biol. 15, 2021–2026. doi: 10.1016/j.cub.2005.10.040
Korb, E., Herre, M., Zucker-Scharff, I., Gresack, J., Allis, C. D., and Darnell, R. B. (2017). Excess translation of epigenetic regulators contributes to fragile X syndrome and is alleviated by BRD4 inhibition. Cell 170, 1209.e20–1223.e20. doi: 10.1016/j.cell.2017.07.033
Lane, A. E., Young, R. L., Baker, A. E., and Angley, M. T. (2010). Sensory processing subtypes in autism: association with adaptive behavior. J. Autism Dev. Disord. 40, 112–122. doi: 10.1007/s10803-009-0840-2
Lin, H. H., Chu, L. A., Fu, T. F., Dickson, B. J., and Chiang, A. S. (2013). Parallel neural pathways mediate CO2 avoidance responses in Drosophila. Science 340, 1338–1341. doi: 10.1126/science.1236693
Mansilla, A., Chaves-Sanjuan, A., Campillo, N. E., Semelidou, O., Martínez-González, L., Infantes, L., et al. (2017). Interference of the complex between NCS-1 and Ric8a with phenothiazines regulates synaptic function and is an approach for fragile X syndrome. Proc. Natl. Acad. Sci. U.S.A. 114, E999–E1008. doi: 10.1073/pnas.1611089114
Marco, E. J., Hinkley, L. B., Hill, S. S., and Nagarajan, S. S. (2011). Sensory processing in autism: a review of neurophysiologic findings. Pediatr. Res. 69, 48R–54R. doi: 10.1203/PDR.0b013e3182130c54
Maurin, T., Lebrigand, K., Castagnola, S., Paquet, A., Jarjat, M., Popa, A., et al. (2018). HITS-CLIP in various brain areas reveals new targets and new modalities of RNA binding by fragile X mental retardation protein. Nucleic Acids Res. doi: 10.1093/nar/gky267 [Epub ahead of print].
McBride, S. M., Choi, C. H., Wang, Y., Liebelt, D., Braunstein, E., Ferreiro, D., et al. (2005). Pharmacological rescue of synaptic plasticity, courtship behavior, and mushroom body defects in a Drosophila model of fragile X syndrome. Neuron 45, 753–764. doi: 10.1016/j.neuron.2005.01.038
Moody, T. W., Taylor, D. P., and Pert, C. B. (1981). Effects of guanine nucleotides on CNS neuropeptide receptors. J. Supramol. Struct. Cell. Biochem. 15, 153–159. doi: 10.1002/jsscb.1981.380150206
Morgan, A. J., Murray, K. J., and Challiss, R. A. (1993). Comparison of the effect of isobutylmethylxanthine and phosphodiesterase-selective inhibitors on cAMP levels in SH-SY5Y neuroblastoma cells. Biochem. Pharmacol. 45, 2373–2380. doi: 10.1016/0006-2952(93)90216-J
Owen, J. P., Marco, E. J., Desai, S., Fourie, E., Harris, J., Hill, S. S., et al. (2013). Abnormal white matter microstructure in children with sensory processing disorders. Neuroimage Clin. 2, 844–853. doi: 10.1016/j.nicl.2013.06.009
Parsons, W. J., Ramkumar, V., and Stiles, G. L. (1988). Isobutylmethylxanthine stimulates adenylate cyclase by blocking the inhibitory regulatory protein, Gi. Mol. Pharmacol. 34, 37–41.
Ronemus, M., Iossifov, I., Levy, D., and Wigler, M. (2014). The role of de novo mutations in the genetics of autism spectrum disorders. Nat. Rev. Genet. 15, 133–141. doi: 10.1038/nrg3585
Skoulakis, E. M., Kalderon, D., and Davis, R. L. (1993). Preferential expression in mushroom bodies of the catalytic subunit of protein kinase A and its role in learning and memory. Neuron 11, 197–208. doi: 10.1016/0896-6273(93)90178-T
Suh, G. S., Wong, A. M., Hergarden, A. C., Wang, J. W., Simon, A. F., Benzer, S., et al. (2004). A single population of olfactory sensory neurons mediates an innate avoidance behaviour in Drosophila. Nature 431, 854–859. doi: 10.1038/nature02980
Keywords: Fragile X syndrome, sensory response dysfunction, Drosophila, cAMP, cGMP, avoidance response, IBMX
Citation: Androschuk A, He RX, Weber S, Rosenfelt C and Bolduc FV (2018) Stress Odorant Sensory Response Dysfunction in Drosophila Fragile X Syndrome Mutants. Front. Mol. Neurosci. 11:242. doi: 10.3389/fnmol.2018.00242
Received: 04 January 2018; Accepted: 22 June 2018;
Published: 08 August 2018.
Edited by:
Regina Dahlhaus, Friedrich-Alexander-Universität Erlangen-Nürnberg, GermanyReviewed by:
Efthimios M. C. Skoulakis, Alexander Fleming Biomedical Sciences Research Center, GreeceBarbara Bardoni, UMR7275 Institut de Pharmacologie Moléculaire et Cellulaire (IPMC), France
Copyright © 2018 Androschuk, He, Weber, Rosenfelt and Bolduc. This is an open-access article distributed under the terms of the Creative Commons Attribution License (CC BY). The use, distribution or reproduction in other forums is permitted, provided the original author(s) and the copyright owner(s) are credited and that the original publication in this journal is cited, in accordance with accepted academic practice. No use, distribution or reproduction is permitted which does not comply with these terms.
*Correspondence: Francois V. Bolduc, fbolduc@ualberta.ca