Epigenetic embedding of childhood adversity: mitochondrial metabolism and neurobiology of stress-related CNS diseases
- 1Department of Psychiatry, New York University Grossman School of Medicine, New York, NY, United States
- 2Center for Dementia Research, Nathan S. Kline Institute for Psychiatric Research, Orangeburg, NY, United States
- 3Harold and Margaret Milliken Hatch Laboratory of Neuroendocrinology, Rockefeller University, New York, NY, United States
- 4Department of Population Health, New York University Grossman School of Medicine, New York, NY, United States
- 5Department of Neuroscience and Physiology, New York University Grossman School of Medicine, New York, NY, United States
This invited article ad memoriam of Bruce McEwen discusses emerging epigenetic mechanisms underlying the long and winding road from adverse childhood experiences to adult physiology and brain functions. The conceptual framework that we pursue suggest multidimensional biological pathways for the rapid regulation of neuroplasticity that utilize rapid non-genomic mechanisms of epigenetic programming of gene expression and modulation of metabolic function via mitochondrial metabolism. The current article also highlights how applying computational tools can foster the translation of basic neuroscience discoveries for the development of novel treatment models for mental illnesses, such as depression to slow the clinical manifestation of Alzheimer’s disease. Citing an expression that many of us heard from Bruce, while “It is not possible to roll back the clock,” deeper understanding of the biological pathways and mechanisms through which stress produces a lifelong vulnerability to altered mitochondrial metabolism can provide a path for compensatory neuroplasticity. The newest findings emerging from this mechanistic framework are among the latest topics we had the good fortune to discuss with Bruce the day before his sudden illness when walking to a restaurant in a surprisingly warm evening that preluded the snowstorm on December 18th, 2019. With this article, we wish to celebrate Bruce’s untouched love for Neuroscience.
Mitochondrial metabolism and epigenetic function
Epigenetic mechanisms are involved in the pathophysiology of stress-related diseases, including depressive and cognitive disorders as well as opioid and alcohol use disorders, and are emerging as potential targets for therapeutic interventions(Sweatt, 2010; Robison and Nestler, 2011; Nestler, 2014; McEwen et al., 2015). Work from our group introduced the McEwen lab to acetyl-L-carnitine (LAC), a central mitochondrial metabolite that was best known for its role in fatty acid oxidation (Fritz and McEwen, 1959; Pettegrew et al., 2000; McEwen et al., 2015; McEwen, 2018). In rodents, administration of LAC leads to a rapid and persistent antidepressant-like response by activating histone acetyltransferases (e.g.: P300) to regulate histone acetylation and expression of key genes, including the metabotropic glutamate receptor-2 (mGlu2, inhibitor of spontaneous glutamate release) and the downstream brain-derived neurotrophic factor BDNF (Flight, 2013; Nasca et al., 2013; Russo and Charney, 2013; Nasca et al., 2017). Boosting mitochondrial metabolism of LAC also leads to the amelioration of specific cognitive domains (Barnes et al., 1990; Liu et al., 2002). These potent epigenetic effects of LAC occur in brain areas such as the hippocampus, which is implicated in the pathophysiology of major depressive disorders (MDD) and is among the first brain structures to degenerate in Alzheimer’s disease (AD) (Braak et al., 1993; McEwen et al., 2016). Furthermore, the rapid effects of LAC extend to other key brain areas important not only for mood disorders but also for substance abuse disorders such as the prefrontal cortex, nucleus accumbens and amygdala (Nasca et al., 2013; Lau et al., 2017; Cherix et al., 2020). In rodent models (e.g., mice after exposure to chronic stress or the flinders sensitive genetic line FSL rats), peripheral and central (e.g., in the hippocampus and prefrontal cortex) LAC levels are decreased; and boosting mitochondrial metabolism of LAC rapidly regulates a metabolic dysfunction known as insulin resistance (IR) (Eriksson et al., 2012; Nasca et al., 2013; Bigio et al., 2016). In recent years, this mechanistic framework in rodents led to test novel hypotheses in humans in pursuit of developing disease-modifying drugs and precision medicine strategies for stress-related main CNS diseases.
From basic neuroscience discoveries to translational research
In subjects suffering from MDD, LAC levels are decreased as compared to age-and sex-matched controls; the degree of LAC deficiency reflected both the severity and age of onset of depression (Nasca et al., 2018; Post, 2018; Nasca et al., 2020). We found the lowest levels of LAC in severe clinical phenotypes of treatment resistant depression associated with early life stress in the form of childhood emotional trauma. Utilization of esketamine as an antidepressant increases LAC levels (Rotroff et al., 2016). Decreased levels of LAC are also predictive of lack of antidepressant responses to the insulin-sensitizing agent pioglitazone used as an antidepressant in subjects suffering from MDD (Nasca et al., 2021). Recently, we also showed a relationship between the epigenetic modulation of glutamatergic function and central IR as assessed by measures of the insulin signaling cascade in exosomes enriched for the neural cell adhesion molecule L1 (L1-CAM), a protein highly expressed in the brain (Takahashi et al., 2012; Lonsdale et al., 2013; Uhlen et al., 2015; Nasca et al., 2020). As we and others reviewed elsewhere (Kenna et al., 2013; Biessels and Reagan, 2015; Grillo et al., 2015; Rasgon and McEwen, 2016; Arnold et al., 2018; Ferrario and Reagan, 2018; Watson et al., 2018), in addition to systemic energy metabolism, insulin signaling contributes to regulate neuroplasticity and is reflective of cerebral hypo-metabolism and aberrant intrinsic connectivity of intra-and inter-hippocampal circuits. A growing literature suggests that IR—which is ameliorated by boosting mitochondrial metabolism of LAC in rodent models—is one of the steps in the irreversible activation of the cascade leading from mood disorders to AD (Byers and Yaffe, 2011; De Felice et al., 2014; Rasgon and McEwen, 2016; De Felice et al., 2022).
