- 1Institute of Immunology, Centre for Infection Medicine, Freie Universität Berlin, Berlin, Germany
- 2Department of Medical Microbiology and Parasitology, Kenyatta University, Nairobi, Kenya
- 3Kenya Medical Research Institute, Centre for Global Health Research, Kisumu, Kenya
- 4Department of Medical Laboratory Science, Kenyatta University, Nairobi, Kenya
- 5Eastern and Southern Africa Centre of International Parasite Control, Kenya Medical Research Institute, Nairobi, Kenya
The most important soil-transmitted helminths (STHs) affecting humans are roundworms, whipworms, and hookworms, with a large proportion of the world’s population infected with one or more of these intestinal parasites. On top of that, concurrent infections with several viruses, bacteria, protozoa, and other helminths such as trematodes are common in STH-endemic areas. STHs are potent immunomodulators, but knowledge about the effects of STH infection on the direction and extent of coinfections with other pathogens and vice versa is incomplete. By focusing on Kenya, a country where STH infections in humans are widespread, we provide an exemplary overview of the current prevalence of STH and co-occurring infections (e.g. with Human Immunodeficiency Virus, Plasmodium falciparum, Giardia duodenalis and Schistosoma mansoni). Using human data and complemented by experimental studies, we outline the immunomechanistic interactions of coinfections in both acutely STH transmigrated and chronically infected tissues, also highlighting their systemic nature. Depending on the coinfecting pathogen and immunological readout, STH infection may restrain, support, or even override the immune response to another pathogen. Furthermore, the timing of the particular infection and host susceptibility are decisive for the immunopathological consequences. Some examples demonstrated positive outcomes of STH coinfections, where the systemic effects of these helminths mitigate the damage caused by other pathogens. Nevertheless, the data available to date are rather unbalanced, as only a few studies have considered the effects of coinfection on the worm’s life cycle and associated host immunity. These interactions are complex and depend largely on the context and biology of the coinfection, which can act in either direction, both to the benefit and detriment of the infected host.
1 Introduction
Humans are susceptible to more than 1,200 pathogens, including viruses, bacteria, helminths, protozoa, and fungi. Coinfection with multiple pathogens is common and can result in a range of interactions, either beneficial or antagonistic for the host. Soil-transmitted helminths (STHs) are potent immunomodulators, yet evidence on the effects of STH infections on the direction and extent of concurrent infections with other pathogens is inconsistent. STHs refer to intestinal worms that infect humans and animals alike, and are transmitted through contaminated soil (Figure 1). A large proportion of the world’s population is infected with one or more of these STHs: ~807-1,121 million people with roundworms (Ascaris lumbricoides), ~604-795 million with whipworms (Trichuris trichiura), and ~576-740 million with hookworms (Ancylostoma duodenale and Necator americanus) (Centers for Disease Control and Prevention, 2022).
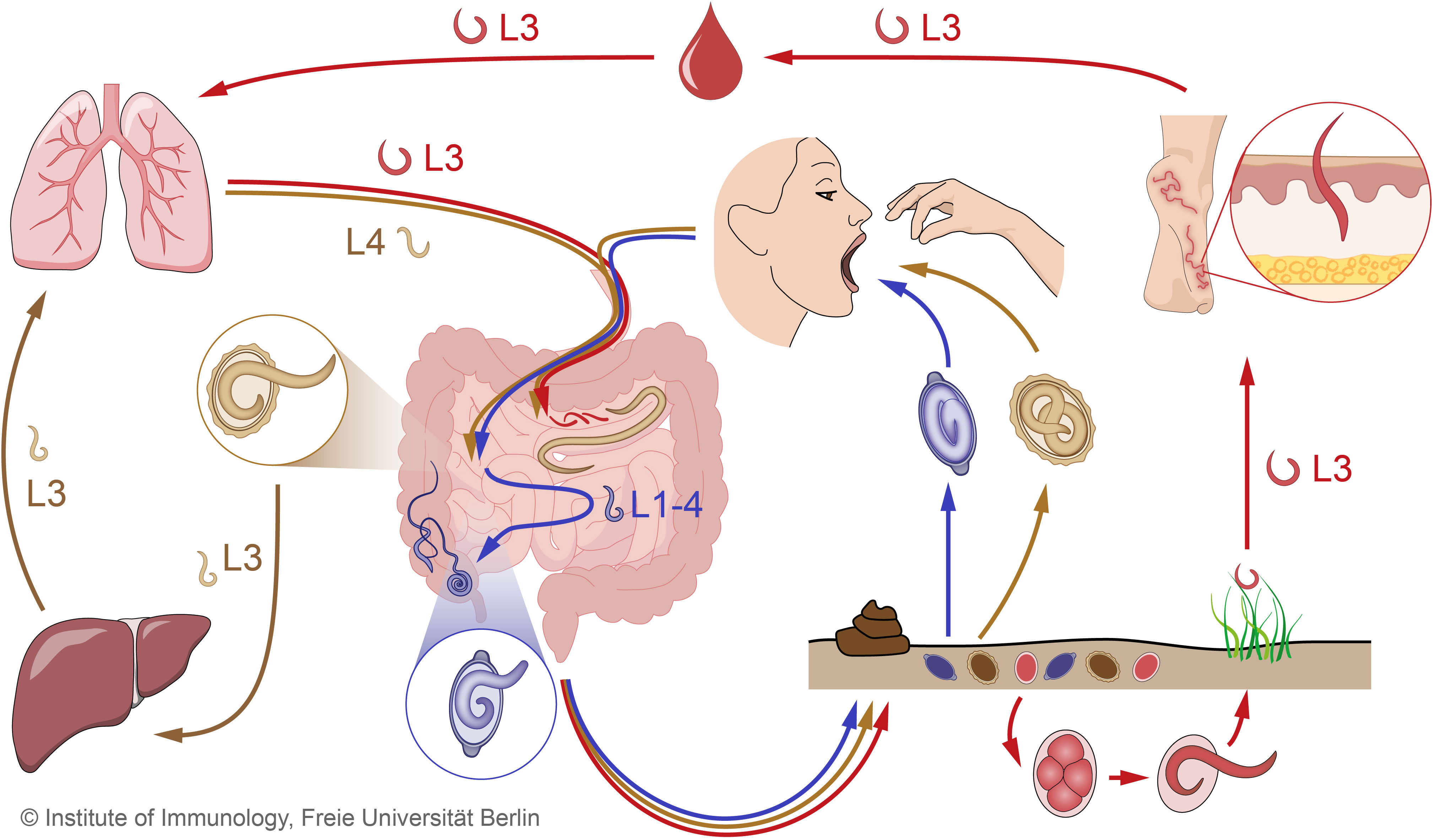
Figure 1 Combined STH life cycles for hookworms, Trichuris trichiura and Ascaris lumbricoides. Unembryonated eggs of all three STHs are excreted in the feces and develop into different larval stages over time in the soil. The infective eggs of A. lumbricoides (beige) and T. trichiura (purple), which contain the 3rd and 1st larval stages (L3, L1), respectively, enter the small intestine after oral ingestion, where the larvae hatch. In the case of hookworm eggs (red), the larvae hatch while still in the environment and develop into infective L3. When hookworm L3 come into contact with the human host, they penetrate the skin and are transported through the blood vessels to the heart and then to the lungs. Similar to hookworms, Ascaris larvae undergo extensive tissue migration. The L3 enter the proximal parts of the colon and migrate to the liver via the portal blood stream before reaching the lungs via systemic circulation. In the lungs, Ascaris L3 and hookworm L3 enter the alveoli, ascend the bronchial tree to the pharynx, and are swallowed. Ascaris L4 and hookworm L3 enter the small intestine, where they mature into adult worms. Adult Ascaris worms live exclusively in the lumen of the small intestine, while hookworms attach to the intestinal wall to feed on blood. Trichuris larvae mature intestinally without body migration and settle as adults in the colon, where they penetrate the mucosa with the anterior part. In the intestine, adult females of all three STHs produce eggs that are shed with the feces. STH, soil-transmitted helminth.
STH infections occur primarily in areas with warm and humid climates where sanitation and hygiene are inadequate. STHs are considered neglected tropical diseases (NTDs), causing tremendous disability and suffering but can be controlled or eliminated. Coinfections of STHs with other pathogens (e.g. viruses, bacteria, protozoa, other helminths) represent an important clinical and epidemiological problem, especially in children suffering from high parasite burdens. In many cases, the pathogenic mechanisms of these coinfections are not fully understood, and the results obtained are inconclusive. The biology and current disease burden, pathogenesis, and efforts to combat STH were recently reviewed (Loukas et al., 2021). This review highlights the epidemiology of STH coinfections with a focus on Kenya, where the high disease burden was recently documented in nationwide surveys of the prevalence of STH and other infectious diseases. Human data will be complemented by studies in animal models to outline the various mechanisms by which helminths and associated immune responses interact with different microbial pathogens and other helminth species. Prevalence and mechanistic data concerning coinfections with schistosomes, a group of helminths not belonging to the STHs, were included where appropriate. The review concludes with a discussion on therapeutic interventions and future directions.
1.1 Epidemiology of STH and coinfections in Kenya
1.1.1 Prevalence of STH infections
Low- and middle-income countries are particularly burdened by infectious diseases caused by pathogens such as helminths, viruses, bacteria, and protozoa (Bygbjerg, 2012). In Kenya, STH infections are common and most prevalent in western and coastal regions (Figure 2). In STH endemic areas in Kenya, multiple infections with different pathogens are very likely, although the prevalence of coinfections and their interaction are often poorly studied. The majority of published data on STH prevalence are from school-aged children, collected mainly through the National School-Based Deworming (NSBD) Program in Kenya. Notably, the STH non-endemic regions in Kenya are not covered by the NSBD Program due to the anticipated low prevalence and transmission rates (Pullan et al., 2011; Okoyo et al., 2016), hence there is a conspicuous lack of STH prevalence data for these regions from both the NSBD Program and other studies. The most recent NSBD survey reported an overall STH prevalence of 12.9% among children aged 4-15 years from six regions of Kenya in 2020 (Okoyo et al., 2020). In the same period, A. lumbricoides was the most prevalent STH species at 9.7%, while T. trichiura and hookworms accounted for 3.6% and 1.0%, respectively. Other individual studies in children of similar ages reported STH prevalences of up to 44.05% and 20.9% in Western and Coastal Kenya, respectively, with A. lumbricoides and hookworm infections predominating (Ngonjo et al., 2016; Halliday et al., 2019). Notably, STH prevalence in Coastal Kenya for 2020 was slightly higher among children aged 4-15 years using precision mapping (Kepha et al., 2023), highlighting the importance of the sampling method in assessing STH prevalence. Although preschool-aged children and adults may act as reservoirs for STH transmission, there are limited data on STH prevalence in these age groups in Kenya. The reported prevalence of STH among preschool children in Busia County, Western Kenya, was 17.0% in 2020 (Masaku et al., 2020). A recent study found an STH prevalence of 12.4% among pregnant women in Western Kenya (Araka et al., 2022). With the introduction of mass drug administration (MDA) programs in several helminth endemic countries, the prevalence of severe STH infections has gradually declined to moderate and mild infections (Mwandawiro et al., 2019). Nevertheless, the actual STH prevalence in Kenya may be slightly higher than reported because most STH prevalence studies use the Kato-Katz method for screening, which provides low sensitivity to low infection intensities (Okoyo et al., 2018).
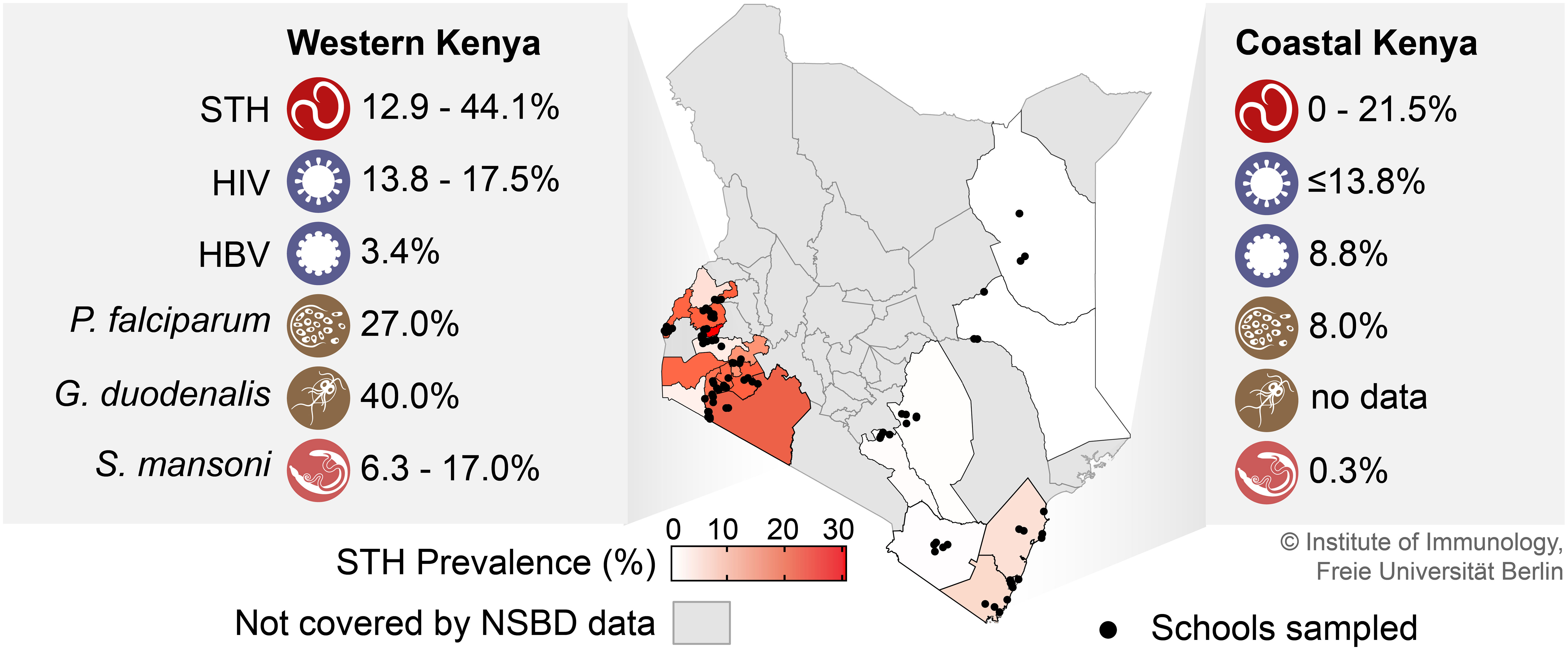
Figure 2 STH prevalence in Kenyan counties and co-occurring infections in areas of high STH prevalence (Western and Coastal Kenya). Prevalence data shown here for STH and S. mansoni infections were adapted from the 2018 survey for the National School-Based Deworming (NSBD) Program in Kenya (Okoyo et al., 2020) complemented with data from (Ngonjo et al., 2016; Halliday et al., 2019; Masaku et al., 2020; Araka et al., 2022; Kepha et al., 2023). The prevalence data for the co-occuring viral infections were taken from (Walson et al., 2010; Storey et al., 2017; Awili et al., 2020; Onyango et al., 2021; Wahome et al., 2022), and for the co-occurring protozoan infections from (Kepha et al., 2015; Pickering et al., 2019; Division of National Malaria Programme (DNMP) [Kenya] and ICF, 2021; Kamau et al., 2021). NSBD, National School-Based Deworming Program; STH, soil-transmitted helminth; HIV, human immunodeficiency virus; HBV, hepatitis B virus; P. falciparum, Plasmodium falciparum; G. duodenalis, Giardia duodenalis; S. mansoni, Schistosoma mansoni.
