Radiation chemical processes in the water layer on the surface of PuO2
- 1UK National Nuclear Laboratory, Culham Science Centre, Abingdon, Oxfordshire, United Kingdom
- 2UK National Nuclear Laboratory, Central Laboratory, Sellafield, Seascale, United Kingdom
It is generally accepted that radiolysis of water on the surface of PuO2 by alpha particles is the source of H2 which can cause pressurisation in sealed storage containers if the material is not adequately conditioned before packing. The mechanisms for this have not been discussed in detail previously. Radiolysis mechanisms of bulk water are summarised and then applied to water at the surface of PuO2. It is shown that the radiolysis processes occurring on timescales of less than 1 ps after energy deposition could have an impact on the storage behaviour of the PuO2 and the potential gas volume generated. Some of the radiolysis products are highly reactive and would be expected to react with plutonium at the surface, affecting the usual water radiolysis processes. A corollary of this observation is that the surface should not be considered a completely crystalline PuO2 solid. It is also highlighted that whilst there are significant uncertainties in the radiolysis process at the PuO2 surface there are also significant uncertainties in H2 formation mechanisms in bulk water. Finally, methods to model the radiolysis process at the surface and the prospects for predictive models are briefly discussed with suggestions for future areas of development.
1 Introduction
The potential for radiolytic production of H2 from moist PuO2 was recognised as soon there was a requirement to store plutonium powder outside of glovebox containment. As long ago as 1965 there was a report on pressurised sealed cans containing plutonium residues in the United Kingdom (UK) (Hodkin et al., 1965), and internationally there have been documented pressurisation events involving stored plutonium, with detailed reports most readily available in the public domain from the United States (US) (US DOE, 1979; Eller et al., 1999). These events led to pragmatic requirements for the conditioning of plutonium for storage in sealed cans based on a mixture of empirical studies and operational experience. Requirements for storage were initially developed for/by individual facilities, but with limited underpinning information published (Cuillerdier et al., 1984). It was in the early 1990s that the US Department of Energy issued the 3013 standard for storage of plutonium-bearing materials, which was first issued in 1994 to apply to US government facilities and has been updated several times based on new information from operational experience and research (US DOE, 2018).
It is only relatively recently that attempts have been made to systematically study the radiolytic gas generation from stored plutonium oxide; initially by Duffey and Livingston (2002) followed by several others (Vladimirova and Kulikov, 2002; Veirs et al., 2012; Sims et al., 2013; Narlesky, 2018; Veirs et al., 2019; Venault et al., 2019; Webb et al., 2023). Most of these papers reported measurements of H2 production but little mention of mechanisms was provided although some general comments were made by Sims et al. (2013) From these studies it is seen that H2 production increases with increasing water content, and in most studies radiation chemical yields of H2 per unit dose [G(H2)]1 increased with water content, implying the H2 production process is more efficient as the number of water layers increases. Key observations in the study by Duffey and Livingston (2002) were that, even with quite high levels of moisture on the PuO2, adding an overpressure of H2 to the apparatus initially resulted in a reduction in the net production rate of H2, whilst increasing the pressure still further resulted in consumption of hydrogen. This indicates that there was a hydrogen removal mechanism. More recent studies of high purity PuO2 in small, sealed vessels by Veirs et al. measured simultaneously the gas pressure, gas composition, and relative humidity providing further important insights. Specifically, that 1) molecular water on the surface reacts through a radiolytic process to form a phase that does not contribute to hydrogen generation, 2) the hydrogen and oxygen concentration reaches a peak in a closed system and then gradually decreases, and 3) radiolytic O2 production is only formed at higher water contents (Veirs et al., 2012). Venault et al. (2019) studied the yield of hydrogen from PuO2 exposed to constant relative humidity but observed some differences from other studies; the initial production rate of H2 decreased until a steady state hydrogen concentration was reached that was much lower in concentrations for freshly produced PuO2 than observed in US or UK studies. In contrast, the steady state was not observed for samples of PuO2 that had aged. Repeated heating of the aged PuO2 restored the original hydrogen generation behaviour suggesting a reaction that was dependent on the surface condition. Finally, experiments by Webb et al. (2023) have shown that subtle effects from the production route of the PuO2 and ambient atmosphere influences the rate of radiolytic hydrogen production. Similar observations of varying radiolytic H2 production rate with ambient atmosphere have also recently been made during irradiation of alumina (Parker-Quaife et al., 2020).
There have been a large number of measurements of H2 formation during irradiation of water adsorbed on oxide surfaces other than PuO2 as well as slurries of oxide powders (Nakashima and Masaki, 1996; Petrik et al., 2001; LaVerne and Tandon, 2003). This field was reviewed by LaVerne et al. (2011) and by Le Caër (2011) in 2011 and the topic remains an active area of research with significant further publications (Ali et al., 2012; Fourdrin et al., 2013; Lainé et al., 2017; Yin et al., 2019; McGrady et al., 2021; Conrad et al., 2022; McGrady et al., 2023). In the first major systematic study of oxides, Petrik et al. (Petrik et al., 2001) divided metal oxides into three groups: those that enhanced the yield of H2 relative to their reference/control experiments with water/water vapour in sealed ampoules (Ga2O3, Y2O3, La2O3, Nd2O3, Sm2O3, Eu2O3, Gd2O3, Yb2O3, Er2O3, HfO2, and ZrO2); those that had yields similar to reference experiments (MgO, CaO, SrO, BaO, ZnO, CdO, Cu2O, NiO, Cr2O3, Al2O3, CeO2, SiO2, TiO2, Nb2O5 and WO3); and those that had lower hydrogen yields than the reference experiments (MnO2, Co3O4, CuO, and Fe2O3). However, there is some uncertainty in these groupings because later studies measuring G(H2), evaluated with respect to the radiation energy directly absorbed by the adsorbed water layer, have found high yields for water adsorbed on CuO (Reiff and LaVerne, 2015a), Fe2O3 (Reiff and LaVerne, 2015b), ZnO (Southworth et al., 2018), and Al2O3 (Reiff and LaVerne, 2017) compared with the yield expected for bulk water (0.45 molec.100eV−1, assuming scavenging of OH radicals) despite these oxides being grouped by Petrik et al. as oxides reducing or not affecting the yield. These differences may be a consequence of material properties and the basis of comparison between reference experiments by Petrik et al. that recorded G(H2) in the range 0.1–3 molec.100eV−1, but it seems most oxides tend to increase the yield of hydrogen from radiolysis of adsorbed moisture relative to bulk water. The high yield of H2 for water adsorbed on oxide surfaces implies an effective mechanism of energy transfer from the oxide to the water. The energy transfer mechanism has not been conclusively elucidated but the formation of excitons (electron-hole bound pairs) in the oxide from irradiation that migrate to the water interface and react causing dissociation of water has been proposed (Petrik et al., 2001).
For PuO2, where self-irradiation of adsorbed water is from alpha radiation from the oxide, G(H2) has been consistently found to be very low for the first monolayer of adsorbed water and increases with increasing water content towards the yield for bulk water (Sims et al., 2013). This implies that energy transfer from the oxide, whatever the mechanism, does not occur effectively in this case. This could be because of the low band gap in PuO2 (Pegg et al., 2018), the reactivity of the PuO2 surface, or radiation damage within the PuO2 results in effective trapping of excitons in competition with migration to the oxide/water interface. Consequently, energy transfer from the solid to adsorbed water is not discussed further in this review and we focus on the physicochemical effects at the surface of PuO2 from radiation chemistry within the water. A further conclusion from this observation is that studies with what are often considered as surrogate oxides for PuO2, such as CeO2, are not relevant to PuO2 in the context of radiation studies.
Haschke proposed that H2 from PuO2 may arise from a chemical reaction (Haschke et al., 2000; Haschke and Haire, 2002) (Eq. 1):
This mechanism has been debated in the literature (Neck et al., 2007a; Neck et al., 2007b; Seibert et al., 2010a; Conradson et al., 2004; Gouder et al., 2007). However, in the context of the present discussion of radiolysis, it is clear that the observed H2 yield in experiments is dose rate dependent, i.e., proportional to the specific activity of the PuO2, and not dominated by a thermal chemical reaction. In this paper we concentrate on radiolysis in the adsorbed H2O layer on PuO2 and specifically H2 and potential O2 production.
The precise mechanism resulting in the small yield of radiolytic H2 from water on PuO2 may seem a little esoteric but the presence or absence of that H2 can have significant ramifications for storage of PuO2. Considerable effort has been put into establishing robust operational practice and controls for conditioning and packaging PuO2 for storage (US DOE, 2018). However, by understanding the mechanisms involved and the factors that may affect the boundary between safe storage and potential pressurisation from gas generation, operational controls can be optimised to ensure robust safe storage while avoiding excessive conservatism in safety cases. This article supports this objective by discussing the differences in the fundamental radiolysis processes that occur in homogenous, bulk water and water adsorbed on the surface of PuO2 powders and aims to discuss the remaining uncertainties and challenges in defining the mechanisms involved. Furthermore, the topic is of basic scientific interest because of the relevance of the fundamental radiolysis processes in water and the apparently unusual behaviour of PuO2 compared with other metal oxides.