In the connection between mitochondrial metabolism and aging, prior work reported decreased levels of LAC in subjects with AD as compared to cognitively healthy controls, with intermediate levels in subjects with subjective memory complaint or mild cognitive impairment (MCI) (Cristofano et al., 2016). As we elaborated above, these translational findings are an outgrowth of a mechanistic model in rodents with impaired plasticity of key brain areas relevant to mood, cognitive, opioid and alcohol use disorders, wherein LAC levels are markedly decreased and signal abnormal brain and systemic functions. The current mechanistic model compels further research to identify new signaling pathways and mechanisms for developing novel treatment models for mental illness, ultimately to slow the clinical manifestation to dementia (Byers and Yaffe, 2011; Rasgon and McEwen, 2016).
Early life stress and adverse childhood experiences
At the same time, while we continue to learn the role of multidimensional biological pathways—which utilize rapid non-genomic mechanisms of epigenetic regulation of gene expression and modulation of metabolic function—in neuroplasticity (McEwen et al., 2015), there is increasing recognition that adverse childhood experiences disproportionately influence lifelong vulnerability to develop mood and cognitive disorders (McEwen, 2003; McEwen et al., 2015; Nemeroff, 2016). Prior gene expression studies showed a brain that continually changes with experience and that the biological embedding of trajectories of neuroplasticity starts in early life (Shonkoff et al., 2009; Gray et al., 2014). Adverse experiences, such as decreased maternal care early in life, leads to lifelong changes in the epigenome (e.g.: histone acetylation) and the related expression of key genes for the responses to stress in the hippocampus; and these changes are accompanied by behavioral deficits (Meaney et al., 1988; Weaver et al., 2004). Regarding excessive glutamate overflow, mice with inherent anxiety at baseline show elevated expression of the mineralocorticoid receptors (MR) in the hippocampus that predisposes to a stress-induced suppression of mGlu2 expression and development of depressive-like behavior. Blocking MR receptors and interfering with glucocorticoids stimulation of glutamate activity counteracts stress-induced behavioral abnormalities. Yet, the nature of the experiences of the animals that develop increased MR expression is not known but might involve epigenetic experiences early in life, such as maternal care and stressors in the neonatal nesting environment as we fully described in the epigenetic allostasis model (Nasca et al., 2015).
Exposure to early life stress also leads to a decrease in hippocampal volume in adult subjects suffering from MDD (Saleh et al., 2017). Recent studies also showed a decreased hippocampal volume in children with depression (Barch et al., 2019; Zovetti et al., 2022). Timing of the stress is found to negatively affect hippocampal volume; the strongest effects were found when stress exposure occurred before 5 years of age (Gerritsen et al., 2015; Humphreys et al., 2019). Anxiety and co-dependence of the mother during the first weeks after birth also resulted in long-lasting effects on the hippocampal volume in young adult offspring, as well as in children from low socioeconomic status households (Hanson et al., 2015; Mareckova et al., 2018). In the connection to metabolism, studies in humans showed that childhood trauma is not only a risk factor for aberrant mitochondrial metabolism in severe clinical phenotypes of treatment resistant depression, but also for IR as well as for shortening of leukocyte telomere length (LTL, a marker of cellular aging) (Price et al., 2013; Nasca et al., 2018; Post, 2018; Nasca et al., 2021). We reported that emotional trauma is a critical factor for the decreased LAC levels in severe clinical phenotypes of treatment resistant depression (Nasca et al., 2018; Post, 2018). We showed a relationship between emotional trauma, but none of the other subscales of the childhood trauma, and antidepressant responses as a function of LAC levels and the corresponding IR and LTL (Nasca et al., 2021). The specificity of these effects is in agreement with prior studies showing that the consequences of emotional maltreatments in childhood differ from those of physical and sexual abuse (Nemeroff, 2016; Williams et al., 2016). There is also considerable evidence that childhood trauma, particularly emotional maltreatment, impairs responses to antidepressant drugs (Nemeroff et al., 2003; Nemeroff, 2016). Although there are fewer studies describing how specific subscales of childhood trauma affect the responses to drugs that ameliorate cognitive function, prior work suggested that adverse childhood experiences result in poorer response to cognitive-behavioral therapy (Short and Baram, 2019). Collectively, the current work raises the hypothesis for future studies that targeting mitochondrial metabolism opens windows of epigenetic plasticity to re-direct the life course trajectories toward more positive health outcomes when adverse childhood experiences occurred.
