- 1Department of Biological and Life Sciences The Technical University of Kenya, Nairobi, Kenya
- 2Medical Research Council Unit The Gambia at London School of Hygiene and Tropical Medicine, Banjul, Gambia
- 3Biological Sciences Department, Kisii University, Kisii, Kenya
Background: COVID-19, caused by SARS-CoV-2, is a communicable respiratory viral disease with no effective curative medicine. The structure of SARS-CoV-2 spike protein has conserved modules that facilitate both infection and fusion into human host cells. These modules serve as crucial targets for synthesized and natural antivirals and therapeutics. In this study, our objective was to explore the potential of ethno-medicinal practice and molecular modelling of phytochemicals in informing drug targets against COVID-19 infections.
Methods: The study was conducted at four sites in western Kenya’s Lake Victoria basin. We used purposive sampling and snowballing to identify traditional health practitioners (THPs) and documented their experience and response to COVID-19. Extracts from various medicinal plants identified by the THP were subjected to phytochemical analysis on Gas Chromatography-Mass-spectrometry (GC-MS). The resulting active phytochemicals were screened against SARS-CoV-2 S-protein in silico using molecular modelling tools.
Results: Seventeen (17) THPs with experience in response to COVID-19 were identified, of whom 92% had more than 10 years of experience in the practice of traditional medicine. Whereas 43 medical plants were identified and documented, only seven plants, prescribed consistently by all THPs were investigated further. Phytochemical analysis on GC-MS identified compounds belonging to different chemical classes: terpenes, alkanes, alkene, alcohols, acridone, pyrazine, and carboxylic acid esters. Molecular modelling revealed the S-protein to possess multiple active sites. The phytochemicals studied showed potential multiple conformers interacting with each of the S-protein active sites.
Conclusion: Overall, we identified and modelled phytochemicals with the SARS-CoV-2 S-protein and explained their bioactivities as reported by traditional herbalists. The study underscores the beneficial contribution of traditional medicine in settings where access to comprehensive healthcare services for COVID-19 is limited. The identified plants and/or their phytochemicals could be targeted for conservation and future investigations including in-vivo studies targeting different aspects of the virus replication process, followed by the development of drugs with novel mode of action. The phytochemicals can also be screened for activities against other viruses in the Coronaviridae family.
1 Introduction
Coronavirus disease 2019 (COVID-19), caused by severe acute respiratory syndrome coronavirus 2 (SARS-CoV-2), reveals the potential of emerging infectious diseases to profoundly impact public health systems and significantly alter human economic and social wellbeing globally (Shi et al., 2021; WHO, 2024). Whilst a number of vaccines against COVID-19 have been produced, effective drugs that can prevent or halt disease progression is still considered necessary. At the height of COVID-19, many patients in sub-Saharan Africa (SSA) relied on herbal-based traditional medicine to manage the disease. A case example, is in Madagascar where use of herbal medicine, COVID-organics, was approved by the government even though its efficacy was not clinically proven (Atabong, 2020).
In many SSA countries, the use of herbal-based traditional medicines to boost immunity and/or serve as preventive measures against infections is not uncommon (UNESCO, 2020). The world Health Organization (WHO) estimates circa 80% of the people in underdeveloped nations to rely on traditional medicine (WHO, 2019). So far, over thousands of plant species with medicinal properties have been documented worldwide. The Plant Resources of Tropical Africa (PROTA) program has recorded 6,377 plant species used in Tropical Africa, of which more than 4,000 are used as medicinal plants (Bosch et al., 2002). These medicinal plants can serve as potential sources to discover new molecules for the treatment of future recurrent COVID-19. Nonetheless, with COVID-19, limited knowledge of ethno-medicine represents weak links to effective identification of new compounds against the disease. Indeed, few studies have empirically linked medicinal plants and/or their secondary metabolites to the prevention and treatment of COVID-19 (Adhikari et al., 2021). Recent studies have highlighted crucial phytochemicals screened for antiviral activities that could potentially be explored as therapeutic alternatives for SARS-CoV-2 (Marmitt et al., 2021). To identify leading compounds that may be used for the design and development of SARS-CoV-2 inhibitors, most studies have focused on screening via in vitro and in silico techniques of synthetic compounds belonging to different chemical groups (Nazir et al., 2024).
SARS-CoV-2 uses their membrane surface receptor binding proteins to infect human host cells, making them crucial drug and vaccine targets (Yan et al., 2020). The SARS-CoV-2 virion RNA genome codes four structural proteins - the membrane (M), spike (S), envelope (E), and nucleocapsid (N) proteins (Jafary et al.,2021). The S protein is initially synthesized as two subunits - amino (N)-terminal (S1) and carboxyl (C)-terminal (S2) regions, which exist in a non-covalent union until their cleavage to facilitate viral fusion into host membrane (Xu et al., 2021). During infection, the S1 subunit binds the human angiotensin converting enzyme (hACE-2) via its receptor binding domain (RBD) while S2 after cleavage fuses with the host cell membrane via two heptad motifs (Huang et al., 2020; Winger and Caspari, 2021). Reportedly, frequent mutations of the residues in the RBD regions of S1 alter how the viral S-protein interacts with the human host cells (Jafary et al., 2021; Xu et al., 2021). In fact, the RBD region is a critical but an elusive and highly mutable target for neutralizing antibodies against SARS viruses. In contrast, the S2 fusion peptides are highly conserved and therefore most stable targets for designing antiviral inhibitors (Huang et al., 2020).
The hACE-2, enzymatic receptors expressed on surface of many human cells, is the default ligand for the S-protein, mediating attachment and priming by transmembrane protease serine 2 (TMPRS2) to induce entry into the host cell (Huang et al., 2020; Saxena et al., 2020; Xu et al., 2021). Thus, the binding affinity of hACE-2 and S-protein is vital for the SARS-CoV-2 infection efficiency. However, hACE-2 is crucial in converting angiotensin II to angiotensin (1–7) thereby mitigating the negative effects of its precursor ACE, inducing anti-inflammatory burst and induction of vasodilations (Turner, 2015; Saxena et al., 2020). For these reasons, it is necessary to guard the functionality of hACE-2 against any extraneous molecules such as the antigenic SARS-CoV-2 S protein. Ultimately, stopping the S protein from accessing the hACE-2 and aiding entry into the host cells is the most viable approach to stop the infection and spread of COVID-19. Already, several anti-SARS-CoV-2 therapeutics including neutralizing antibodies, main protease inhibitors, fusion peptide inhibitors, and many vaccine types are under active research (Huang et al., 2020; Guan et al., 2021). Unfortunately, the approaches currently being used are lengthy, complex, and costly. With these in mind, we sought to identify from our ethno-medicinal traditional practitioners the active phytochemicals with propensity to binding onto the S-protein active binding sites acting as anti-S epitope targets using established in silico approaches. Here we present our findings.
2 Materials and methods
2.1 Study site
This study was conducted in western Kenya, which shares its border with Uganda to the West and Tanzania to the South. The climate in this region is generally tropical and the major economic activities are farming and fishing. The study area has a total flora of 1,969 angiosperm plants in the List of East African plants (Knox, 1996) and is in the K5 floristic region that falls within the Lake Victoria regional mosaic plant belt of Africa. The natural vegetation is of a graded type with relict tropical rain-forest, bush grassland (dominated by Themeda-Hyparrhenia) and wooded grassland vegetation of the Combretum-Dodonaea-Balanites-Acacia matrix. The region is rich in biodiversity and hosts indigenous forests that are accessible to the local community from where they obtain medicinal plants for the management of various ailments. The study sites included Ugenya, Alego, and Bondo in Siaya County and Rusinga Island in Homa Bay County (Figure 1).
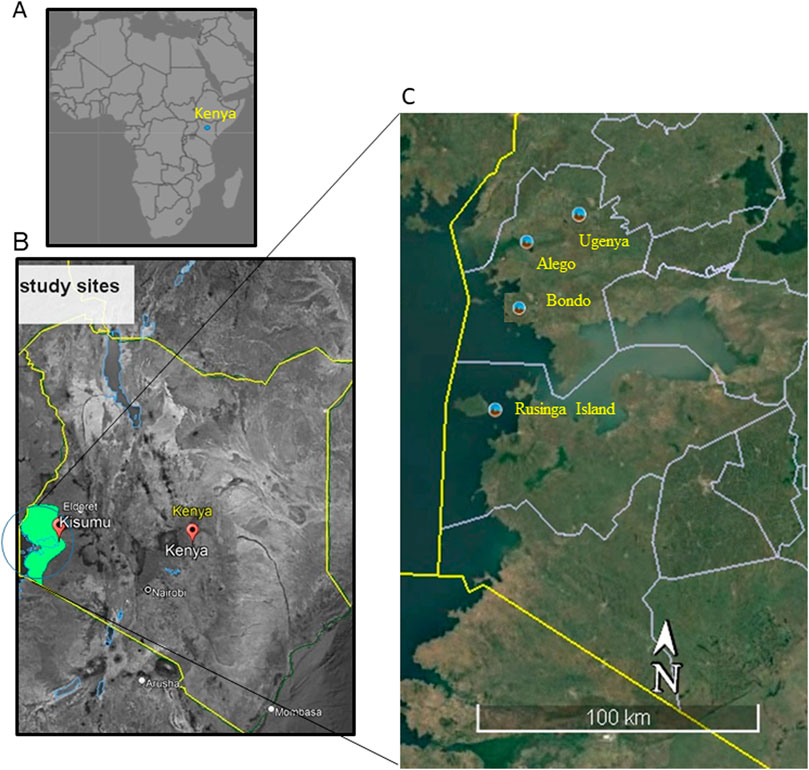
Figure 1. Map showing the study sites (A) Map of Africa (B) Map of Kenya (C) Map of the sample collection site. Each dot indicates the sampling site.
