- Department of Thoracic Surgery, The Affiliated Hospital of Guizhou Medical University, Guiyang, Guizhou, China
Lung cancer is a highly prevalent malignant tumor worldwide, with high incidence and death rates. Recently, there has been increasing recognition of the role of ferroptosis, a unique cell death mechanism, in lung cancer. This review aims to summarize the current research progress on the relationship between ferroptosis and lung cancer. It also provides a comprehensive analysis of the regulatory processes of ferroptosis in various stages, including epigenetics, transcription, post-transcription, translation, and post-translation. Additionally, the review explores the dual nature of ferroptosis in lung cancer progression, which presents interesting therapeutic possibilities. On one hand, ferroptosis can promote the escape of immune surveillance and reduce the efficacy of treatment in the early stages of tumors. On the other hand, it can counter drug resistance, enhance radiosensitivity, and promote immunotherapy. The article also discusses various combination treatment strategies based on the mechanism of ferroptosis. Overall, this review offers a holistic perspective on the role of ferroptosis in the onset, progression, and treatment of lung cancer. It aims to contribute to future research and clinical interventions in this field.
1 Introduction
Lung cancer is a malignant tumor with high incidence and high mortality. Epidemiological statistics show that lung cancer is the leading cause of cancer-related death, with an incidence rate of 11.4% and a mortality rate of 18% among all malignant tumors in the world in 2020 (1). Studies indicate a grim forecast for lung cancer incidence, with many countries anticipating an increase by 2035 (2). While early-stage lung cancer is typically surgically treatable, most cases are diagnosed at intermediate or advanced stages, necessitating conservative treatments. Medication resistance remains a predominant challenge in lung cancer therapy, which most advanced Non-small cell lung cancer (NSCLC) patients develop resistance to current treatments, leading to a worsening of the condition (3). With a 5-year survival rate under 18% (4), the quest for new therapeutic targets, approaches to counteract drug resistance, and ways to improve patients’ quality and duration of life are pivotal in lung cancer research.
Introduced by Stockwell et al., “ferroptosis” represents a unique regulated cell death propelled by iron-dependent lipid peroxidation (5). Its morphology diverges from other forms of cell death characterized by membrane rupture, shrinkage of mitochondrial volume, reduction or disappearance of mitochondrial cristae, and an absence of nuclear condensation and chromatin marginalization. Ferroptosis’ biochemical characteristics are primarily reflected in three aspects. Firstly, the build-up of reactive oxygen species (ROS) is deemed a direct trigger for ferroptosis. Within mitochondria, the accumulation of ROS amplifies oxidative stress by inducing further ROS release through a positive feedback loop. In this milieu, polyunsaturated fatty acid phospholipids (PUFA-PL) are oxidized to form cytotoxic polyunsaturated fatty acid phospholipid hydroperoxides (PUFA-PL-OOH), which causes endoplasmic reticulum membrane stiffness and directly damages cell membranes, leading to cell death. Secondly, lipid peroxidation is considered the central feature of ferroptosis. During the process of ferroptosis, an unrestrained increase in lipid peroxidation can be observed (6). Lipid peroxidation denotes a process where oxidants extract unstable hydrogen atoms from the methylene bridges of polyunsaturated fatty acid (PUFA), culminating in the generation of a plethora of lipid peroxidation free radicals and hydrogen peroxide. During this process, Acyl-CoA synthetase long-chain family member 4 (ACSL4) and lysophosphatidylcholine acyltransferase 3 (LPCAT3) engage sequentially, facilitating the conversion of PUFA, ultimately resulting in the formation of phospholipids with PUFA-PL. Subsequently, catalyzed by lipoxygenase (LOX), these phospholipids undergo further oxidation to form PUFA- PL-OOH. LOX is widely regarded as a key regulator in modulating ferroptosis (7). In the end, in iron metabolism, the Fenton reaction involving Fe2+ and hydrogen peroxide produces vast hydroxyl free radicals, triggering intense oxidative stress and ROS generation, and consequently ferroptosis.
Ferroptosis may play a crucial role in regulating tumor growth. Up to now, numerous studies have been conducted on ferroptosis in relation to tumors. As a regulated cell death form, ferroptosis has been shown to stifle multiple tumors’ growth through drug and cytokine responses. Interestingly, drug-resistant cancer cells, especially those with enhanced metastatic potential, are more susceptible to ferroptosis (6). In lung cancer, especially lung adenocarcinoma, EMT occurs frequently and mainly originates from alveolar type II epithelial cells (8). EMT is associated with PD-L1 expression, especially in lung cancer, where elevated levels of PD-L1 suppress the immune system and make cancer more likely to spread (9). In addition, the integration of ferroptosis into standard treatment protocols has been investigated across a range of malignancies, including hepatocellular carcinoma, colorectal cancer, ovarian cancer, and glioblastoma (10). A surge in research is observed on ferroptosis’s influence in lung cancer, with a rise in related publications, as seen in Figure 1. The objective of this paper is to delve into the function of ferroptosis in lung cancer, its intricate regulatory systems, and potential biological markers, with aspirations of offering novel perspectives for lung cancer management and outcome prediction.
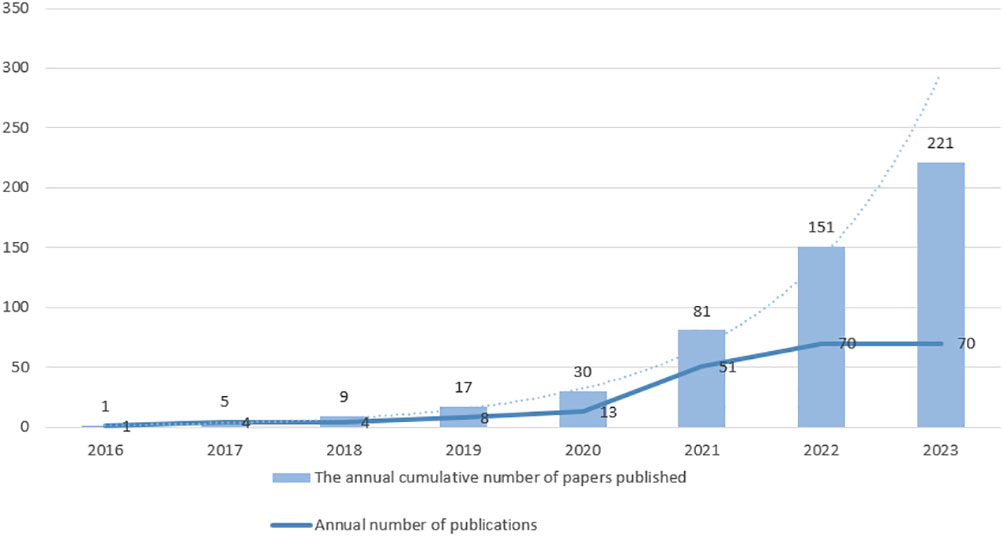
Figure 1 The publications on lung cancer and ferroptosis from 2016 to October 2023. The number of publications related to “lung cancer” and “ferroptosis” from 2016 to October 2023 was searched in PubMed. The broken line represents the number of annual number of publications, and the bar graph represents the annual cumulative number of papers published. The dashed line represents the predicted trend of the index, whose prediction formula is .
2 Ferroptosis in lung cancer
2.1 Ferroptosis
The primary cause of ferroptosis is the accumulation of lipid peroxides (LPO) and ROS within cells, and its mechanism can be summarized as an imbalance of the cellular antioxidant system, iron overload, and lipid peroxidation.
The cystine/glutamate antiporter system (system Xc-) situated on the cellular membrane plays a vital role in the cell’s antioxidative response. Comprising the light chain solute carrier family 7 member 11 (SLC7A11) and the heavy chain solute carrier family 3 member 2 (SLC3A2), system Xc- functions effectively. SLC7A11, a multi-pass transmembrane protein, facilitates the anti-transport activity of cystine/glutamate, exchanging extracellular cystine with intracellular glutamate at a 1:1 ratio. Once inside the cell, cystine gets reduced to cysteine and subsequently collaborates with glutamate and glycine to produce glutathione (GSH). GSH then, under the influence of glutathione peroxidase 4 (GPX4), converts LPO to cell membrane-friendly lipids, countering lipid peroxidation caused by increased ROS (11). SLC7A11 emerges as a central figure in the ferroptosis process, affected by various factors like glutamate and NF-E2-related factor 2(NRF2) ultimately determining the progression of ferroptosis. For example, NRF2 promoted SLC7A11 transcription by binding to the antioxidant response elements in the promoter region of SLC7A11 (12). Erastin treatment can reduce the occupancy of H2Bub1 in the regulatory region of SLC7A11 gene, inhibit the expression of SLC7A11, and increase the sensitivity of cells to ferroptosis (13). Moreover, specific compounds such as RAS selective lethal 3 (RSL3) and ferroptosis inducer 56 (FIN56) target the fundamental factor GPX4 to modulate ferroptosis.
In nature, iron primarily exists as Fe2+ and Fe3+. Dietary iron predominantly appears as Fe3+, which transforms into Fe2+ upon ingestion, making its way to the duodenum. Subsequently, it’s transported into intestinal epithelial cells via Divalent metal transporter 1 (DMT1) and is expelled from the basolateral membrane. It’s been observed that by modulating DMT1 expression, one can influence ferroptosis (14). Excessive intracellular Fe2+, when oxidized to Fe3+, binds to transferrin, forming a state known as the “labile pool”. Iron overload facilitates the Fenton reaction, producing a plethora of hydroxyl radicals, leading to pronounced oxidative stress and spawning a surge in ROS, a prelude to ferroptosis (15). Patel and his team found that GSH can interact with Fe2+ (16). A depletion of GSH not only leads to GPX4 deactivation but also propels Fe2+ towards the Fenton reaction, amplifying lipid peroxide production and accelerating ferroptosis (16). Hence, the regulation of iron metabolism emerges as a crucial intervention point for controlling ferroptosis.
A crucial juncture in ferroptosis is the formation of LPO. Following the involvement of acetyl-CoA in the formation of unbound PUFA, ACSL4 and LPCAT3 aid in activating and embedding PUFA into membrane lipids, resulting in PUFA-PL. With the synergistic effect of Fe2+, PUFA-PL is oxidized by ROS to produce PUFA-PL-OOH. Research emphasizes the central role of LOX in the oxidation of PUFA-PL. Inhibitors targeting LOX can effectively counteract ferroptosis induced by agents like erastin or RSL3 (17). Ultimately, PUFA-PL-OOH causes damage to the cell membrane, leading to cell death.
2.2 Ferroptosis is inhibited in lung cancer
Ferroptosis, although capable of inducing tumor cell death, is prominently suppressed in lung cancer. Studies have identified that in the regulation of the Xc- system, SLC7A11 is overexpressed on the cytoplasmic membrane of NSCLC (18). When SLC7A11 is overexpressed in normal airway epithelial cells, there is an increased glutamine dependence, reduced ROS production, and subsequent ferroptosis suppression, which correlates with a predicted decline in the 5-year survival rate for NSCLC patients (18). In a separate study, it was discovered that LncRNA T-UCR Uc.339 exhibited increased expression levels in patients with LUAD. This upregulation of Uc.339 was found to inhibit ferroptosis and promote LUAD metastasis (19). The mechanism behind this effect involved the inhibition of miR-339, which in turn led to the suppression of the negative regulation of SLC7A11 by miR-339 (19). Zhang Wenjing et al. found that lung cancer tissues exhibit increased expression of RNA binding motif single stranded interacting protein 1 (RBMS1), which may contribute to the evasion of ferroptosis and consequently accelerate the progression of lung cancer (20). Similarly, Lai Yuanyang’s team observed elevated expression of Serine/Threonine/Tyrosine Kinase 1 in NSCLC tissues, and it leads to higher levels of GPX4, promoting cell proliferation and inhibiting ferroptosis (21).