Importance of the anterior (human) or ventral (rodent) hippocampus in stress and CNS disorders
Growing literature showed that the ventral hippocampus (vHIPP) in rodents (anterior hippocampus in humans) is a stress-sensitive circuit and a neural hub key for the regulation of behaviors implicated in depression, such as social interaction and anhedonia, as well as for cognitive functions. The vHIPP is also a target for antidepressant action of rapid acting agents, such as ketamine and LAC (Jett et al., 2015; Carreno et al., 2016). The vHIPP is connected to limbic areas involved in affective, reward and cognitive functions. It receives intense inputs from the ventromedial parts of the entorhinal cortex, carrying information arising from the infralimbic and prelimbic cortices as well as from the ventral tegmental area in the midbrain and the locus coeruleus and raphe nuclei in the brain stem. In turn, the vHIPP sends projections to the prefrontal cortex, nucleus accumbens, amygdala, and hypothalamus (Jonas and Lisman, 2014) among other brain areas. Therefore, plasticity of the ventral hippocampus is key in mediating changes in behaviors and cognitive functions. For example, vHIPP glutamatergic afferents to the nucleus accumbens regulate susceptibility to social defeat stress and the corresponding behavioral responses as shown by optogenetic studies (Bagot et al., 2015).
Computational approaches and multidimensional predictors of health trajectories
This is a time of enormous technological advance in so many aspects of neuroscience and medicine that is bringing together basic, computational, and clinical laboratories to develop novel mechanism-based treatment models for specific clinical phenotypes of stress-related disorders. We are now increasingly recognizing that heterogeneous psychiatric disorders, such as MDD, are likely much more biologically distinct than are captured by self-report symptom clusters. Using functional magnetic resonance imaging (fMRI), recent work showed that subjects suffering from depression can be subdivided into four neurophysiological subtypes defined by distinct patterns of dysfunctional connectivity in limbic and frontostriatal networks (Drysdale et al., 2017). Subtypes 1 and 4 are mainly characterized by reduced connectivity of frontoamygdala and increased anxiety; subtypes 3 and 4 are characterized by hyper-connectivity of thalamic and frontostriatal networks with increased anhedonia and psychomotor retardation. The four biotypes also predicted different antidepressant responses to repetitive transcranial magnetic stimulation. Toward understanding of the molecular mechanisms that might characterize the four neurophysiological subtypes, recent work showed that boosting mitochondrial metabolism leads to a rapid amelioration of anhedonia-related behaviors in rodent models deficient in LAC, which we now know is also a factor in clinical phenotypes of treatment resistant depression, suggesting it might be a potential treatment target, especially for these specific biotypes (Nasca et al., 2013, 2018). It is also important to note that subjects with opioid use disorders, particularly those in methadone and buprenorphine treatment programs, manifest a significant anhedonia which is in turn linked to poorer responses to treatment for tobacco use (Leventhal et al., 2009; Roys et al., 2016; Cook et al., 2017; Parker et al., 2020; Streck et al., 2020). These findings compel further research to understand whether certain aspects of mitochondrial metabolism and the related anhedonic states might serve as new targets for the development of more effective mechanism-based treatment models for psychiatric and substance abuse disorders.
Using hierarchical clustering to integrate molecular measures with clinical symptoms in those patients suffering from MDD characterized by a LAC deficiency, we also found that specific symptoms related to anhedonia, depressed mood, feelings of guilt, and suicidality are accompanied by a brain metabolic dysfunction known as IR as showed by an increase and sex-specific phosphorylation in the expression of IRS1, a key marker of the insulin signaling cascade, in discrete exosomes enriched for the brain (Nasca et al., 2020). These findings provided the closest available in vivo molecular signature for brain IR in depression and showed important sex differences in these pathways. In keeping with the important role of childhood trauma on adult physiology and brain functions, prediction profile modelling revealed that those patients suffering from MDD and characterized by decreased baseline LAC levels, elevated BMI and high reported rates of emotional abuse show the worst antidepressant responses; conversely, those patients with increased baseline LAC levels, decreased BMI and low reported rates of emotional abuse show decreased depression severity at the HDRS-21 in the responses to the insulin-sensitizing agent pioglitazone used as an antidepessant (Nasca et al., 2021). These recent findings suggest that multidimensional factors spanning mitochondrial metabolism, cellular aging, metabolic function, and childhood trauma provide more detailed signatures to predict antidepressant responses. Integrating molecular and circuit-level approaches has the potential to transform our understanding of the molecular underpinning of circuit-level abnormalities, and inform future efforts to develop personalized interventions with the potential for significantly enhanced efficacy compared to the current standard-of-care.
An additional application of computational tools includes the integration of multidimensional phenotypic measures to identify those mechanisms that predispose apparently healthy individuals to develop maladaptive coping strategies from those that confer resilience. Using a high-throughput unbiased automated phenotyping platform that collects >2000 behavioral features based on machine learning, recent work showed that a rich set of behavioral alterations distinguish susceptible versus resilient phenotypes after exposure to social defeat stress (SDS) (Lorsch et al., 2021). Interrelated brain–body marker characterize these phenotypes before any applied stress. We showed that a subgroup of mice characterized, at baseline, by increased anxiety at the light–dark test, the corresponding elevation of pro-inflammatory cytokine IL-6 as well as smaller hippocampal volume develops behavioral and neurobiological deficits after exposure to SDS, with social withdrawal and impaired transcriptomic-wide changes in ventral dentate gyrus (Nasca et al., 2019). At the individual level, a computational approach used to integrate in vivo measures of anxiety and immune system function predicted if a given animal developed SDS-induced social withdrawal, or remained resilient, with a sensitivity of 80% that is stronger than the categorization power based on either individual measure alone (Nasca et al., 2019). The findings of a priori multidimensional biomarkers for predicting the behavioral deficits resulting from exposure to SDS suggests a unique approach to examine the individual trajectories of adaptative and maladaptive responses to stress, paving the way to develop integrative models of mechanisms leading to susceptibility versus resilience to stress.