2.2 The plants and ethno-botanical data collection
The study was conducted in May and June of 2022. Purposive sampling and snowballing were used to identify the THPs and data collection was via face-to-face interview and observation. The following information were captured using a semi-structured questionnaire: (1) socio-demographic characteristics, (2) indigenous name of the plants used to treat COVID-19 associated symptoms, (3) plant part(s) used (i.e., leaves, stem roots, and root bark) (4), method of preparation and administration. The plants were photographed, samples collected, wrapped in newspaper, and transported to the laboratory at the Department of Biochemistry and Biotechnology of the Technical University of–Kenya (TU-K) for further identification and scientific naming by a botanist. Some plants had more than one local name and these documented names fit the ethno-systematic categories and criteria in Martin, with primary (unitary), secondary (binomial), and complex (polynomial) names recorded (Martin, 2010). Semantically, certain plant names are associated with symptoms of chest conditions. For instance, “Roko” signifies the sharp, rough, and penetrating pain typical of such ailments; “Ochol” suggests the sensation of straining or pushing also associated with chest or abdominal conditions; “Rachier” colloquially means to bring back or resuscitate from a weakening or fainting state similar to when someone is not breathing well. These names reflect what the medicinal plants relieve.
2.3 Sample preparation and phytochemical analysis using GC-MS
The samples were air dried at room temperature for 2 weeks and thereafter crushed into fine powder using an electric grinder (Model Retsch SM100, Germany). Twenty (20) mg of selected samples were extracted in 1 mL of dichloromethane then vortexed for 10 s and sonicated for 10 min. Homogenates were centrifuged at 14,000 rpm for 5 min and the supernatant filtered. The filtrate was then subjected to analysis using GC-MS. To generate the phytochemical profiles, 40 µL of each sample was placed in a GC vial insert and 2 µL of each sample analyzed on a 7890B gas chromatograph (Agilent Technologies, Inc., Santa Clara, CA, United States) coupled to a mass spectrometer (5977A series). The GC was equipped with an HP-5 MS column (30 m × 0.25 mm ID., 0.25 μm film thickness, Agilent, Palo Alto, California, United States). Helium served as the carrier gas at a flow rate of 1.2 mL per min. The oven temperature was programmed from 70°C and increased at 10°C/min to 285°C. The samples were each analyzed in duplicate. The plant compounds were identified based on mass spectra library data from Adams2.L, Chemecol.L, National Institute of Standards and Technology (NIST05a.L) and authentic standards analyzed in the same conditions were used for comparison.
2.4 Molecular modeling and docking
We used the profiled GC-MS phytochemicals to interrogate compound interactions with the S protein using molecular docking experiments. For this purpose, we chose terpenes based on reports that they are a diverse class of natural compounds with known antiviral activities (de Santana Souza et al., 2014). The interactions were assessed using binding energy values, which were evaluated based on binding energies of Arbidol-spike interaction (Singh et al., 2022). It is important to point out that Arbidol is a broad-spectrum antiviral used to manage COVID-19. The crystallographic structures of the phytochemicals were identified and downloaded from the NCBI PubChem database (https://pubchem.ncbi.nlm.nih.gov/). The 3-D structure of the SARS-CoV-2 S protein was modeled in silico using full length SARS-CoV-2 1273 amino acids downloaded from NCBI database (https://www.ncbi.nlm.nih.gov/protein/YP_009724390.1). The modeling was done using I-TASSER (Zhang, 2008; Roy et al., 2010; https://zhanggroup.org/I-TASSER/about.html). The optimized forms of the crystallographic structures of the phytochemicals were prepared using Open Babel chemical toolbox prior to docking experiments (O’Boyle et al., 2011). The structure of the S-protein was set as a rigid macromolecule while those of phytochemical ligands were set flexible. Subsequently, docking of the active phytochemicals against the S protein was done using AutoDock Vina version 1.2.0 (Eberhardt et al., 2021) found in PyRx version 0.9.2 (Dallakyan and Olson, 2015). The docked conformations were ranked based on the binding affinities thereafter, visualized using PyMOL version 3.0.4 (Shrödinger and DeLano, 2020). The interacting residues of receptor and atoms of ligands were analyzed using BIOVIA Discovery Studio Visualizer version 4.5 (Hossain et al., 2019).
2.5 Study ethics and research approvals
Ethical approval was obtained from Mount Kenya University Ethical Review Committee (Approval No. 1176). Additional research approval to conduct research was obtained from the National Commission for Science and Technology (License No. NACOSTI/P/22/16530; www.nacosti.go.ke) of Kenya. Written consent was obtained from all study participants. Confidentiality of data was maintained by omitting names and house locations and numbers during data analysis.
2.6 Statistical analysis
Data entry and statistical analysis were performed using IBM SPSS statistics for Windows version 21 (IBM Corp, 2013). Variables were described by use of descriptive statistics and presented using simple frequencies with percentages for quantitative variables. Differences in the chemical composition between plants were evaluated using Principal component analysis (PCA) and heat map performed in PAST v4.06b (Hammer et al., 2001). Random Forest Analysis (RFA) performed on random forest package in R software version 3.4.3 (R core development team; https://www.r-project.org/) was used to identify a minimum set of most important volatile phyto-compounds that are abundant and permanently present in the most prescribed plants. The relative abundance of each identified volatile organic compound following GC-MS analysis was used in the RFA and analysis were performed as previously described (Milugo et al., 2021).
3 Results
3.1 Socio-demographic characteristics and knowledge of COVID-19
Seventeen (17) THPs, majority of whom were females (77%) were identified (Figure 2A). Their age ranged between 45 and 87 years, median of 71 years and majority (59%) lacked formal educated (Figure 2B). Notably, 92% of the THPs had more than 10 years of experience in traditional medicine (Figure 2C). Regarding the source of knowledge on the practice of ethno-medicine, 90% had acquired the knowledge through filial inheritance while the rest through the community via observation (Figure 2D). Approximately two-thirds (67%) of the THPs sourced their medicinal herbs from the jungle, while the remaining proportion (33%) obtained plants from both the jungle and home gardens (domesticated) (Figure 2E). Symptoms associated with COVID-19 and treated using identified medicinal plants were identified as: difficulty in breathing, cough, fever, chest pains, throat pain, flue, sneezing and chills. Of these, the most reported symptoms were flu (18%), cough (23%), and breathing difficulties (20%) (Figure 2F).
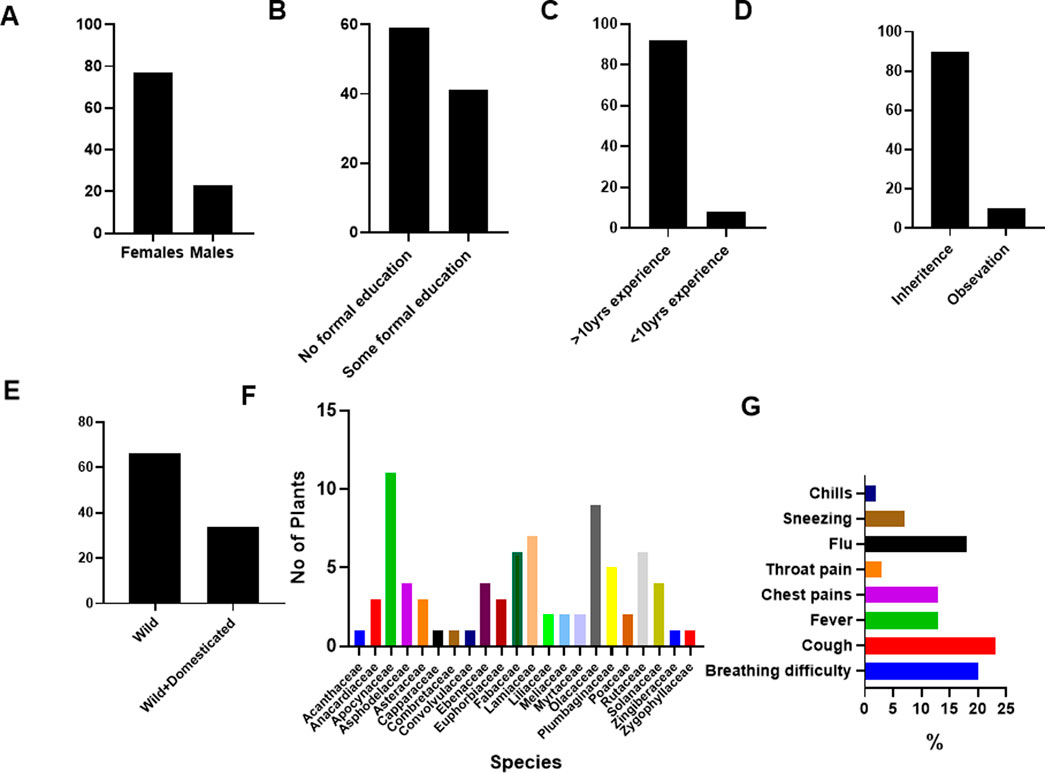
Figure 2. Study Population demographics and ethnomedicine then (a) Participant sex (b) Participant education level (c) Traditional health practitioner experience (d) Source of healing knowledge (e) Plant material source (f) Medicinal plant families (g) Symptoms treated with medicinal plants.