2 Radiolysis of bulk water
There has been a vast amount of work on radiolysis of homogenous water and aqueous solutions and this is reviewed in detail elsewhere (Allen, 1961; Buxton, 2008). However, to contrast with the conditions within water adsorbed on a PuO2 surface the basic processes in water radiolysis are summarised here. As alpha particles pass through water they form tracks of discrete energy loss events. There is a spectrum of energy loss events from a few eV up to the kinematic limit, but for alpha particles in water the vast majority of events are less than 100 eV and the most probable and mean energy events around 20 eV and 60 eV, respectively (Pimblott and LaVerne, 2007).
The initial energy deposition processes are ionisation, to give an electron and water cation, and direct excitation to give an excited state H2O∗ (Eqs 2, 3):
The secondary electrons generated from the primary ionisation events also have a range of energies, with the most probable and mean electron energy approximately 10 eV and 50 eV, respectively (Pimblott and LaVerne, 2007). These secondary electrons may in turn cause further ionisations and excitations, but most have a range of around 10 nm such that most energy is deposited close to the primary ionisation event and form a spur that is a cluster of a small number of ions and excited molecules. A small number of higher energy electrons are generated that form tracks of their own, containing around half the total number of ionisations (Lea, 1962).
These spurs are widely separated with low Linear Energy Transfer (LET) radiation, typical of gamma and beta radiation, but they overlap for higher LET. For example, the range of 3 MeV 4He2+ in water is 1.8 × 10−3 cm (Ziegler et al., 2010), so the average LET is 170 eV nm−1. This implies that the primary energy loss events in water form almost a contiguous track of excited/ionised water molecules, with overlapping spurs forming a narrow cylindrical track. This overlap favours second order reactions of radicals in the track, which compete with diffusion away from the spur compared with the more isolated spurs of low LET radiation.
In times up to 1 ps the ionisation of water is followed by several fast reactions:
(i) The electrons from the ionisations lose their energy over a period of 100 fs, thermalise and then become hydrated as
These electrons are referred to as pre-hydrated electrons,
(ii) Recombination of
(iii) H2O+ also reacts rapidly by proton transfer to an adjacent water molecule (Loh et al., 2020; Lin et al., 2021) (Eq. 6):
(iv) excited states can form H2, H• and OH• (Eqs 7, 8):
Where O(1D) and O(3P) are the singlet first excited state and triplet ground state of atomic oxygen. The branching ratios for reactions 7 and 8 are not known in liquid water but in the gas phase reactions 7 and 8 give (Rosen et al., 2000):
However, cage effects in water could be important. The yield of O(3P) is reported to be very small at low and high LET (Brown and Hart, 1972; Brown et al., 1978), and O(1D) if formed would subsequently form H2O2 (Taube, 1957).
At 1 ps after energy deposition the radiolysis products are
Subsequent reactions are close to diffusion controlled in many cases. Although possibly formed by decay of excited states, H2O2 is probably largely formed by (Eq. 9):
This leaves the question as to how H2 is formed and where surprisingly there is some disagreement in the literature. It has long been known there are two components to the yield of H2 (Taube, 1957), one easy to scavenge and another difficult to scavenge; the latter referred to here as “direct” H2. The easy to scavenge H2 is formed by inter-reactions of H• and
The source of “direct” H2 is still debated but generally it is thought to arise from either or both of two mechanisms occurring in timescales of <1 ps. That is, (i) decay of excited states formed either by recombination of the cation and electron or by direct excitation (Eq 13):
Or (ii), dissociative electron attachment whereby a high energy electron (>6 eV) reacts (Eqs 14, 15):
It is difficult to distinguish experimentally between the two mechanisms but some of the aspects were discussed by Sterniczuk and Bartels (2016). It is beyond the scope of this paper to discuss all the arguments, but the fact that reactants which are known to react efficiently with the pre-hydrated electron suppress H2 in the order of their electron reactivity (Pastina et al., 1999) would tend to favour reaction (13) as the mechanism, and the strong dependence on LET indicates a second order process that suggests the excited state from recombination of the water cation and pre-hydrated electron (LaVerne and Pimblott, 2000). Whether H2 is a direct product from the excited state or formed via an energetic hydrogen atom is also debatable. Finally, it is possible that H2 is formed by reactions (10–12) in the spur. It is pertinent to observe that the primary yield for alpha radiolysis water is G(H2) = 1.4 molec.100 eV−1 while the most probably energy loss event for an alpha particle is around 20 eV; consequently, only a fraction of these energy loss events result in the formation of a H2 molecule.
Whatever the mechanism, it is thought that more than 50% of the H2 yield from gamma radiolysis arises from this direct route, G(H2)direct = 0.25–0.35 molec. 100 eV−1 where G(H2)total = 0.45 molec.100 eV−1 (Pastina et al., 1999). The situation at higher LET is less clear. Bartels estimated G(H2)direct = 1 molec.100eV−1 using the Stern-Volmer plots which seems high (Sterniczuk and Bartels, 2016), Pimblott used a value of G(H2)direct = 0.6 molec. 100eV−1 for high LET whereas other modelers have used the same values as low LET (Sanguanmith et al., 2021). Only the e−/H2O+ route might be expected to have an LET dependence along with reactions (10–12). In early deterministic modelling
Many, but not all, radiation chemical reactions between radiolysis products are fast and close to diffusion controlled (ca. 1010 dm3 mol−1 s−1) (Buxton et al., 1988). It is important to note though that there is only one aqueous phase radiation chemical reaction to remove H2 which is the slow reaction (Buxton et al., 1988) (Eq. 16):
Furthermore, O2 is not a direct radiolysis product and is formed by a set of reactions initiated by another relatively slow reaction (Eqs 17, 18):
3 Radiolysis of water on the surface of PuO2
The microstructure of PuO2 creates a very different environment for the radiolysis of adsorbed water compared with bulk water. The microstructure has some dependence on the method and conditions used to prepare plutonium dioxide powder. Industrial scale production has typically used thermal decomposition of Pu(III) or Pu(IV) oxalate (Orr et al., 2015) or thermal denitration of plutonium nitrate (Koizumi et al., 1983) but decomposition of hydroxides or peroxides has also been used (Drummond and Welch, 1957). Waste or residue materials are also be generated from plutonium metal oxidation routes.
3.1 Microstructure of PuO2
For PuO2 produced from plutonium oxalate the particle size is largely determined by the oxalate precipitation conditions and is typically in the 5–30 µm range. Examples of scanning electron microscopy images of Magnox PuO2 produced at Sellafield are shown in Figure 1A illustrating the typical size and morphology of particles. The Specific Surface Area (SSA), determined by the Brunauer Emmett Teller method using nitrogen as the adsorbate, is typically 5–20 m2 g−1 and the crystallite size, determined by analysis of the powder X-ray diffraction line width, is typically 8–50 nm. The SSA decreases with increasing calcination temperature (Orr et al., 2015) and crystallite size increases with higher calcination temperature, Figure 1B. Consequently, particles are porous aggregates of much smaller crystallites. The small crystallite size in PuO2 powders means that the pore dimensions are very small. For example, the pore size distribution of a typical Magnox PuO2 sample produce by calcination at 600°C, analysed using the BJH method (Barrett et al., 1951), is shown in Figure 1C. This indicates a large fraction of the surface area is associated with pores that are a few nm in diameter. Therefore, migration of gas into and out of the porosity of particles occurs by Knudsen diffusion, where molecules are much more likely to collide with surfaces than other gases.
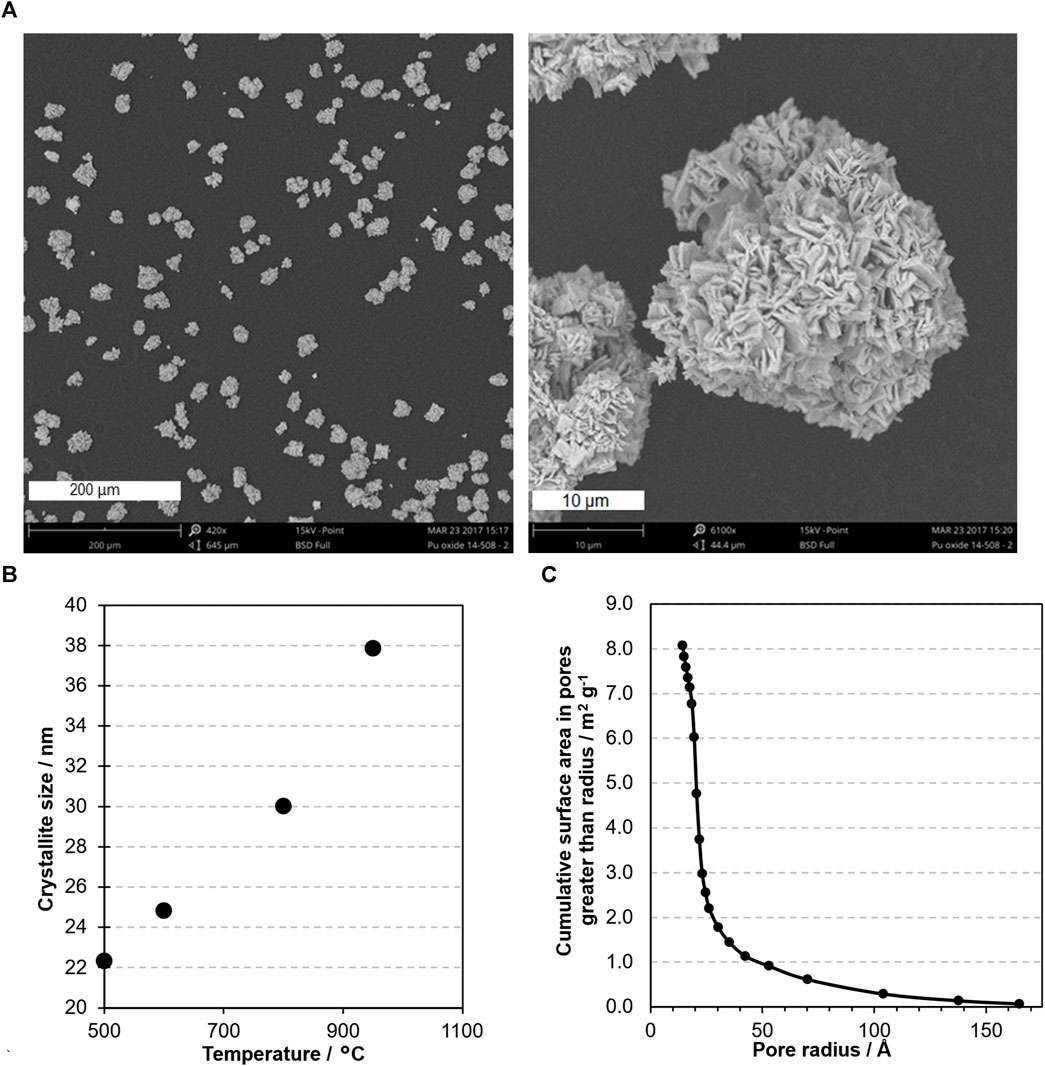
Figure 1. (A) SEM micrograph of Magnox PuO2 illustrating the typical particle size and porous microstructure. (B) Example of crystallite size with calcination temperature of Magnox PuO2. (C) Pore size distribution of typical Magnox PuO2.