Future directions: in pursuit of “windows of epigenetic plasticity” to re-direct trajectories of brain function
While prevention is paramount, identifying novel biological pathways and mechanisms through which stress, including childhood trauma, affects adult systemic physiology and brain functions is a timely topic crucial for the development of new mechanistic frameworks to build resilience or decrease vulnerability to main CNS diseases, where adverse events have happened. Beyond recognizing resilience as “achieving a positive outcome in the face of adversity” (McEwen et al., 2015), there appears to be a common denominator in the trajectories to stress-related disorders that we propose involves an epigenetic embedding of early life experiences through the mitochondrial metabolite LAC that, when supplemented, rapidly alters gene expression profiles to ameliorate behaviors and cognitive function in animal models deficient in LAC because of stress-induced causes. The concept of epigenetic embedding of early life experiences is akin to the original definition of epigenetics, wherein the emergence of characteristics of each individual is not evident from prior stages of development (Waddington, 1942). While it is not possible to “roll back the clock,” deeper understanding of the biological pathways and mechanisms through which adverse childhood experiences produce a lifelong vulnerability to altered mitochondrial metabolism can provide a path for compensatory neuroplasticity toward more positive health directions (Figure 1). The COVID-19 pandemic and the related biological and social fallouts further highlight the need for basic neuroscience research to identify new signaling pathways and mechanisms underlying the effects of stress on the brain and the rest of the body to develop mechanistic-based treatment models (COVID-19 Mental Disorders Collaborators, 2021; WHO, 2022).
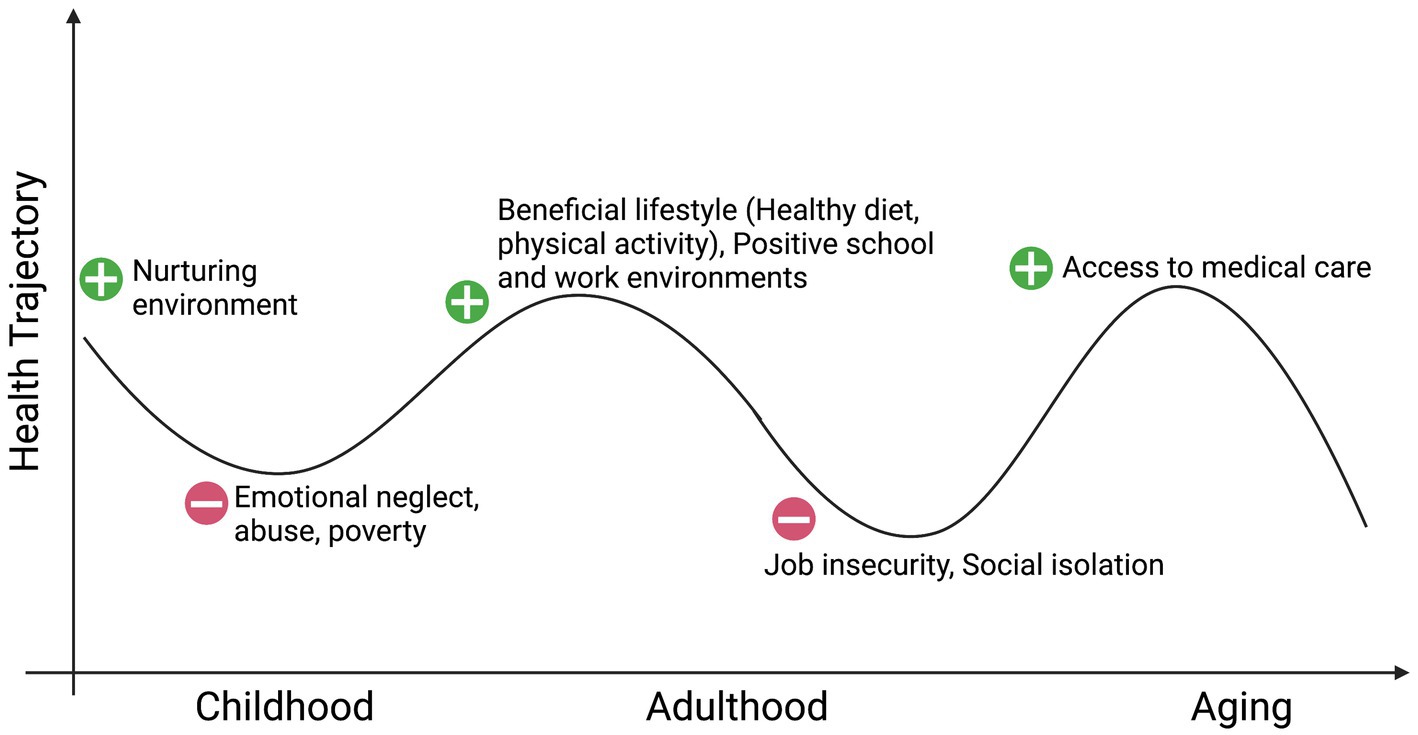
Figure 1. Social environment and health: in pursuit of windows of epigenetic plasticity. Created with BioRender.com.