3.2 Medicinal plants used against COVID-19
In total, 43 medicinal plant species belonging to 22 angiosperm families were identified and documented (Supplementary Table S1; Figure 2G). The majority of these belonged to the family Apocynaceae, followed by Olacaceae and Lamiaceae (Figure 2G). The least prescribed plant species belonged to the family: Zingiberaceae, Zygophyllaceae, Capparaceae, Combretaceae, Convolvulaceae, and Acanthaceae (Figure 2G). Of the 43 medicinal plants, only seven were prescribed consistently by all the THPs (Table 1) and these include: Ximenia americana L. (Olacaceae), Euclea divinorum Hiern (Ebenaceae), Carissa spinarum L. (Apocynaceae), Aloe sp. (Asphodelaceae), Ocimum gratissimum L. (Lamiaceae), Withania somnifera (L.) Dunal (Solanaceae), and Plumbago zeylanica L. (Plumbaginaceae). The most preferred plant part utilized in the management of COVID-19 was roots whilst boiling for oral administration and fumigation represented the most used method for preparation of the cocktail used against COVID-19. Notably, showering was mainly prescribed when handling cases of severe fever (Table 1).
3.3 Phytochemical profiles of the most prescribed plants against COVID-19
Phytochemical analysis using GC-MS revealed the presence of compounds belonging to different chemical classes, namely: alkanes, alkyls, aldehydes, terpenes, alcohols, acridones, pyrazines, and esters (Figure 3A). Almost two-thirds of the identified compounds were either alkanes or terpenes (Figure 3A). Moreover, the compounds varied in quantity across different plant type as shown in Figure 3B. Notably, importance ranking of the compounds using Mean Decrease in Accuracy (MDA) as estimated by random forest analysis revealed 17 compounds, mostly alkanes and terpenes (Figure 3C). Further analysis using PCA showed the plants to vary based on their chemical composition further corroborating the findings from heat map (Figure 3D).
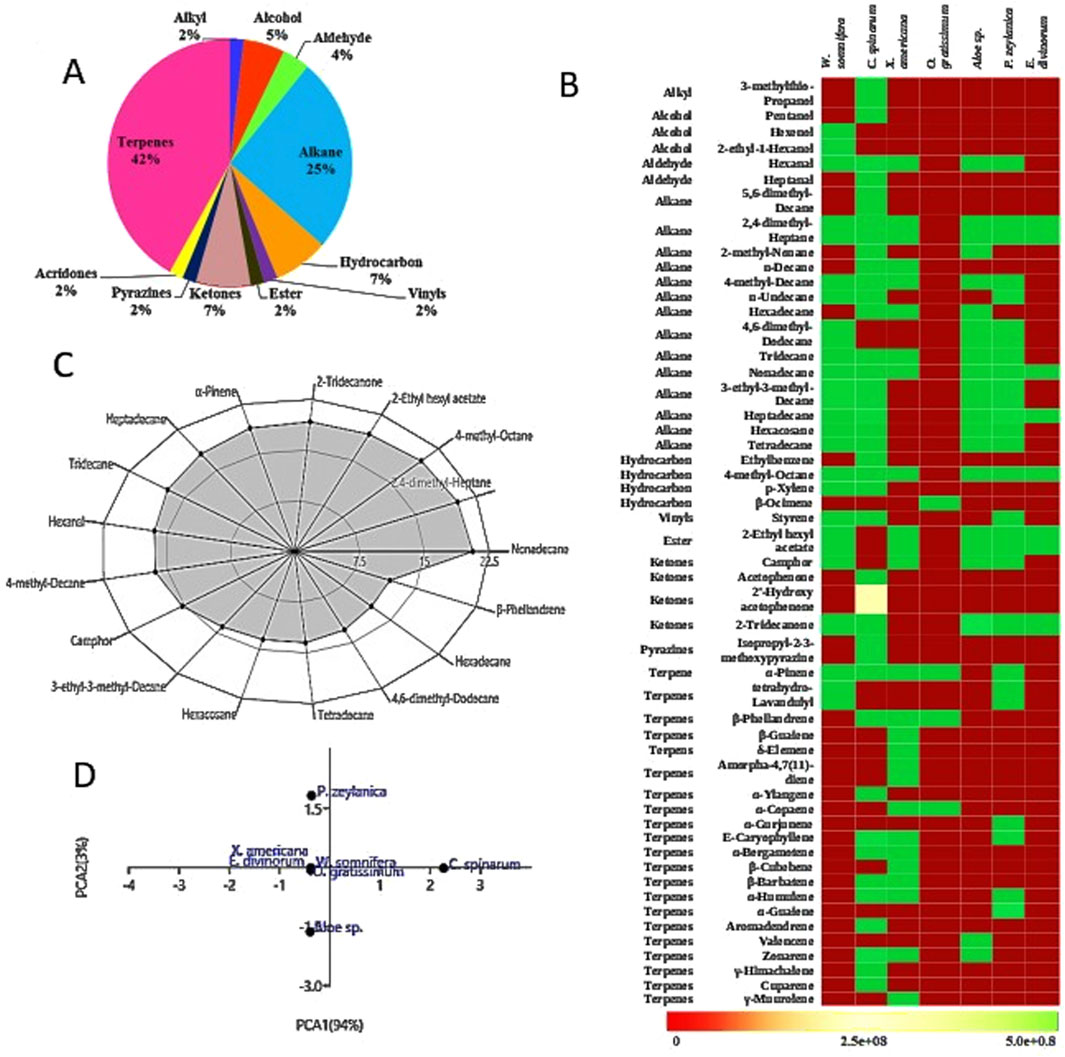
Figure 3. (A) Pie chart showing distribution of metabolites by class. Most compounds were alkanes and terpenes (B) Heat map showing distribution of metabolites across most commonly prescribed plants (C) Radar plots indicating most abundant and important compounds as projected by MDA (D) PCA plots of plants based on their VOCs. Plots were generated using the first two components (PC1 and PC2), PC1 explained 94% of the total variance while PC2 explained 3%.
3.4 Molecular modeling and docking experiments
From the GC-MS profiles, we investigated 24 terpenes and found them to bind to the S protein with high affinities, ranging between −6.0 and −8.3 kcal/mol, relative to Arbidol binding free energy of −5.9 kcal/mol (Singh et al., 2022; Supplementary Table S2). The S-protein possesses multiple active sites, within the major conserved domains S1 and S2.The S1 domain comprises the NTD, RBD, and the D614G site. The S2 domains start from the furin (S1/S2 cleavage), S′ cleavage site, FP, HR1/2, CH-CD, and TMD (Figure 4). Our docking experiments using the best conformers of each phytochemicals showed varied affinity for the RBD. The phytochemicals bound at different sites of the RBD subdomains, here designated as RBD 1 (which has receptor binding motive (RBM) 1 and 2), RBD2, 3, and 4 (Figure 5A). Within the range of 4.0 Å (Å), our results indicate that the studied terpenes interacted with specific key amino acids including LYS368, TYR459, TYR464, PHE458, and TYR456 of RBM1 at appreciable binding affinities (Figures 5B a–e). The interaction at RBM2 involved TYR418, LEU419, PRO438, and TYR450 (Figure 5B v). At the RBD2, collectively, the target residues include PHE340, TYR362, PHE392, PHE427, GLU473, and LEU474 (Figures 5B g–o). At RBD3, the residues are ASN475, ALA476, PHE517, and PHE519; and all of them utilize conventional hydrogen, pi-alkyl or alkyl interactions (Figures 5B p–s). The active residues at the RBD4, a binding site coinciding with the heparin sulfate (HS) site, another host membrane protein involved in host cell recognition in concert with the ACE-2 (Paiardi et al., 2022), are PHE310, ASN311, VAL334, LEU335, and PHE340 (Figures 5B t,u).