Iron metabolism plays a crucial role in ferroptosis. In both lung cancer tissues and associated cell lines, the expression of iron-sulfur cluster assembly enzyme 1 is enhanced, leading to decreased iron release from the cells and thus inhibition of ferroptosis (22). Serum ferritin (SF) level is increased in NSCLC patients. Lung cancer cells can up-regulate iron-sulfur, inhibit Iron Regulatory Proteins and promote SF expression through NFS1 high expression. Further investigation of this pathway may lead to a better understanding of the mechanism of ferroptosis inhibition in lung cancer.
Lung cancer might also further inhibit ferroptosis by suppressing lipid synthesis. Specifically, lymphoid-specific helicase (LSH) can activate genes associated with lipid metabolism, ferroptosis-related genes and fatty acid desaturase 2, thereby regulating lipid metabolism to inhibit ferroptosis (23). Additionally, a related study found that LSH overexpression decreases intracellular ROS and Fe2+ levels, further inhibiting ferroptosis (24).
Despite these insights, research on ferroptosis inhibition in lung cancer remains in its nascent stages, with most studies predominantly focusing on LUAD. Delving deeper into the inhibitory mechanisms across various lung cancer cell lines will undoubtedly enrich our comprehension of lung cancer progression and unveil novel therapeutic strategies.
3 Regulation of ferroptosis at different levels in lung cancer
Ferroptosis is driven by intracellular iron overload through the Fenton reaction, which catalyzes ROS production and induces lipid peroxidation. Ferroptosis in lung cancer cells undergoes multiple aspects of epigenetic, transcriptional, translational, and post-translational modifications (Figure 2).
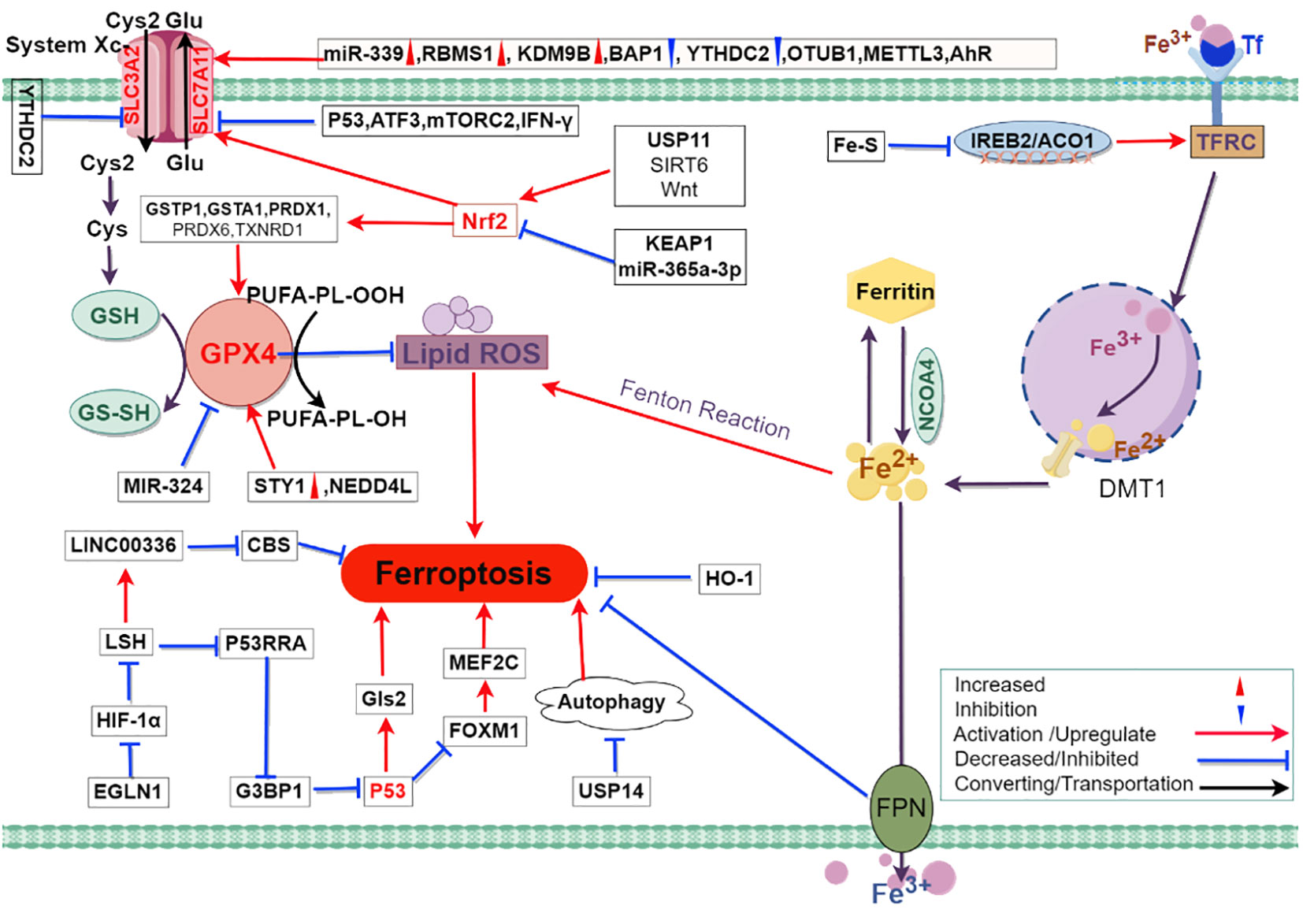
Figure 2 The mechanism of ferroptosis in lung cancer and possible related regulatory pathways. The mechanism of ferroptosis primarily involves iron metabolism, System Xc-, and GPX4. Intracellular iron overload leads to the production of lipid peroxides through the Fenton reaction. System Xc- and GPX4 play a protective role in preventing cell membrane damage caused by lipid peroxides. In the figure, the red triangle marks indicate up-regulated expression of molecules in lung cancer cells, while the blue inverted triangle marks indicate down-regulated expression of molecules in lung cancer cells. The legend in the lower right corner of the figure explains the representation of intermolecular interaction relationships using different lines. Abbreviations: Cys2, cystine; Glu, glutamate; Cys, cysteine; GSH, glutathione; GS-SH, glutathione persulfide; GPX4, glutathione peroxidase 4; SLC3A2, solute carrier family 3 member 2; SLC7A11, solute carrier family 7 member 11; YTHDC2, YTH domain containing 2; PUFAs-OH, hydroxylated polyunsaturated fatty acids; PUFAs-OOH, lipid hydroperoxides; miR-339, microRNA 339; RBMS1, RNA binding motif single stranded interacting protein 1; KDM9B, lysine demethylase 9B; BAP1, BRCA1 associated protein 1; OTUB1, OTU deubiquitinase B1; METTL3, methyltransferase like 3; AhR, aryl hydrocarbon receptor; P53, tumor protein p53; ATF3, activating transcription factor 3; mTORC2, mechanistic target of rapamycin complex 2; IFN-γ, interferon gamma; GSTP1, glutathione S-transferase pi 1; GSTA1, glutathione S-transferase alpha 1; PRDX1, peroxiredoxin 1; PRDX6, peroxiredoxin 6; TXNRD1, thioredoxin reductase 1; USP11, ubiquitin specific peptidase 11; SIRT6, sirtuin 6; Wnt, wingless/integrated; KEAP1, kelch like ECH associated protein 1; miR-365a-3p, microRNA 365a-3p; Nrf2, nuclear factor erythroid 2 related factor 2; ROS, reactive oxygen species; MIR-324, microRNA 324; STY1, stress activated protein kinase STY1/Spc1/Phh1 (fission yeast); NEDD4L, neural precursor cell expressed developmentally downregulated 4 like E3 ubiquitin protein ligase; Fe-S, iron-sulfur; IREB2, iron responsive element binding protein 2; ACO1, aconitase 1; TFR, transferrin receptor; DMT1, divalent metal transporter 1; LINC00336, long intergenic non-protein coding RNA 336; LSH, lymphoid specific helicase; HIF-1α, hypoxia inducible factor 1 alpha; EGLN1, Egl-9 Family Hypoxia Inducible Factor 1; G3BP1, GTPase activating protein binding protein 1; P53RRA, p53 responsive RNA; CBS, cystathionine beta synthase; Gls2, glutaminase 2; FOXM1, forkhead box M1; USP14, ubiquitin specific peptidase 14; HO-1, heme oxygenase 1; FPN, ferroportin.
3.1 Epigenetic regulation of ferroptosis in lung cancer
The epigenetic control of ferroptosis in cancer is intricate, orchestrated by a combination of DNA methylation, RNA modification, and histone modification. At the forefront of DNA methylation, the LSH emerges as a crucial modulator. Studies illustrate that Egl-9 Family Hypoxia Inducible Factor 1 and c-Myc amplify LSH expression by directly inhibiting HIF-1α (23). LSH has the ability to inhibit ferroptosis by down-regulating the tumor suppressor gene LncRNA-P53RRA in NCSC, which is achieved through the mechanism of LSH interfering with the interaction between P53RRA and GTPase-activating protein-binding protein 1(G3BP1) (25). As a result, TP53 is prevented from being released from the G3BP1 complex, thereby inhibiting the promotion of ferroptosis by TP53 (25).
Beyond DNA methylation, m6A methylation stands as a major RNA modification, pivotal in cancer onset, progression, and metastasis. Specifically, the METTL16-mediated m6A modification drive breast cancer progression by inhibiting ferroptosis through the amplification of GPX4 expression. It has been found that that METTL7B is overexpressed in LUAD cells and can up-regulate GPX4 protein level and enzyme activity through m6A modification, which promotes TKI resistance in NSCLC (26). In addition, Ma et al. found that the m6A Reader YTHDC2 can induce ferroptosis by regulating the expression of SLC7A11 (27).Their also revealed that YTHDC2’s influence on the system Xc- extends further, indirectly suppressing the expression of SLC3A2 via the inhibition of Homeo box A13 (27). The N6-methyladenosine (m6A) modification steered by methyltransferase-like 3 was found to stabilize SLC7A11 mRNA, bolstering its translation. This action not only augments LUAD cell growth but also curtails their ferroptosis, offering a promising target for LUAD diagnosis and therapy (28). Bromodomain-containing protein 4 (BRD4) is capable of detecting acetylated histones, thus facilitating gene transcription by recruiting transcription factors. It is noteworthy that BRD4 inhibitors such as JQ1 and JQ4 have demonstrated the ability to induce ferroptosis in lung cancer cells by reducing the expression of SLC7A11 (29).
In summary, further study of the expression of m6A methylation-related molecules in lung cancer cells and the mechanism of ferroptosis regulation by m6A methylation-related molecules such as SLC7A11 and GPX4 may provide a new feasible pathway for the regulation of ferroptosis in lung cancer.
3.2 Transcriptional regulation of ferroptosis in lung cancer
Transcription factors are integral in modulating ferroptosis. One or more such factors can jointly regulate genes associated with ferroptosis, thereby affecting the level of ferroptosis in lung cancer. The current research focus, transcription factor TP53, plays a dual role in regulating ferroptosis. On one front, TP53 suppresses SLC7A11 expression, reducing cystine uptake, promoting glutaminase 2 and heightening cellular sensitivity to ferroptosis. On the other hand, TP53 delayed ferroptosis by upregulating its transcriptional target Cyclin-dependent kinase inhibitor 1A, which inhibited cell cycle progression and slowly consumed glutathione (30). Furthermore, TP53 mutations have been associated with increased resistance to ferroptosis in lung cancer, and this resistance is attributed to the inhibition of forkhead box M1 relieved, which in turn activates myocyte-specific enhancer factor 2C providing stress protection against ferroptosis inducers (31).