PuO2 is generally considered hygroscopic, rapidly adsorbing moisture onto the surface from moist atmospheres. This is similar to other metal oxides and the capacity is largely a consequence of its generally high SSA and tendency of water to dissociate on the surface forming a hydroxyl layer (Seibert et al., 2010b). The structure of water on the surface of PuO2 is an ongoing area of research but it is sufficient here to consider the main observations (Veirs et al., 2019). Water exposed to the surface both reacts and becomes strongly bound at the surface and also physisorbed. The nature of the strongly bound water is not well understood and is discussed further below. Paffett showed that the amount of physisorbed water on the surface depends mainly on the relative humidity of the gas at the surface of the powder (Paffett et al., 2003), and could be reasonably fitted using the BET model with an excess heat of adsorption between 4.3 and 6.8 kJ mol−1 (Brunauer et al., 1938). These extremes of adsorption curves calculated by Paffet et al. are plotted in Figure 2A. The amount of water adsorbed can be quantified in terms of average molecular layers of water and, between 20% and 70% relative humidity, 1 to 3 monolayers of water physisorb, with a water monolayer thickness of about 0.3 nm. In practice, water adsorbed does not build up in gradual layers, rather some areas have thicker layers and others thinner. For example, BET theory models the adsorption as stacks of molecules on the surface and can be used to predict the distribution of stack heights on the surface. This is illustrated schematically in Figure 2B.
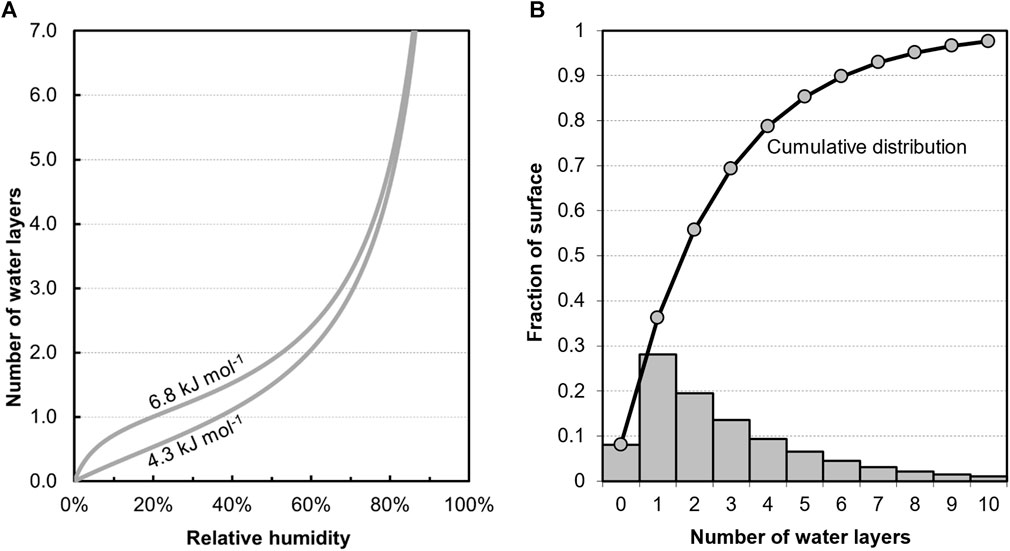
Figure 2. (A) Water physisorption on PuO2 with relative humidity based on Paffett et al. (2003). (B) Site occupation for water adsorption based on BET model calculated for excess heat of adsorption of 5.4 kJ mol−1 and an average of 3 adsorbed water layers (approximately 70% relative humidity).
The range of a 5 MeV alpha particle (typical of 238Pu, 239Pu, 240Pu, 242Pu or 241Pu decay) in crystalline PuO2 is about 12 µm therefore an alpha particle will typically pass through one or two PuO2 particles but will pass several hundred to a few thousand PuO2 crystallites and their associated oxide-water interfaces.
The oxidation state of plutonium at the surface of PuO2 has been investigated as part of studies into the potential for high binary oxides of PuO2. There is evidence of higher oxidation states than Pu(IV) at the surface from X-ray photoelectron spectroscopy (Farr et al., 2004) and X-ray absorption fine structure spectra (Conradson et al., 2004) of PuO2 samples exposed to water. Neck et al. investigated the solubility of plutonium hydrous oxide and concluded the hydrous oxide surface reacts with oxygen forming a mixed valence PuO2+x surface of Pu(IV) and Pu(V) unless reducing or inert conditions are rigorously maintained (Neck et al., 2007c).
3.2 Effects of microstructure and surface on water radiolysis
The purpose of building up this schematic picture of the surface is to contrast the cylindrical track of an alpha particle in homogenous water with the truncated and confined water on the surface in which the spurs can evolve. The high LET of an alpha particle passing through three monolayers of physisorbed water on the surface could cause two or three primary ionisations. With a typical yield of G(H2) = 0.1 molec.100 eV−1 for 3 monolayers of water on PuO2, in the order of only 1 in 10 of these short tracks through the water layer results in net production of a H2 molecule. The track structure through the PuO2 may be more similar to the isolated spurs of low LET radiation in bulk water, reducing second order reactions. Moreover, the small dimensions of the particles and pore cause further confinement of how the spur can evolve compared with the typical diameter of the core of a spur of around 10 nm. Even neglecting interactions of the radiolytically generated species at the surfaces of the water layer, constraining the dimension of the track alone could affect the hydrogen yield, but does not immediately explain the very low yields observed for PuO2.
The surface of PuO2 is not chemically inert. PuO2 undergoes electron transfer reactions which occur, for example, during oxidative dissolution of PuO2 by Ag2+ and reductive dissolution by Cr3+ (Bray and Ryan, 1982; Madic et al., 1992). By analogy it would be expected that OH•,
The first stage of radiolysis, ionisation, involves formation of H2O+ and
This would probably be limited to the first monolayer, the majority of H2O+ species would still undergo proton transfer as in homogenous water.
The pre-hydrated electron,
As noted above, the reaction radius of the pre-hydrated electron in homogeneous water is typically 1 nm. This is important because that is 3–4 times the diameter of a water molecule, typical of the monolayers of physisorbed water on the surface of PuO2 exposed to up to 60%–70% relative humidity. The importance of this reaction depends on the reactivity of
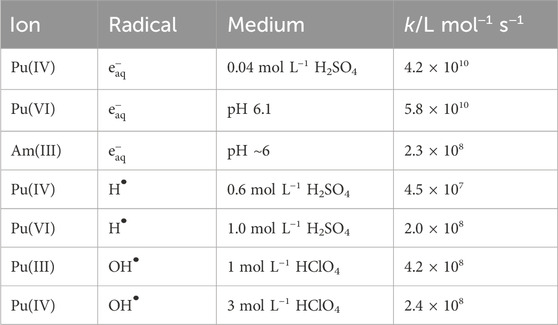
Table 1. Rate constants for reactions of plutonium and americium ions with H, e− and OH Gogolev et al. (1991) and Pikaev et al. (1990).
If H• formed it would be relatively volatile. Sander (2015) gives a Henry’s law constant of 2.6 × 10−4 mol bar−1 for H• compared with H2 7.8 × 10−4 mol bar−1. Diffusion from the water-gas interface would be in competition with reaction in the water or at the oxide surface. However, given the tortuous, narrow porosity in the particles, H• would diffuse via repeated collisions with the pore walls. Gouder et al. (2013) reported that plutonium oxide thin films did not reduce upon contact with atomic hydrogen, but that was for a thin oxide layer formed on plutonium metal. Moist PuO2 may have a layer of Pu(V) at the surface if it has been exposed to trace oxygen or H2 generated leading to oxidation of the surface that may react with H• more readily than Pu(IV).