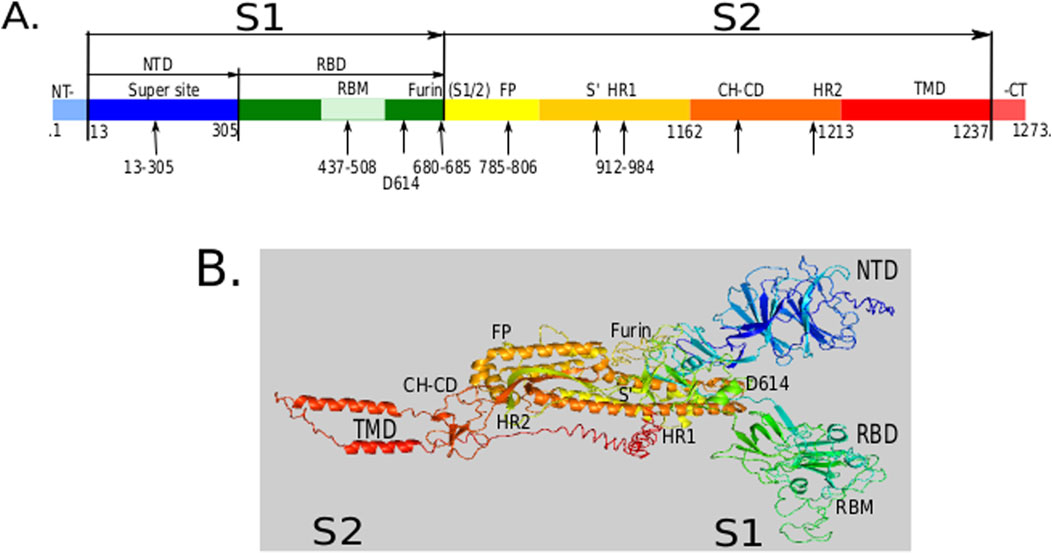
Figure 4. Spike protein 3-D model of key domains. (A) A schematic linear organization of the domains along a S-protein sequence of 1273 amino acids indicating the two major domains S1 and S2 with estimated amino acid positions of the various sub-domains. The S1 comprises signal peptide (SP) in the first 13 amino acids, the super site mutable N-terminal domain (NTD), and receptor binding domain (RBD). (B) The 3-D model of the S-protein with the domains mapped at their approximate regions: S1 comprising NTD, RBD and a region near the D614 mutable site; S2 comprising Furin catalytic site (S1/2 cleavage), fusion peptide (FP) with a second cleavage site S′, heptad repeat (HR) regions HR1 and HR2, the central helix (CH) and connector domains (CD), and the transmembrane domain (TMD). The arrows indicate approximate range of residues for the domains. The full length SARS-CoV-2 1273 amino acid sequence for the S-protein was downloaded from the NCBI (https://www.ncbi.nlm.nih.gov/protein/YP_009724390.1) and the 3-D structure modeled using I-TASSER tool. The model image was annotated using Inkscape version 1.3.2 (https://inkscape.org).
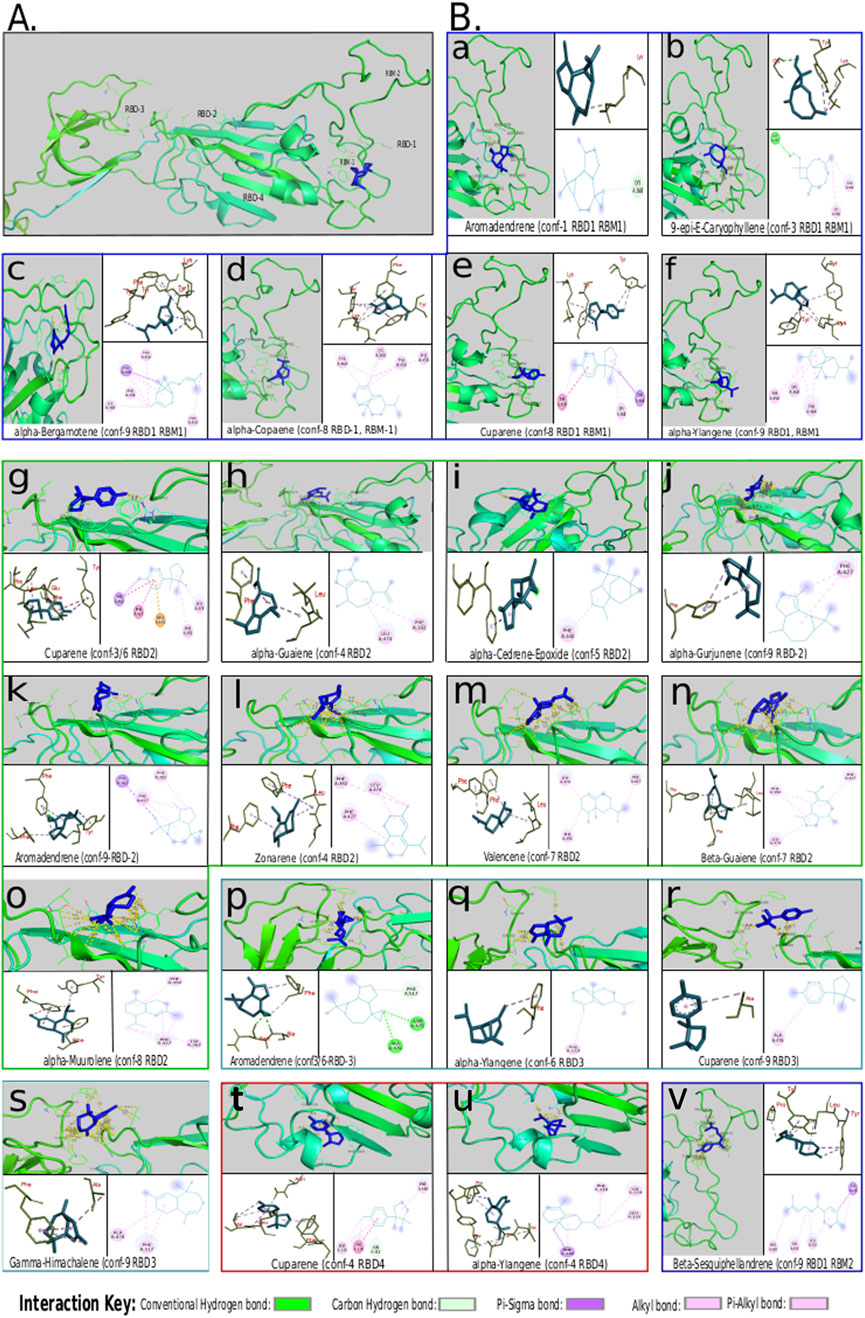
Figure 5. Phytochemicals interacting with SARS-CoV-2 S1 RBD. (A) The S-protein S1 RBD ligand binding sites: RBD1 (RBM1 and RBM2, the native binding sites for ACE-2), RBD2, RBD3, and RBD4. (B) The leading binding phytochemicals (ligands in blue color) onto the S1 RBD binding sites: (a–f) RBD1 RBM1 and (v) RBD1 RBM2 (blue outline) with the best binders as Aromadendrene, 9-epi-E-Caryophyllene, alpha-Bergamotene, alpha-Copaene, Cuparene, and alpha-Ylangene, the best ligand is beta-Sesquiphellandrene; (g–o) ligands onto RBD2 (green outline) are Cuparene, alpha-Guaiene, alpha-Cedrene-Epoxide, alpha-Gurjunene, Aromadendrene, Zonarene, Valencene, alpha-Muurolene, beta-Guaiene; (p–s) ligands onto RBD3 (cyan outline) include Aromadendrene, Cuparene, gamma-Himachalene, and alpha-Ylangene; and (t,u) ligands to RBD4 (red outline) are Cuparene and alpha-Ylangene. The amino acid interactions were modeled using AutoVina and visualized using PyMol program (Shrödinger and DeLano, 2020). The Interacting amino acid atoms were analyzed using Biovia Discovery Studio Visualizer.
4 Discussion
Documenting medicinal plants used in management of COVID-19 not only conserves traditional knowledge but may also unravel novel bioactive compounds for the management of emerging and neglected diseases of concern globally. The concerns ensue on the backdrop of increasing drug resistance against currently available therapeutics and expensive and lengthy process of developing new drugs and vaccines. Also concerning is the insistent burden of prolonged post viral syndrome (also long-COVID) and acute COVID-19 complications often characterized by inflammation, lung fibrosis, overreaction of the immune system and cardiovascular anomalies (Desai et al., 2022; CDC, 2025; John et al., 2021). In ethno-medicine, plants that have anti-inflammatory and immune modulation activity are often exploited to manage inflammatory complications and restore physiological balance: Such plants, may offer valuable complementary strategy for management of inflammatory complications of the respiratory system induced by COVID-19, and potentially, lower mortality (Al-Jamal et al., 2024). Indeed, there has been unprecedented call and advocacy for enhanced research on herbal remedies by many governments and global organizations such as the WHO, perhaps due to their effectiveness in relieving other respiratory infections (World Health Organization (WHO, 2002). Such calls and advocacies, however, can only be taken up when there is evidence-based research that validates the bio-activity and safety profiles of a diversity of medicinal plants. A recent clinical trial using a herbal medicines used in Korean medicine to treat long COVID symptoms reveals the feasibility of ethno-medicine in management of the disease (Kim et al., 2024). In the current study we analyzed the biochemical profiles of plants used in western Kenya ethno-medicine to manage COVID-19-associated symptoms and identified leading compounds of importance that could be investigated further in laboratory experiments and clinical trials.