Another significant player is Nrf2 that renowned for its antioxidative properties, and its heightened expression shields cancer cells from ferroptosis (32). Inhibition of Nrf2 or promotion of its degradation may be beneficial to ferroptosis induction in lung cancer. For example, it has been found that E3 ubiquitin ligase is overexpressed in some lung squamous cell carcinoma and adenocarcinoma samples, and plays a positive regulatory role in ferroptosis by targeted degradation of NRF2 (33). What’s more, study has shown that NRF2 negatively regulates the expression of focal adhesion protein FOCAD and attenuates its effect on enhancing the sensitivity of NSCLC cells to cysteine deprivation induced ferroptosis, while NRF2 inhibitor Brusatol enhances the therapeutic effect of FOCAD on NSCLC cells (34). Ferroptosis suppressor protein 1 (FSP1) is a transcriptional target of Nrf2. Kelch-like ECH associated protein 1(KEAP1) binds to NRF2 and maintains it at a low level. KEAP1 mutant lung cancer lost its inhibitory effect on NRF2, and when NRF2 is activated, it promotes the expression of FSP1, which inhibits ferroptosis by promoting the reduction of CoQ to CoQH2 (35). It provides a potential therapeutic target for KEAP1 mutant lung cancer. Furthermore, studies have shown that Nrf2 can also regulate the light and heavy chains of ferritin, an iron storage protein (36), and control heme degradation and intracellular iron metabolism through heme oxygenase 1 (HO-1). This suggests that Nrf2 may regulate ferroptosis by influencing iron metabolism, but there is no evidence to support this in lung cancer.
Yes-associated protein serves as a transcriptional co-activator and is suggested to function as an oncogene in LUAD. Through XC- system inhibition, researchers decreased endogenous glutamate build-up, further restrained Yes-associated protein, and assessed LUAD cell sensitivity to ferroptosis, suggesting ferroptosis-based treatments are suitable for late-stage or treatment-resistant LUAD patients (37). Activating transcription factor 3 (ATF3) curtails SLC7A11 expression in a p7-independent manner, accentuating erastin-induced ferroptosis (38). Moreover, the signal transducer and transcription activator 1 activated the transcription of SLC7A11, resulting in an increased expression level of SLC7A11, and epigallocatechin gallate inhibited leppin-induced lung cancer cell growth by down-regulating transducer and transcription activator 1 (39). Latest research suggests the aryl hydrocarbon receptor binding to the SLC7A11 promoter boosts NSCLC progression via SLC7A11 expression activation, augmented cell oxidative sensitivity, and ferroptosis inhibition (40). To conclude, the transcriptional regulation of ferroptosis in lung cancer has unraveled various potential therapeutic targets, furthering the prospects for advanced treatments.
3.3 Post-transcriptional regulation of ferroptosis in lung cancer
Long non-coding RNA (lncRNA) and microRNA (miRNA) are critical regulators in the ferroptosis mechanism of lung cancer. Competing endogenous RNA is a post-transcriptional regulation mode. As mentioned above, LncRNA Uc.339 reduces the level of miR-339 by competing with pri-miR-339, alleviating the inhibitory effect on SLC7A11, and promoting the metastasis of lung cancer cells (19). Additionally, contrary to the competing endogenous RNA mechanism, the Metallothionein pseudogene 1 and NrF2-3 ‘-UTR share a consensus binding site on miR-365a-3p, and Metallothionein pseudogene 1 could enhance the inhibitory effect of miR-365a-3p on NRF2 by directly binding to stabilize the RNA inhibition of Mir-365a-3p, thereby promoting ferroptosis in NSCLC (32). It has been reported that ELAVL1 modified LINC00336 through pseudouridine (Ψ) catalyzed by the RNA modification enzyme PUS10 to enhance its expression and inhibit ferroptosis in lung cancer (24). In addition, the sponging effect of miRNA molecules is essential for the post-transcriptional regulation of genes, and circSCN8A reduces its level by sponging miR-1290 and promotes ferroptosis, thereby inhibiting NSCLC proliferation and metastasis (41). Regarding RNA splicing, some articles screened splicing factors related to ferroptosis, such as RBM10 and SRSF2, which can regulate the splicing of ferroptosis-related genes such as TP53 (42). However, there is a lack of evidence for the role of RNA splicing in the regulation of ferroptosis in lung cancer, and this part of the study needs to be further explored. To encapsulate, these revelations shed light on the intricate post-transcriptional regulatory frameworks governing ferroptosis in lung cancer. Delving deeper into these mechanisms will undoubtedly unearth novel treatment targets and pave the way for groundbreaking therapeutic strategies.
3.4 Translation regulation of ferroptosis in lung cancer
RNA binding proteins can regulate the translation of genes associated with ferroptosis, thereby altering the sensitivity of lung cancer cells to ferroptosis. Research indicates that RBMS1, as a translation regulator for ferroptosis, promotes the translation of SLC7A11 through its T3 region in the 3′-UTR, leading to an escape from ferroptosis and furthering lung cancer progression (20). In contrast, inhibiting RNA binding motif protein 15 enhances ferroptosis in lung cancer cells through the TGF-β/Smad2 pathway (43). This not only reduces their proliferation but also provides a potential therapeutic approach for lung cancer treatment. Another layer of regulation involves iron regulatory proteins that bind to mRNA sequences containing iron-responsive elements, affecting their translation or degradation. For instance, iron regulatory proteins ACO1 and IREB2 bind to transferrin receptor (TFRC) mRNA and enhance its translation, with their activity being influenced by intracellular iron-sulfur (Fe-S) levels (44). When these Fe-S levels are low, increasing the levels of TFRC protein enhances the cell’s ability to uptake iron, subsequently reducing free intracellular iron and preventing iron-dependent cell death (44). Importantly, tribetidine has been found to up-regulate HIF-1α and iron regulatory protein 1, inhibit GPX expression, and induce ferroptosis, thereby inhibiting the growth of lung cancer cells (45). These findings reveal a potential therapeutic strategy for NSCLC.
3.5 Post-translational regulation of ferroptosis in lung cancer
The ubiquitin-proteasome system is pivotal in the post-translational regulation of ferroptosis, with GPX4 being a central molecule in this process. Numerous investigations have highlighted the significant impact of modulating the ubiquitination of GPX4 on the process of ferroptosis in lung cancer. For instance, recent research suggests that Timosaponin AIII forms a complex with Heat Shock Protein 90, resulting in the ubiquitination and degradation of GPX4, thereby inducing ferroptosis in NSCLC (46). Additionally, lactate produced during chemotherapy can inhibit ferroptosis by increasing GPX4 levels. NEDD4 like E3 ubiquitin protein ligase (NEDD4L) binds to GPX4, promoting its ubiquitination and degradation. Lactate can enhance mitochondrial ROS production and activate serum-glucocorticoid-regulated kinase 1, which phosphorylates NEDD4L, thereby reducing its impact on GPX4. These findings reveal the potential role of ferroptosis in chemotherapy resistance (47).
Elevated expression of ubiquitin-specific processing protease 11 has been observed in NSCLC patients (SCC subtype), with its deubiquitinating function stabilizing NRF2, rendering it a crucial molecule for cell proliferation and ferroptosis (48). Additionally, the ubiquitin-specific protease 35 can modulate ferroptosis in lung cancer by targeting iron transport protein (49).The ubiquitination regulation extends to SLC7A11 as well. OTU deubiquitinase B1(OTUB1), an atypical deubiquitinase prevalent in numerous human cancers, principally stabilizes SLC7A11 by impeding its ubiquitination and subsequent proteasomal degradation (20, 50). CD44, serving as an adhesive molecule in cancer stem cells, has a variant, CD44v, that ensures the stability of SLC7A11 protein by adjusting interactions between OTUB1 and SLC7A11 (51). Beyond ubiquitination, SLC7A11 also experiences phosphorylation alterations orchestrated by mechanistic target of rapamycin complex 2 (52).
3.6 Relationship between autophagy and ferroptosis in lung cancer
Autophagy, being a significant pathway, regulates ferroptosis, with some research positing ferroptosis as a cell death mechanism contingent on autophagy (53). Numerous studies underline that modulating autophagy can markedly affect ferroptosis in lung cancer. For instance, d-Borneol has the ability to heighten sensitivity to cisplatin by fostering autophagy, instigating ferroptosis, and inhibiting epithelial-mesenchymal transition, thereby amplifying its antitumor efficacy (54). Conversely, a study by Vrushank Bhatt et al. unveiled that escalated autophagy deters ferroptosis, subsequently bolstering lung cancer’s fortitude against Trametinib (55). Thus, this illustrates that autophagy exerts both facilitative and inhibitory regulatory impacts on ferroptosis within lung cancer. However, further research is needed to determine the specific conditions.
4 Dual role of ferroptosis in lung cancer
Ferroptosis potentially plays a dual role in both the formation and treatment of tumors. While it generally suppresses tumor growth, there are instances where it can facilitate its progression, contingent upon the tumor’s type and stage (56). Initially, ferroptosis may accelerate tumor progression by inciting inflammatory reactions, yet as the disease advances, it helps restrain tumor expansion through inducing cell apoptosis. This dual nature extends to lung cancer, with a nuanced understanding of ferroptosis’s specific impact offering new avenues for treatment strategies.
4.1 Ferroptosis to evade immune surveillance and reduce efficacy
Ye, C. et al. elucidated that Fanconi anemia complementation group D2 (FANCD2), acting as a negative regulator of ferroptosis, is found to be overexpressed in LUAD, which implies a diminished response rate to cancer immunotherapy along with a grim prognosis (57). Ferroptosis can promote tumor growth, proliferation, and help it evade immune surveillance through a variety of mechanisms. Particularly in lung cancer studies, ferroptosis appears to have a correlation with immune infiltration in LUSC, where the process of leukocyte transendothelial migration leads to modulation of M2 macrophages, ensuring their proficient infiltration within the immune microenvironment, thereby exerting an immunosuppressive role and propelling LUSC progression (58). A notable inverse relationship is observed between the majority of immune checkpoint proteins and glyceraldehyde-3-phosphate dehydrogenase (GAPDH) expression. Despite the elevated expression of GAPDH in lung cancer, its manifestation is significantly reduced in ferroptosis scenarios, implying that with the uptick in immune checkpoint protein expression during ferroptosis, tumor cells might navigate immune surveillance more adeptly (59). However, the precise regulatory mechanism of GAPDH in ferroptosis remains veiled, hence, meticulous exploration into its specific function and mechanism could unveil new vistas for lung cancer treatment.
The advent of immunotherapy, portraying a novel treatment paradigm for lung cancer, has ushered in substantial advancements in therapeutic outcomes, extending survival for patients. Delving into the mechanisms and targets exploited by lung cancer to utilize ferroptosis for evading immune monitoring could be instrumental in tackling immunotherapy resistance and catalyzing the innovation of new treatment methodologies.
4.2 Potential value of ferroptosis in lung cancer treatment
Ferroptosis has the potential to directly eliminate cancer cells. A large number of studies have shown that inducing ferroptosis combined with conventional treatment can enhance the therapeutic effect and provide a new way for the treatment of lung cancer. There are numerous treatment options that combine ferroptosis with chemotherapy, radiotherapy, and immunotherapy. Furthermore, a range of ferroptosis-related biomarkers are now considered valuable assets in predicting the outlook for lung cancer.
4.2.1 Decreasing chemoresistance
Drug resistance perennially poses a formidable hurdle in the realm of cancer treatment, particularly when employing targeted drugs like gefitinib or platinum-based chemotherapies for lung cancer. A growing body of evidence underscores the immense potential of ferroptosis in diminishing drug resistance in lung cancer treatment.