The above discussion suggests that a process of redox cycling of plutonium at the surface could be an important process in the radiolysis of water on PuO2. (H2) in bulk water is 0.45 molec. 100 eV−1 from gamma radiolysis and 1.4 molec. 100 eV−1 for alpha radiolysis and both these values are much higher than (H2) observed for water on PuO2, which is typically around 0.1 molec. 100 eV−1 for 2–3 monoloyers of water on the surface. This much lower yield on PuO2 would tend to support a hydrogen production mechanism involving either rapid reaction of H2 once formed or reaction of precursors to hydrogen
The fate of H2O2 on the PuO2 surface is not known but is presumably important in determining whether molecular oxygen is produced. Its formation would be suppressed if OH• or e- reacts with the surface and, consequently, may only be significant at high water loadings. It can react as an oxidant or itself be oxidised to O2. Morgenstern and Choppin (1999) reported the reduction of Pu(V) by H2O2; O2 was not measured but it is likely to be the product. Jonsson has shown H2O2 undergoes a complex set of catalytic decomposition reactions on UO2 involving adsorbed OH• radicals (Fidalgo et al., 2018; Kumagai et al., 2019). Analogous reactions may apply to PuO2 but have not been investigated yet. In this scenario, an initial reaction step forming surface bound OH• radicals (Eqs 22, 23) could be followed by a number of possible reactions involving an adsorbed PuO2 ⋯ OH(ads) species (Eqs 24–27).
Once OH• has built up on the surface it can react (Eqs 20, 28):
The rate of reaction and further oxidation of the surface compared with O2 formation reactions (reactions 17 or 23 followed by 18) may be key to understanding conditions resulting in O2 production. The reaction of hydrogen peroxide on the PuO2 surface would be a useful topic for further investigation to clarify the mechanism.
3.3 Effects of radiolysis on the surface
It is tempting to consider the surface of PuO2 as a purely crystalline surface that is distorted by thermal dissociation of molecular water and interaction with overlying H2O molecules to reach a surface at thermal equilibrium. For the purposes of atomistic modelling of the surface this has generally been a necessary simplifying assumption (Tegner et al., 2017). However, it follows from the above discussion that this cannot be the case. The reactions of oxidising and reducing radicals discussed above would suggest that the surface will be oxidised and reduced constantly with the disruption that would necessarily follow.
Since H2 and H• are volatile and experiments show there is little gaseous O2 formed, unless high water loadings are present on the oxide, the surface must become oxidised as H2 is released in order to retain redox balance. Overall, it would be expected that the surface would consist of Pu(V) in contact with PuO2, i.e., PuO2OH(H2O) or surface adsorbed OH•. The reactions of the surface with radicals from water radiolysis provide a possible route to form a kinetically stable, if not thermodynamically stable, phase incorporating water, perhaps as a hydrous oxide. There are two experimental studies that provide evidence for such a process. Firstly, we noted in the introduction that Veirs et al. (2012) have reported the important insight that the relative humidity in sealed reactors containing PuO2 powder with adsorbed moisture decreases at a rate that increases with the specific power of the oxide. Furthermore, the rate of water loss could not be accounted for by the amount of hydrogen produced, implying that the physisorbed water had reacted to form a new surface phase. This reaction could proceed by radical reactions at the surface and/or via the production of H2 and subsequent reaction with the oxidised surface. This difference is important because it determines whether reaction with water occurs in cases where hydrogen is not allowed to accumulate to a significant ambient pressure.
Secondly, Rai and Ryan (1982) reported the interconversion between crystalline, less crystalline and amorphous PuO2 stored as a suspension in aerated water. Crystalline 238PuO2, with its very high specific thermal power, transformed to an amorphous phase with properties similar to Pu(IV) hydrous oxide polymer after prolonged contact with water whereas 239PuO2 and 239Pu hydroxide precipitate aged to form a less crystalline 239PuO2 phase. The radiolysis reactions competing effectively with the thermodynamic aging for the higher activity of 238PuO2. The complete amorphisation of 238PuO2 powder in water suggests the reaction is not limited to the powder surface and it should be able to react with multiple monolayers of physisorbed water present on humidified PuO2 powders. The very low relative humidity that is eventually reached in the small-scale reactor vessel in the experiments by Veirs et al. (2012) suggests the reaction is strongly favoured for powders. Whatever the exact form, thermal analysis of aged PuO2 samples shows that it is necessary to heat samples to over 800°C to fully desorb the water demonstrating that it is strongly chemically bound in the solid. The conditions of a slurry of PuO2 used by Rae and Ryan where an overpressure of H2 could not occur may also suggest that the reaction occurs directly with the radicals from water radiolysis rather than the via formation and reaction of H2. If this picture of surface chemistry is correct, then the implications are that the process may influence aging of the microstructure of the powder as the surface of the oxide undergoes continuous reconstruction. The structure of the surface layer and effect of possible redox cycling of plutonium on local structure (Rotermund et al., 2023) in this context remains to be investigated further.
Further potential complexity in the radiation chemistry at the surface of PuO2 could arise from co-adsorption of other chemical species. In addition to the adsorption of water, PuO2 readily adsorbs CO2 (Colmenares and Terada, 1975), HCl (Webb et al., 2019), and NOx or HNO3/HNO2. The latter is formed from the alpha radiolysis of air in contact with the sample (Harteck and Dondes, 1958; Pshezhetskij and Dmitrieve, 1959; Harteck et al., 1965; Willis et al., 1970; Cuillerdier et al., 1984). These co-adsorbed species have two effects. First, they tend to increase the amount of molecular water adsorbed, for example, co-adsorption of HCl greatly changes the water adsorbed on PuO2 (Sutherland-Harper et al., 2019). Secondly, these species can react with radiolysis products of water. For example, both nitrate and nitrite ions scavenge the pre-hydrated and hydrated electron (Spinks and Wood, 1990). According to Petrik (Aleksandrov et al., 1991), adsorbed NOx can be decomposed radiolytically if, for example, the atmosphere is changed from air to an inert gas. The practical effects of co-adsorbed gases on the H2 yield have not yet been investigated in systematic studies. However, it is often difficult to exclude other gases adsorbing when handling and storing the oxide, which might inadvertently influence the results of experimental studies and adds a complexity to the interpretation of results (Webb et al., 2023).
3.4 Modelling of the radiolysis of water on the PuO2 surface
A few attempts at kinetic modelling of water radiolysis on PuO2 have been reported. Vladimirova developed a kinetic model based on treating water as following the usual homogenous water chemistry of the primary radiolysis products but also allowing for surface reactions of H2O2 and H2O with PuO2 to form PuO2+x (Vladimirova, 2002). The radiation chemical yields of H2 and O2 were derived from fitting experimental measurements while yields of OH• and
None of these models attempted to simulate the fundamental radiolytic processes. To investigate the physical and physico-chemical stages of water radiolysis on a surface, alternative modelling approaches are needed. Simulations of the radiolysis of bulk water break down into two approaches. A stochastic approach which simulates individual ionisation events (Clifford et al., 1986). The other is deterministic (Schwarz, 1969; Burns et al., 1984; Swiatla-Wojcik and Buxton, 1995) where initial product yields and distributions after the formation of
In principle both methods could be used to model processes on the surface of PuO2 but in practice can only be implemented in a general way. Deterministic models are generally limited to the physico-chemical stage, while stochastic models of water at an interface have yet to be developed. Even allowing for those developments, rate constants for reactions of radiolysis products with the surface are required but these are not currently available. However, qualitatively, the effect of including reaction of primary radiolysis species at the PuO2 surface and volatilization at the water-gas interface is simple to infer. For example, if release of H2 and H• at the water-gas interface is included in a model then G(H2) would be reduced because H• is released from the water rather than reacting within the track of the water layer to form H2. Similarly, if reaction of
4 Discussion
In the context of storing PuO2 in sealed containers, it is the overall evolution of the gas phase within the container over time and not just the initial production rate of gases that matters. The initial gas within containers is usually argon, helium, air or nitrogen, dependent on the packaging atmosphere. Of principal concern is the release of hydrogen and oxygen from the radiolysis of adsorbed water, the latter being significant only when relatively large amounts of water are adsorbed (Veirs et al., 2012). The other main source of gas is helium released from alpha decay where the maximum potential release is easily quantified based on the isotopic composition of the plutonium. The release of other gases (mainly N2, CO2 and N2O) from PuO2 has also been observed in some experiments (Narlesky et al., 2022; Berg et al., 2012), presumably from radiolysis of surface adsorbed species, but these are generally observed to be minor components in full size packages where the PuO2 powders have been heated before packaging (Veirs et al., 2018).
As well as gas-forming reactions there are thermal and radiolytic reactions of the gas phase species that decrease the pressure in packages. As already noted, radiolysis of N2 and O2 forms a mixture of ozone and NOx that adsorbs on the oxide surface. Reaction of H2 and O2 may occur by radiolysis (Dautzenberg, 1989; Dautzenberg, 1990) or heterogeneous catalysis on the PuO2 surface (Haschke et al., 2001; Lloyd et al., 1998; Morales, 1998). Homogeneous gas phase radiation chemistry is, in general, highly complex with a plethora of species that can form (Lind, 1961). Within the porosity of PuO2 powder beds, the mean free path of gas species is greater than the pores sizes and the chemistry of the gas phase is presumably further modified by surface reactions. Some of the reactions may be between gas phase species and radiolysis products in the surface layer of the oxide or from radicals/ions generated radiolytically in the gas phase.