Overall, we identified 17 THPs and to ensure authenticity of the data set, we narrowed down our investigations to seven plants that were consistently prescribed by all the THPs. Thus, we are convinced that the selection of these seven plants is accurate, and even with further investigation, this pattern would not have changed. The participants correctly identified COVID-19 symptoms as having a cough, fever, breathing difficulty, and flu-like. Possibly, by administering herbal medicine aimed at relieving or treating these symptoms they might have prevented the clinical presentation and progress of COVID-19. The majority of the THPs sourced their plant materials from the wild and only a few domesticate the plants. This indicates a need to tap into the knowledge and conservation of ecological biodiversity particularly for the plants of medicinal value. The participants further reported frequent use of plant roots and leaves. Whilst plant roots are generally rich in bio-active ingredients, their indiscriminate use poses a threat to flora conservation efforts, especially for wild-crafted materia medica. Precautionary, the THPs should be encouraged to plant vulnerable medicinal species for conservation and sustainable use. With reference to the route of administration, the most used was oral route followed by fumigation (inhalation). This finding was comparable with a study conducted in Ethiopia where similarly oral route was the frequently used route of administration for respiratory illness (Umeta-Chali et al., 2021).
The most commonly used medicinal plants were: Ximenia americana L. (Olacaceae), Euclea divinorum Hiern (Ebenaceae), Carissa spinarum L. (Apocynaceae), Aloe sp. (Asphodelaceae), Ocimum gratissimum L. (Lamiaceae), W. somnifera (L.) Dunal (Solanaceae) and Plumbago zeylanica L. (Plumbaginaceae). The plants were shown to cluster differently based on the classes of the compounds they contain: implying that perhaps their bioactivity against the virus would also vary significantly. Notably, the majority of the compounds were terpenes and alkanes, which also varied in their level of importance and abundance. Nonetheless the current results support some previous studies where plants such as Allium sativum and Zingiber officinale, commonly used as spices, were reported to have bioactivities against COVID-19 symptoms (Khadka et al., 2021; Villena-Tejada et al., 2021; Yashvardhini et al., 2021). Besides, some plants such as Ocimum species, also identified in the current study, are known to contain compounds such as tulsinol (A, B, C, D, E, F, G) and dihydrodieuginol that possess immunomodulatory activities and can potentially inhibit replication of coronavirus (Varshney et al., 2020; Khadka et al., 2021). Another plant that has previously been reported to possess compound with inhibitory roles on SARS-CoV-2 main protease is Aloe vera (Mpiana et al., 2020). The compounds in this species were identified using molecular docking as (feralolide, 9-dihydroxyl-2-O-(z)-cinnamoyl-7-methoxy-Aloesin and Aloeresin) (Mpiana et al., 2020). A detailed review of antiviral activity of compounds from Aloe sp. can be found in (Españo et al., 2022). Additionally, the current findings also align with studies that have shown Withania somnifera to have some activity against COVID-19 (Daneshvar et al., 2021; Parihar, 2022; Shree et al., 2022; Singh et al., 2022). Thus the finding of the current study provides a basis to explore the bio-activity of the seven plants and possibly identify active ingredients for use in formulation of anti-COVID drug. Such investigations will generate scientific evidence to support the application of medicinal plants for COVID-19 treatment. Notably, some of the medicinal plants reported like W. somnifera, are extensively used in other traditional pharmacopoeia.
Phytochemical analysis using GC-MS identified compounds belonging to different chemical classes of which terpenes (Cox-Georgian et al., 2019; Nadjib, 2020; Al-Salihi and Alberti, 2021; Diniz et al., 2021), pyrazines (Seliem et al., 2021) and acridones (Oderinlo et al., 2022) have been shown to have antiviral activities. For instance, the acridone-based alkaloid 5-hydroxynoracronycine alcohol was found to have activity against SARs-CoV-2 main protease (Oderinlo et al., 2022). Among the compounds identified in the current study through GC-MS, to our knowledge, none have previously been documented as having been screened for activity against SARs-CoV-2. Nonetheless, some of the compounds, mainly the terpenes, have undergone evaluation for various biological activities. For example, the monoterpene α-pinene was reported to have a wide range of pharmacological activities, including antibiotic resistance modulation, anticoagulant, antitumor, antimicrobial, antimalarial, antioxidant, anti-inflammatory, anti-Leishmania, and analgesic effects (reviewed in Salehi et al., 2019; Allenspach and Steuer, 2021). Specifically, α-pinene exhibits anti-inflammatory activity by suppressing mitogen-activated protein kinase (MAPKs) and the nuclear factor kappa B (NF-κB) pathways, thus making it a promising agent for the treatment of various inflammatory diseases (Park et al., 2021). Moreover, α-pinene was found to enhance cytotoxicity of natural killer (NK) cells, suggesting that α-pinene can play a role in alleviating viral infection (Park et al., 2021). This is particularly significant given that the possibilities of using NK cell-based immunotherapy in treating COVID-19 has previously been suggested. Similarly, β-phellandrene and camphor have also been shown to have anti-microbial and anti-inflammatory, and activation of NK cells activities (da Silva et al., 2015; Thangaleela et al., 2022).
Among sesquiterpenes, δ-elemene, E-caryophyllene and α-humulene (or α- caryophyllene), aromadendrene, and valencene have also been reported to exhibit anti-inflammatory effects (Fernandes et al., 2007; Francomano et al., 2019; Maffei, 2020; Tan et al., 2021; Dantas et al., 2022; Viveiros et al., 2022; Dalavaye et al., 2024; Yan et al., 2024). The δ-elemene was reported to regulates inflammatory factors such as Tumor Necrosis Factor Alpha (TNF-α), Interferon (IFN), transforming growth factor Beta (TGF-β) and interleukin-6/10 (IL-6/10) (Tan et al., 2021). Likewise, E-caryophyllene and α-humulene exert anti-inflammatory activities by inhibiting inflammatory mediators such as inducible nitric oxide synthase (iNOS), IL-1β, IL-6, TNF-α, NF-κB, cyclooxygenase 1 (COX-1), and COX-2, thus revealing their potential in treating inflammatory conditions (Fernandes et al., 2007; Fidyt et al., 2016; Francomano et al., 2019; Viveiros et al., 2022). Recently, valencene was found to be therapeutically effective against acute and chronic inflammations in murine models. In silico analysis of valencene activity revealed its ability to inhibit COX-2 activity and histamine H1 receptor antagonism, as potential mechanisms by which valencene modulates acute inflammations (Dantas et al., 2022).
The S1 RBD of the S-protein has attracted the most research attention as a target for designing antiviral therapeutics, diagnostics, and vaccines; and apparently, this is the native binding site for the hACE-2 (Huang et al., 2020; Al-Salihi and Alberti, 2021; Pal et al., 2022; Santos et al., 2022; Mushebenge et al., 2023). We observed that the S-protein possesses a conserved structural organization with multiple the key domains and subdomains (Figure 4). From our study, the pertinent ligand binding sites on the S1 RBD are crucial in determining the bio-activity of the plant compounds (Figure 5). The temporal binding of these phytochemicals onto the respective binding sites likely constraint the conformational dynamics of the viral protein. The S1 RBD RBM is a natural binding site for hACE-2 to facilitate viral infectivity and pathogenicity (Choi et al., 2021; Mushebenge et al., 2023). We reveal that the active residues at RBM locate at two specific regions of S1 RBD1, labeled RBM1 and RBM2 (Figure 5A) at which the phytochemicals interact with key residues that fall within the vicinity of previously reported residue contacts between the viral S1 and the hACE-2 ligand (Zhang et al., 2021; Singh et al., 2022). Notably, while the hACE-2 is a large receptor protein that interacts mostly with only the exposed surface residues within the RBM, the phytochemicials are small molecules and can penetrate to inner residues within the binding pockets. This might explain why our results indicate a slight deviation of S-protein-hACE-2 interacting residues from those reported previously (Zhang et al., 2021). The other active site for the phytochemicals is the highly mutable C-terminal domain of the RBD, labeled D614 (Figure 5A). Mutation at the site D614G was previously associated with emergence of highly transmissible and virulent SARS-CoV-2 Omicron strain. Since the onset of the COVID-19 pandemic, the Omicron strain accumulated the most mutations on the S-protein bio-active sites, thus enhancing its infectivity and inactivity against available therapeutics (Guan et al., 2021; Mushebenge et al., 2023). Here, we observed the phytochemicals binding residues around the D614G site, none of them interacting directly with the residue. Speculatively, the interaction of these phytochemicals with their respective binding sites within S1 RBD may aid in altering the transitioning between the ‘down’ and ‘up’ conformations and also interfering with the ionic interactions between the S-protein and native ACE-2 (Zhang et al., 2021). These findings reveal multiple potential sites that the small plant derived metabolites bind onto on the SARS-CoV-2 S-protein. Hopefully, these interactions may retard bio-activities the S1 RBD with its cognate hACE-2 receptors. Potentially, a limitation of the current study is the fact that most of the plants were formulated in combination and we were not able to capture this information comprehensively as most of the participants were not willing to reveal their strategies for fear of losing their trade value. Additionally, it was not possible to standardize the collected plant materials based on other important variables such as age of plants and environmental conditions. Moreover, no in-vivo or in-vitro bioassays were conducted to verify whether the identified phytochemicals can indeed inhibit COVID-19 infection in humans.