Platinum agents form the cornerstone of lung cancer chemotherapy. Harnessing ferroptosis to tackle cisplatin-resistant tumor cells may unveil a powerful therapeutic strategy. Li, Y. et al. illustrated that erastin and sorafenib can efficaciously induce ferroptosis in cisplatin-resistant NSCLC cells (N5CP cells), and when utilized either singularly or concomitantly with a lower dose of cisplatin, they significantly hinder the proliferation of N5CP cells (60). Presently, the spotlight in studies aimed at alleviating drug resistance via ferroptosis is trained on GPX4. Deng, S.H. et al. identified that the overexpression of miR-324-3p gene could target GPX4 directly, substantially amplifying cisplatin-induced ferroptosis in cisplatin-resistant A549 (A549/DDP) cells and counteracting their cisplatin resistance (61). Han, N. et al. recognized that ferroptosis triggered by the downregulation of GPX4 via Dihydroartemisinin can notably boost the efficacy of Photodynamic therapy (62). In conclusion, reducing platinum resistance in lung cancer by inducing ferroptosis may be a powerful measure to improve the efficacy of chemotherapy in lung cancer.
EGFR-TKI resistance is associated with ferroptosis escape in LUAD, which targeting GPX4 can promote ferroptosis in EGFR-TKI resistant LUAD (63). In tackling resistance to EGFR-TKI in lung cancer, Manoalide has been shown to effectively enhance the sensitivity of lung cancer cells to osimertinib by inhibiting the NRF2-SLC7A11 axis and inducing ferroptosis by down-regulation of ferritin heavy chain 1 through mitochondrial Ca2+ overload (64). This highlights the potential of MA as an EGFR-TKI sensitizer, particularly in lung cancer cells with KRAS mutations and resistance to Osimertinib (64). The synergistic use of gefitinib and betulinol can escalate the sensitivity of EGFR wild-type/KRAS mutant NSCLC cells to gefitinib (65). Therefore, the use of ferroptosis inducers in EGFR-TKI resistant lung cancer may be another breakthrough.
The stride in nanotechnology has elevated nanomedicine to a pivotal role in lung cancer treatment. Research indicates that marrying nanocatalytic sensitizers with Amyloid Precursor Protein/Death Receptor 6 Inhibitor 12 can surmount the resistance of NSCLC to osimertinib via the ferroptosis pathway and may thwart its metastasis (66). Recently, academia unearthed that 2-methoxy-6-acetyl-7-methyljuglone can trigger ferroptosis by targeting NAD(P)H quinone oxidoreductase 1 (NQO1) in drug-resistant NSCLC cells, opening the door to a new NQO1-mediated treatment modality to battle resistance (67).
4.2.2 Increased radiosensitivity
Ionizing radiation (IR) is known to trigger ferroptosis, a mechanism that can be leveraged to sensitize radioresistant tumors to radiation therapy (68). Particularly in lung cancer, the protein RBMS1 has been implicated in radioresistance by modulating the expression of SLC7A11. Recent investigations have unveiled that N-desmethyl imipramine hydrochloride (NTP) can alter RBMS1 expression in IR-resistant A549 lung cancer cells, thereby enhancing their susceptibility to radiation therapy, which opens a new therapeutic avenue for lung cancer treatment (20). On a related note, heme is recognized for its ability to neutralize free radicals, thereby guarding cells against oxidative harm. Yet, intriguingly, studies reveal that in irradiated lung cancer cells, heme augments the initial generation of ROS, which catalyzes lipid peroxidation, eventually driving ferroptosis and elevating the radiosensitivity of lung cancer cells (69).
The ongoing research continually unveils the interlink between lung cancer’s radiotherapy sensitivity and ferroptosis. Koppula, P. et al. demonstrated that the use of compounds, such as 4-chlorobenzoic acid, can enhance the radiation tolerance of KEAP1, which is irregularly induced, to effectively treat lung cancers with KEAP1 mutations (35). As of now, no drugs targeting the CoQ-FSP1 pathway have entered clinical trials, and this may be the next feasible research direction for lung cancer treatment. Additionally, with nanotechnology progressing, the exploration concerning the nexus between nanomedicine, radiotherapy, and ferroptosis has commenced, paving new avenues for lung cancer treatment. A marked characteristic of the tumor microenvironment is hypoxia. Studies underscore that hypoxia-induced angiopoietin-like 4 (ANGPTL4) hinders ferroptosis via at least two pathways (intracellular ANGPTL4 and hypoxic exosomal ANGPTL4), bestowing resistance to radiotherapy in NSCLC (70). Clearly, channeling focus on ferroptosis to enhance radiation therapy outcomes is a promising route, meriting further scrutiny.
4.2.3 Enhancing the effect of immunotherapy
Immunotherapy, widely acclaimed as the third revolution in the field of cancer treatment, holds significant promise, particularly in the effective management of advanced non-small cell lung cancer. The mechanism by which ferroptosis promotes tumor immunotherapy is that ferroptosis inducers or immune cells down-regulate ferroptosis resistance genes and increase the incidence of ferroptosis, thereby increasing the generation of immunogenic cell death, improving the immunogenicity of tumor, and activating immune cell attack. As observed by Wang, Song et al., a depletion of intracellular cystine can heighten the T-cell-driven anti-tumor immunological response (71). It has been elucidated that there may be some association between the expression of SLC7A11 and SLC3A2, CD8+T cell count, IFN-γ expression and the prognosis of lung cancer patients (72). Upon activation through immunotherapy, CD8+ T cells can enhance lipid peroxidation specific to tumor cells, which could promote ferroptosis, thereby augmenting the anti-tumor efficacy of the immunotherapeutic approach (73). Furthermore, CD8+ T cells can obstruct the expression of genes SLC3A2 and SLC7A11 through the release of interferon-γ, thereby facilitating the initiation of ferroptosis (74). Ferroptosis can also enhance the effect of tumor immunotherapy by regulating the expression of immune checkpoint molecules on the surface of tumor cells, such as PD-L1 and CTLA-4 (75).
The mechanisms by which ferroptosis enhances tumor cell immune escape and promotes tumor immunotherapy are similar in that they both play a role by regulating ferroptosis related genes and acting on immune checkpoints. The difference may be mainly due to the opposite regulatory direction of the two, such as the former upregulates ferroptosis resistance genes and reduces the incidence of ferroptosis, thereby reducing the production of immunogenic cell death, reducing the immunogenicity of tumor, and escaping the killing of immune cells. Recently, it has been reported that Mitochondrial translocator protein promotes tumor cell immune escape by up-regulating PD-L1 expression through Nrf2-mediated transcription (76).
In conclusion, both immunotherapy and ferroptosis manifest substantial potential in the treatment of lung cancer. Persistent, in-depth probing into novel ferroptosis-based immunotherapies and devising new strategies via combination drug therapies may yield significant advantages for the medical community moving forward.
4.2.4 Predicting prognosis
The search for ferroptosis has led to the search for biomarkers to confirm the occurrence and better induction or regulation of ferroptosis. It could refine the stratification of prognosis groups and formulate strategies to counter resistance in radiotherapy, chemotherapy, or immunotherapy. The latest research shows that Jin Ye’s team made a breakthrough and identified the first specific marker of ferroptosis, peroxiredoxin 3 (77). GPX4, SLC7A11, and FSP1 are considered key targets for modulating ferroptosis and could serve as candidate biomarkers for it. Certain studies posit that dysregulation of GPX4, SLC7A11, and Apoptosis-Inducing Factor Mitochondria-Associated 2 occurs in diverse cancers, suggesting their role as prognostic biomarkers for various malignancies. These markers might aid in assessing the immune cell infiltration within tumor tissues. Analytical data coupled with experimental verification underscores a connection between ferroptosis regulatory elements and tumor immune infiltration in LUAD patients, hinting at their potential as promising biomarkers and treatment targets (78). A summary by Peyman Tabnak et al. outlines ferroptosis-related biomarkers in lung cancer, where markers like ACSL3, FANCD2, and SLC7A11 are associated with adverse prognosis and abbreviated overall survival (79).
Recent findings have unveiled new ferroptosis biomarkers in lung cancer. Deng, B. et al. disclosed that Ribonucleotide Reductase Subunit M2 (RRM2) plays a part in ferroptosis, and is upregulated in LUAD cell lines, suggesting its prospective utility as both a biomarker and therapeutic target for immune infiltration in LUAD (80). Thioredoxin-Interacting Protein shows a positive correlation with immune cell infiltration in SCLC, possibly serving as a biomarker for prognosticating treatment outcomes of chemotherapy and immunotherapy in SCLC patients. Li Lei and his team devised a Ferroptosis-Related Gene Pair Index, which may act as an independent prognostic biomarker for personalized tumor treatment (81). FANCD2, acting as a negative modulator of ferroptosis, can be an independent biomarker indicating unfavorable outcomes in LUAD patients (57). Recent research identifies GAPDH as a ferroptosis marker in LUAD cell lines. The amalgamation of immunotherapy with GAPDH-targeted ferroptosis induction in LUAD could unveil a novel therapeutic avenue (59). Furthermore, Hyper-methylated In Cancer 1 (HIC1) in cancers can not only predict the prognosis (including lung cancer) but also predict the effects of immunotherapy and drug sensitivity, marking HIC1 as a potential biomarker with immune activity (82).
Searching for reliable ferroptosis biomarkers in lung cancer holds immense value for predicting lung cancer prognosis. This endeavor not only aids in anticipating the effectiveness of present treatment modalities but also unveils strategies for combined treatments to tackle drug resistance, thus fostering the advancement of new lung cancer treatments. Nevertheless, the implementation of ferroptosis biomarkers in lung cancer encounters numerous hurdles. A majority of these biomarkers are in the screening phase, with a scant few validated for actual clinical application. The biological mechanisms and functions of certain biomarkers, such as GAPDH, demand further scrutiny, and the precise mechanism of action of HIC1 also calls for thorough investigation.
5 Ferroptosis-related lung cancer therapies
5.1 Medications aimed at lung cancer ferroptosis
Presently, a myriad of drug candidates targeting ferroptosis for lung cancer treatment have been reported. These pharmaceutical agents can be categorized as follows: system Xc- inhibitors that act directly, GPX4 inhibitors, drugs that amplify the Fenton reaction, and those that promote lipid peroxidation. Additionally, certain drugs function indirectly or through a combination of mechanisms. Table 1 summarizes well-characterized drugs in current studies, offering a consolidated view of their mechanisms and efficacy.
As delineated in Table 1, a plethora of therapeutic drugs targeting ferroptosis in lung cancer have been reported. However, the lion’s share of these drug candidates remains in the in vitro research stage. Monotherapy employing ferroptosis-targeted drugs for lung cancer treatment has not yet been fully realized; hence, combination therapies are often advocated for improved outcomes. Interestingly, synthetic drugs make up a smaller proportion of the therapeutic agents in these studies, while naturally-derived compounds dominate. Looking ahead, the burgeoning field of nanomedicine represents a promising frontier for future research efforts.
5.2 Combination therapy strategies aiming at ferroptosis in lung cancer
As previously detailed, enhancing ferroptosis in lung cancer cells substantially elevates their receptivity to diverse treatment protocols. Consequently, an emerging strategy for combating drug-resistant lung cancer involves integrating ferroptosis-targeting drugs with established drug resistance treatments. Table 2 offers a comprehensive summary of existing reports on such combinatory treatment approaches.
Cisplatin is a crucial component of lung cancer chemotherapy. Recent studies have highlighted the effectiveness of combining cisplatin with various ferroptosis inducers. For example, Li, Y. et al. found that low-dose erastin or sorafenib, when used alongside cisplatin, successfully induced ferroptosis in cisplatin-resistant NSCLC N5CP cells (60). Similarly, Liang, Z. et al. observed that the simultaneous use of cisplatin with the erastin analog PRLX93936 suppressed GPX4 expression and triggered ferroptosis (106). Vu, N.T. et al. suggested that the combination of ceramide kinase inhibitors and cisplatin could be a promising therapeutic approach for KRA-mutated NSCLC (115).