Focusing on H2, firstly Veirs et al. (2012) have shown that in small-scale experiments with humidified PuO2 in sealed vessels the partial pressure of hydrogen reaches a maximum before decreasing. Their measurements of a concomitant decrease in the relative humidity suggest the peak in hydrogen concentration is in part driven by the loss of molecular water. When the relative humidity falls to zero, and molecular water is no longer present on the surface, the reactions that normally produce H2 in liquid water (Section 2) cannot now proceed, causing the H2 production rate to decrease to zero. Secondly, Duffey and Livingston (2002) showed that increasing the H2 partial pressure in contact with PuO2 results a decreasing production rate of H2 and at sufficient pressure a net loss of H2 in experiments. Importantly, the conditions in these experiments differ from those of Veirs et al. because the duration of these measurements were (days) compared with those of Veirs et al. (months) and the initial physisorbed water content would have been changing by a relatively small amount in this time. H2 only reacts thermally with PuO2 to reduce the oxide at high temperatures, (Gardner et al., 1965) consequently reaction of H2 with the surface must be radiolytic in nature and the net loss of H2 presumably involves reaction with a previously oxidised surface to maintain redox balance (in addition reduction of PuO2 would presumably be followed by oxidation of the surface by water to reform H2). Reactions of H2 with the aged dry surface may differ from those of the surface with physisorbed water. These two results point to at least two distinct mechanisms that prevent the hydrogen pressure in sealed packages reaching the levels that would result from complete radiolytic decomposition of the water. The former is likely to dominate in relatively dry powders and the latter for higher water levels.
Venault et al. (2019) also found a steady state hydrogen concentration occurred in experiments of freshly prepared PuO2 exposed to a fixed relative humidity but in this case the steady state concentration was very low compared to measurements by Duffy and Livingston or Veirs et al. Aging of the surface affected the recombination process such that the steady state did not occur under their experimental conditions. These observations suggested an effective surface-catalysed recombination processes on the freshly prepared oxide at room temperature, but that the reaction is poisoned by aging of the surface. This discussion highlights the practical significance of the processes occurring and the interdependence of radiolytic gas generation and reactions of H2 but it is beyond the scope of this present work to discuss the gas chemistry in further detail.
It is fortuitous that radiation chemistry of residual water on the surface of PuO2 leads to reactions that result in low yields of H2 and limit the potential for pressurisation by H2. A compelling case has been made here for the effect of the surface on the water radiation chemistry. However, further experimental studies are needed to underpin the mechanistic details. Ideally the reactions of the initial products of water radiolysis at the PuO2 surface would be studied but two challenges are present in taking this forward. Firstly, a consensus on the chemistry in homogeneous water is likely to be needed before progressing to investigate the additional complexity of surface reactions. Secondly, the usual tools of pulse radiolysis are not easily applied where plutonium poses specific challenges for experimental studies owing to its radiotoxicity, yet simulants are unlikely to adequately reproduce the chemical effects. Some further insight may be possible investigating the effect of co-adsorbed scavenging species such as N2O, HNO3 and Cl- with water on PuO2 and its effect on the hydrogen yield.
More amenable to study is the reaction of water with the surface of PuO2, which is of direct practical application to assessments of storage scenarios where this process is likely to be key to limiting the production of hydrogen in sealed containers. It is clearly a radiolytic process but important questions remain to be addressed about the kinetics of the process and the capacity of the oxide to react with water as well as their dependence on SSA, alpha activity, and initial water content. Better understanding is needed of whether the reaction proceeds direct through the initial water radiolysis products reacting with the oxide surface or through production of hydrogen that in turn reacts with the surface as the partial pressure increases. Similarly, the reaction of H2 with the hydrated PuO2 surface investigated by Livingston and Duffey merits further quantification using PuO2 prepared under different conditions: dry PuO2, physisorbed water surface, aged surface with chemically bound water. The effects of hydrogen partial pressure, dose rate, SSA and water coverage are likely to be important. Such studies may be assisted by using thin films where the effects of radiation and thermal chemistry may be better distinguished, building on the studies by Gouder et al. (Seibert et al., 2010a) and preparation and characterisation methods of Wilkerson et al. (Wilkerson et al., 2020) A thin film of ∼10 nm will expose adsorbed water to a very low dose rate, but samples could be irradiated before analysis using an external (Pu,U)O2 pellet to investigate how alpha radiolysis of adsorbed water and reactions with hydrogen or other gases under radiation changes the surface structure. The reaction of H2O2 with the PuO2 surface may also be important to the potential for O2 production. Analogous studies to those undertaken for UO2 by Kumagai et al. (2019) could be pursued and may also provide evidence for stability of surface adsorbed OH• radicals.
Finally, the impact of surfaces on the fundamental water radiolysis processes are not unique to the PuO2 surface, although other transition metal oxides appear to have H2 yields that are generally enhanced by energy transfer from the solid during irradiation. There is a notable gap in the literature on the investigation of other alpha active actinide oxides. Am2O3, AmO2 and NpO2, (Pu,Am)O2 and (Pu,U)O2 powders are obvious candidates to provide comparisons that have different redox properties as well as practical application to their storage. Furthermore, measurements of H2 generation from self-radiolysis of directly synthesised dry plutonium hydroxide would provide evidence that once water has reacted with the surface it does not significantly contribute to gas generation.
With regards to the ultimate goal of a mechanistic kinetic model for radiolysis of water at the surface of PuO2, in the short-term this appears challenging. In the first instance, the outstanding questions around the fundamental processes in bulk homogeneous water need to be resolved and the reaction rates of
5 Conclusion
Mechanistic theories from homogeneous, bulk water radiolysis mechanisms have been applied to water on the surface of PuO2. It is proposed that the surface has a major impact on the radiation chemistry, which in turn changes the structure of the surface as a result of reactions with water radiolysis products. All processes leading to formation of H2 in thin layers of surface water are expected to be affected by the plutonium surface resulting in lower yields than in bulk water, as observed, although effects of the surface should diminish with increasing water loadings. Further experiment and modelling studies are needed to fully underpin the mechanisms involved but significant progress has been made in recently reported work to define these areas of interest.
Data availability statement
The original contributions presented in the study are included in the article/supplementary material, further inquiries can be directed to the corresponding authors.
Author contributions
HS: Conceptualization, Investigation, Writing–original draft, Writing–review and editing. RO: Conceptualization, Investigation, Writing–original draft, Writing–review and editing.
Funding
The authors declare financial support was received for the research, authorship, and/or publication of this article. Sellafield Ltd. and the UK Nuclear Decommissioning Authority are acknowledged for funding experimental studies referenced in the manuscript that the authors were involved in. Journal fees have been funded by the National Nuclear Laboratory.
Acknowledgments
The support of Sellafield Ltd., technical discussion with Dr. Jeff Hobbs and the SNM Value Stream Technical Team, and their permission to include experimental results in Figure 1 is gratefully acknowledged. Valuable discussion with Dr. Kirk Veirs, Los Alamos National Laboratory, and the US DOE Materials Identification and Surveillance program over many years is also acknowledged.
Conflict of interest
Author RO was employed by the UK National Nuclear Laboratory.
The remaining author declares that the research was conducted in the absence of any commercial or financial relationships that could be construed as a potential conflict of interest.
Publisher’s note
All claims expressed in this article are solely those of the authors and do not necessarily represent those of their affiliated organizations, or those of the publisher, the editors and the reviewers. Any product that may be evaluated in this article, or claim that may be made by its manufacturer, is not guaranteed or endorsed by the publisher.
Footnotes
1G(H2) is defined as the number of molecules of H2 produced per 100 eV absorbed. It should be noted that if the amount of water doubles and the amount of H2 doubles G(H2) remains constant, because the energy absorbed increases with water content.
References
Aleksandrov, A. B., Bychkov, A.Yu., Vall, A. I., Petrik, N. G., and Sedov, V. M. (1991). Radiolysis of adsorbed substances on oxide surfaces. Zhurnal Fiz. Khimii 65 (6), 1604–1608.
Ali, I., Imanova, G., Agayev, T., Aliyev, A., Alharbi, O. M. L., Alsubaie, A., et al. (2012). A comparison of hydrogen production by water splitting on the surface of α-, δ- and γ-Al2O3. ChemistrySelect 7, e202202618. doi:10.1002/slct.202202618
Barrett, E. P., Joyner, L. G., and Halenda, P. P. (1951). The determination of pore volume and area distributions in porous substances. I. Computations from nitrogen isotherms. J. Am. Chem. Soc. 73, 373–380. doi:10.1021/ja01145a126
Bartels, D. M., Cook, A. R., Mudaliar, M., and Jonah, C. D. (2000). Spur decay of the solvated electron in picosecond radiolysis measured with time-correlated absorption spectroscopy. J. Phys. Chem. 104, 1686–1691. doi:10.1021/jp992723e
Berg, J. M., Narlesky, J. E., and Veirs, D. K. (2012). A summary of volatile impurity measurements and gas generation studies on MISSTD-1, a high-purity plutonium oxide produced by low-temperature calcination of plutonium oxalate. Los Alamos, New Mexico: Los Alamos National Laboratory. LA-UR-12-21473.