Author contributions
CN and BB wrote the manuscript. BB, YS, OB, JD, OES, PA, and CN provided inputs to this article. All authors contributed to the article and approved the submitted version.
Funding
This work was supported by a R01MH128311, a R56MH125895, and a NARSAD Young Investigator Grant #30051 from the Brain & Behavior Research Foundation to CN, and by the National Institute on Aging (NIA) through the Early Adversity & Later Life Reversibility Pilot Grant to CN under Award Number R24AG06517.
Conflict of interest
The authors declare that the research was conducted in the absence of any commercial or financial relationships that could be construed as a potential conflict of interest.
Publisher’s note
All claims expressed in this article are solely those of the authors and do not necessarily represent those of their affiliated organizations, or those of the publisher, the editors and the reviewers. Any product that may be evaluated in this article, or claim that may be made by its manufacturer, is not guaranteed or endorsed by the publisher.
References
Arnold, S. E., Arvanitakis, Z., Macauley-Rambach, S. L., Koenig, A. M., Wang, H. Y., Ahima, R. S., et al. (2018). Brain insulin resistance in type 2 diabetes and Alzheimer disease: concepts and conundrums. Nat. Rev. Neurol. 14, 168–181. doi: 10.1038/nrneurol.2017.185
Bagot, R. C., Parise, E. M., Peña, C. J., Zhang, H. X., Maze, I., Chaudhury, D., et al. (2015). Ventral hippocampal afferents to the nucleus accumbens regulate susceptibility to depression. Nat. Commun. 6:7062. doi: 10.1038/ncomms8062
Barch, D. M., Tillman, R., Kelly, D., Whalen, D., Gilbert, K., and Luby, J. L. (2019). Hippocampal volume and depression among young children. Psychiatry Res. Neuroimaging 288, 21–28. doi: 10.1016/j.pscychresns.2019.04.012
Barnes, C. A., Markowska, A. L., Ingram, D. K., Kametani, H., Spangler, E. L., Lemken, V. J., et al. (1990). Acetyl-1-carnitine. 2: effects on learning and memory performance of aged rats in simple and complex mazes. Neurobiol. Aging 11, 499–506. doi: 10.1016/0197-4580(90)90110-l
Biessels, G. J., and Reagan, L. P. (2015). Hippocampal insulin resistance and cognitive dysfunction. Nat. Rev. Neurosci. 16, 660–671. doi: 10.1038/nrn4019
Bigio, B., Mathé, A. A., Sousa, V. C., Zelli, D., Svenningsson, P., McEwen, B. S., et al. (2016). Epigenetics and energetics in ventral hippocampus mediate rapid antidepressant action: implications for treatment resistance. Proc. Natl. Acad. Sci. U. S. A. 113, 7906–7911. doi: 10.1073/pnas.1603111113
Braak, H., Braak, E., and Bohl, J. (1993). Staging of Alzheimer-related cortical destruction. Eur. Neurol. 33, 403–408. doi: 10.1159/000116984
Byers, A. L., and Yaffe, K. (2011). Depression and risk of developing dementia. Nat. Rev. Neurol. 7, 323–331. doi: 10.1038/nrneurol.2011.60
Carreno, F. R., Donegan, J. J., Boley, A. M., Shah, A., DeGuzman, M., Frazer, A., et al. (2016). Activation of a ventral hippocampus-medial prefrontal cortex pathway is both necessary and sufficient for an antidepressant response to ketamine. Mol. Psychiatry 21, 1298–1308. doi: 10.1038/mp.2015.176
Cherix, A., et al. (2020). Metabolic signature in nucleus accumbens for anti-depressant-like effects of acetyl-L-carnitine. elife 9:e50631. doi: 10.7554/eLife.50631
Cook, J. W., Lanza, S. T., Chu, W., Baker, T. B., and Piper, M. E. (2017). Anhedonia: its dynamic relations with craving, negative affect, and treatment during a quit smoking attempt. Nicotine Tob. Res. 19, 703–709.