Combining ferroptosis-inducing agents with targeted therapies offers another promising avenue. Ishida, T. et al. found that the combined use of the GPX4 inhibitor RSL3 and tyrosine kinase inhibitors might be an effective approach for treating GIST and EGFR-mutated lung cancer (116). Yan, W.Y. et al. suggest that the conjoint utilization of gefitinib and betulinol can transcend the resistance observed in EGFR wild-type/kras-mutated NSCLC cells to gefitinib, heralding a new pathway for the treatment of such lung cancer manifestations (65). Moreover, Xu, C. et al. found that the combination of β-caryophyllene and erlotinib offers a promising treatment strategy for NSCLC patients (110).
Combining the induction of ferroptosis with radiation or immunotherapy holds promise for innovating lung cancer treatment. Integrating immune checkpoint blockade therapies with statin medications has demonstrated a significant enhancement in therapeutic response for NSCLC. It has been observed that when ferroptosis is either inhibited or at a diminished level, tumor cells are inclined to engender an immunologically cold microenvironment (117). Statin medications, known for inducing ferroptosis by restraining the transcriptional expression of PD-L1, mediate an inflammatory tumor microenvironment, thus bolstering anti-PD-1 immunotherapy in NSCLC and unveiling a potential treatment avenue for immune-cold tumors in NSCLC (118).
5.3 Nanomedical approaches to treating ferroptosis in lung cancer
The substantial progress in nanomedicine and tumor diagnostic technologies has fostered notable advancements in lung cancer diagnosis and treatment. Employing nanodrugs in lung cancer treatment can amplify the sensitivity of traditional chemotherapy and radiotherapy, while diminishing cancer cell resistance.
Nanomedicine can trigger iron through various mechanisms and the potential of death, such as amplification Fenton reaction, depletion of glutathione, regulating lipid peroxide and combination therapy, and can ensure the smallest drug side effects and customize precise targeting of cancer treatment. Wei, F. et al. conducted a study that focused on the development of CaCO3 nanoparticles loaded with a Pt (IV) prodrug. To improve their water solubility and tumor targeting, the surfaces of the nanoparticles were modified using DSPE-PEG2000-Biotin (119). The study investigated various mechanisms involved in the nanoparticle’s action, including mitochondrial Ca2+ overload, glutathione depletion, nuclear DNA platination, ROS and lipid peroxide increment, and highlighted the synergistic effects of apoptosis, ferroptosis, and immunogenic cell death (119). Moreover, Zhu, G. et al. crafted a unique Janus nanoparticle (FTG/L&SMD) that, upon entering tumor cells, employs its glucose oxidase to convert glucose into hydrogen peroxide, which then triggers a reaction with iron to produce hydroxyl radicals, inducing lipid peroxidation and, ultimately, ferroptosis in tumor cells (120). Notably, these nanoparticles exhibited negligible toxicity to normal cells, underscoring their potential for NSCLC treatment. Chen, X. et al. synthesized a chiral ruthenium nanoenzyme (D/L-Arginine@Ru) and used it to enhance macrophage M1 polarization, reversing tumor immune suppression. Additionally, the chiral ruthenium nanoenzyme demonstrated binding with O2 and NO, fighting against tumor activity, and ultimately inducing tumor cell apoptosis and ferroptosis, achieving a “cocktail therapy” for lung cancer (121).
Several methodologies have emerged, proposing the amalgamation of nanomedicines with conventional treatments. For instance, a study by Wang, L. et al. demonstrated that, in conjunction with Amyloid Precursor Protein/Death Receptor 6 Inhibitor 12, the nanocatalytic enhancer (VF/S/A@CaP) significantly curtailed the migration of osimertinib-resistant NSCLC, hinting at a potential nanocatalysis-based methodology for treating severe osimertinib-resistant NSCLC (66). In addition, Wang, J. et al. formulated a metal-organic supramolecular compound (nano PMI@CeO2) capable of restoring TP53 and sensitizing ferroptosis, markedly inhibiting tumor progression in a syngeneic transplant model of lung cancer, while maintaining excellent biocompatibility, thereby presenting a potential candidate drug for cancer treatment (122). Furthermore, the pH-responsive superparamagnetic iron oxide nanoparticle clusters crafted by Li, Y. et al. showcase combined diagnostic and therapeutic capabilities. They fortify on-site iron cell death and apoptosis utilizing radiative treatments and chemodynamic therapy methods (123). Through rational design of nanoparticles and their synergistic combination with various conventional treatments, achieving efficient integrated diagnosis and therapy will become a promising direction for lung cancer treatment.
In conclusion, nanomedicine’s ability to enhance ferroptosis, synergize with standard therapies to bolster treatment responsiveness, mitigate medication resistance, alongside its hallmark of precise treatment approaches and reduced toxicity, harbors immense potential for the diagnosis and treatment of lung cancer.
6 Conclusions and discussions
In recent years, the significance of ferroptosis in tumor biology has increased significantly. Ferroptosis is considered to be an emerging field of lung cancer research, providing new perspectives for the occurrence, development and treatment of lung cancer. This article reviews the multifaceted relationship between lung cancer and ferroptosis, and discusses the regulation and potential therapeutic interventions at different molecular levels.
Ferroptosis may be suppressed in lung cancer due to the self-protection of cancer cells. The current research framework of ferroptosis regulation in lung cancer is relatively complete, but there are still some deficiencies in the details. Epigenetic modifications mainly focus on SLC7A11, while the regulation of other factors such as GPX4 still needs further exploration. P53 has a dual effect on ferroptosis, but there is no clear evidence to support its specific role. Tumor cells can promote immune escape and improve the efficacy of immunotherapy by regulating ferroptosis, and the detailed molecular mechanisms need to be further explored. In addition, we found that the development of ferroptosis-targeted drugs for lung cancer has been a hot topic of research, and many chemical and natural drugs have been discovered. However, as shown in Table 1, most of the drugs are only validated in cell and animal models, and many drugs only have theoretical positive therapeutic effects and have not been further developed. The in-depth study of ferroptosis has potential for the diagnosis and treatment of lung cancer, reducing chemotherapy resistance, increasing radiation sensitivity, etc. Many combination therapy strategies have been proposed, but as shown in Table 2, most of them are only studied in vitro, and theoretically provide direction for lung cancer treatment. Doing basic research in the field of ferroptosis in lung cancer is the cornerstone, and translating it to clinical application is the key, and there is still a long way to go. The application of nanomedicine may be a breakthrough for inducing ferroptosis and promoting lung cancer treatment. Most of the nanomaterials are based on the Fenton reaction, and have advantages such as high efficiency, precision and low toxicity in treatment. Attention to the research of nanomedicine in the precise induction of ferroptosis will open up new possibilities for the treatment of lung cancer.
From the perspective of bioinformatics, screening for specific and sensitive ferroptosis-related biomarkers can help to develop targeted therapy strategies and better predict patient outcomes. Jin Ye et al. found the first specific marker of ferroptosis, peroxiredoxin 3 (77), and recent studies have found that PL-PUFA2s can serve as diagnostic and therapeutic targets for regulating ferroptosis, and may become biomarkers of ferroptosis (124). Although GPX4, SLC7A11, FSP1, ACSL3 and FANCD2 can serve as potential biomarkers in lung cancer, they have some guidance for predicting prognosis, but further screening and identification of lung cancer-specific ferroptosis biomarkers still need to be continued, which is of great significance.
In summary, the role of ferroptosis in lung cancer is a current research hotspot. More in-depth exploration of its regulatory mechanisms in lung cancer, finding more targets and specific biomarkers, and developing targeted drugs are the directions we should consider next. Accelerating the clinical development of ferroptosis-targeted drugs and related combination therapy strategies, accelerating the translation of basic research to clinical practice, and truly benefiting lung cancer patients from the research of ferroptosis are our ultimate goals.
Author contributions
YL: Writing – original draft. XL: Writing – original draft. JL: Writing – review & editing.
Funding
The author(s) declare financial support was received for the research, authorship, and/or publication of this article. This research was supported by the National Natural Science Foundation of China (Regional Foundation) (gyfyhsfc-2022-40) and the Department of Science and Technology of Guizhou Province (Qianhe Foundation-ZK [2023] General 363).
Acknowledgments
Thanks to Figdraw online research mapping platform.
Conflict of interest
The authors declare that the research was conducted in the absence of any commercial or financial relationships that could be construed as a potential conflict of interest.
Publisher’s note
All claims expressed in this article are solely those of the authors and do not necessarily represent those of their affiliated organizations, or those of the publisher, the editors and the reviewers. Any product that may be evaluated in this article, or claim that may be made by its manufacturer, is not guaranteed or endorsed by the publisher.