1.1.2 Prevalence of STH-virus coinfections
While there is extensive knowledge about the burden of helminth and viral infections, relatively little is known about the combinations of helminth and viral infections in Kenya. Here, due to the high burden of HIV/AIDS in STH endemic regions, most studies have focused on the prevalence of STH-HIV coinfection, while data on coinfection with other viruses, particularly enteric, respiratory and hepatotropic viruses, are largely lacking. HIV infection rates in Kenya are highly heterogeneous across the country, with prevalence in Kisumu, Western Kenya, at 17.5%, more than three times the national average (Onyango et al., 2021). Two studies of STH-virus coinfection in Kenya have reported a prevalence of 15.5% to 19.3% (Walson et al., 2010; Storey et al., 2017); however, there were regional differences in the prevalence of STH-HIV coinfection, ranging from 13.8% in the rural coastal and western regions to 44.7% in the urban regions of Nairobi (Walson et al., 2010). Regarding the most common STHs found in HIV-infected individuals, A. lumbricoides was the most common in the majority of studies, followed by hookworms and T. trichiuria. Worldwide, hepatitis A virus (HAV), hepatitis B virus (HBV), and hepatitis C virus (HCV) account for most cases of viral hepatitis, with hepatitis E virus (HEV) emerging and often being underdiagnosed (Shin et al., 2016; Kamar et al., 2017). In particular, the burden of HBV remains enormous in Kenya, with a recent meta-analysis finding an estimated overall prevalence of 7.8% (Makokha et al., 2023). In highly STH endemic regions, HBV prevalence ranged from 3.4% in Western Kenya (Awili et al., 2020) to 8.8% in Coastal Kenya (Wahome et al., 2022). However, despite the clustered regional occurrence, there have been no studies of STH coinfection with hepatotropic viruses in Kenya.
1.1.3 Prevalence of STH-bacteria coinfections
The Global Burden of Disease Study found an estimated 7.7 million deaths, or 13.6% of all global deaths, were associated with bacterial pathogens in 2019 (Ikuta et al., 2022). Importantly, Kenya was listed as a high burden tuberculosis state (World Health Organization, 2016), with a reported prevalence of bacteriologically confirmed pulmonary TB of 558 per 100,000 population (Enos et al., 2018). Furthermore, different parts of Kenya have experienced multiple cholera outbreaks (Mutonga et al., 2013; Kiama et al., 2023), and many cases of infectious diarrhea were linked to bacterial enteropathogens, including diarrheagenic Escherichia coli, Shigella, Salmonella, and others (Shah et al., 2016). While there are a number of publications reporting the prevalence of STH in Kenya, few have examined the prevalence of bacteria in the same studies. A literature search on helminth-bacterial coinfections in Kenya did not reveal a single study. The closest study examined the effects of anthelminthic treatment on the gut microbiota. This study revealed that the presence of A. lumbricoides or N. americanus did not alter the overall microbiota composition compared to age-matched controls (Easton et al., 2019). Consequently, the prevalence and impact of STH-bacteria coinfections in this country remain unknown.
1.1.4 Prevalence of STH-protozoa coinfections
Similar to STH, Plasmodium falciparum is also endemic in Western and Coastal Kenya. Here, the prevalence of malaria is 27.0% in the western region and 8% in the coastal region (Division of National Malaria Programme (DNMP) [Kenya] and ICF, 2021). The prevalence of STH-P. falciparum coinfection among school children in Bungoma in Western Kenya was at 14.3%, with the majority of malaria infections reported asymptomatic (Kepha et al., 2015). In addition to STH infections, Kisumu County, also in the western region of Kenya, reported a prevalence of 18% Schistosoma mansoni-P. falciparum coinfections among adults aged 18-35 years (Kamau et al., 2021). The prevalence of Giardia among children aged 2-5 years in the western region was at 40% (Pickering et al., 2019), whereas no data on Giardia prevalence are available for the coastal region of Kenya.
1.1.5 Prevalence of STH coinfections with other helminths
Other helminths such as S. mansoni are also common in Western and Coastal Kenya with prevalence of 6.3% and 0.3%, respectively, although prevalence in western counties of Kenya can be as high as 17.0% depending on the distance from water bodies (Masaku et al., 2020; Okoyo et al., 2020). Despite the widespread geographic overlap of various STHs and other helminths in Kenya, most studies of STH prevalence in Kenya generally report the prevalence of infections with a single STH species. One study in Western Kenya found a coinfection prevalence of 4.7% among schoolchildren with hookworms and A. lumbricoides (Kepha et al., 2015). Studies in the elderly population show a relatively lower prevalence of STH coinfection with other helminths, likely due to the expected lower prevalence of STH in adults. One such study among women of childbearing age in Kwale County, Coastal Kenya, found a prevalence of Schistosoma haematobium and STH coinfection of 0.4% (Jeza et al., 2022).
1.2 Mechanisms of immunity to STHs
Gut-dwelling STHs modify the microenvironment of the gastrointestinal tract, including the epithelial cell layer and immune cells located in the underlying stroma, but also the surrounding microbiota (Zaph et al., 2014; Harris and Loke, 2018; Loke et al., 2022). In addition, certain STHs, such as A. lumbricoides, A. duodenale, and N. americanus, undergo an extraintestinal phase in which larvae migrate through different tissues before developing into sexually mature adult worms in the intestine (Vacca and Le Gros, 2022).
Following host invasion, worm-induced barrier damage leads to the release of alarmins (TSLP, IL-25, IL-33) from epithelial, mesenchymal, and innate cells (Hepworth et al., 2012; Peng et al., 2022). These early events drive the activation and proliferation of innate immune cells, such as mast cells, dendritic cells, and type 2 innate lymphoid cells (ILC2s), promoting the induction of an adaptive type 2 (Th2) immune response (Peng et al., 2022). In parallel, epithelial tuft cells have recently been shown to be important regulators of the host response to various infections in the gut (Strine and Wilen, 2022). Tuft cells respond to helminth infection by releasing IL-25, which drives the release of IL-5, IL-13, and IL-4 by activated ILC2s (Colombo and Grencis, 2020; Hendel et al., 2022). Subsequently, Th2 cells and ILC2s amplify IL-4 and IL-13 signaling to activate host protective responses at the epithelial barrier, including tuft cell differentiation, hyperplasia of mucin-producing goblet cells, and increased epithelial cell turnover (Colombo and Grencis, 2020). IL-4/-13 and IL-5 produced by Th2 cells also promote the expansion of alternatively activated macrophages (AAM) and the recruitment of eosinophils into affected tissues (Peng et al., 2022). In the gut, IL-4 and IL-13 increase smooth muscle hypercontractility, embodying the “weep-and-sweep” response that eliminates luminal parasites (Sorobetea et al., 2018). Furthermore, IL-4 derived from follicular T-helper cells contributes to effective anti-parasitic immunity in promoting IgG and IgE production by B cells (Zaini et al., 2021). Immune mechanisms directed to STH infections are summarized in Figure 3.
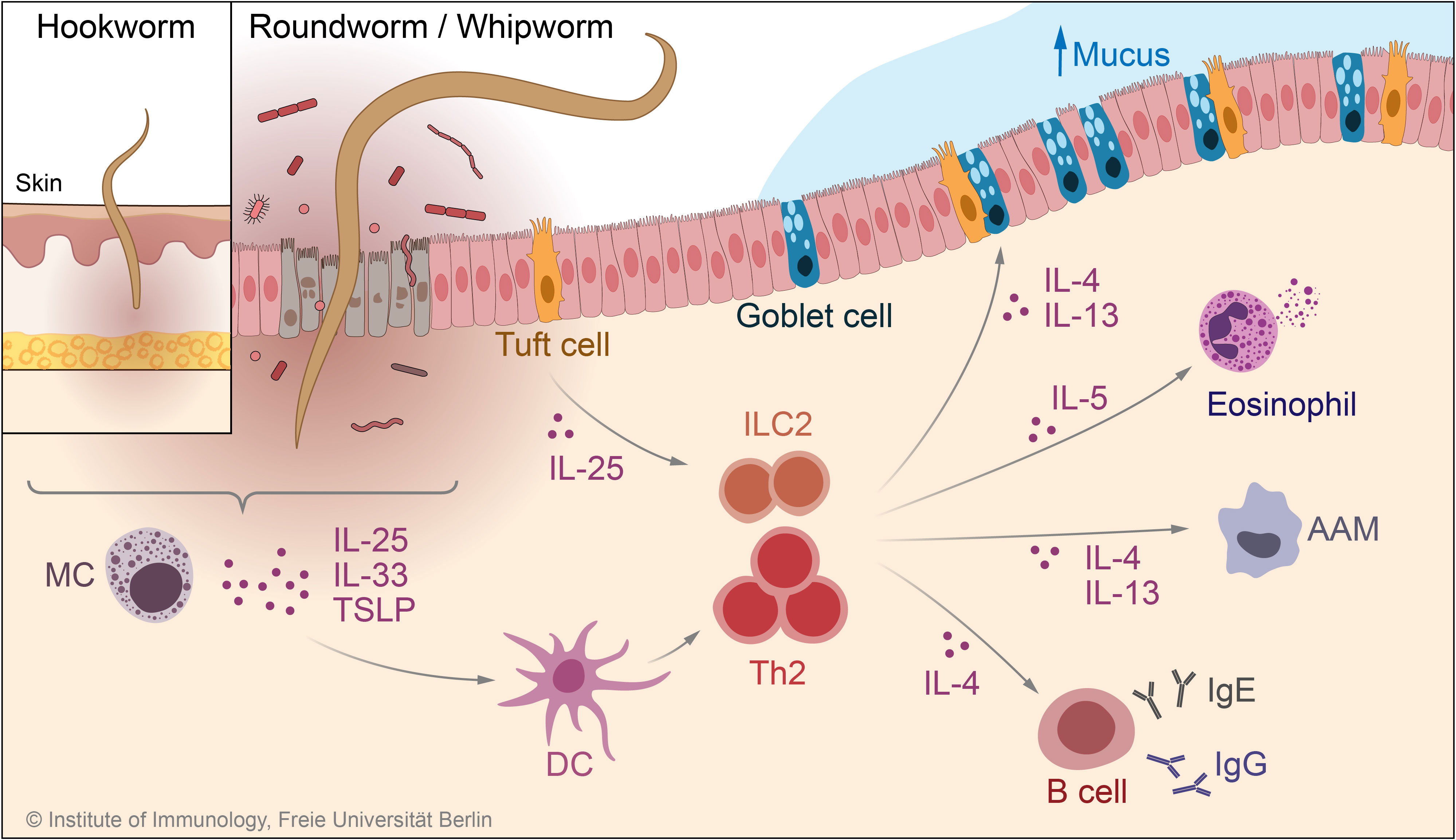
Figure 3 Immune mechanisms in response to infection with STHs. During anti-STH immunity, epithelial cell- and mast cell-derived cytokines such as TSLP and IL-33, together with tuft cell-derived IL-25, lead to activation of the type 2 response by ILC2 and Th2 cells. Upon activation, these cells produce the type 2 cytokines IL-4, IL-5, and IL-13, which in turn activate effector mechanisms involved in the defense against worm infection, such as goblet cell hyperplasia and, increased mucus production, eosinophilia, and IgE/IgG production by B cells. MC, mast cell; TSLP, thymic stromal lymphopoietin; DC, dendritic cell; AAM, alternatively activated macrophage; ILC2, type 2 innate lymphoid cell.
2 Affected host tissues and immunomodulation by STH coinfections
The type of antiparasitic immune response and tissue damage by migrating larval stages of STH are likely to be key elements in determining the outcome of concurrent viral, bacterial, protozoan or helminth infections. As an example, helminths and viruses elicit different innate and adaptive immune signaling pathways that may antagonize each other (Maizels and Gause, 2014; Desai et al., 2021; Midha et al., 2021). Furthermore, worms interfere with the host immune response through the release of immunomodulatory molecules, creating an environment beneficial to the parasite. Immunomodulation may act at the expense of antimicrobial immunity, but in some circumstances may also reduce the immunopathology caused by the microbe. However, simultaneous inflammatory processes driven by coinfections in the same organ system may also exacerbate tissue damage and thus directly affect tissue integrity. In this context, the timing of coinfection relative to the stage of the helminth life cycle, tissue tropism, interaction with the host microbiota and the extent of helminth-driven type 2/regulatory responses play key roles in determining the outcome of coinfection. Nevertheless, the frequency of these coinfections and associated comorbidities and the mechanisms of interactions in humans are poorly understood. Access to organ material is a limiting factor, and studies in animal models can provide valuable mechanistic information in this regard.
In the following sections, human-relevant STH coinfections with viruses, bacteria, protozoa, and other helminths are discussed according to the major organ systems affected, with a particular focus on the most important emerging and reemerging diseases occurring in Africa (Karagiannis-Voules et al., 2015; Fenollar and Mediannikov, 2018; Chauhan et al., 2020; Siwila et al., 2020; Afolabi et al., 2022; Nyaruaba et al., 2022). Where available, human studies are supplemented with data from animal models.
3 STH coinfections with viruses
In endemic areas, coinfections of helminths with certain viral diseases are common, causing different pathologies and associated immune responses depending on the helminth species, viral cell tropism and the timing of coinfection (Desai et al., 2021). Despite the frequent co-occurrence of STHs and viral infections, there is little information on their interactions when coinfected in humans.
3.1 STHs and enteric viruses
Worldwide, and thus also in helminth endemic areas, enteric viruses such as noroviruses and rotaviruses are the main cause of severe acute gastroenteritis in children under five years of age (Djeneba et al., 2007; Potgieter et al., 2023). Reinfection is common, with virus-specific IgA correlating with protection and increasing over time, whereas IFN-γ-dominated specific T cell responses are transient (Malm et al., 2019). However, the effects of STH coinfection with intestinal viruses in humans are not well understood. In mice, acute enteric helminth infections with Heligmosomoides polygyrus and Trichinella spiralis resulted in impaired virus-specific CD8+ T cell responses (Osborne et al., 2014). This defect was STAT6-dependent, associated with the nematode-triggered alternative activation of macrophages, and was partially restored by neutralization of Ym-1, a product released by AAM (Osborne et al., 2014). Another study reported the increased enteric replication of flaviviruses in H. polygyrus-infected mice which was associated with a tuft cell-IL-4 receptor signaling axis leading to dampening of antiviral adaptive immunity (Desai et al., 2021). Associated with goblet cell hyperplasia during murine H. polygyrus coinfection, murine astrovirus replication and shedding was also enhanced (Ingle et al., 2021).
3.2 STHs and hepatotropic viruses
HAV, HBV, and HCV are responsible for most cases of viral hepatitis, with HEV emerging and often being underdiagnosed (Shin et al., 2016; Kamar et al., 2017). Thus, a correlation between human infections with A. lumbricoides and HBV has been demonstrated (Xiao et al., 2015), but the effects on correlated immune responses and liver pathology are unknown. To date, studies addressing the potential impact of helminth infections on the controls of hepatotropic viruses focused on patients coinfected with schistosomes. Interestingly, HEV seroprevalence was very high among Egyptian workers and a Brazilian cohort infected with schistosomes (el-Esnawy and Al-Herrawy, 2000; Passos-Castilho et al., 2016). Here, previous HEV infection was associated with a higher frequency of liver enzyme abnormalities (Passos-Castilho et al., 2016), indicating long-lasting effects of HEV. Similarly, concurrent infections with HBV and HCV in trematode endemic areas are often observed (Abruzzi et al., 2016; Gao et al., 2020). Related to increased Th2 cytokines, patients with concurrent helminth and HBV or HCV infection showed increased viral replication and liver fibrosis (Abruzzi et al., 2016; Li et al., 2016; Dong et al., 2022). Hence, chronic inflammatory processes associated with hepatic viral replication next to Th2-mediated fibrotic responses to Schistosome eggs may result in more extensive liver damage caused mainly by immune-mediated mechanisms (Shin et al., 2016). Furthermore, the modulation of early intrahepatic antiviral immune pathways (e.g. type I IFN) in murine helminth-virus coinfection was shown to be associated with increased viral replication in the liver (Edwards et al., 2005). Whether recurrent tissue damage induced by migrating Ascaris larvae has a similar effect remains to be investigated. Furthermore, it is unclear whether tissue damage induced by blood-feeding hookworm species may affect the immune response to hepatic viruses. A. lumbricoides, A. duodenale and N. americanus, have an extraintestinal phase in which the larvae migrate through various tissues before developing into sexually mature adult worms in the gut. The liver, as an organ that preferentially induces tolerance to immunity, is passed by Ascaris during its life cycle before the larvae reach the lungs with liver larvae attrition occurring under certain circumstances (Midha et al., 2021). This function can be exploited by parasites to evade host immunity. Thus, hepatotropic viruses that exploit similar strategies preferentially infect hepatocytes.