Bray, L. A., and Ryan, J. L. (1982). “Catalyzed electrolytic dissolution of plutonium dioxide,” in Actinide Recovery from waste and low grade sources (Harwood Academic Publishers).
Brown, W. G., and Hart, E. (1972). The oxygen atom, A primary species in irradiated water. Rad. Res. 51, 249–253. doi:10.2307/3573605
Brown, W. G., Hart, E., and Sauer, M. C. (1978). The yield of the O(3P) atom in the radiolysis of water by 20-MeV 2H+ and 40-MeV 4He2+. Rad. Res. 76, 533–539. doi:10.2307/3574802
Brunauer, S., Emmett, P. H., and Teller, E. (1938). Adsorption of gases in multimolecular layers. J. Am. Chem. Soc. 60, 309–319. doi:10.1021/ja01269a023
Burns, W. G., Goodall, J. A. B., and Sims, H. E. (1984). Radiation chemical diffusion kinetic calculations with prescribed and non-prescribed diffusion-I. Radiat. Phys. Chem. 23, 143–180. doi:10.1016/0146-5724(84)90105-5
Buxton, G. V. (2008). “An overview of the radiation chemistry of liquids,” in Radiation chemistry—from Basics to Applications in Material and life sciences. Les ulis, France Editors M. Spotheim- Maurizot, M. Mostafavi, T. Douki, and J. Belloni (Les Ulis, France: EDP Sciences), 35–52.
Buxton, G. V., Greenstock, C. L., Helman, W. P., and Ross, A. (1988). Critical Review of rate constants for reactions of hydrated electrons, hydrogen atoms and hydroxyl radicals (⋅OH/⋅O−) in Aqueous Solution. J. Phys. Chem. Ref. Data 17 (2), 513–886. doi:10.1063/1.555805
Clifford, P., Green, N. J. B., Oldfield, M. J., Pilling, M. J., and Pimblott, S. M. (1986). Stochastic models of multi-species kinetics in radiation-induced spurs. J. Chem. Soc. Faraday Trans. 82, 2673–2689. doi:10.1039/f19868202673
Colmenares, C. A., and Terada, K. (1975). The adsorption of carbon dioxide on uranium and plutonium oxides. J. Nucl. Mater. 58 (3), 336–356. doi:10.1016/0022-3115(75)90125-7
Conrad, J. K., Pu, X., Khanolkar, A., Copeland-Johnson, T. M., Pilgrim, C. D., Wilbanks, J. R., et al. (2022). Radiolytic gas production from aluminum coupons (alloy 1100 and 6061) in helium environments—assessing the extended storage of aluminum clad spent nuclear fuel. Materials 15, 7317. doi:10.3390/ma15207317
Conradson, S. D., Begg, B. D., Clark, D. L., Christophe, D. A., Mei, D., Dorhout, P. K., et al. (2004). Local and nanoscale structure and speciation in the PuO2+x-y(OH)2y·zH2O system. J. Am. Chem. Soc. 126, 13443–13458. doi:10.1021/ja049192e
Conradson, S. D., Begg, B. D., Clark, D. L., Christophe, D. A., Mei, D., Dorhout, P. K., et al. (2023). Local and nanoscale structure and speciation in the PuO2+x-y(OH)2y·zH2O system. J. Am. Chem. Soc. 126, 13443–13458. doi:10.1021/ja049192e
Cuillerdier, C., Cossonnet, C., and Germain, M. (1984). Gas adsorption during storage of plutonium dioxide powders. Lindau: International Conference on Nuclear Reactor and Radiochemistry.
Dautzenberg, D. (1989). Gamma-radiolysis of hydrogen-oxygen-mixtures – Part I. Influences of temperature, vessel wall, pressure and added gases (N2, Ar, H2) on the reactivity of H2/O2- mixtures. Radiat. Phys. Chem. 33, 61–67. doi:10.1016/1359-0197(89)90095-7
Dautzenberg, D. (1990). Gamma-radiolysis of hydrogen-oxygen mixtures—II. Influence of the additives H2O, HNO3, CO2, ethanol, n-pentane and NO2 on the reactivity of H2-O2 and H2-O2-N2-Mixtures. Radiat. Phys. Chem. 36, 767–770. doi:10.1016/1359-0197(90)90176-i
Drummond, J. L., and Welch, G. A. (1957). 964. The preparation and properties of some plutonium compounds. Part VI. Plutonium dioxide. J. Chem. Soc., 4781–4785. doi:10.1039/jr9570004781
Duffey, J. M., and Livingston, R. R. (2002). Gas generation testing of plutonium dioxide. Aiken, South Carolina: Westinghouse Savannah River Company. WSRC-MS-2002-00705.
Eller, P. G., Szempruch, R. W., and McClard, J. W. (1999). Summary of plutonium oxide and metal storage package failures. Los Alamos, New Mexico: Los Alamos National Laboratory. report LA-UR-99-2896.
Farr, J. D., Schulze, R. K., and Neu, M. P. (2004). Surface chemistry of Pu oxides. J. Nucl. Mater. 328, 124–136. doi:10.1016/j.jnucmat.2004.04.001
Fidalgo, A. B., Kumagai, Y., and Jonsson, M. (2018). The role of surface-bound hydroxyl radicals in the reaction between H2O2 and UO2. J. Coord. Chem. 71, 1799–1807. doi:10.1080/00958972.2018.1466287
Flotow, H. E., and Tetenbaum, M. (1981). Heat capacity and thermodynamic functions of β-242Pu2O3 from 8 to 350 K. Contributions to the excess entropy. J. Chem. Phys. 74, 5269–5277. doi:10.1063/1.441691
Fourdrin, C., Aarrachi, H., Latrille, C., Esnouf, S., Bergaya, F., and Le Caër, S. (2013). Water radiolysis in exchanged-montmorillonites: the H2 production mechanisms. Environ. Sci. Technol. 47, 9530–9537. doi:10.1021/es401490t
Gardner, E. R., Markin, T. L., and Street, R. S. (1965). The plutonium-oxygen phase diagram. J. Inorg. Chem. 27, 541–551. doi:10.1016/0022-1902(65)80259-7
Gogolev, A. V., Shilov, V. P., Fedoseev, A. M., and Pikaev, A. K. (1991). The study of reactivity of actinide ions towards hydrated electrons and hydrogen atoms in acid aqueous solutions by a pulse radiolysis method. Int. J. Rad. Applic. Instr. Part C. Rad. Phys. Chem. 37, 531–535. doi:10.1016/1359-0197(91)90031-v
Gouder, T., Seibert, A., Havela, L., and Rebizant, J. (2007). Search for higher oxides of Pu: a photoemission study. Surf. Sci. 601, L77–L80. doi:10.1016/j.susc.2007.04.259
Gouder, T., Shick, A. B., and Huber, F. (2013). Surface interaction of PuO2, UO2+x and UO3 with water ice. Top. Catal. 56, 1112–1120. doi:10.1007/s11244-013-0077-3
Harteck, P., and Dondes, S. (1958). “Radiation chemistry of gases,” in Proceedings of the second united nations international conference on the peaceful uses of atomic energy (Geneva, Switzerland: United National Publication).
Harteck, P., Dondes, S., and Thompson, B. (1965). Ozone: decomposition by ionizing radiation. Science 147, 393–394. doi:10.1126/science.147.3656.393
Haschke, J. M., Allen, T. H., and Morales, L. A. (2001). Reactions of plutonium dioxide with water and hydrogen-oxygen mixtures: mechanisms for corrosion of uranium and plutonium. J. Alloys Compd. 314, 78–91. doi:10.1016/s0925-8388(00)01222-6
Haschke, J. M., Allen, , and Morales, (2000). Reaction of plutonium dioxide with water: formation and properties of PuO2+x. Science 5451, 285–287. doi:10.1126/science.287.5451.285
Haschke, J. M., and Haire, R. G. (2002). “Crystalline solids and corrosion chemistry,” in Advances in plutonium chemistry 1967–2000. Editor D. C. Hoffman (La Grange Park: American Nuclear Society), 212–255.
Hodkin, D. J., Pitman, R. S., and Mardon, P. G. (1965). Gas evolution from solid plutonium bearing residues during storage. Harwell, England. AERE-M 1644.