Glossary
References
1. Siegel RL, Miller KD, Fuchs HE, Jemal A. Cancer statistics, 2021. CA Cancer J Clin. (2021) 71:7–33. doi: 10.3322/caac.21654
2. Luo G, Zhang Y, Etxeberria J, Arnold M, Cai X, Hao Y, et al. Projections of lung cancer incidence by 2035 in 40 countries worldwide: population-based study. JMIR Public Health Surveill. (2023) 9:e43651. doi: 10.2196/43651
3. Wang M, Herbst RS, Boshoff C. Toward personalized treatment approaches for non-small-cell lung cancer. Nat Med. (2021) 27:1345–56. doi: 10.1038/s41591-021-01450-2
4. Zappa C, Mousa SA. Non-small cell lung cancer: current treatment and future advances. Trans Lung Cancer Res. (2016) 5:288–300. doi: 10.21037/tlcr.2016.06.07
5. Dixon SJ, Lemberg KM, Lamprecht MR, Skouta R, Zaitsev EM, Gleason CE, et al. Ferroptosis: an iron-dependent form of nonapoptotic cell death. Cell. (2012) 149:1060–72. doi: 10.1016/j.cell.2012.03.042
6. Jiang X, Stockwell BR, Conrad M. Ferroptosis: mechanisms, biology and role in disease. Nat Rev Mol Cell Biol. (2021) 22:266–82. doi: 10.1038/s41580-020-00324-8
7. Kagan VE, Mao G, Qu F, Angeli JP, Doll S, Croix CS, et al. Oxidized arachidonic and adrenic PEs navigate cells to ferroptosis. Nat Chem Biol. (2017) 13:81–90. doi: 10.1038/nchembio.2238
8. Wang Z, Li Z, Zhou K, Wang C, Jiang L, Zhang L, et al. Deciphering cell lineage specification of human lung adenocarcinoma with single-cell RNA sequencing. Nat Commun. (2021) 12:6500. doi: 10.1038/s41467-021-26770-2
9. Ye X, Weinberg RA. Epithelial-mesenchymal plasticity: A central regulator of cancer progression. Trends Cell Biol. (2015) 25:675–86. doi: 10.1016/j.tcb.2015.07.012
10. Zhao L, Zhou X, Xie F, Zhang L, Yan H, Huang J, et al. Ferroptosis in cancer and cancer immunotherapy. Cancer Commun (London England). (2022) 42:88–116. doi: 10.1002/cac2.12250
11. Yang WS, SriRamaratnam R, Welsch ME, Shimada K, Skouta R, Viswanathan VS, et al. Regulation of ferroptotic cancer cell death by GPX4. Cell. (2014) 156:317–31. doi: 10.1016/j.cell.2013.12.010
12. Feng L, Zhao K, Sun L, Yin X, Zhang J, Liu C, et al. SLC7A11 regulated by NRF2 modulates esophageal squamous cell carcinoma radiosensitivity by inhibiting ferroptosis. J Transl Med. (2021) 19:367. doi: 10.1186/s12967-021-03042-7
13. Wang Y, Yang L, Zhang X, Cui W, Liu Y, Sun QR, et al. Epigenetic regulation of ferroptosis by H2B monoubiquitination and p53. EMBO Rep. (2019) 20:e47563. doi: 10.15252/embr.201847563
14. Song Y, Wang B, Zhu X, Hu J, Sun J, Xuan J, et al. Human umbilical cord blood-derived MSCs exosome attenuate myocardial injury by inhibiting ferroptosis in acute myocardial infarction mice. Cell Biol Toxicol. (2021) 37:51–64. doi: 10.1007/s10565-020-09530-8
15. Volani C, Doerrier C, Demetz E, Haschka D, Paglia G, Lavdas AA, et al. Dietary iron loading negatively affects liver mitochondrial function. Metallomics. (2017) 9:1634–44. doi: 10.1039/C7MT00177K
16. Patel SJ, Frey AG, Palenchar DJ, Achar S, Bullough KZ, Vashisht A, et al. A PCBP1-BolA2 chaperone complex delivers iron for cytosolic [2Fe-2S] cluster assembly. Nat Chem Biol. (2019) 15:872–81. doi: 10.1038/s41589-019-0330-6
17. Yang WS, Kim KJ, Gaschler MM, Patel M, Shchepinov MS, Stockwell BR. Peroxidation of polyunsaturated fatty acids by lipoxygenases drives ferroptosis. Proc Natl Acad Sci U S A. (2016) 113:E4966–75. doi: 10.1073/pnas.1603244113
18. Ji X, Qian J, Rahman SMJ, Siska PJ, Zou Y, Harris BK, et al. xCT (SLC7A11)-mediated metabolic reprogramming promotes non-small cell lung cancer progression. Oncogene. (2018) 37:5007–19. doi: 10.1038/s41388-018-0307-z
19. Zhang N, Huang J, Xu M, Wang Y. LncRNA T-UCR uc.339/miR-339/SLC7A11 axis regulates the metastasis of ferroptosis-induced lung adenocarcinoma. J Cancer. (2022) 13:1945–57. doi: 10.7150/jca.65017
20. Zhang W, Sun Y, Bai L, Zhi L, Yang Y, Zhao Q, et al. RBMS1 regulates lung cancer ferroptosis through translational control of SLC7A11. J Clin Invest. (2021) 131:e152067. doi: 10.1172/JCI152067
21. Lai Y, Zhang Z, Li J, Li W, Huang Z, Zhang C, et al. STYK1/NOK correlates with ferroptosis in non-small cell lung carcinoma. Biochem Biophys Res Commun. (2019) 519:659–66. doi: 10.1016/j.bbrc.2019.09.032
22. Alvarez SW, Sviderskiy VO, Terzi EM, Papagiannakopoulos T, Moreira AL, Adams S, et al. NFS1 undergoes positive selection in lung tumours and protects cells from ferroptosis. Nature. (2017) 551:639–43. doi: 10.1038/nature24637
23. Jiang Y, Mao C, Yang R, Yan B, Shi Y, Liu X, et al. EGLN1/c-myc induced lymphoid-specific helicase inhibits ferroptosis through lipid metabolic gene expression changes. Theranostics. (2017) 7:3293–305. doi: 10.7150/thno.19988
24. Wang M, Mao C, Ouyang L, Liu Y, Lai W, Liu N, et al. Long noncoding RNA LINC00336 inhibits ferroptosis in lung cancer by functioning as a competing endogenous RNA. Cell Death Differ. (2019) 26:2329–43. doi: 10.1038/s41418-019-0304-y
25. Mao C, Wang X, Liu Y, Wang M, Yan B, Jiang Y, et al. A G3BP1-Interacting lncRNA Promotes Ferroptosis and Apoptosis in Cancer via Nuclear Sequestration of p53. Cancer Res. (2018) 78:3484–96. doi: 10.1158/0008-5472.CAN-17-3454
26. Song H, Liu D, Wang L, Liu K, Chen C, Wang L, et al. Methyltransferase like 7B is a potential therapeutic target for reversing EGFR-TKIs resistance in lung adenocarcinoma. Mol Cancer. (2022) 21:43. doi: 10.1186/s12943-022-01519-7
27. Ma L, Zhang X, Yu K, Xu X, Chen T, Shi Y, et al. Targeting SLC3A2 subunit of system X(C)(-) is essential for m(6)A reader YTHDC2 to be an endogenous ferroptosis inducer in lung adenocarcinoma. Free Radical Biol Med. (2021) 168:25–43. doi: 10.1016/j.freeradbiomed.2021.03.023
28. Xu Y, Lv D, Yan C, Su H, Zhang X, Shi Y, et al. METTL3 promotes lung adenocarcinoma tumor growth and inhibits ferroptosis by stabilizing SLC7A11 m(6)A modification. Cancer Cell Int. (2022) 22:11. doi: 10.1186/s12935-021-02433-6
29. Sui S, Zhang J, Xu S, Wang Q, Wang P, Pang D. Ferritinophagy is required for the induction of ferroptosis by the bromodomain protein BRD4 inhibitor (+)-JQ1 in cancer cells. Cell Death Dis. (2019) 10:331. doi: 10.1038/s41419-019-1564-7
30. Tarangelo A, Magtanong L, Bieging-Rolett KT, Li Y, Ye J, Attardi LD, et al. p53 suppresses metabolic stress-induced ferroptosis in cancer cells. Cell Rep. (2018) 22:569–75. doi: 10.1016/j.celrep.2017.12.077
31. Peng M, Hu Q, Wu Z, Wang B, Wang C, Yu F. Mutation of TP53 confers ferroptosis resistance in lung cancer through the FOXM1/MEF2C axis. Am J Pathol. (2023) 193:1587–602. doi: 10.1016/j.ajpath.2023.05.003
32. Gai C, Liu C, Wu X, Yu M, Zheng J, Zhang W, et al. MT1DP loaded by folate-modified liposomes sensitizes erastin-induced ferroptosis via regulating miR-365a-3p/NRF2 axis in non-small cell lung cancer cells. Cell Death Dis. (2020) 11:751. doi: 10.1038/s41419-020-02939-3
33. Wang H, Huang Q, Xia J, Cheng S, Pei D, Zhang X, et al. The E3 ligase MIB1 promotes proteasomal degradation of NRF2 and sensitizes lung cancer cells to ferroptosis. Mol Cancer Res MCR. (2022) 20:253–64. doi: 10.1158/1541-7786.MCR-21-0342
34. Liu P, Wu D, Duan J, Xiao H, Zhou Y, Zhao L, et al. NRF2 regulates the sensitivity of human NSCLC cells to cystine deprivation-induced ferroptosis via FOCAD-FAK signaling pathway. Redox Biol. (2020) 37:101702. doi: 10.1016/j.redox.2020.101702
35. Koppula P, Lei G, Zhang Y, Yan Y, Mao C, Kondiparthi L, et al. A targetable CoQ-FSP1 axis drives ferroptosis- and radiation-resistance in KEAP1 inactive lung cancers. Nat Commun. (2022) 13:2206. doi: 10.1038/s41467-022-29905-1
36. Agyeman AS, Chaerkady R, Shaw PG, Davidson NE, Visvanathan K, Pandey A, et al. Transcriptomic and proteomic profiling of KEAP1 disrupted and sulforaphane-treated human breast epithelial cells reveals common expression profiles. Breast Cancer Res Treat. (2012) 132:175–87. doi: 10.1007/s10549-011-1536-9
37. Zhang X, Yu K, Ma L, Qian Z, Tian X, Miao Y, et al. Endogenous glutamate determines ferroptosis sensitivity via ADCY10-dependent YAP suppression in lung adenocarcinoma. Theranostics. (2021) 11:5650–74. doi: 10.7150/thno.55482
38. Bi R, Hu R, Jiang L, Wen B, Jiang Z, Liu H, et al. Butyrate enhances erastin-induced ferroptosis of lung cancer cells via modulating the ATF3/SLC7A11 pathway. Environ Toxicol. (2023) 39:529–38. doi: 10.1002/tox.23857
39. Li F, Hao S, Gao J, Jiang P. EGCG alleviates obesity-exacerbated lung cancer progression by STAT1/SLC7A11 pathway and gut microbiota. J Nutr Biochem. (2023) 120:109416. doi: 10.1016/j.jnutbio.2023.109416
40. Peng Y, Ouyang L, Zhou Y, Lai W, Chen Y, Wang Z, et al. AhR Promotes the Development of Non-small cell lung cancer by Inducing SLC7A11-dependent Antioxidant Function. J Cancer. (2023) 14:821–34. doi: 10.7150/jca.82066
41. Liu B, Ma H, Liu X, Xing W. CircSCN8A suppresses Malignant progression and induces ferroptosis in non-small cell lung cancer by regulating miR-1290/ACSL4 axis. Cell Cycle (Georgetown Tex). (2023) 22:758–76. doi: 10.1080/15384101.2022.2154543
42. Wu Q, Feng L, Wang Y, Mao Y, Di X, Zhang K, et al. Multi-omics analysis reveals RNA splicing alterations and their biological and clinical implications in lung adenocarcinoma. Signal Transduct Target Ther. (2022) 7:270. doi: 10.1038/s41392-022-01098-5
43. Feng J, Li Y, He F, Zhang F. RBM15 silencing promotes ferroptosis by regulating the TGF-beta/Smad2 pathway in lung cancer. Environ Toxicol. (2023) 38:950–61. doi: 10.1002/tox.23741
44. Chen X, Kang R, Kroemer G, Tang D. Broadening horizons: the role of ferroptosis in cancer. Nat Rev Clin Oncol. (2021) 18:280–96. doi: 10.1038/s41571-020-00462-0
45. Cai S, Ding Z, Liu X, Zeng J. Trabectedin induces ferroptosis via regulation of HIF-1α/IRP1/TFR1 and Keap1/Nrf2/GPX4 axis in non-small cell lung cancer cells. Chem Biol Interact. (2023) 369:110262. doi: 10.1016/j.cbi.2022.110262
46. Zhou C, Yu T, Zhu R, Lu J, Ouyang X, Zhang Z, et al. Timosaponin AIII promotes non-small-cell lung cancer ferroptosis through targeting and facilitating HSP90 mediated GPX4 ubiquitination and degradation. Int J Biol Sci. (2023) 19:1471–89. doi: 10.7150/ijbs.77979