COVID-19 Mental Disorders Collaborators. (2021). Global prevalence and burden of depressive and anxiety disorders in 204 countries and territories in 2020 due to the COVID-19 pandemic. Lancet 398, 1700–1712. doi: 10.1016/S0140-6736(21)02143-7
Cristofano, A., Sapere, N., La Marca, G., Angiolillo, A., Vitale, M., Corbi, G., et al. (2016). Serum levels of Acyl-Carnitines along the Continuum from Normal to Alzheimer’s Dementia. PLoS One 11:e0155694. doi: 10.1371/journal.pone.0155694
De Felice, F. G., Gonçalves, R. A., and Ferreira, S. T. (2022). Impaired insulin signalling and allostatic load in Alzheimer disease. Nat. Rev. Neurosci. 23, 215–230. doi: 10.1038/s41583-022-00558-9
De Felice, F. G., Lourenco, M. V., and Ferreira, S. T. (2014). How does brain insulin resistance develop in Alzheimer's disease? Alzheimers Dement. 10, S26–S32. doi: 10.1016/j.jalz.2013.12.004
Drysdale, A. T., Grosenick, L., Downar, J., Dunlop, K., Mansouri, F., Meng, Y., et al. (2017). Resting-state connectivity biomarkers define neurophysiological subtypes of depression. Nat. Med. 23, 28–38. doi: 10.1038/nm.4246
Eriksson, T. M., Delagrange, P., Spedding, M., Popoli, M., Mathé, A. A., Ögren, S. O., et al. (2012). Emotional memory impairments in a genetic rat model of depression: involvement of 5-HT/MEK/arc signaling in restoration. Mol. Psychiatry 17, 173–184. doi: 10.1038/mp.2010.131
Ferrario, C. R., and Reagan, L. P. (2018). Insulin-mediated synaptic plasticity in the CNS: anatomical, functional and temporal contexts. Neuropharmacology 136, 182–191. doi: 10.1016/j.neuropharm.2017.12.001
Flight, M. H. (2013). Antidepressant epigenetic action. Nat. Rev. Neurosci. 14, 226–227. doi: 10.1038/nrn3466
Fritz, I. B., and McEwen, B. S. (1959). Effects of carnitine on fatty-acid oxidation by muscle. Science 129, 334–335. doi: 10.1126/science.129.3345.334
Gerritsen, L., Kalpouzos, G., Westman, E., Simmons, A., Wahlund, L. O., Bäckman, L., et al. (2015). The influence of negative life events on hippocampal and amygdala volumes in old age: a life-course perspective. Psychol. Med. 45, 1219–1228. doi: 10.1017/S0033291714002293
Gray, J. D., Rubin, T. G., Hunter, R. G., and McEwen, B. S. (2014). Hippocampal gene expression changes underlying stress sensitization and recovery. Mol. Psychiatry 19, 1171–1178. doi: 10.1038/mp.2013.175
Grillo, C. A., Piroli, G. G., Lawrence, R. C., Wrighten, S. A., Green, A. J., Wilson, S. P., et al. (2015). Hippocampal insulin resistance impairs spatial learning and synaptic plasticity. Diabetes 64, 3927–3936. doi: 10.2337/db15-0596
Hanson, J. L., Nacewicz, B. M., Sutterer, M. J., Cayo, A. A., Schaefer, S. M., Rudolph, K. D., et al. (2015). Behavioral problems after early life stress: contributions of the hippocampus and amygdala. Biol. Psychiatry 77, 314–323. doi: 10.1016/j.biopsych.2014.04.020
Humphreys, K. L., King, L. S., Sacchet, M. D., Camacho, M. C., Colich, N. L., Ordaz, S. J., et al. (2019). Evidence for a sensitive period in the effects of early life stress on hippocampal volume. Dev. Sci. 22:e12775. doi: 10.1111/desc.12775
Jett, J. D., Boley, A. M., Girotti, M., Shah, A., Lodge, D. J., and Morilak, D. A. (2015). Antidepressant-like cognitive and behavioral effects of acute ketamine administration associated with plasticity in the ventral hippocampus to medial prefrontal cortex pathway. Psychopharmacology 232, 3123–3133. doi: 10.1007/s00213-015-3957-3
Jonas, P., and Lisman, J. (2014). Structure, function, and plasticity of hippocampal dentate gyrus microcircuits. Front. Neural Circ. 8:107. doi: 10.3389/fncir.2014.00107
Kenna, H., Hoeft, F., Kelley, R., Wroolie, T., DeMuth, B., Reiss, A., et al. (2013). Fasting plasma insulin and the default mode network in women at risk for Alzheimer's disease. Neurobiol. Aging 34, 641–649. doi: 10.1016/j.neurobiolaging.2012.06.006
Lau, T., Bigio, B., Zelli, D., McEwen, B. S., and Nasca, C. (2017). Stress-induced structural plasticity of medial amygdala stellate neurons and rapid prevention by a candidate antidepressant. Mol. Psychiatry. 22, 227–234. doi: 10.1038/mp.2016.68
Leventhal, A. M., Waters, A. J., Kahler, C. W., Ray, L. A., and Sussman, S. (2009). Relations between anhedonia and smoking motivation. Nicotine Tob. Res. 11, 1047–1054.