3.3 STHs and respiratory viruses
Tissue-invasive STHs that migrate through the lungs evoke inflammatory changes and tissue damage that can negatively impact concurrent respiratory viral infections or even pave the way for secondary infections. Respiratory syncytial virus (RSV) is the most common cause of acute respiratory diseases in children worldwide (Gatt et al., 2023). An imbalance in the Th1/Th2 cytokine immune response has been associated with the RSV pathogenesis of bronchiolitis (Pinto et al., 2006). Thus, Th1/Th2 ratios, helminth-specific-IgE and TLR4-gene-polymorphisms are related to infant RSV disease severity in parasite-endemic areas (Caballero et al., 2015; Buendía et al., 2022). Research is also underway to determine how coinfection with STHs can influence inflammatory immune activity in SARS-CoV-2 infection and thus disease severity, but also the efficacy of vaccination against SARS-CoV-2 (Adjobimey et al., 2022; Akelew et al., 2022). Interestingly, the prevalence and mortality due to COVID-19 have remained moderate on the African continent (Cepon-Robins and Gildner, 2020). However, Ascaris antigens affected immune reactivity to SARS-CoV-2 peptides by reducing virus-reactive Th cells and type 1 cytokines in COVID-19 patients, suggesting a negative impact of concurrent helminth infestation on antiviral immunity (Adjobimey et al., 2022). In line, Ascaris-infected pigs were shown to be more susceptible to respiratory virus infection (Oba et al., 2023). Indicating a bidirectional interaction, pulmonary Ascaris and vaccinia virus coinfection in mice weakened virus-specific immunity and not only exacerbated virus-associated pathology, but also reduced lung larval loads (Gazzinelli-Guimarães et al., 2017). However, in addition to the detrimental effects in STH coinfections, strictly enteric helminth infection with H. polygyrus protected mice from severe pulmonary RSV infection by microbiota-dependent type I interferon production, with Th2 responses dispensable (McFarlane et al., 2017).
3.4 STHs and viruses with immune cell tropism
Herpesviruses have a marked tropism for immune cells and latently infected cells remain for life with virus reactivation occurring under certain circumstances (Lan and Luo, 2017). Because of their high prevalence, many people are coinfected with herpesviruses and STHs, and although both pathogens modulate the host’s immunity (Peacock et al., 2003; Barton et al., 2007; Lan and Luo, 2017), their interactions in humans remain largely unexplored. The local cytokine environment during acute helminth infection alters latent herpesvirus infection, whereby IL-4 reactivated human Kaposi’s sarcoma-associated herpesvirus from latency in cultured human cells (Reese et al., 2014). The extent of immunity to helminth infection may be relevant here, as no reactivation of latent Epstein-Barr virus infection was observed in multiple sclerosis patients who underwent low-dose therapeutic oral hookworm administration (Maple et al., 2020). A “two-signal” model for viral reactivation was postulated, as exogenous IL-4 and IFN-γ-blockade reactivated latent murine gammaherpesvirus-68 (MHV68) infection in vivo (Reese et al., 2014), which required macrophage IL-4-receptor-expression. Accordingly, Nippostrongylus brasiliensis infected mice developed type 2 immune-driven enhanced pathology upon genital herpesvirus infection (Chetty et al., 2021), a finding also translatable to humans, since hookworm-infected women in an African cohort were at high risk for viral infections in the genital tract (Holali Ameyapoh et al., 2021). However, IL-4 also has beneficial effects on IFN-γ-dependent antiviral effector responses, as helminth-mediated (N. brasiliensis, H. polygyrus) conditioning of virtual memory CD8+ T cells (TVMs) enhanced control of murine gammaherpesvirus 4 (MHV-4) infection (Hussain et al., 2023). Of note, this helminth-mediated increase in TVMs is transient and absent in aged mice (Hussain et al., 2023) which may be particularly relevant to herpesvirus reactivation in elderly worm-infected patients.
Human retroviruses such as HIV cause damage to lymphoid and mucosal tissues, leading to progressive immunodeficiency (Heron and Elahi, 2017). Sub-Saharan Africa is burdened by HIV/AIDS and helminthiasis, and there is considerable overlap between these infections, with poor sanitation and socioeconomic status influencing the occurrence of HIV and STH coinfections (Mpaka-Mbatha et al., 2023). Compared to HIV-infected patients in Africa, adults coinfected with HIV and intestinal helminths (e.g. hookworms) have been found to have increased susceptibility to micronutrient deficiencies and anaemia, as well as higher viral loads and lower CD4+ T cell counts (Mkhize-Kwitshana et al., 2011; Morawski et al., 2017; Amoo et al., 2018; Wondmieneh et al., 2020). According to two studies in South Africa, HIV- and A. lumbricoides-coinfected individuals with high Ascaris-specific-IgE levels and eosinophilia displayed the highest viral loads and reduced levels of type 1 cytokines, indicating a suppressive effect of helminth-mediated immunity on HIV control (Mkhize-Kwitshana et al., 2011; Mkhize-Kwitshana et al., 2014). However, findings are conflicting, as serum cytokine profiling of HIV- and STH-coinfected pregnant women in Nigeria revealed increased IFN-γ and IL-17 levels (Rabiu et al., 2022). In line, Peruvian patients coinfected with another retrovirus, HTLV-1, and Strongyloides stercoralis have higher parasite burdens and lower type 2 immune responses than patients with strongyloidiasis alone (Montes et al., 2009).
In conclusion, STH coinfections with viruses can have profound effects on the outcome of each infection through the following interactions (Figure 4A): i.) STHs facilitate viral replication by remodeling tissue morphology and counteracting classical IFN-γ/TNF-mediated type 1 inflammatory pathways due to initiated type 2 immune circuits; ii.) Depending on the timing and extent of virus-induced inflammation, an unfavorable environment for migrating STH larvae is created, which may compromise the development of patent worm infection; iii.) STHs may also exert direct type 2 cytokine-mediated and indirect microbiota-dependent remote effects in non-infested organs, resulting in enhanced antiviral immunity that protects against virus-related pathology. Thus, infections with STH can alter host immunity and tissue integrity both directly in parasitized organs and indirectly in uninfected organs, and therefore have systemic effects that are highly complex and appear to depend largely on the context and biology of viral coinfection in a bidirectional manner.
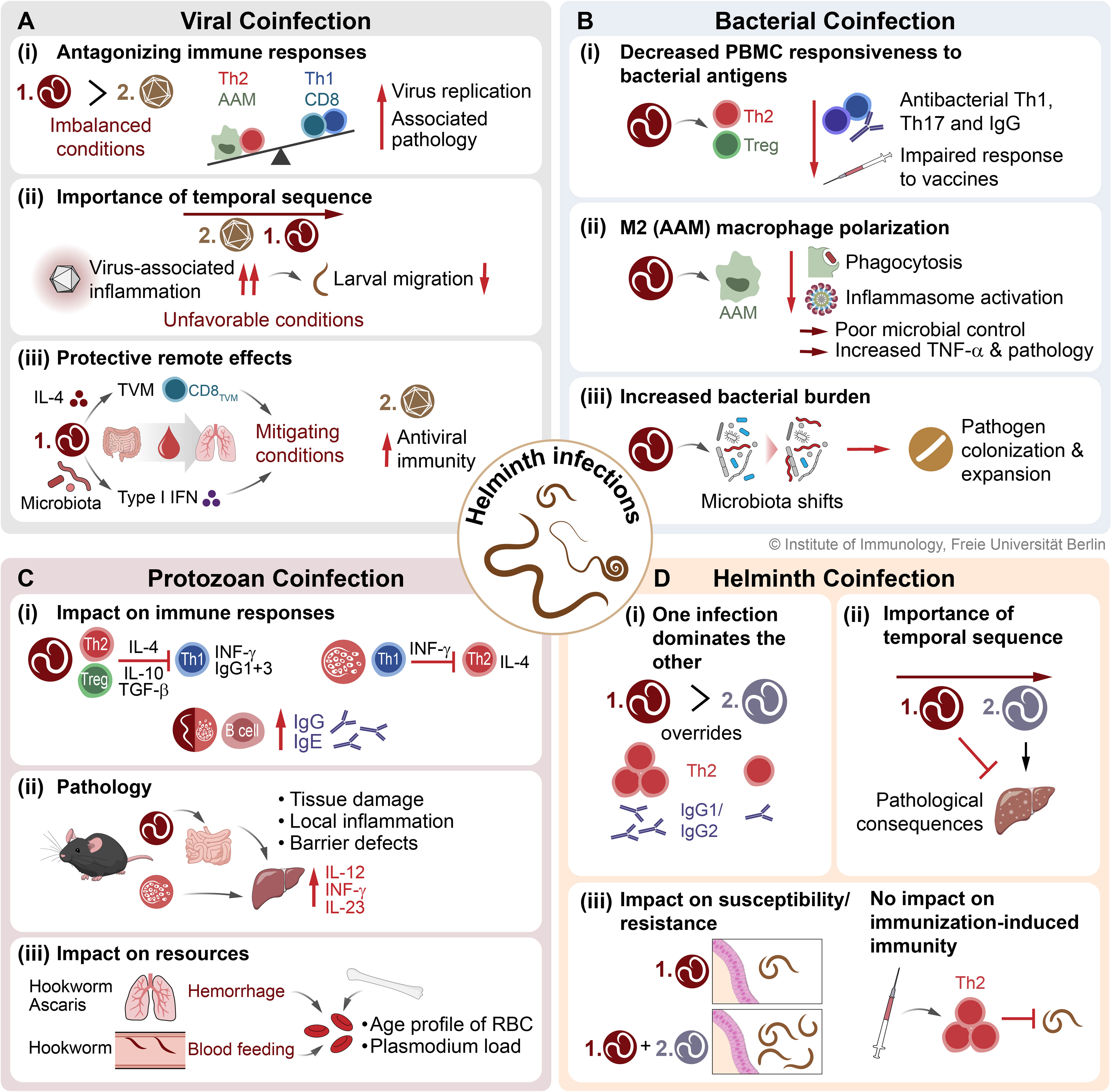
Figure 4 The consequences of STH coinfections on host immunity and infection outcome. Here, the interactions and associated effects on immune responses and pathologies of STH coinfections with (A) viruses, (B) bacteria, (C) protozoa (exemplary for Plasmodium), and (D) other helminths are presented. STH, soil-transmitted helminth; TVM, virtual memory CD8+ T cell; AAM, alternatively activated macrophage; CAM, classically activated macrophage; Th1, type 1 T helper cell; Th2, type 2 T helper cell; Treg, regulatory T cell; RBC, red blood cell.
4 STH coinfections with bacteria
Interactions between helminths and bacteria can occur at the site of infection in the intestine, in sites impacted by helminth tissue migration such as the lungs, or indirectly via the microbiota. STHs migrating through and residing in the intestine are surrounded by microbes and numerous studies have documented alterations to the host microbiota composition during helminth infections as well as direct interactions between STHs and microbes reviewed in (Midha et al., 2017; Midha et al., 2021). Here we consider data concerning enteric pathogens such as Salmonella spp. which may also become systemic in the case of typhoid fever, and respiratory pathogens such as Mycobacterium tuberculosis.
4.1 STHs and enteric bacteria
As with enteric viruses, coinfections with STHs and enteric bacteria, some of which share the fecal-oral route of transmission, are also widespread. Between human and animal studies of STH-bacterial coinfections as well as data from other helminths such as vector-borne filarial nematodes and trematodes such as Schistosoma, there is considerable evidence for helminth immunomodulation leading to increased bacterial burdens and pathology (Rocha et al., 1971; Gendrel et al., 1994; Bobat et al., 2014; Reynolds et al., 2017). The outcomes of coinfection can also be influenced by the pathogens in question as well as by timing. Mice coinfected with S. mansoni and Listeria monocytogenes displayed increased resistance to Listeria infection early in Schistosoma infection, and decreased resistance to bacterial infection later in the course of Schistosoma infection (Collins et al., 1972).
Infection with A. lumbricoides is negatively associated with IFN-γ and IL-7 production in response to LPS as demonstrated in Tsimane hunter-horticulturalists (Schneider-Crease et al., 2021). This negative association is also associated with impaired responses to bacterial vaccines. PBMCs from teenage Ecuadorian patients infected with A. lumbricoides had impaired cholera toxin B-specific IL-2 and IFN-γ responses following vaccination with CVD 103-HgR, a live attenuated Vibrio cholerae strain used for cholera vaccination (Cooper et al., 2001). Interestingly, anthelmintic treatment of the same ascariasis patients enhanced the vibriocidal antibody response (Cooper et al., 2000). In pigs, A. suum infection negatively affects the protective response of Mycoplasma hypopneumoniae vaccination, including impaired seroconversion and antibody titres, while increasing lung pathology (Steenhard et al., 2009).
Clinical observations indicate that coinfection with Schistosoma and Salmonella is quite common; helminth-infected Gabonese children and rural Congolese villagerss were more often found to carry Salmonella (Gendrel et al., 1994; Mbuyi-Kalonji et al., 2020) and schistosomiasis patients were found to suffer from prolonged bacteremia and salmonellosis (Rocha et al., 1971), a situation which can be improved by anthelmintic treatment (Hathout et al., 1966; Gendrel et al., 1984). These observations are well supported by coinfection studies in mice where Schistosoma-Salmonella coinfected mice have higher bacterial burdens and increased mortality compared to mice infected only with Salmonella (Njunda and Oyerinde, 1996). Importantly, similar outcomes are observed in STH-Salmonella coinfection, including with N. brasiliensis (Bobat et al., 2014) and H. polygyrus (Reynolds et al., 2017) wherein helminth-impaired host resistance to Salmonella is restored by deworming (Brosschot et al., 2021).
Protective responses against Salmonella are typically initiated by bacterial recognition via patterns-associated molecular patterns such as lipopolysaccharide (LPS), type-3 secretion system proteins, flagella, fimbriae, and bacterial DNA combined with endogenous danger-associated molecular patterns, leading to neutrophil and macrophage recruitment and pro-inflammatory cytokine secretion, including IL-1β, IL-6, IL-17, TNF-α, and IFN-γ (de Jong et al., 2012). Innate antibacterial responses by neutrophils and macrophages can be influenced by helminth infection. Su and colleagues demonstrated that H. polygyrus can worsen intestinal inflammation during S. Typhimurium infection by compromising neutrophil recruitment and control of bacterial replication (Su et al., 2014). Furthermore, helminth antigens trigger IL-4 and IL-13 release by basophils leading to alternative activation of macrophages, impaired IFN-γ and IL-17 production, and thus impaired anti-Salmonella protective Th1/Th17 responses (Knuhr et al., 2018; Schramm et al., 2018). Similar observations have been made in mice coinfected with H. polygyrus and Citrobacter rodentium, a gram-negative model to investigate enteropathogenic and enterohaemorrhagic E. coli (EPEC and EHEC, respectively) infections and colitis (Collins et al., 2014), where coinfected mice display considerable morbidity and mortality (Chen et al., 2005) and higher bacterial loads compared to single-infected mice (Weng et al., 2007). The Th2-driven alternative activation of macrophages resulted in downregulated protective IFN-γ and corresponding impaired bacterial killing alongside worsened colitis mediated by enhanced TNF-α responses (Chen et al., 2005; Weng et al., 2007). H. polygyrus infection can also promote S. Typhimurium infection independently of a modulated Th2 response. Reynolds and colleagues demonstrated the host intestinal metabolome was altered by H. polygyrus to enhance the expression of Salmonella pathogenicity island 1 genes, leading to increased bacterial invasion (Reynolds et al., 2017).