Jonah, C. D., Miller, J. R., and Matheson, M. S. (1977). The reaction of the precursor of the hydrated electron with electron scavengers. J. Phys. Chem. 81, 1618–1622. doi:10.1021/j100532a003
Koizumi, M., Ohtsuka, K., Ohshima, H., Isagawa, H., Akiyama, H., Todokoro, A., et al. (1983). Development of a process for Co-conversion of Pu-U nitrate mixed solutions to mixed oxide powder using microwave heating method. J. Nucl. Sci. Tech. 20, 529–536. doi:10.1080/18811248.1983.9733431
Kumagai, Y., Fidalgo, A. B., and Jonsson, M. (2019). Impact of stoichiometry on the mechanism and kinetics of oxidative dissolution of UO2 induced by H2O2 and γ-irradiation. J. Phys. Chem. 123, 9919–9925. doi:10.1021/acs.jpcc.9b00862
Lainé, M., Balan, E., Allard, T., Paineau, E., Jeunesse, P., Mostafavi, M., et al. (2017). Reaction mechanisms in swelling clays under ionizing radiation: influence of the water amount and of the nature of the clay mineral. RSC Adv. 7, 526–534. doi:10.1039/c6ra24861f
LaVerne, J. (2011). “Radiation chemistry of water with ceramic oxides,” in Charged particle and photon interactions with matter: recent advances, applications, and interfaces. Editors Y. Hatano, Y. Katsumura, and A. Mozumder (Florida, USA: CRC Press), 425–445. doi:10.1021/jp013098s
LaVerne, J. A., and Pimblott, S. M. (2000). New mechanism for H2 formation in water. J. Phys. Chem. 104, 9820–9822. doi:10.1021/jp002893n
LaVerne, J. A., and Tandon, L. (2002). H2 production in the radiolysis of water on CeO2 and ZrO2. J. Phys. Chem. B 106, 380–386. doi:10.1021/jp013098s
LaVerne, J. A., and Tandon, L. (2003). H2 production in the radiolysis of water on UO2 and other oxides. J. Phys. Chem. B 107, 13623–13628. doi:10.1021/jp035381s
Lea, D. E. (1962). Actions of radiations on living cells. 2nd Edition. Cambridge: Cambridge University Press.
Le Caër, S. (2011). Water radiolysis, influence of oxide surfaces on H2 production under ionizing radiation. Water 3 (1), 235–253. doi:10.3390/w3010235
Lin, M. F., Singh, N., Liang, S., Mo, M., Nunes, J. P. F., Ledbetter, K., et al. (2021). Imaging the short-lived hydroxyl-hydronium pair in ionized liquid water. Science 374, 92–95. doi:10.1126/science.abg3091
Lloyd, J. A., Eller, P. G., and Hyder, L. (1998). Literature search on hydrogen/oxygen recombination and generation in plutonium storage environments. Los Alamos, New Mexico: Los Alamos National Laboratory. LA-UR-98-4557.
Loh, Z. H., Doum, y G., Arnold, C., Kjellsson, L., Southworth, S. H., Al Haddad, A., et al. (2020). Observation of the fastest chemical processes in the radiolysis of water. Science 367, 179–182. doi:10.1126/science.aaz4740
Ma, J., Wang, F., and Mostafavi, M. (2018). Ultrafast chemistry of water radical cation, H2O•+, in aqueous solutions. Molecules 23, 244–258. doi:10.3390/molecules23020244
Madic, C., Berger, P., and Manchuron-Mandard, X. (1992). in ‘Mechanisms of the rapid dissolution of plutonium dioxide in acidic media under oxidizing or reducing conditions’ in Transuranium Elements, A Half Century. Editors L. R. Morss, and J. Fuger (Washington, DC: American Chemical Society), 457–468. (Chapter 44).
McGrady, J., Yamashita, S., Kano, S., Yang, H., and Abe, H. (2021). Charge transfer across the Cr2O3, Fe2O3, and ZrO2 oxide/water interface: a pulse radiolysis study. Radiat. Phys. Chem. 180, 109240. doi:10.1016/j.radphyschem.2020.109240
McGrady, J., Yamashita, S., Kano, S., Yang, H., Kimura, A., Taguchi, M., et al. (2023). Radiolysis of water at the surface of ZrO2 nanoparticles. Radiat. Phys. Chem. 209, 110970. doi:10.1016/j.radphyschem.2023.110970
Migus, A., Gaudue, Y., Martin, J. L., and Antonetti, A. (1987). Excess electrons in liquid water, First evidence of a prehydrated state with femtosecond lifetime. Phys. Rev. Lett. 58, 1559–1562. doi:10.1103/physrevlett.58.1559
Morales, L. (1998). ‘Preliminary report on the recombination rates of hydrogen and oxygen over pure and pure plutonium oxides. Los Alamos, New Mexico: Los Alamos National Laboratory. LA-UR-98-5200.
Morales, L., Behrens, R. G., Lyman, J., Tandon, L., Kennison, J., and Paffett, M. (2000). The scientific basis for plutonium stabilization with particular emphasis on gas generation. Tucson, Az: Waste Management.
Morgenstern, A., and Choppin, G. R. (1999). Kinetics of the reduction of Pu(V)O2+ by hydrogen peroxide. Radiochim. Acta 86, 109–114. doi:10.1524/ract.1999.86.34.109
Nakashima, M., and Masaki, N. M. (1996). Radiolytic hydrogen gas formation from water adsorbed on type Y zeolites. Radiat. Phys. Chem. 47, 241–245. doi:10.1016/0969-806x(94)00176-k
Narlesky, J. E. (2018). Investigating the dependence of hydrogen generation from high- purity plutonium oxides on radiolysis. San Deigo: Plutonium Futures – The Science 2018.
Narlesky, J. E., Veirs, D. K., Berg, J. M., Carrillo, A., Martinez, N. A., and Worl, L. A. (2022). ‘MIS shelf-life final report for plutonium oxide item CAN92 (SSR147) from rocky flats analytical laboratory operations. Los Alamos, New Mexico: Los Alamos National Laboratory. LA-UR-22-29337.
Neck, V., Altmaier, M., and Fanghänel, Th. (2007a). Thermodynamic data for hydrous and anhydrous PuO2+x(s). J. Alloys Compd. 444-445, 464–469. doi:10.1016/j.jallcom.2007.01.159
Neck, V., Altmaier, M., and Fanghänel, T. (2007c). Solubility of plutonium hydroxides/hydrous oxides under reducing conditions and in the presence of oxygen. Comptes Rendus Chim. 10, 959–977. doi:10.1016/j.crci.2007.02.011
Neck, V., Altmaier, M., Seibert, A., Yun, J. I., Marquardt, C. M., and Fanghänel, Th. (2007b). Solubility and redox reactions of Pu(IV) hydrous oxide: evidence for the formation of PuO2+x(s, hyd). Radiochim. Acta 95, 193–207. doi:10.1524/ract.2007.95.4.193
Orr, R. M., Sims, H. E., and Taylor, R. J. (2015). A review of plutonium oxalate decomposition reactions and effects of decomposition temperature on the surface area of the plutonium dioxide product. J. Nucl. Mater. 465, 756–773. doi:10.1016/j.jnucmat.2015.06.058
Paffett, M. T., Kelly, D., Joyce, S. A., Morris, J., and Veirs, K. (2003). A critical examination of the thermodynamics of water adsorption on actinide oxide surfaces. J. Nucl. Mater. 322, 45–56. doi:10.1016/s0022-3115(03)00315-5
Parker-Quaife, E. H., Verst, C., Heathman, C. R., Zalupski, P. R., and Horne, G. P. (2020). Radiation-induced molecular hydrogen gas generation in the presence of aluminum alloy 1100. Radiat. Phys. Chem. 177, 109117. doi:10.1016/j.radphyschem.2020.109117
Pastina, B., LaVerne, J. A., and Pimblott, S. M. (1999). Dependence of molecular hydrogen formation in water on scavengers of the precursor to the hydrated electron. J. Phys. Chem. 103, 5841–5846. doi:10.1021/jp991222q
Pegg, J. T., Shields, A. E., Storr, M. T., Wills, A. S., Scanlon, D. O., and de Leeuw, N. H. (2018). Hidden magnetic order in plutonium dioxide nuclear fuel. Phys. Chem. Chem. Phys. 20, 20943–20951. doi:10.1039/c8cp03583k
Petrik, N. G., Alexandrov, A. B., and Vall, A. I. (2001). Interfacial energy transfer during gamma radiolysis of water on the surface of ZrO2 and some other oxides. J. Phys. Chem. B 105, 5935–5944. doi:10.1021/jp004440o
Pikaev, A. K., Gogolev, A. V., Shilov, V. P., and Fedoseev, A. M. (1990). Reactivity of ions of actinides towards inorganic free radicals in irradiated aqueous solutions. Isotopenpraxis 26, 465–469. doi:10.1080/10256019008624364
Pimblott, S. M., and LaVerne, J. A. (2007). Production of low-energy electrons by ionizing radiation. Rad. Phys. Chem. 76, 1244–1247. doi:10.1016/j.radphyschem.2007.02.012
Pshezhetskij, S. Y., and Dmitrieve, M. T. (1959). Nitrogen fixation by the action of ionizing radiations. Int. J. Appl. Radiat. Isotopes 5, 67–69. doi:10.1016/0020-708x(59)90354-0
Rai, D., and Ryan, J. (1982). Crystallinity and solubility of Pu (IV) oxide and hydrous oxide in aged aqueous suspensions. Radiochim. Acta 30, 213–216. doi:10.1524/ract.1982.30.4.213
Reiff, S. C., and LaVerne, J. A. (2015a). Gamma and He ion radiolysis of copper oxides. Jounrnal Phys. Chem. C 119, 8821–8828. doi:10.1021/acs.jpcc.5b02079