47. Cheng F, Dou J, Yang Y, Sun S, Chen R, Zhang Z, et al. Drug-induced lactate confers ferroptosis resistance via p38-SGK1-NEDD4L-dependent upregulation of GPX4 in NSCLC cells. Cell Death Discov. (2023) 9:165. doi: 10.1038/s41420-023-01463-5
48. Meng C, Zhan J, Chen D, Shao G, Zhang H, Gu W, et al. The deubiquitinase USP11 regulates cell proliferation and ferroptotic cell death via stabilization of NRF2 USP11 deubiquitinates and stabilizes NRF2. Oncogene. (2021) 40:1706–20. doi: 10.1038/s41388-021-01660-5
49. Tang Z, Jiang W, Mao M, Zhao J, Chen J, Cheng N. Deubiquitinase USP35 modulates ferroptosis in lung cancer via targeting ferroportin. Clin Trans Med. (2021) 11:e390. doi: 10.1002/ctm2.390
50. Koppula P, Zhuang L, Gan B. Cystine transporter SLC7A11/xCT in cancer: ferroptosis, nutrient dependency, and cancer therapy. Protein Cell. (2021) 12:599–620. doi: 10.1007/s13238-020-00789-5
51. Ishimoto T, Nagano O, Yae T, Tamada M, Motohara T, Oshima H, et al. CD44 variant regulates redox status in cancer cells by stabilizing the xCT subunit of system xc(-) and thereby promotes tumor growth. Cancer Cell. (2011) 19:387–400. doi: 10.1016/j.ccr.2011.01.038
52. Gu Y, Albuquerque CP, Braas D, Zhang W, Villa GR, Bi J, et al. mTORC2 regulates amino acid metabolism in cancer by phosphorylation of the cystine-glutamate antiporter xCT. Mol Cell. (2017) 67:128–38 e7. doi: 10.1016/j.molcel.2017.05.030
53. Liu J, Kuang F, Kroemer G, Klionsky DJ, Kang R, Tang D. Autophagy-dependent ferroptosis: machinery and regulation. Cell Chem Biol. (2020) 27:420–35. doi: 10.1016/j.chembiol.2020.02.005
54. Li J, Yuan J, Li Y, Wang J, Xie Q, Ma R, et al. d-Borneol enhances cisplatin sensitivity via autophagy dependent EMT signaling and NCOA4-mediated ferritinophagy. Phytomedicine Int J phytotherapy phytopharmacology. (2022) 106:154411. doi: 10.1016/j.phymed.2022.154411
55. Bhatt V, Lan T, Wang W, Kong J, Lopes EC, Wang J, et al. Inhibition of autophagy and MEK promotes ferroptosis in Lkb1-deficient Kras-driven lung tumors. Cell Death disease. (2023) 14:61. doi: 10.1038/s41419-023-05592-8
56. Badgley MA, Kremer DM, Maurer HC, DelGiorno KE, Lee HJ, Purohit V, et al. Cysteine depletion induces pancreatic tumor ferroptosis in mice. Science. (2020) 368:85–9. doi: 10.1126/science.aaw9872
57. Ye C, Lu Y, Yuan Z, Mi M, Qi L, Yuan Y, et al. Ferroptosis regulator FANCD2 is associated with immune infiltration and predicts worse prognosis in lung adenocarcinoma. Front Genet. (2022) 13:922914. doi: 10.3389/fgene.2022.922914
58. Lu Y, Yang H, Cao Y, Wang Y, Wu M, He B, et al. A survival model for prognostic prediction based on ferroptosis-associated genes and the association with immune infiltration in lung squamous cell carcinoma. PloS One. (2023) 18:e0282888. doi: 10.1371/journal.pone.0282888
59. Ouyang X, Zhu R, Lin L, Wang X, Zhuang Q, Hu D. GAPDH is a novel ferroptosis-related marker and correlates with immune microenvironment in lung adenocarcinoma. Metabolites. (2023) 13:142. doi: 10.3390/metabo13020142
60. Li Y, Yan H, Xu X, Liu H, Wu C, Zhao L. Erastin/sorafenib induces cisplatin-resistant non-small cell lung cancer cell ferroptosis through inhibition of the Nrf2/xCT pathway. Oncol Lett. (2020) 19:323–33. doi: 10.3892/ol.2019.11066
61. Deng SH, Wu DM, Li L, Liu T, Zhang T, Li J, et al. miR-324-3p reverses cisplatin resistance by inducing GPX4-mediated ferroptosis in lung adenocarcinoma cell line A549. Biochem Biophys Res Commun. (2021) 549:54–60. doi: 10.1016/j.bbrc.2021.02.077
62. Han N, Li LG, Peng XC, Ma QL, Yang ZY, Wang XY, et al. Ferroptosis triggered by dihydroartemisinin facilitates chlorin e6 induced photodynamic therapy against lung cancerthrough inhibiting GPX4 and enhancing ROS. Eur J Pharmacol. (2022) 919:174797. doi: 10.1016/j.ejphar.2022.174797
63. Zhang C, Wang C, Yang Z, Bai Y, Shukuya T, Poh ME, et al. Identification of GPX4 as a therapeutic target for lung adenocarcinoma after EGFR-TKI resistance. Trans Lung Cancer Res. (2022) 11:786–801. doi: 10.21037/tlcr-22-318
64. Ni Y, Liu J, Zeng L, Yang Y, Liu L, Yao M, et al. Natural product manoalide promotes EGFR-TKI sensitivity of lung cancer cells by KRAS-ERK pathway and mitochondrial Ca(2+) overload-induced ferroptosis. Front Pharmacol. (2022) 13:1109822. doi: 10.3389/fphar.2022.1109822
65. Yan WY, Cai J, Wang JN, Gong YS, Ding XB. Co-treatment of betulin and gefitinib is effective against EGFR wild-type/KRAS-mutant non-small cell lung cancer by inducing ferroptosis. Neoplasma. (2022) 69:648–56. doi: 10.4149/neo_2022_211103N1568
66. Wang L, Fu H, Song L, Wu Z, Yu J, Guo Q, et al. Overcoming AZD9291 resistance and metastasis of NSCLC via ferroptosis and multitarget interference by nanocatalytic sensitizer plus AHP-DRI-12. Small (Weinheim an der Bergstrasse Germany). (2023) 19:e2204133. doi: 10.1002/smll.202204133
67. Yu J, Zhong B, Zhao L, Hou Y, Ai N, Lu JJ, et al. Fighting drug-resistant lung cancer by induction of NAD(P)H:quinone oxidoreductase 1 (NQO1)-mediated ferroptosis. Drug Resist Updat. (2023) 70:100977. doi: 10.1016/j.drup.2023.100977
68. Lei G, Zhang Y, Koppula P, Liu X, Zhang J, Lin SH, et al. The role of ferroptosis in ionizing radiation-induced cell death and tumor suppression. Cell Res. (2020) 30:146–62. doi: 10.1038/s41422-019-0263-3
69. Endale HT, Tesfaye W, Mengstie TA. ROS induced lipid peroxidation and their role in ferroptosis. Front Cell Dev Biol. (2023) 11:1226044. doi: 10.3389/fcell.2023.1226044
70. Zhang Y, Liu X, Zeng L, Zhao X, Chen Q, Pan Y, et al. Exosomal protein angiopoietin-like 4 mediated radioresistance of lung cancer by inhibiting ferroptosis under hypoxic microenvironment. Br J Cancer. (2022) 127:1760–72. doi: 10.1038/s41416-022-01956-7
71. Wang S, Chen S, Ying Y, Ma X, Shen H, Li J, et al. Comprehensive analysis of ferroptosis regulators with regard to PD-L1 and immune infiltration in clear cell renal cell carcinoma. Front Cell Dev Biol. (2021) 9:676142. doi: 10.3389/fcell.2021.676142
72. Galvan I, Inacio A, Danino M, Corbi-Llopis R, Monserrat MT, Bernabeu-Wittel J. High SLC7A11 expression in normal skin of melanoma patients. Cancer Epidemiol. (2019) 62:101582. doi: 10.1016/j.canep.2019.101582
73. Wang W, Green M, Choi JE, Gijon M, Kennedy PD, Johnson JK, et al. CD8(+) T cells regulate tumour ferroptosis during cancer immunotherapy. Nature. (2019) 569:270–4. doi: 10.1038/s41586-019-1170-y
74. Kong R, Wang N, Han W, Bao W, Lu J. IFNgamma-mediated repression of system xc(-) drives vulnerability to induced ferroptosis in hepatocellular carcinoma cells. J Leukoc Biol. (2021) 110:301–14. doi: 10.1002/JLB.3MA1220-815RRR
75. Zheng Y, Sun L, Guo J, Ma J. The crosstalk between ferroptosis and anti-tumor immunity in the tumor microenvironment: molecular mechanisms and therapeutic controversy. Cancer Commun (London England). (2023) 43:1071–96. doi: 10.1002/cac2.12487
76. Zhang D, Man D, Lu J, Jiang Y, Ding B, Su R, et al. Mitochondrial TSPO promotes hepatocellular carcinoma progression through ferroptosis inhibition and immune evasion. Adv Sci (Weinh). (2023) 10:e2206669. doi: 10.1002/advs.202206669
77. Cui S, Ghai A, Deng Y, Li S, Zhang R, Egbulefu C, et al. Identification of hyperoxidized PRDX3 as a ferroptosis marker reveals ferroptotic damage in chronic liver diseases. Mol Cell. (2023) 83:3931–9 e5. doi: 10.1016/j.molcel.2023.09.025
78. Tang B, Xu W, Wang Y, Zhu J, Wang H, Tu J, et al. Identification of critical ferroptosis regulators in lung adenocarcinoma that RRM2 facilitates tumor immune infiltration by inhibiting ferroptotic death. Clin Immunol (Orlando Fla). (2021) 232:108872. doi: 10.1016/j.clim.2021.108872
79. Tabnak P, HajiEsmailPoor Z, Soraneh S. Ferroptosis in lung cancer: from molecular mechanisms to prognostic and therapeutic opportunities. Front Oncol. (2021) 11:792827. doi: 10.3389/fonc.2021.792827
80. Deng B, Xiang J, Liang Z, Luo L. Identification and validation of a ferroptosis-related gene to predict survival outcomes and the immune microenvironment in lung adenocarcinoma. Cancer Cell Int. (2022) 22:292. doi: 10.1186/s12935-022-02699-4
81. Li L, Wang B. One ferroptosis-related gene-pair signature serves as an original prognostic biomarker in lung adenocarcinoma. Front Genet. (2022) 13:841712. doi: 10.3389/fgene.2022.841712
82. Wu Y, Lin Z, Tang X, Tong Z, Ji Y, Xu Y, et al. Ferroptosis-related gene HIC1 in the prediction of the prognosis and immunotherapeutic efficacy with immunological activity. Front Immunol. (2023) 14:1182030. doi: 10.3389/fimmu.2023.1182030
83. Zhang T, Sun B, Zhong C, Xu K, Wang Z, Hofman P, et al. Targeting histone deacetylase enhances the therapeutic effect of Erastin-induced ferroptosis in EGFR-activating mutant lung adenocarcinoma. Trans Lung Cancer Res. (2021) 10:1857–72. doi: 10.21037/tlcr-21-303
84. Yuan B, Liao F, Shi ZZ, Ren Y, Deng XL, Yang TT, et al. Dihydroartemisinin inhibits the proliferation, colony formation and induces ferroptosis of lung cancer cells by inhibiting PRIM2/SLC7A11 axis. Onco Targets Ther. (2020) 13:10829–40. doi: 10.2147/OTT.S248492
85. Zhang Q, Yi H, Yao H, Lu L, He G, Wu M, et al. Artemisinin derivatives inhibit non-small cell lung cancer cells through induction of ROS-dependent apoptosis/ferroptosis. J Cancer. (2021) 12:4075–85. doi: 10.7150/jca.57054
86. Iida Y, Okamoto-Katsuyama M, Maruoka S, Mizumura K, Shimizu T, Shikano S, et al. Effective ferroptotic small-cell lung cancer cell death from SLC7A11 inhibition by sulforaphane. Oncol letters. (2021) 21:71. doi: 10.3892/ol.2020.12332
87. Xia X, Fan X, Zhao M, Zhu P. The relationship between ferroptosis and tumors: A novel landscape for therapeutic approach. Curr Gene Ther. (2019) 19:117–24. doi: 10.2174/1566523219666190628152137
88. Guo J, Xu B, Han Q, Zhou H, Xia Y, Gong C, et al. Ferroptosis: A novel anti-tumor action for cisplatin. Cancer Res Treat. (2018) 50:445–60. doi: 10.4143/crt.2016.572