Interestingly, bacterial infection can also impact parasite burdens. Mice coinfected with S. japonicum and S. Typhimurium had reduced adult worm burdens and reduced mortality compared to S. japonicum infection alone (Zhu et al., 2017). In these mice, Schistosoma infection was associated with increased serum IFN-γ and IL-4 levels compared to uninfected controls while coinfection further increased IFN-γ, but reduced IL-4 to uninfected levels (Zhu et al., 2017). Another important consideration is the order of infection. In the case of N. brasiliensis-S. Typhimurium coinfection, an established helminth infection impairs control of subsequent bacterial infection while simultaneous coinfection impaired protective immunity to both pathogens despite robust antibody responses (Bobat et al., 2014). Interestingly, Salmonella infection restrained the regulatory response induced by N. brasiliensis as reflected by reduced IL-10 secretion by splenocytes (Bobat et al., 2014).
4.2 STHs and respiratory bacteria
The lung is a major site of immunomodulation with an impact on coinfecting bacteria. Clinical outcomes of helminth-respiratory bacterial coinfection are mixed though there is ample evidence to suggest that as with intestinal infections, helminth immunomodulation alters responses to respiratory pathogens as well. Higher prevalence of helminth infections have been reported in Ethiopian and Brazilian patients with active tuberculosis (TB) compared to matched controls (Tristão-Sá et al., 2002; Elias et al., 2006) as well as eosinophilia, a prominent feature of helminth infections (Elliott et al., 2003). Urban Indian adults coinfected with Strongyloides stercoralis and M. tuberculosis have higher circulating levels of matrix metalloproteinases and tissue inhibitors of metalloproteinases compared to M. tuberculosis single infection, indicating that coinfection could result in greater degradation of the basement membrane thereby creating an immune privileged site for the growth of M. tuberculosis (Kathamuthu et al., 2021). In one study, STHs, namely Ascaris and Trichuris, were identified as a risk factor for increased pneumococcal carriage density in Ecuadorian children (Law et al., 2021) In the same report, T. muris-infected mice coinfected with Streptococcus pneumoniae had increased pneumococcal carriage density in the nasopharynx and enhanced bacterial dissemination into the lungs (Law et al., 2021). Another study found coinfection with H. polygyrus and Bordatella bronchiseptica led to higher bacterial loads in the lungs and higher mortality (Lass et al., 2013). Similarly, mice coinfected with A. suum (at day 8 post-infection during A. suum’s lung migration) and Pasteurella multocida developed more severe pneumonia and septicemia than did mice infected with bacteria alone (Tjørnehøj et al., 1992), suggesting that while STHs are actively migrating through the lungs, the host may be at an increased risk for bacterial coinfection.
As with Salmonella, resistance to mycobacterial infection is initiated with inflammatory responses by phagocytes triggered by pattern recognition receptors and control is mediated by Th1 and Th17 cells while the progression of tuberculosis is associated with the regulatory type-2 cytokines IL-4 and IL-10 (Babu and Nutman, 2016; Cadmus et al., 2020; Bustamante-Rengifo et al., 2021; Bhengu et al., 2022). Numerous studies have documented helminth-impaired reactivity to tuberculin antigens and compromised tuberculosis diagnostics (Babu and Nutman, 2016). Specifically, helminth infection appears to dampen the efficacy of Bacille Calmette-Guerin (BCG) vaccination, an attenuated strain of Mycobacterium bovis, and helminth infection can reduce mycobacterial-induced IFN-γ production in Bangladeshi children and pregnant Ethiopian mothers (Cadmus et al., 2020; Bhengu et al., 2022). S. stercoralis-M. tuberculosis coinfections in Indian adults are associated with lower circulating levels of inflammatory cytokines, including IFN-γ, TNF-α, IL-17A, and IL-17F alongside increased type 2 cytokines IL-4, IL-5, and IL-13 (George et al., 2015). Similarly, Indian TB patients coinfected with hookworms had fewer Mycobacterium-specific Th1 and Th17 cells and associated circulating cytokines (TNF-α, IFN-γ, IL-2, IL-17A) but increased Th2 cells and associated cytokines (IL-4, IL-13) as well as increased frequencies of regulatory T cells compared to patients infected with M. tuberculosis alone (George et al., 2013).
In conclusion, helminth coinfections can modulate antibacterial immune responses against bacterial pathogens in different organs. A general picture emerges whereby a helminth-induced regulatory type 2 response dampens cellular responses to bacteria and their antigens, polarizes macrophages away from an antibacterial proinflammatory state, and alters antibody responses against bacteria, thus establishing an environment better suited to the proliferation of bacterial pathogens (Figure 4B).
5 STH coinfections with protozoa
5.1 STH and malaria infection
Malaria as well as STH infections remain highly endemic in sub-Saharan Africa. A number of studies investigated the potential impact of STH infection on malaria prevalence, infection intensity and the associated immunopathology in humans as well as in experimental animal models. Several studies reported that infection with A. lumbricoides, T. trichiura, and hookworms were associated with an increased risk for the development of clinical, non-severe malaria (Nacher et al., 2002; Spiegel et al., 2003; Degarege et al., 2012; Zeukeng et al., 2014; Babamale et al., 2018), which was further increased with the number of coinfecting intestinal helminth species (Nacher et al., 2002; Degarege et al., 2012). Similarly, studies performed in murine models reported higher peak parasitemia in mice coinfected with H. polygyrus or N. brasiliensis (Su et al., 2005; Noland et al., 2008; Helmby, 2009; Hoeve et al., 2009; Segura et al., 2009; Tetsutani et al., 2009), and, in some cases, accelerated mortality associated with uncontrolled liver pathology (Helmby, 2009; Craig and Scott, 2017). By contrast, studies in humans found a protective effect of Ascaris and hookworm infection against the development of severe malaria as well as malaria-related acute renal failure (Nacher et al., 2000; Nacher et al., 2001). Timing is important for the outcome of GI nematode/Plasmodium coinfection in mice. Nematode infection one to two weeks prior to infection with P. chabaudi had limited impact on the course of Plasmodium infection, whereas simultaneous coinfection led to a dramatic rise in mortality, associated with highly increased liver damage. The latter was dependent on the elevated expression of IL-12, IFN-γ and IL-23 in coinfected mice (Helmby, 2009). Increased mortality coincided with larval-driven intestinal barrier damage, suggesting that danger signals reaching the liver via the portal system promoted overt hepatic inflammation when mice were simultaneously coinfected with Plasmodium parasites (Helmby, 2009).
Several studies reported impaired IFN-γ responses in coinfected mice, arguing for a detrimental effect of type 2 responses driven by nematode coinfection (Su et al., 2005; Noland et al., 2008; Tetsutani et al., 2009). However, peak parasitemia was also increased in coinfected STAT-6-/- mice incapable of inducing a Th2 response to H. polygyrus (Segura et al., 2009). Importantly, a placebo-controlled deworming trial in Indonesia reported increased immune responsiveness after anthelmintic treatment (Wammes et al., 2016). Regular deworming resulted in lower expression of the suppressive molecule CTLA-4 by T cells, coinciding with significantly increased Plasmodium-specific TNF-α/IFN-γ and elevated Ascaris-specific IL-2 production (Wammes et al., 2016).
While some studies determined a higher risk for anemia and low body weight in STH coinfected patients (Degarege et al., 2010; Zeukeng et al., 2014), others found no effect on P. falciparum-induced anemia (Abanyie et al., 2013; Babamale et al., 2018). Investigating experimental infections in mice, Griffith and colleagues reported suppressed immune responses, yet smaller Plasmodium populations in mice coinfected with N. brasiliensis (Griffiths et al., 2015). In this model, target cell availability exerted a stronger effect on the dynamics of malaria replication than suppression of IFN-γ and IgG2a responses, potentially linked to altered mean age and population size of red blood cells in mice afflicted with hemorrhage induced during migration of nematode larvae through the lung. Considering resource-mediated next to immune-mediated mechanisms may hence be essential in understanding the dynamics of helminth-Plasmodium coinfections (Griffiths et al., 2015).
Th1 responses associated with the production of cytophilic IgG antibodies (IgG1, IgG3) are important for the clearance of Plasmodium blood-stage infection (Torre et al., 2002; Courtin et al., 2009). By contrast, helminth infections predominantly cause the production of IgG4 and IgE in a Th2-dependent manner (Harris and Gause, 2011). Accordingly, several studies reported a negative effect of concurrent helminth infection on the humoral response to malaria, seen in the reduction of P. falciparum-specific IgG (Ateba-Ngoa et al., 2016), IgG1 and IgG3, and a rise in IgG4 (Roussilhon et al., 2010; Courtin et al., 2011). On the other hand, two studies found higher IgG1, IgG2 and IgG3 responses to P. falciparum antigens in schistosome coinfected individuals (Diallo et al., 2010; Tokplonou et al., 2020). Similarly, Amoani and colleagues reported a positive effect of hookworm coinfection on the levels of IgG3 directed against the malaria vaccine candidate GMZ2 at baseline and a decline in the antibody level after albendazole treatment (Amoani et al., 2021). Another recent study surveyed antibody responses directed against a broad array of Plasmodium as well as STH antigens in Mozambican children and found consistently higher IgG levels against Plasmodium and nematode antigens as well as higher total IgE levels in the coinfected group (Santano et al., 2021). In trend, the study confirmed earlier reports of higher Plasmodium density in coinfected children and found significantly higher Trichuris worm burdens in malaria-positive subjects. Finally, socioeconomic score and improved sanitary conditions clearly impacted overall IgE levels and IgG levels against some Plasmodium and helminth antigens (Santano et al., 2021). Hence, synergies may exist between the Th2-related support of antibody production in helminth infection and humoral responses directed against Plasmodium parasites. However, as the risk of being infected and Plasmodium density tend to increase with helminth coinfection, it seems that such synergistic effects reflect the increased exposure rather than protection of coinfected patients. Although the gut microbiota may play a role in Plasmodium infections and contribute to the complexity of coinfection with STH (Easton et al., 2020), further studies are needed to specify this interaction.
In conclusion, there are at least three potential interactions between helminths and Plasmodium coinfection (Figure 4C): i) By the induction of Th2 responses, STH coinfection may interfere with Th1/IFN-γ driven effector/cytophilic IgG1/IgG3 responses required for the control of Plasmodium infection. Conversely, a strong Th1-bias may hamper effective type-2 driven control of STH infections. However, coinfection was also reported to be associated with enhanced parasite-specific IgG and IgE responses compared to single infections; ii) Some animal models suggest that simultaneous tissue damage driven by malaria parasites and helminths may result in an adverse inflammatory response, worsening disease outcome. However, STH infection rather seems to protect from severe malaria in humans; iii) Coinfection with Ascaris/hookworms is associated with hemorrhage in the liver during lung stage infection. In addition, adult hookworms feed on host blood. This may alter the age profile of erythrocytes and thereby limit resources for Plasmodium species favoring matured blood cells for infection.
5.2 STH and giardia infection
Giardia duodenalis colonizes the small intestinal tract where the trophozoite stage attaches to epithelial cells. The infection is often asymptomatic, but may also be associated with diarrheal disease, leading to malabsorption and, under chronic exposure, stunted growth (Klotz and Aebischer, 2015; Fekete et al., 2021). Experimental Giardia infections are controlled by Th17 and IgA responses and elevated Th17 activity was also linked with protective immunity against human giardiasis (Dann et al., 2015; Saghaug et al., 2016; Paerewijck et al., 2019). Despite the fact that Giardia shares the small intestinal habitat with Ascaris, hookworms and threadworms, relatively few studies tested for potential interactions between enteric nematode and Giardia infections. Furthermore, although the prevalence of Giardia infection is often high in areas where STH and/or schistosome infections are highly endemic (Fofana et al., 2019; Geus et al., 2019; Archer et al., 2020), data on potential interference of immune responses directed against the two types of parasites are largely lacking.
A survey of Plasmodium, Ascaris and Giardia infection in Rwandan schoolchildren showed that parasitic coinfections were common, but the clinical picture was mostly associated with Plasmodium infection and not modified by Ascaris or Giardia coinfection. However, malnutrition was more pronounced in children coinfected with Ascaris and Giardia compared to individuals with single infections (Geus et al., 2019). Similarly, a study in subtropical Argentina observed an association between stunting in older children and the presence of enteric parasites, multi-parasitism and giardiasis (Rivero et al., 2018). A long-term survey of over 80 villages in Bolivia revealed an antagonistic relationship between STH and Giardia infection (Blackwell et al., 2013). Both hookworm and Ascaris infection were negatively associated with Giardia infection in a cross-sectional as well as in a longitudinal survey. However, anthelminthic treatment only resulted in a marginal rise of the risk of being infected with Giardia on the next visits (Blackwell et al., 2013). Concerning modifications of the immune profile, one study reported reduced IL-2, IL-12 and TNF-α serum levels in 3-year old children coinfected with Ascaris and Giardia compared with Giardia mono-infected individuals, while Th2 cytokine levels were not different from the controls in any of the groups (Weatherhead et al., 2017). Finally, high Giardia-specific IgG and IgE levels were seen in a cohort affected by light, but not moderate Ascaris infection and high IL-10 levels in individuals with moderate Ascaris infection associated with poor inflammatory cytokine responses against Giardia antigens (Hagel et al., 2011).
Hence, evidence of the detrimental effects of STH on Giardia infection and the associated sequelae exists. However, more work in experimental models is needed addressing immunological cross-regulation, competition for resources and potential changes in barrier functions, mucus composition and gut microbial communities in the context of STH-Giardia coinfections.
6 STH coinfections with other helminths
Coinfections with Ascaris, whipworms and hookworms as well as schistosomes are common in endemic areas, especially among children in regions with poor socioeconomic status, low hygiene and poor sanitation (Pullan et al., 2008).
6.1 Host tissues affected by helminth-helminth coinfections
The life cycles of STHs differ significantly between the major species. The larval stages of Ascaris and hookworm species undergo extensive migration through the body. Ascaris larvae hatch in the intestine followed by migration via the liver and lung before being coughed up and swallowed. Upon maturation, the worms reproduce in the small intestine (Figure 1). Thus, in Ascaris infection the small intestine, the liver and the lungs are dominantly affected and inflammation as well as cellular remodeling and modulation are detectable (Midha et al., 2021). In contrast, hookworm larvae invade the host through the skin and reach the blood circulation which they leave in the lungs (Figure 1). Like Ascaris larvae, hookworm larvae are then coughed up and swallowed, reaching finally the small intestine. Similar to Ascaris, hookworm larvae migrate through the body and thus affect the skin, blood circulation, the lungs and the small intestine of infected hosts. In comparison, whipworm infections in humans occur when the eggs are ingested, as is the case with Ascaris infections. The larvae hatch in the small intestine and adult worms establish and dwell in the large intestine (Figure 1). Whipworms do not show a body migration, the organ primarily affected is the intestine. However, intriguingly, intestinal worms without body migration also do affect the entire mucosal system and anti-helminth effector and memory responses are clearly detectable in the lungs (Yordanova et al., 2022).