Future prospects should aim to determine the antiviral activity of the compounds identified in the current study to position them as leads for further uptake in drug design and development. Future research should concentrate on screening plants from the angiosperm families; Apocynaceae, Olacaceae, and Lamiaceae to identify compounds that target various stages of the COVID-19 virus. This challenge can be addressed by employing advanced biotechnological methods. For example, cell bioengineering can be leveraged to enhance the production of plant-based compounds, while synthetic chemistry can facilitate large-scale production and optimize their potency profiles.
5 Conclusion
In conclusion, we identified and documented 43 medical plants used in ethno-medicine against COVID-19 around the Lake Victoria region in western Kenya. Also identified, through GC-MS analysis, were 55 compounds belonging to the chemical classes: terpenes, alkanes, alkene, alcohols, acridone, pyrazine, and carboxylic acid esters. Molecular modelling revealed the S-protein to possess multiple active sites, and the best binders were identified as aromadendrene, 9-epi-E-caryophyllene, alpha-bergamotene, alpha-copaene, cuparene, and alpha-ylangene. Overall, our findings contribute to the preservation of cultural knowledge relating to traditional herbal medicine, bolster confidence in herbal medicine, and encourage the conservation of medicinal plants within the study region. The study provides a basis for pharmaceutical industries and researchers to look into the effectiveness and safety of the seven medicinal plants identified in the present study.
Data availability statement
The datasets presented in this study can be found in online repositories. The names of the repository/repositories and accession number(s) can be found in the article/Supplementary Material.
Ethics statement
The studies involving humans were approved by Mount Kenya University Ethical Review Committee (Approval No. 1176). Additional research approval to conduct research was obtained from the National Commission for Science and Technology (License No. NACOSTI/P/22/16530; www.nacosti.go.ke) of Kenya. The studies were conducted in accordance with the local legislation and institutional requirements. The participants provided their written informed consent to participate in this study.
Author contributions
TM: Writing – original draft, Formal Analysis, Methodology, Data curation, Validation, Resources, Project administration, Investigation, Software, Supervision, Writing – review and editing, Funding acquisition, Conceptualization. BO: Writing – original draft, Formal Analysis, Methodology, Investigation, Writing – review and editing, Data curation. PO: Validation, Writing – review and editing, Methodology, Writing – original draft, Investigation. KC: Validation, Data curation, Writing – original draft, Visualization, Writing – review and editing, Formal Analysis, Software. GO: Supervision, Writing – review and editing, Software, Investigation, Methodology, Formal Analysis, Visualization, Writing – original draft, Data curation, Validation.
Funding
The author(s) declare that financial support was received for the research and/or publication of this article. We gratefully acknowledge the financial support for this research by the following organizations and agencies: Royal Society of Tropical Medicine and Hygiene (RSTMH Grant Number: 20210824) and Mawazo Institute and its partners (Grant Number: 2022-2-08).
Acknowledgments
This work greatly profited from the invaluable knowledge and rich experience of THPs from Homa Bay and Siaya counties of the Republic of Kenya; they are the rightful owners of the indigenous knowledge presented in this paper and are partakers of any benefits accruing from this work. We in particular acknowledge with gratitude the time and logistical guidance provided by Kinaga Ogeno and Charles Ogola during the Rusinga Island expedition. The authors thank Andrew Kasiti TU-K and Xavier Cheseto (icipe) for their technical assistance.
Conflict of interest
The authors declare that the research was conducted in the absence of any commercial or financial relationships that could be construed as a potential conflict of interest.
Generative AI statement
The author(s) declare that no Generative AI was used in the creation of this manuscript.
Publisher’s note
All claims expressed in this article are solely those of the authors and do not necessarily represent those of their affiliated organizations, or those of the publisher, the editors and the reviewers. Any product that may be evaluated in this article, or claim that may be made by its manufacturer, is not guaranteed or endorsed by the publisher.
Author disclaimer
The views expressed herein do not necessarily reflect the official opinion of the donors.
Supplementary material
The Supplementary Material for this article can be found online at: https://www.frontiersin.org/articles/10.3389/fntpr.2025.1597609/full#supplementary-material
SUPPLEMENTARY MATERIAL | List of plants used in traditional medicine to treat COVID-19 associated symptoms.
References
Adhikari, B., Marasini, B. P., Rayamajhee, B., Bhattarai, B. R., Lamichhane, G., Khadayat, K., et al. (2021). Potential roles of medicinal plants for the treatment of viral diseases focusing on COVID-19: a review. Phytotherapy Res. 35 (3), 1298–1312. doi:10.1002/ptr.6893
Al-Jamal, H., Idriss, S., Roufayel, R., Abi Khattar, Z., Fajloun, Z., and Sabatier, J. M. (2024). Treating COVID-19 with medicinal plants: is it even conceivable? A comprehensive review. Viruses 16 (3), 320. doi:10.3390/v16030320
Allenspach, M., and Steuer, C. (2021). α-Pinene: a never-ending story. Phytochemistry 190 (112857), 112857–7. doi:10.1016/j.phytochem.2021.112857
Al-Salihi, A. A. S., and Alberti, F. (2021). Naturally occurring terpenes: a promising class of organic molecules to address influenza pandemics. Nat. Prod. Bioprospecting. 11, 405–419. doi:10.1007/s13659-021-00306-z
Atabong, A. B. (2020). Essay series: african journalism and media in the time of covid-19: how pan-african media helped Madagascar advance its claim of a covid-19 ‘miracle cure’ as a form of medical diplomacy. Available online at: https://media.africaportal.org/documents/202012-03_AP-WITS_EssaySeries_Atabong_PROOF-3_002.pdf (Accessed February 17, 2021).
Bosch, C. H., Siemonsma, J. S., Lemmens, R. H. M. J., and Oyen, L. P. A. (2002). Plants Resources of Tropical Africa:/Ressources Vegetales de l’Afrique Tropicale. Basic list of species and commodity grouping, 341. Wageningen, Netherlands: PROTA Programme. Available online at: https://edepot.wur.nl/417519 (Accessed April 10, 2025).
CDC (2025). Signs and symptoms of long COVID, COVID-19. Available online at: https://www.cdc.gov/covid/long-term-effects/long-covid-signs-symptoms.html (Accessed April 10, 2025).
Choi, Y. K., Yiwei Cao, Y., Frank, M., Woo, H., Park, S., Yeom, M. S., et al. (2021). Structure, dynamics, receptor binding, and antibody binding of the fully glycosylated full-length SARS-CoV-2 spike protein in a viral membrane. J. Chem. Theory Comput. 17, 2479–2487. doi:10.1021/acs.jctc.0c01144
Cox-Georgian, D., Ramadoss, N., Dona, C., and Basu, C. (2019). “Therapeutic and medicinal uses of terpenes,” in Medicinal plants. Editors N. Joshee, S. Dhekney, and P. Parajuli (Cham: Springer), 12, 333–359.
Dalavaye, N., Nicholas, M., Pillai, M., Erridge, S., and Sodergren, M. H. (2024). The clinical translation of α-humulene - a scoping review. Planta Med. 90 (9), 664–674. doi:10.1055/a-2307-8183
Dallakyan, S., and Olson, A. J. (2015). Small-molecule library screening by docking with PyRx. Methods Mol. Biol. 1263, 243–250. doi:10.1007/978-1-4939-2269-7_19
Daneshvar, M., Heidari-Soureshjani, R., Zakerimoghadam, M., Mortezanasab, M., and Aloweiwi, W. (2021). Withania somnifera and COVID-19: current evidence and future prospective. Future Nat. Prod. Herb. Med. Winter Spring 7 (1), 24–47.