89. Yang H, Zhao L, Gao Y, Yao F, Marti TM, Schmid RA, et al. Pharmacotranscriptomic analysis reveals novel drugs and gene networks regulating ferroptosis in cancer. Cancers. (2020) 12:3272. doi: 10.3390/cancers12113273
90. Xu R, Wu J, Luo Y, Wang Y, Tian J, Teng W, et al. Sanguinarine represses the growth and metastasis of non-small cell lung cancer by facilitating ferroptosis. Curr Pharm Design. (2022) 28:760–8. doi: 10.2174/1381612828666220217124542
91. Wu W, Geng Z, Bai H, Liu T, Zhang B. Ammonium Ferric Citrate induced Ferroptosis in Non-Small-Cell Lung Carcinoma through the inhibition of GPX4-GSS/GSR-GGT axis activity. Int J Med Sci. (2021) 18:1899–909. doi: 10.7150/ijms.54860
92. Wu CY, Yang YH, Lin YS, Chang GH, Tsai MS, Hsu CM, et al. Dihydroisotanshinone I induced ferroptosis and apoptosis of lung cancer cells. Biomed Pharmacother. (2021) 139:111585. doi: 10.1016/j.biopha.2021.111585
93. Yang L, Chen X, Yang Q, Chen J, Huang Q, Yao L, et al. Broad spectrum deubiquitinase inhibition induces both apoptosis and ferroptosis in cancer cells. Front Oncol. (2020) 10:949. doi: 10.3389/fonc.2020.00949
94. Zhai FG, Liang QC, Wu YY, Liu JQ, Liu JW. Red ginseng polysaccharide exhibits anticancer activity through GPX4 downregulation-induced ferroptosis. Pharm Biol. (2022) 60:909–14. doi: 10.1080/13880209.2022.2066139
95. Freire Boullosa L, Van Loenhout J, Flieswasser T, De Waele J, Hermans C, Lambrechts H, et al. Auranofin reveals therapeutic anticancer potential by triggering distinct molecular cell death mechanisms and innate immunity in mutant p53 non-small cell lung cancer. Redox Biol. (2021) 42:101949. doi: 10.1016/j.redox.2021.101949
96. Zhou W, Zhang J, Yan M, Wu J, Lian S, Sun K, et al. Orlistat induces ferroptosis-like cell death of lung cancer cells. Front Med. (2021) 15:922–32. doi: 10.1007/s11684-020-0804-7
97. Palmer LD, Jordan AT, Maloney KN, Farrow MA, Gutierrez DB, Gant-Branum R, et al. Zinc intoxication induces ferroptosis in A549 human lung cells. Metallomics. (2019) 11:982–93. doi: 10.1039/c8mt00360b
98. Chen P, Wu Q, Feng J, Yan L, Sun Y, Liu S, et al. Erianin, a novel dibenzyl compound in Dendrobium extract, inhibits lung cancer cell growth and migration via calcium/calmodulin-dependent ferroptosis. Signal Transduct Target Ther. (2020) 5:51. doi: 10.1038/s41392-020-0149-3
99. Meng M, Huang M, Liu C, Wang J, Ren W, Cui S, et al. Local anesthetic levobupivacaine induces ferroptosis and inhibits progression by up-regulating p53 in non-small cell lung cancer. Aging. (2021) 13. doi: 10.18632/aging.203138
100. Shao M, Jiang Q, Shen C, Liu Z, Qiu L. Sinapine induced ferroptosis in non-small cell lung cancer cells by upregulating transferrin/transferrin receptor and downregulating SLC7A11. Gene. (2022) 827:146460. doi: 10.1016/j.gene.2022.146460
101. Bow YD, Ko CC, Chang WT, Chou SY, Hung CT, Huang JL, et al. A novel quinoline derivative, DFIQ, sensitizes NSCLC cells to ferroptosis by promoting oxidative stress accompanied by autophagic dysfunction and mitochondrial damage. Cancer Cell Int. (2023) 23:171. doi: 10.1186/s12935-023-02984-w
102. Jiaqi L, Siqing H, Qin W, di Z, Bei Z, Jialin Y. Andrographolide promoted ferroptosis to repress the development of non-small cell lung cancer through activation of the mitochondrial dysfunction. Phytomedicine. (2023) 109:154601. doi: 10.1016/j.phymed.2022.154601
103. Han B, Liu Y, Zhang Q, Liang L. Propofol decreases cisplatin resistance of non-small cell lung cancer by inducing GPX4-mediated ferroptosis through the miR-744-5p/miR-615-3p axis. J proteomics. (2023) 274:104777. doi: 10.1016/j.jprot.2022.104777
104. Feng S, Li Y, Huang H, Huang H, Duan Y, Yuan Z, et al. Isoorientin reverses lung cancer drug resistance by promoting ferroptosis via the SIRT6/Nrf2/GPX4 signaling pathway. Eur J Pharmacol. (2023) 954:175853. doi: 10.1016/j.ejphar.2023.175853
105. Lou JS, Zhao LP, Huang ZH, Chen XY, Xu JT, Tai WC, et al. Ginkgetin derived from Ginkgo biloba leaves enhances the therapeutic effect of cisplatin via ferroptosis-mediated disruption of the Nrf2/HO-1 axis in EGFR wild-type non-small-cell lung cancer. Phytomedicine. (2021) 80:153370. doi: 10.1016/j.phymed.2020.153370
106. Liang Z, Zhao W, Li X, Wang L, Meng L, Yu R. Cisplatin synergizes with PRLX93936 to induce ferroptosis in non-small cell lung cancer cells. Biochem Biophys Res Commun. (2021) 569:79–85. doi: 10.1016/j.bbrc.2021.06.088
107. Lai XY, Shi YM, Zhou MM. Dihydroartemisinin enhances gefitinib cytotoxicity against lung adenocarcinoma cells by inducing ROS-dependent apoptosis and ferroptosis. Kaohsiung J Med Sci. (2023) 39:699–709. doi: 10.1002/kjm2.12684
108. Liu M, Fan Y, Li D, Han B, Meng Y, Chen F, et al. Ferroptosis inducer erastin sensitizes NSCLC cells to celastrol through activation of the ROS-mitochondrial fission-mitophagy axis. Mol Oncol. (2021) 15:2084–105. doi: 10.1002/1878-0261.12936
109. Gai C, Yu M, Li Z, Wang Y, Ding D, Zheng J, et al. Acetaminophen sensitizing erastin-induced ferroptosis via modulation of Nrf2/heme oxygenase-1 signaling pathway in non-small-cell lung cancer. J Cell Physiol. (2020) 235:3329–39. doi: 10.1002/jcp.29221
110. Xu C, Jiang ZB, Shao L, Zhao ZM, Fan XX, Sui X, et al. β-Elemene enhances erlotinib sensitivity through induction of ferroptosis by upregulating lncRNA H19 in EGFR-mutant non-small cell lung cancer. Pharmacol Res. (2023) 191:106739. doi: 10.1016/j.phrs.2023.106739
111. Freire Boullosa L, Van Loenhout J, Flieswasser T, Hermans C, Merlin C, Lau HW, et al. Auranofin synergizes with the PARP inhibitor olaparib to induce ROS-mediated cell death in mutant p53 cancers. Antioxidants (Basel Switzerland). (2023) 12:667. doi: 10.3390/antiox12030667
112. Almahi WA, Yu KN, Mohammed F, Kong P, Han W. Hemin enhances radiosensitivity of lung cancer cells through ferroptosis. Exp Cell Res. (2022) 410:112946. doi: 10.1016/j.yexcr.2021.112946
113. Pan X, Lin Z, Jiang D, Yu Y, Yang D, Zhou H, et al. Erastin decreases radioresistance of NSCLC cells partially by inducing GPX4-mediated ferroptosis. Oncol Lett. (2019) 17:3001–8. doi: 10.3892/ol.2019.9888
114. Ye LF, Chaudhary KR, Zandkarimi F, Harken AD, Kinslow CJ, Upadhyayula PS, et al. Radiation-induced lipid peroxidation triggers ferroptosis and synergizes with ferroptosis inducers. ACS Chem Biol. (2020) 15:469–84. doi: 10.1021/acschembio.9b00939
115. Vu NT, Kim M, Stephenson DJ, MacKnight HP, Chalfant CE. Ceramide kinase inhibition drives ferroptosis and sensitivity to cisplatin in mutant KRAS lung cancer by dysregulating VDAC-mediated mitochondria function. Mol Cancer Res MCR. (2022) 20:1429–42. doi: 10.1158/1541-7786.MCR-22-0085
116. Ishida T, Takahashi T, Kurokawa Y, Nishida T, Hirota S, Serada S, et al. Targeted therapy for drug-tolerant persister cells after imatinib treatment for gastrointestinal stromal tumours. Br J Cancer. (2021) 125:1511–22. doi: 10.1038/s41416-021-01566-9
117. Jiang Z, Lim SO, Yan M, Hsu JL, Yao J, Wei Y, et al. TYRO3 induces anti-PD-1/PD-L1 therapy resistance by limiting innate immunity and tumoral ferroptosis. J Clin Invest. (2021) 131:e139434. doi: 10.1172/JCI139434
118. Mao W, Cai Y, Chen D, Jiang G, Xu Y, Chen R, et al. Statin shapes inflamed tumor microenvironment and enhances immune checkpoint blockade in non-small cell lung cancer. JCI Insight. (2022) 7:e161940. doi: 10.1172/jci.insight.161940
119. Wei F, Ke L, Gao S, Karges J, Wang J, Chen Y, et al. In situ oxidative polymerization of platinum(iv) prodrugs in pore-confined spaces of CaCO(3) nanoparticles for cancer chemoimmunotherapy. Chem Sci. (2023) 14:7005–15. doi: 10.1039/D3SC02264A
120. Zhu G, Chi H, Liu M, Yin Y, Diao H, Liu Z, et al. Multifunctional "ball-rod" Janus nanoparticles boosting Fenton reaction for ferroptosis therapy of non-small cell lung cancer. J Colloid Interface Sci. (2022) 621:12–23. doi: 10.1016/j.jcis.2022.04.021
121. Chen X, Yang Y, Ye G, Liu S, Liu J. Chiral ruthenium nanozymes with self-cascade reaction driven the NO generation induced macrophage M1 polarization realizing the lung cancer "Cocktail therapy". Small (Weinheim an der Bergstrasse Germany). (2023) 19:e2207823. doi: 10.1002/smll.202207823
122. Wang J, Yang W, He X, Zhang Z, Zheng X. Assembling p53 activating peptide with ceO(2) nanoparticle to construct a metallo-organic supermolecule toward the synergistic ferroptosis of tumor. Front Bioeng Biotechnol. (2022) 10:929536. doi: 10.3389/fbioe.2022.929536
123. Li Y, Yang J, Gu G, Guo X, He C, Sun J, et al. Pulmonary delivery of theranostic nanoclusters for lung cancer ferroptosis with enhanced chemodynamic/radiation synergistic therapy. Nano letters. (2022) 22:963–72. doi: 10.1021/acs.nanolett.1c03786
Keywords: lung cancer, ferroptosis, combination therapy, biomarkers, nanomedicine
Citation: Li Y, Li X and Li J (2024) Ferroptosis in lung cancer: dual role, multi-level regulation, and new therapeutic strategies. Front. Oncol. 14:1360638. doi: 10.3389/fonc.2024.1360638
Received: 23 December 2023; Accepted: 26 February 2024;
Published: 07 March 2024.
Edited by:
Giorgio Scagliotti, Department of Oncology - University of Torino, ItalyReviewed by:
Michael Epperly, University of Pittsburgh, United StatesHailiang Hu, Southern University of Science and Technology, China
Copyright © 2024 Li, Li and Li. This is an open-access article distributed under the terms of the Creative Commons Attribution License (CC BY). The use, distribution or reproduction in other forums is permitted, provided the original author(s) and the copyright owner(s) are credited and that the original publication in this journal is cited, in accordance with accepted academic practice. No use, distribution or reproduction is permitted which does not comply with these terms.
*Correspondence: Jian Li, Leesward@163.com
†These authors have contributed equally to this work
‡ORCID: Xiaosong Li, orcid.org/0009-0003-2389-449X