6.2 Influence of helminth-helminth coinfections on the host immune response
Although multiple helminths are detected in infected individuals (Donohue et al., 2019), data on host immunological consequences of helminth coinfections are sparse. Some studies in animal models with poly-helminth infections indicate significant immunological effects of the second worm infection. For instance, in pigs coinfected with T. suis and Oesophagostomum dentatum the O. dentatum-specific IgG1 and IgG2 levels in poly-infected pigs were two-fold higher than in O. dentatum mono-infected pigs (Andreasen et al., 2015). Thereby, the Trichuris infection enhanced the typically weak response induced by O. dendatum. These data were underpinned in addition by a stronger Th2 response, measured by parasite-specific IL-4 secreting cells in PBMCs and associated gene expression (IL-13, ARG1, CCL11) at the site of infection as well as by peripheral eosinophil counts. Thus, one helminth infection overrides the coinfecting second worm infection (Figure 4D). A prominent effect was also demonstrated in a coinfection with the nematode H. polygyrus and the trematode S. mansoni in mice. Here, a pre-infection with H. polygyrus resulted in a marked reduction of hepatic egg-induced granulomatous inflammation associated with dampened proinflammatory cytokines in mice coinfected with S. mansoni (Bazzone et al., 2008). Thereby a dominant Th2-polarized environment induced by one infection ameliorated the immunopathology induced by the second helminth infection (Figure 4D). Another study in mice using coinfections of T. muris and H. polygyrus showed that coinfections are capable of changing susceptibility to infection (Colombo et al., 2022). The authors observed a complete impairment of T. muris expulsion in immunocompetent mice when coinfected with H. polygyrus. Interestingly, this drastic effect was not coupled with a change in parasite-specific cytokine production in draining lymph nodes or mucosal barrier immune responses in coinfected mice. However, changed susceptibility did also not impair immunization-induced immunity (Figure 4D) which was attributed to helminth excretory-secretory products (ES products) (Colombo et al., 2022).
In conclusion, multiple helminth infections are common and have significant consequences on the outcome of each infection (Figure 4D): i) one helminth infection overrules the immune response to the second infection; ii) the temporal sequence of the respective infection is important for the immunopathological consequences; iii) host susceptibility or resistance can be changed by the coinfecting helminth but is independent of immunization-induced immunity.
7 Therapeutic interventions in STH coinfections
The main intervention to control STH in endemic regions is preventive chemotherapy recommended by the World Health Organization (WHO), defined as the regular administration of anthelmintics on a large scale to populations at risk (World Health Organization, 2022). There are different anthelmintics recommended by the WHO for the treatment of STHs: the two benzimidazoles, mebendazole (MBD) and albendazole (ALB), whereby ivermectin was added recently for the control of S. stercoralis (World Health Organization, 2023). To reduce morbidity from STH infections, particularly in preschool and school-aged children, but also in high-risk groups (i.e., pregnant women, lactating women, or adults in high-risk occupations), the WHO has established guidelines for large-scale preventive chemotherapy through mass drug administration (MDA) programs (World Health Organization, 2023). MBD and ALB are widely used in MDA campaigns in endemic countries. When administered orally once, both drugs are highly effective against A. lumbricoides but significantly less effective against T. trichiura. ALB, on the other hand, has better efficacy against hookworms compared to MBD (Moser et al., 2019). However, regular treatment is needed because eggs or larvae that remain in the environment for many months can be the cause of reinfection (Midha et al., 2021). In addition, a network meta-analysis revealed an alarming decline in ALB and MBD efficacy, which may indicate the development of anthelmintic resistance (Moser et al., 2018).
Where STH and schistosomiasis coincide, the WHO recommends that praziquantel (for schistosomiasis) and MBD/ALB can be safely administered together, and this is being done, particularly in Sub-Saharan Africa, where most of these infections occur (World Health Organization, 2022).
For other coinfections with STH, including viral and bacterial coinfections, there are no clear guidelines for therapeutic interventions other than treating each infection once it is diagnosed. There are conflicting results on the effect of deworming in HIV and STH coinfected individuals receiving concomitant antiretroviral therapy. A study in Tanzania found a low prevalence of intestinal helminths in HIV-infected patients compared with HIV-negative participants, presumably because the HIV-infected participants received anthelmintics for prophylaxis (Mwambete et al., 2010). Studies have also reported lower viral loads and higher CD4+ T cell counts in HIV-STH coinfected individuals treated with anthelmintics (Wolday et al., 2002; Ivan et al., 2015; Means et al., 2016), while other studies have found no effect (Lawn et al., 2000; Modjarrad et al., 2005; Hosseinipour et al., 2007). Therefore, further long-term studies are needed to determine the safety and benefit of preventive chemotherapy to control STH in HIV-positive patients.
When STH and malaria coinfections occur, each disease is often treated with a different strategy. For STH, preventive chemotherapy is used; for malaria, intermittent preventive treatment (IPT) and seasonal malaria chemoprevention (SMC) are used. Artemisinin-based combination therapies are currently the most effective and widely used agents for malaria control. SMC is used in endemic malaria areas during the period of highest malaria risk and involves the administration of the full dose of antimalarials at monthly intervals to maintain drug concentrations in the blood (Coldiron et al., 2017). Efforts are underway to integrate a unified strategy to control co-occurring diseases such as malaria and helminths, and evidence suggests that intermittent preventive malaria treatment and deworming reduces the prevalence of anemia and leads to improved cognitive skills in school-aged children (Opoku et al., 2016; Afolabi et al., 2022). Even with disease-specific approaches, some studies have reported benefits beyond the targeted disease. For example, quarterly administration of ALB in children older than 5 years resulted in a lower incidence of clinical malaria (Ndibazza et al., 2012). However, the results of a number of studies are contradictory. For example, a study in Madagascar found a significant increase in P. falciparum parasitemia in children aged ≥15 years treated with the anthelmintic levamisole for two months compared with negative controls (Brutus et al., 2006). Several other studies have found no effect of deworming on malaria (Kirwan et al., 2010; Wiria et al., 2013; Kepha et al., 2016). Despite some of these conflicting results, there is a consensus that intensive deworming does not alter the risk of malaria in school-aged children.
However, in addition to the WHO recommendations to treat STH and schistosome coinfections accordingly, general guidelines for other coinfections are lacking. As mentioned above, helminth coinfections can influence the immune response in a way that favors the development and spread of co-occurring microbial infections. Hence, it should follow that treatment of the microbial coinfection might be more promising if the STH infection has been eliminated before or, at the latest, in parallel if there are no concerns about deleterious drug interactions.
8 Outstanding questions and future directions
STHs can change host immunity both locally at the site of infection and remotely in tissues that are not parasitized. Therefore, the interactions are very complex and seem to depend largely on the context and biology of the coinfection in a bidirectional manner. Pioneering studies in rodent models provided important mechanistic insights into different coinfection scenarios, however, limitations of the translatability of the rodent models are obvious, since no natural infections with STHs relevant for humans occur in them. Here, the pig represents a valuable human-relevant model for the study of infectious diseases (Schlosser et al., 2014; Ebner et al., 2017; Schmidt et al., 2020), since closely related STH species with even zoonotic potential (e.g. T. suis, A. suum) and viruses (e.g. HEV, influenza A virus) or bacterial species (e.g. S. Typhimurium), relevant to humans, occur naturally in the pig. In the future, more complex in vitro systems integrating stromal and immune cells and microengineering technologies with organoids offer great potential to further advance animal-based research on STHs and relevant coinfecting pathogens while expanding knowledge of mechanistic processes.
Despite the ameliorative effect of some coinfections with enteric helminths in a few studies (Chowaniec et al., 1972; McFarlane et al., 2017; Rolot et al., 2018), some experimental studies have shown that the timing of parasite infection and host age can be crucial for the outcome of concurrent microbial disease (Furze et al., 2006; Hussain et al., 2023) and that prior tissue damage from larval migration may also be particularly important (Wescott and Todd, 1966; Gazzinelli-Guimarães et al., 2017). Nevertheless, only few studies that addressed interactions between helminths and other pathogens examined the effects of coinfection on the worm’s life cycle and related host immune responses, such as modulation of larval migration, adult worm establishment, and persistence in the gut (Coomes et al., 2015; Ahmed et al., 2017). The question of how disruption of the epithelial barrier by coinfecting intestinal pathogens might drive or even hinder the establishment of helminth infection in the intestine and what consequences this has for the anti-STH immune response is therefore only beginning to be elucidated and requires further investigation. Whether altered hepatic immune responses and profibrotic mechanisms during coinfection have an impact on larval migration and subsequent intestinal settlement of adult worms remains to be elucidated as well. As little is known to date, targeted studies investigating the effect of therapeutic interventions in STH and microbial coinfections are urgently needed to develop consensus guidelines for the treatment of co-occurring infections.
However, efforts to reduce helminth infections through preventive chemotherapy may have spillover effects on other infections or even metabolic disorders, which in turn can be beneficial or detrimental to the host’s health. In this context, it is therefore controversial to discuss whether certain susceptibilities to infectious diseases and associated morbidities in populations underscore the need to restore the lost biodiversity of eukaryotic symbionts such as helminths.
Author contributions
Conceptualization, JS-B, AM, SH. Writing — original draft preparation, JS-B, AM, SR, SH, RM, GG, EN, DN. Writing — review and editing, JS-B, AM, SR, SH, RM, GG, EN, DN. All authors contributed to the article and approved the submitted version.
Funding
This work was funded by the Deutsche Forschungsgemeinschaft (DFG): grant HA 2542-11-1 to SH and DFG GRK 2046 to SH; by the National Institute of Allergy and Infectious Diseases-National Institute of Health (NIAID-NIH): award number 1R01AI160433-01 to EN; by the MERCK Schistosomiasis Research Grant Initiative with the project title “Molecular Surveillance of Praziquantel Resistance” to EN; by the National Research Fund (NRF) South Africa, Covid-19 Africa Rapid Grant Fund with the project title “Uptake of Preventive Measures, Sero-Surveillance and Complementary Management of Covid-19 in Kenya” to EN; by the Freie Universität Berlin in Germany to promote young female scientists in the fields of public health and clinical infectious diseases to JS-B. We acknowledge support by the Open Access Publication Fund of the Freie Universität Berlin.
Acknowledgments
We thank Dr. Anne Winkler, Institute of Immunology, Freie Universität Berlin, for the graphic realization and design of the figures.
Conflict of interest
The authors declare that the research was conducted in the absence of any commercial or financial relationships that could be construed as a potential conflict of interest.
Publisher’s note
All claims expressed in this article are solely those of the authors and do not necessarily represent those of their affiliated organizations, or those of the publisher, the editors and the reviewers. Any product that may be evaluated in this article, or claim that may be made by its manufacturer, is not guaranteed or endorsed by the publisher.
References
Abanyie F. A., McCracken C., Kirwan P., Molloy S. F., Asaolu S. O., Holland C. V., et al. (2013). Ascaris co-infection does not alter malaria-induced anaemia in a cohort of Nigerian preschool children. Malar. J. 12, 1. doi: 10.1186/1475-2875-12-1
Abruzzi A., Fried B., Alikhan S. B. (2016). Coinfection of schistosoma species with hepatitis b or hepatitis c viruses. Adv. Parasitol. 91, 111–231. doi: 10.1016/bs.apar.2015.12.003
Adjobimey T., Meyer J., Terkeš V., Parcina M., Hoerauf A. (2022). Helminth antigens differentially modulate the activation of CD4+ and CD8+ T lymphocytes of convalescent COVID-19 patients in vitro. BMC Med. 20, 241. doi: 10.1186/s12916-022-02441-x
Afolabi M. O., Adebiyi A., Cano J., Sartorius B., Greenwood B., Johnson O., et al. (2022). Prevalence and distribution pattern of malaria and soil-transmitted helminth co-endemicity in sub-Saharan Africa 2000-2018: a geospatial analysis. PloS Negl. Trop. Dis. 16, e0010321. doi: 10.1371/journal.pntd.0010321
Ahmed N., French T., Rausch S., Kühl A., Hemminger K., Dunay I. R., et al. (2017). Toxoplasma Co-infection prevents Th2 differentiation and leads to a helminth-specific Th1 response. Front. Cell Infect. Microbiol. 7. doi: 10.3389/fcimb.2017.00341
Akelew Y., Andualem H., Ebrahim E., Atnaf A., Hailemichael W. (2022). Immunomodulation of COVID-19 severity by helminth co-infection: implications for COVID-19 vaccine efficacy. Immunity Inflammation Dis. 10, e573. doi: 10.1002/iid3.573
Amoani B., Gyan B., Sakyi S. A., Abu E. K., Nuvor S. V., Barnes P., et al. (2021). Effect of hookworm infection and anthelmintic treatment on naturally acquired antibody responses against the GMZ2 malaria vaccine candidate and constituent antigens. BMC Infect. Dis. 21, 332. doi: 10.1186/s12879-021-06027-5
Amoo J. K., Akindele A. A., Amoo A. O. J., Efunshile A. M., Ojurongbe T. A., Fayemiwo S. A., et al. (2018). Prevalence of enteric parasitic infections among people living with HIV in abeokuta, Nigeria. Pan Afr Med. J. 30, 66. doi: 10.11604/pamj.2018.30.66.13160
Andreasen A., Petersen H. H., Kringel H., Iburg T. M., Skovgaard K., Dawson H., et al. (2015). Immune and inflammatory responses in pigs infected with trichuris suis and oesophagostomum dentatum. Veterinary Parasitol. 207, 249–258. doi: 10.1016/j.vetpar.2014.12.005
Araka S., Masaku J., Kimani B., Simiyu E., Matendechero S., Okoyo C., et al. (2022). Assessment of soil-transmitted helminth infections among pregnant women in Western Kenya: a cross-sectional study. East Afr. J. Health Sci. 5, 105–118. doi: 10.37284/eajhs.5.1.670
Archer J., O’Halloran L., Al-Shehri H., Summers S., Bhattacharyya T., Kabaterine N. B., et al. (2020). Intestinal schistosomiasis and giardiasis Co-infection in Sub-Saharan Africa: can a one health approach improve control of each waterborne parasite simultaneously? Trop. Med. Infect. Dis. 5, 137. doi: 10.3390/tropicalmed5030137
Ateba-Ngoa U., Jones S., Zinsou J. F., Honkpehedji J., Adegnika A. A., Agobe J.-C. D., et al. (2016). Associations between helminth infections, plasmodium falciparum parasite carriage and antibody responses to sexual and asexual stage malarial antigens. Am. J. Trop. Med. Hygiene 95, 394–400. doi: 10.4269/ajtmh.15-0703
Awili H. O., Gitao G. C., Muchemi G. M. (2020). Seroprevalence and risk factors for hepatitis b virus infection in adolescent blood donors within selected counties of Western Kenya. BioMed. Res. Int. 2020, 8578172. doi: 10.1155/2020/8578172
Babamale O. A., Ugbomoiko U. S., Heukelbach J. (2018). High prevalence of plasmodium falciparum and soil-transmitted helminth co-infections in a periurban community in kwara state, Nigeria. J. Infect. Public Health 11, 48–53. doi: 10.1016/j.jiph.2017.03.002
Babu S., Nutman T. B. (2016). Helminth-tuberculosis Co-infection: an immunologic perspective. Trends Immunol. 37, 597–607. doi: 10.1016/j.it.2016.07.005
Barton E. S., White D. W., Cathelyn J. S., Brett-McClellan K. A., Engle M., Diamond M. S., et al. (2007). Herpesvirus latency confers symbiotic protection from bacterial infection. Nature 447, 326–329. doi: 10.1038/nature05762
Bazzone L. E., Smith P. M., Rutitzky L. I., Shainheit M. G., Urban J. F., Setiawan T., et al. (2008). Coinfection with the intestinal nematode heligmosomoides polygyrus markedly reduces hepatic egg-induced immunopathology and proinflammatory cytokines in mouse models of severe schistosomiasis. Infection Immun. 76, 5164–5172. doi: 10.1128/IAI.00673-08
Bhengu K. N., Naidoo P., Singh R., Mpaka-Mbatha M. N., Nembe N., Duma Z., et al. (2022). Immunological interactions between intestinal helminth infections and tuberculosis. Diagnostics 12, 2676. doi: 10.3390/diagnostics12112676
Blackwell A. D., Martin M., Kaplan H., Gurven M. (2013). Antagonism between two intestinal parasites in humans: the importance of co-infection for infection risk and recovery dynamics. Proc. R. Soc. B: Biol. Sci. 280, 20131671. doi: 10.1098/rspb.2013.1671
Bobat S., Darby M., Mrdjen D., Cook C., Logan E., Auret J., et al. (2014). Natural and vaccine-mediated immunity to salmonella typhimurium is impaired by the helminth nippostrongylus brasiliensis. PloS Negl. Trop. Dis. 8, e3341. doi: 10.1371/journal.pntd.0003341
Brosschot T. P., Lawrence K. M., Moeller B. E., Kennedy M. H. E., FitzPatrick R. D., Gauthier C. M., et al. (2021). Impaired host resistance to salmonella during helminth co-infection is restored by anthelmintic treatment prior to bacterial challenge. PloS Negl. Trop. Dis. 15, e0009052. doi: 10.1371/journal.pntd.0009052
Brutus L., Watier L., Briand V., Hanitrasoamampionona V., Razanatsoarilala H., Cot M. (2006). Parasitic co-infections: does ascaris lumbricoides protect against plasmodium falciparum infection? Am. J. Trop. Med. Hyg. 75, 194–198. doi: 10.4269/ajtmh.2006.75.194
Buendía J. A., Lindarte E. F., Polack F. P. (2022). TLR4 gene polymorphisms interaction with ascaris infection in severe RSV bronchiolitis. Front. Pediatr. 10. doi: 10.3389/fped.2022.876882
Bustamante-Rengifo J. A., Astudillo-Hernández M., del Pilar Crespo-Ortiz M. (2021). Effect of helicobacter pylori and helminth coinfection on the immune response to mycobacterium tuberculosis. Curr. Microbiol. 78, 3351–3371. doi: 10.1007/s00284-021-02604-8
Bygbjerg I. C. (2012). Double burden of noncommunicable and infectious diseases in developing countries. Science 337, 1499–1501. doi: 10.1126/science.1223466
Caballero M. T., Serra M. E., Acosta P. L., Marzec J., Gibbons L., Salim M., et al. (2015). TLR4 genotype and environmental LPS mediate RSV bronchiolitis through Th2 polarization. J. Clin. Invest. 125, 571–582. doi: 10.1172/JCI75183
Cadmus S. I., Akinseye V. O., Taiwo B. O., Pinelli E. O., van Soolingen D., Rhodes S.G. (2020). Interactions between helminths and tuberculosis infections: implications for tuberculosis diagnosis and vaccination in Africa. PloS Negl. Trop. Dis. 14, e0008069. doi: 10.1371/journal.pntd.0008069
Centers for Disease Control and Prevention (2022) CDC - soil-transmitted helminths. Available at: https://www.cdc.gov/parasites/sth/index.html (Accessed March 30, 2023).