Dantas, L. B. R., Alcântara, I. S., Júnior, C. P. S., de Oliveira, M. R. C., Martins, A. O. B. P. B., Dantas, T. M., et al. (2022). In vivo and in silico anti-inflammatory properties of the sesquiterpene valencene. Biomed. and Pharmacother. 153, 113478. doi:10.1016/j.biopha.2022.113478
da Silva, E. T., da Silva Araújo, A., Moraes, A. M., de Souza, L. A., Silva Lourenço, M. C., de Souza, M. V., et al. (2015). Synthesis and biological activities of camphor hydrazone and imine derivatives. Sci. Pharm. 84 (3), 467–483. doi:10.3390/scipharm84030467
Desai, A. D., Lavelle, M., Boursiquot, B. C., and Wan, E. Y. (2022). Long-term complications of COVID-19. Am. J. Physiology - Cell Physiology 322 (1), 1–11. doi:10.1152/ajpcell.00375.2021
de Santana Souza, M. T., Almeida, J. R., Araujo, A. A., Duarte, M. C., Gelain, D. P., Moreira, J. C., et al. (2014). Structure–activity relationship of terpenes with anti-inflammatory profile – a systematic review. Basic and Clin. Pharmacol. and Toxicol. 115 (3), 244–256. doi:10.1111/bcpt.12221
Diniz, L. R. L., Perez-Castillo, Y., Elshabrawy, H. A., Filho, C. D. S. M. B., and de Sousa, D. P. (2021). Bioactive terpenes and their derivatives as potential SARS-CoV-2 proteases inhibitors from molecular modeling studies. Biomolecules 11 (1), 74. doi:10.3390/biom11010074
Eberhardt, J., Santos-Martins, D., Tillack, A. F., and Forli, S. (2021). AutoDock Vina 1.2.0: new docking methods, expanded force field, and Python bindings. J. Chem. Inf. Model. 61 (8), 3891–3898. doi:10.1021/acs.jcim.1c00203
Españo, E., Kim, J., and Kim, J.-K. (2022). Utilization of Aloe compounds in combatting viral diseases. Pharmaceuticals 15 (5), 599. doi:10.3390/ph15050599
Fernandes, E. S., Passos, G., Medeiros, R., da Cunha, F., Ferreira, J., Campos, M., et al. (2007). Anti-inflammatory effects of compounds alpha-humulene and (−)-trans-caryophyllene isolated from the essential oil of Cordia verbenacea. Eur. J. Pharmacol. 569, 228–236. doi:10.1016/j.ejphar.2007.04.059
Fidyt, K., Fiedorowicz, A., Strządała, L., and Szumny, A. (2016). β-caryophyllene and β-caryophyllene oxide-natural compounds of anticancer and analgesic properties. Cancer Med. 5 (10), 3007–3017. doi:10.1002/cam4.816
Francomano, F., Caruso, A., Barbarossa, A., Fazio, A., La Torre, C., Ceramella, J., et al. (2019). β-Caryophyllene: a sesquiterpene with countless biological properties. Appl. Sci. 9 (24), 5420. doi:10.3390/app9245420
Guan, X., Yang, Y., and Du, L. (2021). Advances in SARS-CoV-2 receptor-binding domain-based COVID-19 vaccines. Expert Rev. Vaccines. 22 (1), 422–439. doi:10.1080/14760584.2023.2211153
Hammer, Ø., Harper, D. A. T., and Ryan, P. D. (2001). PAST: paleontological Statistics software package for education and data analysis. Palaeont. Electr. 4 (1), 9. Available online at: http://palaeo-electronica.org/2001_1/past/issue1_01.htm.
Hossain, S., Sarkar, B., Prottoy, N. I., Araf, Y., Taniya, M. A., and Ullah, A. (2019). Thrombolytic activity, drug likeness property and ADME/T analysis of isolated phytochemicals from ginger (zingiber officinale) using in silico approaches. Mod. Res. Inflamm. 8 (3). doi:10.4236/mri.2019.83003
Huang, Y. F., Bai, C., He, F., Xie, Y., and Zhou, H. (2020). Review on the potential action mechanisms of Chinese medicines in treating coronavirus disease 2019 (COVID-19). Pharmacol. Res. 158, 104939. doi:10.1016/j.phrs.2020.104939
Jafary, F., Jafari, S., and Ganjalikhany, M. R. (2021). In silico investigation of critical binding pattern in SARS-CoV-2 spike protein with angiotensin-converting enzyme 2. Sci. Rep. 11 (1), 6927. doi:10.1038/s41598-021-86380-2
John, K. J., Nayar, J., Mishra, A. K., Selvaraj, V., Khan, M. S., and Lal, A. (2021). In-hospital clinical complications of COVID-19: a brief overview. Future Virol. 16 (11), 717–723. doi:10.2217/fvl-2021-0200
Khadka, D., Dhamala, M. K., Li, F., Aryal, P. C., Magar, P. R., Bhatta, S., et al. (2021). The use of medicinal plants to prevent COVID-19 in Nepal. J. Ethnobiol. Ethnomed 17 (261), 26–27. doi:10.1186/s13002-021-00449-w
Kim, T. H., Yoon, J., Kim, S., Kang, B. K., Kang, J. W., and Kwon, S. (2024). Herbal medicines for long COVID: a phase 2 pilot clinical study. Heliyon 10 (18), e37920. doi:10.1016/j.heliyon.2024.e37920
Knox, E. (1996). List of East African plants, 329. Nairobi: National Museums of Kenya. Available online at: https://museums.or.ke/botany-department/ (Accessed April 7, 2025).
Maffei, M. E. (2020). Plant natural sources of the endocannabinoid (E)-β-Caryophyllene: a systematic quantitative analysis of published literature. Int. J. Mol. Sci. 21 (18), 6540. doi:10.3390/ijms21186540
Marmitt, D. J., Goettert, M. I., and Rempel, C. (2021). Compounds of plants with activity against SARS-CoV-2 targets. Expert Rev. Clin. Pharmacol. 14 (5), 623–633. doi:10.1080/17512433.2021.1903317
Milugo, T. K., Tchouassi, D. P., Kavishe, R. A., Dinglasan, R. R., and Torto, B. (2021). Root exudate chemical cues of an invasive plant modulate oviposition behavior and survivorship of a malaria mosquito vector. Sci. Rep. 11, 14785. doi:10.1038/s41598-021-94043-5
Mpiana, P. T., Ngbolua, K. T., Tshibangu, D. S. T., Kilembe, J. T., Gbolo, B. Z., Mwanangombo, D. T., et al. (2020). Identification of potential inhibitors of SARS-CoV-2 main protease from Aloe vera compounds: a molecular docking study. Chem. Phys. Lett. 754, 137751. doi:10.1016/j.cplett.2020.137751
Mushebenge, A. G., Ugbaja, S. C., Mbatha, N. A., Khan, R. B., and Kumalo, H. M. (2023). A comprehensive analysis of structural and functional changes induced by SARS-CoV-2 spike protein mutations. COVID 3 (9), 1454–1472. doi:10.3390/covid3090100
Nadjib, B. M. (2020). Effective antiviral activity of essential oils and their characteristic terpenes against coronaviruses: an update. J. Pharmacol. Clin. Toxicol. 8 (1), 1138.
Nazir, M. S., Ahmad, M., Aslam, S., Rafiq, A., Al-Hussain, S. A., and Zaki, M. E. A. (2024). A comprehensive update of anti-COVID-19 activity of heterocyclic compounds. Drug Des. Devel Ther. 18, 1547–1571. doi:10.2147/DDDT.S450499
O'Boyle, N. M., Banck, M., James, C. A., Morley, C., Vandermeersch, T., and Hutchison, G. R. (2011). Open Babel: an open chemical toolbox. J. Cheminf. 3, 33. doi:10.1186/1758-2946-3-3
Oderinlo, O. O., Iwegbulam, C. G., Ekweli, O. A., Alawode, T. T., and Oyeneyin, O. E. (2022). Acridone alkaloids: in- silico investigation against SARS-CoV-2 main protease. Chem. Afr. 5, 1441–1450. doi:10.1007/s42250-022-00440-2
Paiardi, G., Richter, S., Oreste, P., Urbinati, C., Rusnati, M., and Wade, R.C. (2022). The binding of heparin to spike glycoprotein inhibits SARS-CoV-2 infection by three mechanisms. J Biol Chem. 298 (2), 101507. doi:10.1016/j.jbc.2021.101507
Pal, S., Chowdhury, T., Paria, K., Manna, S., Parveen, S., Singh, M., et al. (2022). Brief survey on phytochemicals to prevent COVID-19. J. Indian Chem. Soc. 99 (2022), 100244. doi:10.1016/j.jics.2021.10024
Parihar, S. (2022). Anti-viral activity of Withania somnifera phytoconstituents against corona virus (SARS-COV-2). J. Pharmacovigil. Drug Res. 3 (2), 22–26. doi:10.53411/jpadr.2022.3.2.5Available online at: https://www.jpadr.com/index.php/jpadr/article/view/61.