Liu, J., Head, E., Gharib, A. M., Yuan, W., Ingersoll, R. T., Hagen, T. M., et al. (2002). Memory loss in old rats is associated with brain mitochondrial decay and RNA/DNA oxidation: partial reversal by feeding acetyl-L-carnitine and/or R-α-lipoic acid. Proc. Natl. Acad. Sci. U. S. A. 99, 2356–2361. doi: 10.1073/pnas.261709299
Lonsdale, J., Thomas, J., Salvatore, M., Phillips, R., Lo, E., Shad, S., et al. (2013). The Genotype-Tissue Expression (GTEx) project. Nat Genet. 45, 580–585. doi: 10.1038/ng.2653
Lorsch, Z. S., Ambesi-Impiombato, A., Zenowich, R., Morganstern, I., Leahy, E., Bansal, M., et al. (2021). Computational analysis of multidimensional behavioral alterations after chronic social defeat stress. Biol. Psychiatry 89, 920–928. doi: 10.1016/j.biopsych.2020.10.010
Mareckova, K., et al. (2018). Perinatal stress and human hippocampal volume: findings from typically developing young adults. Sci. Rep. 8:4696. doi: 10.1038/s41598-018-23046-6
McEwen, B. S. (2003). Early life influences on life-long patterns of behavior and health. Ment. Retard. Dev. Disabil. Res. Rev. 9, 149–154. doi: 10.1002/mrdd.10074
McEwen, B. S. (2018). Redefining neuroendocrinology: epigenetics of brain–body communication over the life course. Front. Neuroendocrinol. 49, 8–30. doi: 10.1016/j.yfrne.2017.11.001
McEwen, B. S., Bowles, N. P., Gray, J. D., Hill, M. N., Hunter, R. G., Karatsoreos, I. N., et al. (2015). Mechanisms of stress in the brain. Nat. Neurosci. 18, 1353–1363. doi: 10.1038/nn.4086
McEwen, B. S., Gray, J., and Nasca, C. (2015). Recognizing resilience: learning from the effects of stress on the brain. Neurobiol. Stress 1, 1–11. doi: 10.1016/j.ynstr.2014.09.001
McEwen, B. S., Nasca, C., and Gray, J. D. (2016). Stress effects on neuronal structure: hippocampus, amygdala, and prefrontal cortex. Neuropsychopharmacology 41, 3–23. doi: 10.1038/npp.2015.171
Meaney, M. J., Aitken, D. H., van Berkel, C., Bhatnagar, S., and Sapolsky, R. M. (1988). Effect of neonatal handling on age-related impairments associated with the hippocampus. Science 239, 766–768. doi: 10.1126/science.3340858
Nasca, C., Barnhill, O., DeAngelis, P., Watson, K., Lin, J., Beasley, J., et al. (2021). Multidimensional predictors of antidepressant responses: integrating mitochondrial, genetic, metabolic and environmental factors with clinical outcomes. Neurobiol Stress 15:100407. doi: 10.1016/j.ynstr.2021.100407
Nasca, C., Bigio, B., Lee, F. S., Young, S. P., Kautz, M. M., Albright, A., et al. (2018). Acetyl-L-carnitine deficiency in patients with major depressive disorder. Proc. Natl. Acad. Sci. U. S. A. 115, 8627–8632. doi: 10.1073/pnas.1801609115
Nasca, C., Bigio, B., Zelli, D., de Angelis, P., Lau, T., Okamoto, M., et al. (2017). Role of the Astroglial glutamate exchanger xCT in ventral Hippocampus in resilience to stress. Neuron 96, 402–413.e5. doi: 10.1016/j.neuron.2017.09.020
Nasca, C., Bigio, B., Zelli, D., Nicoletti, F., and McEwen, B. S. (2015). Mind the gap: glucocorticoids modulate hippocampal glutamate tone underlying individual differences in stress susceptibility. Mol. Psychiatry 20, 755–763. doi: 10.1038/mp.2014.96
Nasca, C., Dobbin, J., Bigio, B., Watson, K., de Angelis, P., Kautz, M., et al. (2020). Insulin receptor substrate in brain-enriched exosomes in subjects with major depression: on the path of creation of biosignatures of central insulin resistance. Mol. Psychiatry 26, 5140–5149. doi: 10.1038/s41380-020-0804-7
Nasca, C., Menard, C., Hodes, G., Bigio, B., Pena, C., Lorsch, Z., et al. (2019). Multidimensional predictors of susceptibility and resilience to social defeat stress. Biol. Psychiatry 86, 483–491. doi: 10.1016/j.biopsych.2019.06.030
Nasca, C., Xenos, D., Barone, Y., Caruso, A., Scaccianoce, S., Matrisciano, F., et al. (2013). L-acetylcarnitine causes rapid antidepressant effects through the epigenetic induction of mGlu2 receptors. Proc. Natl. Acad. Sci. U. S. A. 110, 4804–4809. doi: 10.1073/pnas.1216100110
Nemeroff, C. B. (2016). Paradise lost: the neurobiological and clinical consequences of child abuse and neglect. Neuron 89, 892–909. doi: 10.1016/j.neuron.2016.01.019
Nemeroff, C. B., Heim, C. M., Thase, M. E., Klein, D. N., Rush, A. J., Schatzberg, A. F., et al. (2003). Differential responses to psychotherapy versus pharmacotherapy in patients with chronic forms of major depression and childhood trauma. Proc. Natl. Acad. Sci. U. S. A. 100, 14293–14296. doi: 10.1073/pnas.2336126100
Nestler, E. J. (2014). Epigenetic mechanisms of depression. JAMA Psychiat. 71, 454–456. doi: 10.1001/jamapsychiatry.2013.4291
Parker, M. A., Weinberger, A. H., and Villanti, A. C. (2020). Quit ratios for cigarette smoking among individuals with opioid misuse and opioid use disorder in the United States. Drug Alcohol Depend 214:108164.
Pettegrew, J. W., Levine, J., and McClure, R. J. (2000). Acetyl-L-carnitine physical-chemical, metabolic, and therapeutic properties: relevance for its mode of action in Alzheimer's disease and geriatric depression. Mol. Psychiatry 5, 616–632. doi: 10.1038/sj.mp.4000805
Post, R. M. (2018). Myriad of implications of acetyl-L-carnitine deficits in depression. Proc. Natl. Acad. Sci. U. S. A. 115, 8475–8477. doi: 10.1073/pnas.1811389115
Price, L. H., Kao, H.-T., Burgers, D. E., Carpenter, L. L., and Tyrka, A. R. (2013). Telomeres and early-life stress: an overview. Biol. Psychiatry 73, 15–23. doi: 10.1016/j.biopsych.2012.06.025
Rasgon, N. L., and McEwen, B. S. (2016). Insulin resistance-a missing link no more. Mol. Psychiatry 21, 1648–1652. doi: 10.1038/mp.2016.162
Robison, A. J., and Nestler, E. J. (2011). Transcriptional and epigenetic mechanisms of addiction. Nat. Review. Neurosci. 12, 623–637.