Cepon-Robins T. J., Gildner T. E. (2020). Old friends meet a new foe: a potential role for immune-priming parasites in mitigating COVID-19 morbidity and mortality. Evol. Med. Public Health 2020, 234–248. doi: 10.1093/emph/eoaa037
Chauhan R. P., Dessie Z. G., Noreddin A., El Zowalaty M. E. (2020). Systematic review of important viral diseases in Africa in light of the ‘One health’ concept. Pathogens 9, 301. doi: 10.3390/pathogens9040301
Chen C.-C., Louie S., McCormick B., Walker W. A., Shi H. N. (2005). Concurrent infection with an intestinal helminth parasite impairs host resistance to enteric citrobacter rodentium and enhances citrobacter-induced colitis in mice. Infect. Immun. 73, 5468–5481. doi: 10.1128/IAI.73.9.5468-5481.2005
Chetty A., Darby M. G., Vornewald P. M., Martín-Alonso M., Filz A., Ritter M., et al. (2021). Il4ra-independent vaginal eosinophil accumulation following helminth infection exacerbates epithelial ulcerative pathology of HSV-2 infection. Cell Host Microbe 29, 579–593.e5. doi: 10.1016/j.chom.2021.02.004
Chowaniec W., Wescott R. B., Congdon L. L. (1972). Interaction of nematospiroides dubius and influenza virus in mice. Exp. Parasitol. 32, 33–44. doi: 10.1016/0014-4894(72)90007-0
Coldiron M. E., Von Seidlein L., Grais R. F. (2017). Seasonal malaria chemoprevention: successes and missed opportunities. Malaria J. 16, 481. doi: 10.1186/s12936-017-2132-1
Collins F. M., Boros D. L., Warren K. S. (1972). The effect of schistosoma mansoni infection on the response of mice to salmonella enteritidis and listeria monocytogenes. J. Infect. Dis. 125, 249–256. doi: 10.1093/infdis/125.3.249
Collins J. W., Keeney K. M., Crepin V. F., Rathinam V. A. K., Fitzgerald K. A., Finlay B. B., et al. (2014). Citrobacter rodentium: infection, inflammation and the microbiota. Nat. Rev. Microbiol. 12, 612–623. doi: 10.1038/nrmicro3315
Colombo S. A. P., Grencis R. K. (2020). Immunity to soil-transmitted helminths: evidence from the field and laboratory models. Front. Immunol. 11. doi: 10.3389/fimmu.2020.01286
Colombo S. A. P., Thompson S., Bancroft A. J., Grencis R. K. (2022). Anti-trichuris mucosal responses are maintained during h. bakeri co-infection despite impaired parasite expulsion. Parasite Immunol. 44, e12936. doi: 10.1111/pim.12936
Coomes S. M., Pelly V. S., Kannan Y., Okoye I. S., Czieso S., Entwistle L. J., et al. (2015). IFNγ and IL-12 restrict Th2 responses during Helminth/Plasmodium Co-infection and promote IFNγ from Th2 cells. PloS Pathog. 11, e1004994. doi: 10.1371/journal.ppat.1004994
Cooper P. J., Chico M. E., Sandoval C., Espinel I., Guevara A., Kennedy M. W., et al. (2000). Human infection with ascaris lumbricoides is associated with a polarized cytokine response. J. Infect. Dis. 182, 1207–1213. doi: 10.1086/315830
Cooper P. J., Chico M., Sandoval C., Espinel I., Guevara A., Levine M. M., et al. (2001). Human infection with ascaris lumbricoides is associated with suppression of the interleukin-2 response to recombinant cholera toxin b subunit following vaccination with the live oral cholera vaccine CVD 103-HgR. Infect. Immun. 69, 1574–1580. doi: 10.1128/IAI.69.3.1574-1580.2001
Courtin D., Djilali-Saïah A., Milet J., Soulard V., Gaye O., Migot-Nabias F., et al. (2011). Schistosoma haematobium infection affects plasmodium falciparum-specific IgG responses associated with protection against malaria. Parasite Immunol. 33, 124–131. doi: 10.1111/j.1365-3024.2010.01267.x
Courtin D., Oesterholt M., Huismans H., Kusi K., Milet J., Badaut C., et al. (2009). The quantity and quality of African children’s IgG responses to merozoite surface antigens reflect protection against plasmodium falciparum malaria. PloS One 4, e7590. doi: 10.1371/journal.pone.0007590
Craig J. M., Scott A. L. (2017). Antecedent nippostrongylus infection alters the lung immune response to plasmodium berghei. Parasite Immunol. 39(8). doi: 10.1111/pim.12441
Dann S. M., Manthey C. F., Le C., Miyamoto Y., Gima L., Abrahim A., et al. (2015). IL-17A promotes protective IgA responses and expression of other potential effectors against the lumen-dwelling enteric parasite giardia. Exp. Parasitol. 156, 68–78. doi: 10.1016/j.exppara.2015.06.003
Degarege A., Animut A., Legesse M., Erko B. (2010). Malaria and helminth co-infections in outpatients of alaba kulito health center, southern Ethiopia: a cross sectional study. BMC Res. Notes 3, 143. doi: 10.1186/1756-0500-3-143
Degarege A., Legesse M., Medhin G., Animut A., Erko B. (2012). Malaria and related outcomes in patients with intestinal helminths: a cross-sectional study. BMC Infect. Dis. 12, 291. doi: 10.1186/1471-2334-12-291
de Jong H. K., Parry C. M., van der Poll T., Wiersinga W. J. (2012). Host-pathogen interaction in invasive salmonellosis. PloS Pathog. 8, e1002933. doi: 10.1371/journal.ppat.1002933
Desai P., Diamond M. S., Thackray L. B. (2021). Helminth–virus interactions: determinants of coinfection outcomes. Gut Microbes 13, 1961202. doi: 10.1080/19490976.2021.1961202
Diallo T. O., Remoue F., Gaayeb L., Schacht A.-M., Charrier N., Clerck D. D., et al. (2010). Schistosomiasis coinfection in children influences acquired immune response against plasmodium falciparum malaria antigens. PloS One 5, e12764. doi: 10.1371/journal.pone.0012764
Division of National Malaria Programme (DNMP) [Kenya] and ICF (2021). Kenya Malaria indicator survey 2020 (Nairobi, Kenya and Rockville, Maryland, USA: DNMP and ICF). Available at: https://dhsprogram.com/pubs/pdf/MIS36/MIS36.pdf.
Djeneba O., Damintoti K., Denise I., Christelle N. W. M., Virgilio P., Adrien B., et al. (2007). Prevalence of rotavirus, adenovirus and enteric parasites among pediatric patients attending saint Camille medical centre in Ouagadougou. Pak J. Biol. Sci. 10, 4266–4270. doi: 10.3923/pjbs.2007.4266.4270
Dong H., Zhao L., Sun H., Shang M., Lv G., Yu X., et al. (2022). Coinfection of clonorchis sinensis and hepatitis b virus: clinical liver indices and interaction in hepatic cell models. Parasit Vectors 15, 460. doi: 10.1186/s13071-022-05548-5
Donohue R. E., Cross Z. K., Michael E. (2019). The extent, nature, and pathogenic consequences of helminth polyparasitism in humans: a meta-analysis. PloS Negl. Trop. Dis. 13, e0007455. doi: 10.1371/journal.pntd.0007455
Easton A. V., Quiñones M., Vujkovic-Cvijin I., Oliveira R. G., Kepha S., Odiere M. R., et al. (2019). The impact of anthelmintic treatment on human gut microbiota based on cross-sectional and pre- and postdeworming comparisons in Western Kenya. mBio 10(2):e00519-19. doi: 10.1128/mBio.00519-19
Easton A. V., Raciny-Aleman M., Liu V., Ruan E., Marier C., Heguy A., et al. (2020). Immune response and microbiota profiles during coinfection with plasmodium vivax and soil-transmitted helminths. mBio 11, e01705–e01720. doi: 10.1128/mBio.01705-20
Ebner F., Schwiertz P., Steinfelder S., Pieper R., Zentek J., Schütze N., et al. (2017). Pathogen-reactive T helper cell analysis in the pig. Front. Immunol. 8. doi: 10.3389/fimmu.2017.00565
Edwards M. J., Buchatska O., Ashton M., Montoya M., Bickle Q. D., Borrow P. (2005). Reciprocal immunomodulation in a schistosome and hepatotropic virus coinfection model. J. Immunol. 175, 6275–6285. doi: 10.4049/jimmunol.175.10.6275
el-Esnawy N. A., Al-Herrawy A. Z. (2000). Seroprevalence of certain hepatitis viruses among Egyptian workers infected with schistosomiasis. J. Egypt Public Health Assoc. 75, 357–366. Available at: https://pubmed.ncbi.nlm.nih.gov/17216928/.