Reiff, S. C., and LaVerne, J. A. (2015b). Radiation-Induced chemical changes to iron oxides. J. Phys. Chem. B 119, pp7358–7365. doi:10.1021/jp510943j
Reiff, S. C., and LaVerne, J. A. (2017). Radiolysis of water with aluminum oxide surfaces. Radiat. Phys. Chem. 131, 46–50. doi:10.1016/j.radphyschem.2016.10.022
Rosen, S., Derkatch, A., Semaniak, J., Neau, A., Al-Khalili, A., Le Padellec, A., et al. (2000). Recombination of simple molecular ions studied in storage ring: dissociative recombination of H2O+. Faraday Discuss. 115, 295–330. doi:10.1039/a909314a
Rotermund, B. M., Sperling, J. M., Horne, G. P., Beck, N. B., Wineinger, H. B., Bai, Z., et al. (2023). Co-crystallization of plutonium(III) and plutonium(IV) diglycolamides with Pu(III) and Pu(IV) hexanitrato anions: a route to redox variants of [puIII,IV(DGA)3] [puIII,IV(NO3)6]x. Inorg. Chem. 62, 12905–12912. doi:10.1021/acs.inorgchem.3c01590
Sander, R. (2015). Compilation of Henry’s law constants (version 4.0) for water as solvent. Atmos. Chem. Phys. 15, 4399–4981. doi:10.5194/acp-15-4399-2015
Sanguanmith, S., Meesungnoen, J., Muroya, Y., and Jay-Gerin, J.-P. (2021). Scavenging of “dry” electrons prior to hydration by azide ions: effect on the formation of H2 in the radiolysis of water by 60Co γ-rays and tritium β-electrons. Can. J. Chem. 99, 881–889. doi:10.1139/cjc-2020-0504
Savolainen, J., Uhlig, F., Ahmed, S., Hamm, P., and Jungwirth, P. (2014). Direct observation of the collapse of the delocalized excess electron in water. Nat. Chem. 6 (8), 697–701. doi:10.1038/nchem.1995
Schwarz, H. A. (1969). Applications of the spur diffusion model to the radiation chemistry of aqueous solutions. J. Phys. Chem. 73, 1928–1937. doi:10.1021/j100726a047
Seibert, A., Gouder, T., and Huber, F. (2010a). Interaction of PuO2 thin films with water. Radiochim. Acta 98, 647–657. doi:10.1524/ract.2010.1765
Seibert, A., Gouder, T., and Huber, F. (2010b). Interaction of PuO2 thin films with water. Radiochim. Acta 98, 647–657. doi:10.1524/ract.2010.1765
Sims, H. E., Webb, K. J., Brown, D., Morris, D., and Taylor, R. J. (2013). Hydrogen yields from water on the surface of plutonium dioxide. J. Nucl. Mat. 437, 359–364. doi:10.1016/j.jnucmat.2013.02.040
Southworth, J., Koehler, S. P. K., and Pimblott, S. M. (2018). Gas production from the radiolysis of water adsorbed on ZnO nanoparticles. J. Phys. Chem. C 122, 25158–25164. doi:10.1021/acs.jpcc.8b04276
Spinks, J. W. T., and Wood, R. J. (1990). An introduction to radiation chemistry. 3rd edn. New York: Wiley-Interscience.
Sterniczuk, M., and Bartels, D. M. (2016). Source of molecular hydrogen in high-temperature water radiolysis. J. Phys. Chem. 120, 200–209. doi:10.1021/acs.jpca.5b12281
Sutherland-Harper, S., Livents, F., Pearce, C., Hobbs, J., Orr, R., Taylor, R., et al. (2019). Interactions of HCl and H2O with the surface of PuO2. J. Nucl. Mater. 518 (2019), 256–264. doi:10.1016/j.jnucmat.2019.02.036
Swiatla-Wojcik, D., and Buxton, G. V. (1995). Modeling of radiation spur processes in water at temperatures up to 300°C. J. Phys. Chem. 99, 11464–11471. doi:10.1021/j100029a026
Sworski, T. J. (1964). Kinetic evidence for H3O as the precursor of molecular hydrogen in the radiolysis of water. J. Am. Chem. Soc. 86, 5034–5035. doi:10.1021/ja01076a080
Taube, H. (1957). Photochemical reactions of ozone in solution. Trans. Farad. Soc. 53, 656–665. doi:10.1039/tf9575300656
Tegner, B. E., Molinari, M., Kerridge, A., Parker, S. C., and Kaltsoyannis, N. (2017). Water adsorption on AnO2 {111}, {110}, and {100} surfaces (An = U and Pu), A density functional theory + U study. J. Phys. Chem. C 121 (3), 1675–1682. doi:10.1021/acs.jpcc.6b10986
US Department of Energy (2018). Stabilization, packaging and storage of plutonium bearing materials. Washington, DC: United States Department of Energy. DOE- STD-3013-2018.
Van Nostrand, D., Draganić, I. G., and Draganić, Z. D. (1971). The radiation chemistry of water. New York: Academic Press.
Veirs, D. K., Berg, J. M., Hill, D. D., Harradine, D. M., Narlesky, J. E., Romero, E. L., et al. (2012). Water radiolysis on plutonium dioxide: initial results identifying a threshold relative humidity for oxygen gas generation. Los Alamos, New Mexico: Los Alamos National Laboratory. Report LA-UR-12-26523.
Veirs, D. K., Joyce, S. A., Sims, H. E., Orr, R., Shaw, S., Smith, P. H., et al. (2019b). in ‘Plutonium packing and storage’ in the plutonium handbook. Editors D. Clark, D. A. Geeson, and R. J. Hanrahan 2nd Edition (American Nuclear Society).
Veirs, D. K., Kelly, E. J., Berg, J. M., Nguyen, B., McLard, J. W., Hensel, S. J., et al. (2018). ‘Gas composition observed by destructive examination of 3013 containers. Los Alamos, New Mexico: Los Alamos National Laboratory. LA-UR-17-31465.
Veirs, K. V., Stroud, M. A., Narlesky, J. E., Berg, J. M., Romero, E. L., Rios, D., et al. (2019a). “Investigating the dependence of hydrogen and oxygen generation from high-purity plutonium oxides in sealed containers,” in Proceedings of the 19th international symposium on the packaging and transportation of radioactive materials PATRAM 2019 (New Orleans).
Venault, L., Deroche, A., Gaillard, J., Lemaire, O., Budanova, N., Vermeulen, J., et al. (2019). Dihydrogen H2 steady state in α-radiolysis of water adsorbed on PuO2 surface. Radiat. Phys. Chem. 162, 136–145. doi:10.1016/j.radphyschem.2018.09.022
Vladimirova, M. V. (2002). A mathematical model of radiolysis of water sorbed on PuO2. Radiochemistry 44, 501–507. doi:10.1023/a:1021143827755
Vladimirova, M. V., and Kulikov, I. A. (2002). Formation of H2 and O2 in radiolysis of water sorbed on PuO2. Radiochemistry 44 (1), 86–90. doi:10.1023/a:1014839621040
Webb, K., Gregson, C., Holt, J., McLuckie, B., Orr, R., Sims, H., et al. (2023). Effects of relative humidity, surface area and production route on hydrogen yields from water on the surface of plutonium dioxide. Front. Nucl. Eng. 2, 1127504. doi:10.3389/fnuen.2023.1127504
Webb, K., Taylor, R., Campbell, C., Carrott, M., Gregson, C., Hobbs, J., et al. (2019). Thermal processing of chloride- contaminated plutonium dioxide. ACS Omega 4, 12524–12536. doi:10.1021/acsomega.9b00719
Wilkerson, M. P., Dorhout, J. M., Graham, K. S., Joyce, J. J., Kruk, I. I., Majewski, J., et al. (2020). Structural properties, thicknesses, and qualities of plutonium oxide thin films prepared by polymer assisted deposition. Surf. Sci. 701, 121696. doi:10.1016/j.susc.2020.121696
Willis, C., Boyd, A. W., and Young, M. J. (1970). Radiolysis of air and nitrogen–oxygen mixtures with intense electron pulses: determination of a mechanism by comparison of measured and computed yields. Can. J. Chem. 48, 1515–1525. doi:10.1139/v70-247
Yin, C., Dannoux-Papin, A., Haas, J., and Renault, J.-P. (2019). Influence of calcium to silica ratio on H2 gas production in calcium silicate hydrate. Radiat. Phys. Chem. 162, 66–71. doi:10.1016/j.radphyschem.2019.04.035
Keywords: plutonium oxide, radiation chemistry, hydrogen, water radiolysis, plutonium storage
Citation: Sims HE and Orr RM (2024) Radiation chemical processes in the water layer on the surface of PuO2. Front. Nucl. Eng. 3:1294584. doi: 10.3389/fnuen.2024.1294584
Received: 15 September 2023; Accepted: 15 January 2024;
Published: 17 April 2024.
Edited by:
Edgar C. Buck, Pacific Northwest National Laboratory (DOE), United StatesReviewed by:
Hye-Ryun Cho, Korea Atomic Energy Research Institute (KAERI), Republic of KoreaGregory Holmbeck, Idaho National Laboratory (DOE), United States
Copyright © 2024 Sims and Orr. This is an open-access article distributed under the terms of the Creative Commons Attribution License (CC BY). The use, distribution or reproduction in other forums is permitted, provided the original author(s) and the copyright owner(s) are credited and that the original publication in this journal is cited, in accordance with accepted academic practice. No use, distribution or reproduction is permitted which does not comply with these terms.
*Correspondence: Howard E. Sims, howard.e.sims@uknnl.com; Robin M. Orr, robin.orr@uknnl.com