Park, B. B., An, J. Y., and Park, S. U. (2021). Recent studies on pinene and its biological and pharmacological activities. EXCLI J. 20, 812–818. doi:10.17179/excli2021-3714
Roy, A., Kucukural, A., and Zhang, Y. (2010). I-TASSER: a unified platform for automated protein structure and function prediction. Nat. Protoc. 5, 725–738. doi:10.1038/nprot.2010.5
Salehi, B., Upadhyay, S., Erdogan Orhan, I., Kumar Jugran, A. L. D., Jayaweera, S., A. Dias, D., et al. (2019). Therapeutic potential of α- and β-pinene: a miracle gift of nature. Biomolecules 9 (11), 738. doi:10.3390/biom9110738
Santos, S., Barata, P., Charmier, A., Lehmann, I., Rodrigues, S., Melosini, M. M., et al. (2022). Cannabidiol and terpene formulation reducing SARS-CoV-2 infectivity tackling a therapeutic strategy. Front. Immunol. 13, 841459. doi:10.3389/fimmu.2022.841459
Saxena, S. K., Kumar, S., Baxi, P., Srivastava, N., Puri, B., and Ratho, R. K. (2020). Chasing COVID-19 through SARS-CoV-2 spike glycoprotein. VirusDis 31 (4), 399–407. doi:10.1007/s13337-020-00642-7
Seliem, I. A., Girgis, A. S., Moatasim, Y., Kandeil, A., Mostafa, A., Ali, M. A., et al. (2021). New pyrazine conjugates: synthesis, computational studies, and antiviral properties against SARS-CoV-2. Chem. Med. Chem. 16 (22), 3418–3427. doi:10.1002/cmdc.202100476
Shi, B., Zheng, J., Xia, S., Lin, S., Wang, X., Liu, Y., et al. (2021). Accessing the syndemic of COVID-19 and malaria intervention in Africa. Infect. Dis. Poverty 10 (5), 5. doi:10.1186/s40249-020-00788-y
Shree, P., Mishra, P., Selvara, C., Singh, S. K., Chaube, R., Garg, N., et al. (2022). Targeting COVID-19 (SARS-CoV-2) main protease through active phytochemicals of ayurvedic medicinal plants – Withania somnifera (Ashwagandha), Tinospora cordifolia (Giloy) and Ocimum sanctum (Tulsi) – a molecular docking study. J. Biomol. Struct. Dyn. 40 (1), 190–203. doi:10.1080/07391102.2020.1810778
Shrödinger, L., and DeLano, W. (2020). PyMOL. Available online at: http://www.pymol.org/pymol (Accessed April 10, 2025).
Singh, P., Chauhan, S. S., Pandit, S., Sinha, M., Gupta, S., Gupta, A., et al. (2022). The dual role of phytochemicals on SARS-CoV-2 inhibition by targeting host and viral proteins. J. Traditional Complementary Med. 12 (1), 90–99. doi:10.1016/j.jtcme.2021.09.001
Tan, T., Li, J., Luo, R., Wang, R., Yin, L., Liu, M., et al. (2021). Recent advances in understanding the mechanisms of elemene in reversing drug resistance in tumor cells: a review. Molecules 26 (19), 5792. doi:10.3390/molecules26195792
Thangaleela, S., Sivamaruthi, B. S., Kesika, P., Tiyajamorn, T., Bharathi, M., and Chaiyasut, C. (2022). A narrative review on the bioactivity and health benefits of alpha-phellandrene. Sci. Pharm. 90 (4), 57. doi:10.3390/scipharm90040057
Turner, A. J. (2015). ACE2 cell Biology, regulation, and physiological functions. Prot. Arm Renin–Angiotensin Syst. (RAS), 185–189. doi:10.1016/B978-0-12-801364-9.00025-0
Umeta-Chali, B., Melaku, T., Berhanu, N., Mengistu, B., Milkessa, G., Mamo, G., et al. (2021). Traditional medicine practice in the context of COVID-19 pandemic: community claim in jimma zone, oromia, Ethiopia. Infect. Drug Resist. 14, 3773–3783. doi:10.2147/IDR.S331434
United Nations Educational, Scientific and Cultural Organization (UNESCO) (2020). The place of african traditional medicine in response to COVID-19 and beyond. Available online at: https://en.unesco.org/news/place-african-traditional-medicine-response-covid-19-and-beyond (Accessed February 17, 2021).
Varshney, K. K., Varshney, M., and Nath, B. (2020). Molecular Modeling of isolated phytochemicals from Ocimum sanctum towards exploring potential inhibitors of SARS coronavirus main protease and papain-like protease to treat COVID-19. Available online at: https://papers.ssrn.com/sol3/papers.cfm?abstract_id=3554371SSRN3554371 (Accessed April 7, 2025).
Villena-Tejada, M., Vera-Ferchau, I., Cardona-Rivero, A., Zamalloa-Cornejo, R., Quispe-Florez, M., Frisancho-Triveño, Z., et al. (2021). Use of medicinal plants for COVID-19 prevention and respiratory symptom treatment during the pandemic in Cusco, Peru: a cross-sectional survey. PLoS One 16 (9), e0257165. doi:10.1371/journal.pone.0257165
Viveiros, M. M. H., Silva, M. G., da Costa, J. G. M., de Oliveira, A. G., Rubio, C., Padovani, C. R., et al. (2022). Anti-inflammatory effects of α-humulene and β-caryophyllene on pterygium fibroblasts. Int. J. Ophthalmol. 15 (12), 1903–1907. doi:10.18240/ijo.2022.12.02
Winger, A., and Caspari, T. (2021). The spike of concern—the novel variants of SARS-CoV-2. Viruses 13 (6), 1002. doi:10.3390/v13061002
World Health Organization (WHO) (2002). World Health Organization traditional medicine strategy 2002-2005. Geneva: World Health Organization. Available online at: https://www.who.int/publications/i/item/WHO-EDM-TRM-2002.1 (Accessed March 20, 2021).
World Health Organization (WHO) (2019). WHO global report on traditional and complementary medicine 2019. Geneva: World Health Organization. Available online at: https://www.who.int/traditional-complementary-integrativemedicine (Accessed February 17, 2021).
World Health Organization (WHO) (2024). Fact sheet about malaria, WORLD MALARIA REPORT. Available online at: https://www.who.int/news-room/fact-sheets/detail/malaria (Accessed February 4, 2025).
Xu, C., Wang, Y., Liu, C., Zhang, C., Han, W., Hong, X., et al. (2021). Conformational dynamics of SARS-CoV-2 trimeric spike glycoprotein in complex with receptor ACE2 revealed by Cryo-EM. Sci. Adv. 7 (1), eabe5575–13. doi:10.1126/sciadv.abe5575
Yan, R., Zhang, Y., Li, Y., Xia, L., Guo, Y., and Zhou, Q. (2020). Structural basis for the recognition of SARS-CoV-2 by full-length human ACE2. Science 367, 1444–1448. doi:10.1126/science.abb2762
Yan, X., Lin, J., Liu, Z., David, S. D., Liang, D., Nie, S., et al. (2024). The recent progress of tricyclic aromadendrene-type sesquiterpenoids: biological activities and biosynthesis. Biomolecules 14 (9), 1133. doi:10.3390/biom14091133
Yashvardhini, N., Samiksha, S., and Jha, D. K. (2021). Pharmacological intervention of various Indian medicinal plants in combating COVID-19 infection. Biomed. Res. Ther. 8 (7), 4461–4475. doi:10.15419/bmrat.v8i7.685
Zhang, Q., Xiang, R., Huo, S., Zhou, Y., Jiang, S., Wang, Q., et al. (2021). Molecular mechanism of interaction between SARS-CoV-2 and host cells and interventional therapy. Signal Transduct. Target. Ther. 6, 233. doi:10.1038/s41392-021-00653-w
Keywords: antiviral activity, molecular modeling, spike-protein, human ACE-2, COVID-19, medicinal plants, phytochemicals, traditional health practitioners (THPs)
Citation: Milugo TK, Owuor B, Okanya PW, Chepukosi K and Obiero GF (2025) Natural compounds from ethno-medicinal plants exhibit multiple binding activities on SARS-CoV-2 spike protein receptor-binding domains. Front. Nat. Prod. 4:1597609. doi: 10.3389/fntpr.2025.1597609
Received: 21 March 2025; Accepted: 24 April 2025;
Published: 09 May 2025.
Edited by:
Islam Husain, University of Mississippi, United StatesReviewed by:
Prashant Mishra, National Institute for Research in Tribal Health (ICMR), IndiaSahar Naveed, Nuclear Institute for Agriculture and Biology, Pakistan
Copyright © 2025 Milugo, Owuor, Okanya, Chepukosi and Obiero. This is an open-access article distributed under the terms of the Creative Commons Attribution License (CC BY). The use, distribution or reproduction in other forums is permitted, provided the original author(s) and the copyright owner(s) are credited and that the original publication in this journal is cited, in accordance with accepted academic practice. No use, distribution or reproduction is permitted which does not comply with these terms.
*Correspondence: Trizah K. Milugo, dG1pbHVnb0BtcmMuZ20=; George F. Obiero, Z2VvcmdlZi5vYmllcm9AdHVrZW55YS5hYy5rZQ==
†ORCID: Trizah K. Milugo, orcid.org/0000-0003-4730-3773; George F. Obiero, orcid.org/0000-0001-5628-7002