Elias D., Mengistu G., Akuffo H., Britton S. (2006). Are intestinal helminths risk factors for developing active tuberculosis? Trop. Med. Int. Health 11, 551–558. doi: 10.1111/j.1365-3156.2006.01578.x
Elliott A. M., Kyosiimire J., Quigley M. A., Nakiyingi J., Watera C., Brown M., et al. (2003). Eosinophilia and progression to active tuberculosis in HIV-1-infected ugandans. Trans. R. Soc. Trop. Med. Hygiene 97, 477–480. doi: 10.1016/S0035-9203(03)90096-4
Enos M., Sitienei J., Ong’ang’o J., Mungai B., Kamene M., Wambugu J., et al. (2018). Kenya Tuberculosis prevalence survey 2016: challenges and opportunities of ending TB in Kenya. PloS One 13, e0209098. doi: 10.1371/journal.pone.0209098
Fekete E., Allain T., Siddiq A., Sosnowski O., Buret A. G. (2021). Giardia spp. and the gut microbiota: dangerous liaisons. Front. Microbiol. 11. doi: 10.3389/fmicb.2020.618106
Fenollar F., Mediannikov O. (2018). Emerging infectious diseases in Africa in the 21st century. New Microbes New Infect. 26, S10–S18. doi: 10.1016/j.nmni.2018.09.004
Fofana H. K. M., Schwarzkopf M., Doumbia M. N., Saye R., Nimmesgern A., Landouré A., et al. (2019). Prevalence of giardia intestinalis infection in schistosomiasis-endemic areas in south-central Mali. Trop. Med. Infect. Dis. 4, 86. doi: 10.3390/tropicalmed4020086
Furze R. C., Hussell T., Selkirk M. E. (2006). Amelioration of influenza-induced pathology in mice by coinfection with trichinella spiralis. Infect. Immun. 74, 1924–1932. doi: 10.1128/IAI.74.3.1924-1932.2006
Gao Y., Li Y., Liu X., Zhang T., Yu G., Wang Y., et al. (2020). High prevalence of clonorchis sinensis infections and coinfection with hepatitis virus in riverside villages in northeast China. Sci. Rep. 10, 11749. doi: 10.1038/s41598-020-68684-x
Gatt D., Martin I., AlFouzan R., Moraes T. J. (2023). Prevention and treatment strategies for respiratory syncytial virus (RSV). Pathogens 12, 154. doi: 10.3390/pathogens12020154
Gazzinelli-Guimarães P. H., de Freitas L. F. D., Gazzinelli-Guimarães A. C., Coelho F., Barbosa F. S., Nogueira D., et al. (2017). Concomitant helminth infection downmodulates the vaccinia virus-specific immune response and potentiates virus-associated pathology. Int. J. Parasitol. 47, 1–10. doi: 10.1016/j.ijpara.2016.08.007
Gendrel D., Kombila M., Beaudoin-Leblevec G., Richard-Lenoble D. (1994). Nontyphoidal salmonellal septicemia in gabonese children infected with schistosoma intercalatum. Clin. Infect. Dis. 18, 103–105. doi: 10.1093/clinids/18.1.103
Gendrel D., Richard-Lenoble D., Kombila M., Engohan E., Nardou M., Moussavou A., et al. (1984). Schistosoma intercalatum and relapses of salmonella infection in children. Am. J. Trop. Med. Hyg 33, 1166–1169. doi: 10.4269/ajtmh.1984.33.1166
George P. J., Anuradha R., Kumaran P. P., Chandrasekaran V., Nutman T. B., Babu S. (2013). Modulation of mycobacterial-specific Th1 and Th17 cells in latent tuberculosis by coincident hookworm infection. J. Immunol. 190, 5161–5168. doi: 10.4049/jimmunol.1203311
George P. J., Pavan Kumar N., Jaganathan J., Dolla C., Kumaran P., Nair D., et al. (2015). Modulation of pro- and anti-inflammatory cytokines in active and latent tuberculosis by coexistent strongyloides stercoralis infection. Tuberculosis 95, 822–828. doi: 10.1016/j.tube.2015.09.009
Geus D., Sifft K. C., Habarugira F., Mugisha J. C., Mukampunga C., Ndoli J., et al. (2019). Co-Infections with plasmodium, ascaris and giardia among Rwandan schoolchildren. Trop. Med. Int. Health 24, 409–420. doi: 10.1111/tmi.13206
Griffiths E. C., Fairlie-Clarke K., Allen J. E., Metcalf C. J. E., Graham A. L. (2015). Bottom-up regulation of malaria population dynamics in mice co-infected with lung-migratory nematodes. Ecol. Lett. 18, 1387–1396. doi: 10.1111/ele.12534
Hagel I., Cabrera M., Puccio F., Santaella C., Buvat E., Infante B., et al. (2011). Co-Infection with ascaris lumbricoides modulates protective immune responses against giardia duodenalis in school Venezuelan rural children. Acta Tropica 117, 189–195. doi: 10.1016/j.actatropica.2010.12.001
Halliday K. E., Oswald W. E., Mcharo C., Beaumont E., Gichuki P. M., Kepha S., et al. (2019). Community-level epidemiology of soil-transmitted helminths in the context of school-based deworming: baseline results of a cluster randomised trial on the coast of Kenya. PloS Negl. Trop. Dis. 13, e0007427. doi: 10.1371/journal.pntd.0007427
Harris N., Gause W. C. (2011). To b or not to b: b cells and the Th2-type immune response to helminths. Trends Immunol. 32, 80–88. doi: 10.1016/j.it.2010.11.005
Harris N. L., Loke P. (2018). Recent advances in type-2-Cell-Mediated immunity: insights from helminth infection. Immunity 48, 396. doi: 10.1016/j.immuni.2018.02.011
Hathout S., Abd el-Ghaffar Y., Awny A. Y., Hassan K. (1966). Relation between urinary schistosomiasis and chronic enteric urinary carrier state among egyptians. Am. J. Trop. Med. Hyg 15, 156–161. doi: 10.4269/ajtmh.1966.15.156
Helmby H. (2009). Gastrointestinal nematode infection exacerbates malaria-induced liver pathology. J. Immunol. 182, 5663–5671. doi: 10.4049/jimmunol.0803790
Hendel S. K., Kellermann L., Hausmann A., Bindslev N., Jensen K. B., Nielsen O. H. (2022). Tuft cells and their role in intestinal diseases. Front. Immunol. 13. doi: 10.3389/fimmu.2022.822867
Hepworth M. R., Daniłowicz-Luebert E., Rausch S., Metz M., Klotz C., Maurer M., et al. (2012). Mast cells orchestrate type 2 immunity to helminths through regulation of tissue-derived cytokines. Proc. Natl. Acad. Sci. U.S.A. 109, 6644–6649. doi: 10.1073/pnas.1112268109
Heron S. E., Elahi S. (2017). HIV Infection and compromised mucosal immunity: oral manifestations and systemic inflammation. Front. Immunol. 8. doi: 10.3389/fimmu.2017.00241
Hoeve M. A., Mylonas K. J., Fairlie-Clarke K. J., Mahajan S. M., Allen J. E., Graham A. L. (2009). Plasmodium chabaudi limits early nippostrongylus brasiliensis-induced pulmonary immune activation and Th2 polarization in co-infected mice. BMC Immunol. 10, 60. doi: 10.1186/1471-2172-10-60
Holali Ameyapoh A., Katawa G., Ritter M., Tchopba C. N., Tchadié P. E., Arndts K., et al. (2021). Hookworm infections and sociodemographic factors associated with female reproductive tract infections in rural areas of the central region of Togo. Front. Microbiol. 12. doi: 10.3389/fmicb.2021.738894
Hosseinipour M. C., Napravnik S., Joaki G., Gama S., Mbeye N., Banda B., et al. (2007). HIV And parasitic infection and the effect of treatment among adult outpatients in Malawi. J. Infect. Dis. 195, 1278–1282. doi: 10.1086/513274
Hussain T., Nguyen A., Daunt C., Thiele D., Pang E. S., Li J., et al. (2023). Helminth infection-induced increase in virtual memory CD8 T cells is transient, driven by IL-15, and absent in aged mice. J. Immunol. 210, 297–309. doi: 10.4049/jimmunol.2200316
Ikuta K. S., Swetschinski L. R., Aguilar G. R., Sharara F., Mestrovic T., Gray A. P., et al. (2022). Global mortality associated with 33 bacterial pathogens in 2019: a systematic analysis for the global burden of disease study 2019. Lancet 400, 2221–2248. doi: 10.1016/S0140-6736(22)02185-7
Ingle D. J., Ambrose R. L., Baines S. L., Duchene S., Gonçalves da Silva A., Lee D. Y. J., et al. (2021). Evolutionary dynamics of multidrug resistant salmonella enterica serovar 4,[5],12:i:- in Australia. Nat. Commun. 12, 4786. doi: 10.1038/s41467-021-25073-w
Ivan E., Crowther N. J., Mutimura E., Rucogoza A., Janssen S., Njunwa K. K., et al. (2015). Effect of deworming on disease progression markers in HIV-1–infected pregnant women on antiretroviral therapy: a longitudinal observational study from Rwanda. Clin. Infect. Dis. 60, 135–142. doi: 10.1093/cid/ciu715
Jeza V. T., Mutuku F., Kaduka L., Mwandawiro C., Masaku J., Okoyo C., et al. (2022). Schistosomiasis, soil transmitted helminthiasis, and malaria co-infections among women of reproductive age in rural communities of kwale county, coastal Kenya. BMC Public Health 22, 136. doi: 10.1186/s12889-022-12526-0
Kamar N., Izopet J., Pavio N., Aggarwal R., Labrique A., Wedemeyer H., et al. (2017). Hepatitis e virus infection. Nat. Rev. Dis. Primers 3, 17086. doi: 10.1038/nrdp.2017.86
Kamau E., Yates A., Maisiba R., Singoei V., Opot B., Adeny R., et al. (2021). Epidemiological and clinical implications of asymptomatic malaria and schistosomiasis co-infections in a rural community in western Kenya. BMC Infect. Dis. 21, 937. doi: 10.1186/s12879-021-06626-2
Karagiannis-Voules D.-A., Biedermann P., Ekpo U. F., Garba A., Langer E., Mathieu E., et al. (2015). Spatial and temporal distribution of soil-transmitted helminth infection in sub-Saharan Africa: a systematic review and geostatistical meta-analysis. Lancet Infect. Dis. 15, 74–84. doi: 10.1016/S1473-3099(14)71004-7
Kathamuthu G. R., Moideen K., Thiruvengadam K., Sridhar R., Baskaran D., Babu S. (2021). Helminth coinfection is associated with enhanced plasma levels of matrix metalloproteinases and tissue inhibitor of metalloproteinases in tuberculous lymphadenitis. Front. Cell. Infection Microbiol. 11. doi: 10.3389/fcimb.2021.680665
Kepha S., Nuwaha F., Nikolay B., Gichuki P., Edwards T., Allen E., et al. (2015). Epidemiology of coinfection with soil transmitted helminths and plasmodium falciparum among school children in bumula district in western Kenya. Parasites Vectors 8, 314. doi: 10.1186/s13071-015-0891-5
Kepha S., Nuwaha F., Nikolay B., Gichuki P., Mwandawiro C. S., Mwinzi P. N., et al. (2016). Effect of repeated anthelminthic treatment on malaria in school children in Kenya: a randomized, open-label, equivalence trial. J. Infect. Dis. 213, 266–275. doi: 10.1093/infdis/jiv382
Kepha S., Ochol D., Wakesho F., Omondi W., Njenga S. M., Njaanake K., et al. (2023). Precision mapping of schistosomiasis and soil-transmitted helminthiasis among school age children at the coastal region, Kenya. PloS Negl. Trop. Dis. 17, e0011043. doi: 10.1371/journal.pntd.0011043
Kiama C., Okunga E., Muange A., Marwanga D., Langat D., Kuria F., et al. (2023). Mapping of cholera hotspots in Kenya using epidemiologic and water, sanitation, and hygiene (WASH) indicators as part of kenya’s new 2022–2030 cholera elimination plan. PloS Negl. Trop. Dis. 17, e0011166. doi: 10.1371/journal.pntd.0011166
Kirwan P., Jackson A. L., Asaolu S. O., Molloy S. F., Abiona T. C., Bruce M. C., et al. (2010). Impact of repeated four-monthly anthelmintic treatment on plasmodium infection in preschool children: a double-blind placebo-controlled randomized trial. BMC Infect. Dis. 10, 277. doi: 10.1186/1471-2334-10-277
Klotz C., Aebischer T. (2015). The immunological enigma of human giardiasis. Curr. Trop. Med. Rep. 2, 119–127. doi: 10.1007/s40475-015-0050-2
Knuhr K., Langhans K., Nyenhuis S., Viertmann K., Kildemoes A. M. O., Doenhoff M. J., et al. (2018). Schistosoma mansoni egg-released IPSE/alpha-1 dampens inflammatory cytokine responses via basophil interleukin (IL)-4 and IL-13. Front. Immunol. 9. doi: 10.3389/fimmu.2018.02293
Lan K., Luo M.-H. (2017). Herpesviruses: epidemiology, pathogenesis, and interventions. Virol. Sin. 32, 347–348. doi: 10.1007/s12250-017-4108-2
Lass S., Hudson P. J., Thakar J., Saric J., Harvill E., Albert R., et al. (2013). Generating super-shedders: co-infection increases bacterial load and egg production of a gastrointestinal helminth. J. R. Soc. Interface 10, 20120588. doi: 10.1098/rsif.2012.0588
Law A. E., Shears R. K., Lopez Rodas A. A., Grencis R. K., Cooper P. J., Neill D. R., et al. (2021). Intestinal helminth co-infection is an unrecognised risk factor for increased pneumococcal carriage density and invasive disease. Sci. Rep. 11, 6984. doi: 10.1038/s41598-021-86508-4
Lawn S. D., Karanja D. M. S., Mwinzi P., Andove J., Colley D. G., Folks T. M., et al. (2000). The effect of treatment of schistosomiasis on blood plasma HIV-1 RNA concentration in coinfected individuals. AIDS 14, 2437. doi: 10.1097/00002030-200011100-00004
Li W., Dong H., Huang Y., Chen T., Kong X., Sun H., et al. (2016). Clonorchis sinensis Co-infection could affect the disease state and treatment response of HBV patients. PloS Negl. Trop. Dis. 10, e0004806. doi: 10.1371/journal.pntd.0004806
Loke P., Lee S. C., Oyesola O. O. (2022). Effects of helminths on the human immune response and the microbiome. Mucosal Immunol. 15, 1224–1233. doi: 10.1038/s41385-022-00532-9
Loukas A., Maizels R. M., Hotez P. J. (2021). The yin and yang of human soil-transmitted helminth infections. Int. J. Parasitol. 51, 1243–1253. doi: 10.1016/j.ijpara.2021.11.001
Maizels R. M., Gause W. C. (2014). Immunology. how helminths go viral. Science 345, 517–518. doi: 10.1126/science.1258443
Makokha G. N., Zhang P., Hayes C. N., Songok E., Chayama K. (2023). The burden of hepatitis b virus infection in Kenya: a systematic review and meta-analysis. Front. Public Health 11. doi: 10.3389/fpubh.2023.986020
Malm M., Hyöty H., Knip M., Vesikari T., Blazevic V. (2019). Development of T cell immunity to norovirus and rotavirus in children under five years of age. Sci. Rep. 9, 3199. doi: 10.1038/s41598-019-39840-9
Maple P. A. C., Gran B., Tanasescu R., Pritchard D. I., Constantinescu C. S. (2020). An absence of Epstein-Barr virus reactivation and associations with disease activity in people with multiple sclerosis undergoing therapeutic hookworm vaccination. Vaccines 8, 487. doi: 10.3390/vaccines8030487
Masaku J., Njomo D. W., Njoka A., Okoyo C., Mutungi F. M., Njenga S. M. (2020). Soil-transmitted helminths and schistosomiasis among pre-school age children in a rural setting of busia county, Western Kenya: a cross-sectional study of prevalence, and associated exposures. BMC Public Health 20, 356. doi: 10.1186/s12889-020-08485-z
Mbuyi-Kalonji L., Barbé B., Nkoji G., Madinga J., Roucher C., Linsuke S., et al. (2020). Non-typhoidal salmonella intestinal carriage in a schistosoma mansoni endemic community in a rural area of the democratic republic of Congo. PloS Negl. Trop. Dis. 14, e0007875. doi: 10.1371/journal.pntd.0007875
McFarlane A. J., McSorley H. J., Davidson D. J., Fitch P. M., Errington C., Mackenzie K. J., et al. (2017). Enteric helminth-induced type I interferon signaling protects against pulmonary virus infection through interaction with the microbiota. J. Allergy Clin. Immunol. 140, 1068–1078.e6. doi: 10.1016/j.jaci.2017.01.016
Means A. R., Risher K. A., Ujeneza E. L., Maposa I., Nondi J., Bellan S. E. (2016). Impact of age and sex on CD4+ cell count trajectories following treatment initiation: an analysis of the Tanzanian HIV treatment database. PloS One 11, e0164148. doi: 10.1371/journal.pone.0164148
Midha A., Ebner F., Schlosser-Brandenburg J., Rausch S., Hartmann S. (2021). Trilateral relationship: ascaris, microbiota, and host cells. Trends Parasitol. 37, 251–262. doi: 10.1016/j.pt.2020.09.002
Midha A., Schlosser J., Hartmann S. (2017). Reciprocal interactions between nematodes and their microbial environments. Front. Cell. Infect. Microbiol. 7. doi: 10.3389/fcimb.2017.00144
Mkhize-Kwitshana Z. L., Mabaso M. L., Walzl G. (2014). Proliferative capacity and cytokine production by cells of HIV-infected and uninfected adults with different helminth infection phenotypes in south Africa. BMC Infect. Dis. 14, 499. doi: 10.1186/1471-2334-14-499
Mkhize-Kwitshana Z. L., Taylor M., Jooste P., Mabaso M. L., Walzl G. (2011). The influence of different helminth infection phenotypes on immune responses against HIV in co-infected adults in south Africa. BMC Infect. Dis. 11, 273. doi: 10.1186/1471-2334-11-273
Modjarrad K., Zulu I., Redden D. T., Njobvu L., Lane H. C., Bentwich Z., et al. (2005). Treatment of intestinal helminths does not reduce plasma concentrations of HIV-1 RNA in coinfected Zambian adults. J. Infect. Dis. 192, 1277–1283. doi: 10.1086/444543
Montes M., Sanchez C., Verdonck K., Lake J. E., Gonzalez E., Lopez G., et al. (2009). Regulatory T cell expansion in HTLV-1 and strongyloidiasis co-infection is associated with reduced IL-5 responses to strongyloides stercoralis antigen. PloS Negl. Trop. Dis. 3, e456. doi: 10.1371/journal.pntd.0000456
Morawski B. M., Yunus M., Kerukadho E., Turyasingura G., Barbra L., Ojok A. M., et al. (2017). Hookworm infection is associated with decreased CD4+ T cell counts in HIV-infected adult ugandans. PloS Negl. Trop. Dis. 11, e0005634. doi: 10.1371/journal.pntd.0005634
Moser W., Sayasone S., Xayavong S., Bounheuang B., Puchkov M., Huwyler J., et al. (2018). Efficacy and tolerability of triple drug therapy with albendazole, pyrantel pamoate, and oxantel pamoate compared with albendazole plus oxantel pamoate, pyrantel pamoate plus oxantel pamoate, and mebendazole plus pyrantel pamoate and oxantel pamoate against hookworm infections in school-aged children in Laos: a randomised, single-blind trial. Lancet Infect. Dis. 18, 729–737. doi: 10.1016/S1473-3099(18)30220-2
Moser W., Schindler C., Keiser J. (2019). “Chapter five - drug combinations against soil-transmitted helminth infections,” in Advances in parasitology highlighting operational and implementation research for control of helminthiasis. Ed. Keiser J. (Adv Parasitol: Academic Press), 91–115. doi: 10.1016/bs.apar.2018.08.002
Mpaka-Mbatha M. N., Naidoo P., Islam M. M., Singh R., Mkhize-Kwitshana Z. L. (2023). Demographic profile of HIV and helminth-coinfected adults in KwaZulu-natal, south Africa. S Afr J. Infect. Dis. 38, 466. doi: 10.4102/sajid.v38i1.466
Mutonga D., Langat D., Mwangi D., Tonui J., Njeru M., Abade A., et al. (2013). National surveillance data on the epidemiology of cholera in kenya 1997–2010. J. Infect. Dis. 208, S55–S61. doi: 10.1093/infdis/jit201
Mwambete K. D., Justin-Temu M., Peter S. (2010). Prevalence and management of intestinal helminthiasis among HIV-infected patients at muhimbili national hospital. J. Int. Assoc. Physicians AIDS Care (Chic) 9, 150–156. doi: 10.1177/1545109710368138
Mwandawiro C., Okoyo C., Kihara J., Simiyu E., Kepha S., Campbell S. J., et al. (2019). Results of a national school-based deworming programme on soil-transmitted helminths infections and schistosomiasis in Kenya: 2012–2017. Parasites Vectors 12, 76. doi: 10.1186/s13071-019-3322-1
Nacher M., Gay F., Singhasivanon P., Krudsood S., Treeprasertsuk S., Mazier D., et al. (2000). Ascaris lumbricoides infection is associated with protection from cerebral malaria. Parasite Immunol. 22, 107–113. doi: 10.1046/j.1365-3024.2000.00284.x
Nacher M., Singhasivanon P., Silachamroon U., Treeprasertsuk S., Vannaphan S., Traore B., et al. (2001). Helminth infections are associated with protection from malaria-related acute renal failure and jaundice in Thailand. Am. J. Trop. Med. Hygiene 65, 834–836. doi: 10.4269/ajtmh.2001.65.834
Nacher M., Singhasivanon P., Yimsamran S., Manibunyong W., Thanyavanich N., Wuthisen R., et al. (2002). Intestinal helminth infections are associated with increased incidence of plasmodium falciparum malaria in Thailand. J. Parasitol. 88, 55–58. doi: 10.1645/0022-3395(2002)088[0055:IHIAAW]2.0.CO;2
Ndibazza J., Mpairwe H., Webb E. L., Mawa P. A., Nampijja M., Muhangi L., et al. (2012). Impact of anthelminthic treatment in pregnancy and childhood on immunisations, infections and eczema in childhood: a randomised controlled trial. PloS One 7, e50325. doi: 10.1371/journal.pone.0050325
Ngonjo T., Okoyo C., Andove J., Simiyu E., Lelo A. E., Kabiru E., et al. (2016). Current status of soil-transmitted helminths among school children in kakamega county, Western Kenya. J. Parasitol. Res. 2016, e7680124. doi: 10.1155/2016/7680124
Njunda A. L., Oyerinde J. P. (1996). Salmonella typhi infection in schistosoma mansoni infected mice. West Afr J. Med. 15, 24–30. Available at: https://pubmed.ncbi.nlm.nih.gov/8652437/.