Rotroff, D. M., Corum, D. G., Motsinger-Reif, A., Fiehn, O., Bottrel, N., Drevets, W. C., et al. (2016). Metabolomic signatures of drug response phenotypes for ketamine and esketamine in subjects with refractory major depressive disorder: new mechanistic insights for rapid acting antidepressants. Transl. Psychiatry 6:e894. doi: 10.1038/tp.2016.145
Roys, M., Weed, K., Carrigan, M., and MacKillop, J. (2016). Associations between nicotine dependence, anhedonia, urgency and smoking motives. Addict. Behav. 62, 145–151. doi: 10.1016/j.addbeh.2016.06.002
Russo, S. J., and Charney, D. S. (2013). Next generation antidepressants. Proc. Natl. Acad. Sci. U. S. A. 110, 4441–4442. doi: 10.1073/pnas.1301593110
Saleh, A., Potter, G. G., McQuoid, D. R., Boyd, B., Turner, R., MacFall, J. R., et al. (2017). Effects of early life stress on depression, cognitive performance, and brain morphology. Psychol. Med. 47, 171–181. doi: 10.1017/S0033291716002403
Shonkoff, J. P., Boyce, W. T., and McEwen, B. S. (2009). Neuroscience, molecular biology, and the childhood roots of health disparities: building a new framework for health promotion and disease prevention. JAMA 301, 2252–2259. doi: 10.1001/jama.2009.754
Short, A. K., and Baram, T. Z. (2019). Early-life adversity and neurological disease: age-old questions and novel answers. Nat. Rev. Neurol. 15, 657–669. doi: 10.1038/s41582-019-0246-5
Streck, J. M., Sigmon, S. C., Priest, J., Bergeria, C. L., Davis, D. R., Hughes, J. R., et al. (2020). Investigating tobacco withdrawal in response to reduced nicotine cigarettes among smokers with opioid use disorder and other vulnerabilities. Experimental Clinical Psychopharmacology.
Sweatt, J. D. (2010). Neuroscience. Epigenetics and cognitive aging. Science 328, 701–702. doi: 10.1126/science.1189968
Takahashi, H., Lassmann, T., Murata, M., and Carninci, P. (2012). 5’ end-centered expression profiling using cap-analysis gene expression and next-generation sequencing. Nat. Prot. 7, 542–561. doi: 10.1038/nprot.2012.005
Uhlen, M., Fagerberg, L., Hallstrom, B. M., Lindskog, C., Oksvold, P., Mardinoglu, A., et al. (2015). Tissue-based map of the human proteome. Science 347:1260419. doi: 10.1126/science.1260419
Watson, K., Nasca, C., Aasly, L., McEwen, B., and Rasgon, N. (2018). Insulin resistance, an unmasked culprit in depressive disorders: promises for interventions. Neuropharmacology 136, 327–334. doi: 10.1016/j.neuropharm.2017.11.038
Weaver, I. C., et al. (2004). Epigenetic programming by maternal behavior. Nat. Neurosci. 7, 847–854. doi: 10.1038/nn1276
WHO. Mental health and COVID-19: early evidence of the pandemic’s impact. Available at: https://www.who.int/publications/i/item/WHO-2019-nCoV-Sci_Brief-Mental_health-2022.1 (2022).
Williams, L. M., Debattista, C., Duchemin, A. M., Schatzberg, A. F., and Nemeroff, C. B. (2016). Childhood trauma predicts antidepressant response in adults with major depression: data from the randomized international study to predict optimized treatment for depression. Transl. Psychiatry 6:e799. doi: 10.1038/tp.2016.61
Keywords: hippocampus, acetyl-L-carnitine, glutamate, histone, mood disorders, depression, cognition, opioid use disorders
Citation: Bigio B, Sagi Y, Barnhill O, Dobbin J, El Shahawy O, de Angelis P and Nasca C (2023) Epigenetic embedding of childhood adversity: mitochondrial metabolism and neurobiology of stress-related CNS diseases. Front. Mol. Neurosci. 16:1183184. doi: 10.3389/fnmol.2023.1183184
Edited by:
Milena Cannella, Mediterranean Neurological Institute Neuromed (IRCCS), ItalyReviewed by:
Pawel Licznerski, Yale University, United StatesCopyright © 2023 Bigio, Sagi, Barnhill, Dobbin, El Shahawy, de Angelis and Nasca. This is an open-access article distributed under the terms of the Creative Commons Attribution License (CC BY). The use, distribution or reproduction in other forums is permitted, provided the original author(s) and the copyright owner(s) are credited and that the original publication in this journal is cited, in accordance with accepted academic practice. No use, distribution or reproduction is permitted which does not comply with these terms.
*Correspondence: Carla Nasca, carla.nasca@nyulangone.org