Noland G. S., Urban J. F., Fried B., Kumar N. (2008). Counter-regulatory anti-parasite cytokine responses during concurrent plasmodium yoelii and intestinal helminth infections in mice. Exp. Parasitol. 119, 272–278. doi: 10.1016/j.exppara.2008.02.009
Nyaruaba R., Okoye C. O., Akan O. D., Mwaliko C., Ebido C. C., Ayoola A., et al. (2022). Socio-economic impacts of emerging infectious diseases in Africa. Infect. Dis. 54, 315–324. doi: 10.1080/23744235.2021.2022195
Oba P., Wieland B., Mwiine F. N., Erume J., Dione M. M. (2023). Co-Infections of respiratory pathogens and gastrointestinal parasites in smallholder pig production systems in Uganda. Parasitol. Res 122 (4), 953–962. doi: 10.1007/s00436-023-07797-4
Okoyo C., Campbell S. J., Williams K., Simiyu E., Owaga C., Mwandawiro C. (2020). Prevalence, intensity and associated risk factors of soil-transmitted helminth and schistosome infections in Kenya: impact assessment after five rounds of mass drug administration in Kenya. PloS Negl. Trop. Dis. 14, e0008604. doi: 10.1371/journal.pntd.0008604
Okoyo C., Nikolay B., Kihara J., Simiyu E., Garn J. V., Freeman M. C., et al. (2016). Monitoring the impact of a national school based deworming programme on soil-transmitted helminths in Kenya: the first three years 2012 – 2014. Parasites Vectors 9, 408. doi: 10.1186/s13071-016-1679-y
Okoyo C., Simiyu E., Njenga S. M., Mwandawiro C. (2018). Comparing the performance of circulating cathodic antigen and kato-Katz techniques in evaluating schistosoma mansoni infection in areas with low prevalence in selected counties of Kenya: a cross-sectional study. BMC Public Health 18, 478. doi: 10.1186/s12889-018-5414-9
Onyango D. O., van der Sande M. A. B., Musingila P., Kinywa E., Opollo V., Oyaro B., et al. (2021). High HIV prevalence among decedents received by two high-volume mortuaries in kisumu, western Kenya 2019. PloS One 16, e0253516. doi: 10.1371/journal.pone.0253516
Opoku E. C., Olsen A., Browne E., Hodgson A., Awoonor-Williams J. K., Yelifari L., et al. (2016). Impact of combined intermittent preventive treatment of malaria and helminths on anaemia, sustained attention, and recall in northern ghanaian schoolchildren. Global Health Action 9, 32197. doi: 10.3402/gha.v9.32197
Osborne L. C., Monticelli L. A., Nice T. J., Sutherland T. E., Siracusa M. C., Hepworth M. R., et al. (2014). Virus-helminth coinfection reveals a microbiota-independent mechanism of immunomodulation. Science 345, 578–582. doi: 10.1126/science.1256942
Paerewijck O., Maertens B., Gagnaire A., De Bosscher K., Geldhof P. (2019). Delayed development of the protective IL-17A response following a giardia muris infection in neonatal mice. Sci. Rep. 9, 8959. doi: 10.1038/s41598-019-45544-x
Passos-Castilho A. M., de Sena A., Domingues A. L. C., Lopes-Neto E. P., Medeiros T. B., Granato C. F. H., et al. (2016). Hepatitis e virus seroprevalence among schistosomiasis patients in northeastern Brazil. Braz. J. Infect. Dis. 20, 262–266. doi: 10.1016/j.bjid.2016.03.001
Peacock J. W., Elsawa S. F., Petty C. C., Hickey W. F., Bost K. L. (2003). Exacerbation of experimental autoimmune encephalomyelitis in rodents infected with murine gammaherpesvirus-68. Eur. J. Immunol. 33, 1849–1858. doi: 10.1002/eji.200323148
Peng J., Federman H. G., Hernandez C. M., Siracusa M. C. (2022). Communication is key: innate immune cells regulate host protection to helminths. Front. Immunol. 13. doi: 10.3389/fimmu.2022.995432
Pickering A. J., Njenga S. M., Steinbaum L., Swarthout J., Lin A., Arnold B. F., et al. (2019). Effects of single and integrated water, sanitation, handwashing, and nutrition interventions on child soil-transmitted helminth and giardia infections: a cluster-randomized controlled trial in rural Kenya. PloS Med. 16, e1002841. doi: 10.1371/journal.pmed.1002841
Pinto R. A., Arredondo S. M., Bono M. R., Gaggero A. A., Díaz P. V. (2006). T Helper 1/T helper 2 cytokine imbalance in respiratory syncytial virus infection is associated with increased endogenous plasma cortisol. Pediatrics 117, e878–e886. doi: 10.1542/peds.2005-2119
Potgieter N., Heine L., Ngandu J. P. K., Ledwaba S. E., Zitha T., Mudau L. S., et al. (2023). High burden of Co-infection with multiple enteric pathogens in children suffering with diarrhoea from rural and peri-urban communities in south Africa. Pathogens 12, 315. doi: 10.3390/pathogens12020315
Pullan R. L., Bethony J. M., Geiger S. M., Cundill B., Correa-Oliveira R., Quinnell R. J., et al. (2008). Human helminth Co-infection: analysis of spatial patterns and risk factors in a Brazilian community. PloS Negl. Trop. Dis. 2, e352. doi: 10.1371/journal.pntd.0000352
Pullan R. L., Gething P. W., Smith J. L., Mwandawiro C. S., Sturrock H. J. W., Gitonga C. W., et al. (2011). Spatial modelling of soil-transmitted helminth infections in Kenya: a disease control planning tool. PloS Negl. Trop. Dis. 5, e958. doi: 10.1371/journal.pntd.0000958
Rabiu O. R., Dada-Adegbola H., Falade C. O., Arinola O. G., Odaibo A. B., Ademowo O. G. (2022). Serum cytokine profile of pregnant women with malaria, intestinal helminths and HIV infections in ibadan, Nigeria. Parasitol. Res. 121, 1983–1992. doi: 10.1007/s00436-022-07531-6
Reese T. A., Wakeman B. S., Choi H. S., Hufford M. M., Huang S. C., Zhang X., et al. (2014). Helminth infection reactivates latent γ-herpesvirus via cytokine competition at a viral promoter. Science 345, 573–577. doi: 10.1126/science.1254517
Reynolds L. A., Redpath S. A., Yurist-Doutsch S., Gill N., Brown E. M., van der Heijden J., et al. (2017). Enteric helminths promote salmonella co-infection by altering the intestinal metabolome. J. Infect. Dis 215 (8), 1245–1254. doi: 10.1093/infdis/jix141
Rivero M., De Angelo C., Nuñez P., Salas M., Liang S. (2018). Intestinal parasitism and nutritional status among indigenous children from the Argentinian Atlantic forest: determinants of enteroparasites infections in minority populations. Acta Tropica 187, 248–256. doi: 10.1016/j.actatropica.2018.08.015
Rocha H., Kirk J. W., Hearey C. D. (1971). Prolonged salmonella bacteremia in patients with schistosoma mansoni infection. Arch. Intern. Med. 128, 254–257. doi: 10.1001/archinte.1971.00310200090009
Rolot M., Dougall A. M., Chetty A., Javaux J., Chen T., Xiao X., et al. (2018). Helminth-induced IL-4 expands bystander memory CD8+ T cells for early control of viral infection. Nat. Commun. 9, 4516. doi: 10.1038/s41467-018-06978-5
Roussilhon C., Brasseur P., Agnamey P., Pérignon J.-L., Druilhe P. (2010). Understanding human-plasmodium falciparum immune interactions uncovers the immunological role of worms. PloS One 5, e9309. doi: 10.1371/journal.pone.0009309
Saghaug C. S., Sørnes S., Peirasmaki D., Svärd S., Langeland N., Hanevik K. (2016). Human memory CD4+ T cell immune responses against giardia lamblia. Clin. Vaccine Immunol. 23, 11–18. doi: 10.1128/CVI.00419-15
Santano R., Rubio R., Grau-Pujol B., Escola V., Muchisse O., Cuamba I., et al. (2021). Plasmodium falciparum and helminth coinfections increase IgE and parasite-specific IgG responses. Microbiol. Spectr. 9, e0110921. doi: 10.1128/Spectrum.01109-21
Schlosser J., Eiden M., Vina-Rodriguez A., Fast C., Dremsek P., Lange E., et al. (2014). Natural and experimental hepatitis e virus genotype 3-infection in European wild boar is transmissible to domestic pigs. Vet. Res. 45, 121. doi: 10.1186/s13567-014-0121-8
Schmidt S., Ebner F., Rosen K., Kniemeyer O., Brakhage A. A., Löffler J., et al. (2020). The domestic pig as human-relevant large animal model to study adaptive antifungal immune responses against airborne aspergillus fumigatus. Eur. J. Immunol. 50, 1712–1728. doi: 10.1002/eji.201948524
Schneider-Crease I., Blackwell A. D., Kraft T. S., Thompson M. E., Suarez I. M., Cummings D. K., et al. (2021). Helminth infection is associated with dampened cytokine responses to viral and bacterial stimulations in tsimane hunter-horticulturalists Evol Med Public Health 9 (1), 349–359. doi: 10.1101/2021.09.29.462428
Schramm G., Suwandi A., Galeev A., Sharma S., Braun J., Claes A.-K., et al. (2018). Schistosome eggs impair protective Th1/Th17 immune responses against salmonella infection. Front. Immunol. 9. doi: 10.3389/fimmu.2018.02614
Segura M., Matte C., Thawani N., Su Z., Stevenson M. M. (2009). Modulation of malaria-induced immunopathology by concurrent gastrointestinal nematode infection in mice. Int. J. Parasitol. 39, 1525–1532. doi: 10.1016/j.ijpara.2009.08.007
Shah M., Kathiiko C., Wada A., Odoyo E., Bundi M., Miringu G., et al. (2016). Prevalence, seasonal variation, and antibiotic resistance pattern of enteric bacterial pathogens among hospitalized diarrheic children in suburban regions of central Kenya. Trop. Med. Health 44, 39. doi: 10.1186/s41182-016-0038-1
Shin E.-C., Sung P. S., Park S.-H. (2016). Immune responses and immunopathology in acute and chronic viral hepatitis. Nat. Rev. Immunol. 16, 509–523. doi: 10.1038/nri.2016.69
Siwila J., Mwaba F., Chidumayo N., Mubanga C. (2020). Food and waterborne protozoan parasites: the African perspective. Food Waterborne Parasitol. 20, e00088. doi: 10.1016/j.fawpar.2020.e00088
Sorobetea D., Svensson-Frej M., Grencis R. (2018). Immunity to gastrointestinal nematode infections. Mucosal Immunol. 11, 304–315. doi: 10.1038/mi.2017.113
Spiegel A., Tall A., Raphenon G., Trape J. F., Druilhe P. (2003). Increased frequency of malaria attacks in subjects co-infected by intestinal worms and plasmodium falciparum malaria. Trans. R Soc. Trop. Med. Hyg 97, 198–199. doi: 10.1016/s0035-9203(03)90117-9
Steenhard N. R., Jungersen G., Kokotovic B., Beshah E., Dawson H. D., Urban J. F., et al. (2009). Ascaris suum infection negatively affects the response to a mycoplasma hyopneumoniae vaccination and subsequent challenge infection in pigs. Vaccine 27, 5161–5169. doi: 10.1016/j.vaccine.2009.05.075
Storey H. L., Singa B., Naulikha J., Horton H., Richardson B. A., John-Stewart G., et al. (2017). Soil transmitted helminth infections are not associated with compromised antibody responses to previously administered measles and tetanus vaccines among HIV-1 infected, ART naïve Kenyan adults. Parasite Epidemiol. Control 2, 13–20. doi: 10.1016/j.parepi.2016.12.003
Strine M. S., Wilen C. B. (2022). Tuft cells are key mediators of interkingdom interactions at mucosal barrier surfaces. PloS Pathog. 18, e1010318. doi: 10.1371/journal.ppat.1010318
Su Z., Segura M., Morgan K., Loredo-Osti J. C., Stevenson M. M. (2005). Impairment of protective immunity to blood-stage malaria by concurrent nematode infection. Infect. Immun. 73, 3531–3539. doi: 10.1128/IAI.73.6.3531-3539.2005
Su L., Su C., Qi Y., Yang G., Zhang M., Cherayil B. J., et al. (2014). Coinfection with an intestinal helminth impairs host innate immunity against salmonella enterica serovar typhimurium and exacerbates intestinal inflammation in mice. Infect. Immun. 82, 3855–3866. doi: 10.1128/IAI.02023-14
Tetsutani K., Ishiwata K., Ishida H., Tu L., Torii M., Hamano S., et al. (2009). Concurrent infection with heligmosomoides polygyrus suppresses anti-plasmodium yoelii protection partially by induction of CD4(+)CD25(+)Foxp3(+) treg in mice. Eur. J. Immunol. 39, 2822–2830. doi: 10.1002/eji.200939433
Tjørnehøj K., Eriksen L., Aalbaek B., Nansen P. (1992). Interaction between ascaris suum and pasteurella multocida in the lungs of mice. Parasitol. Res. 78, 525–528. doi: 10.1007/BF00931575
Tokplonou L., Nouatin O., Sonon P., M’po G., Glitho S., Agniwo P., et al. (2020). Schistosoma haematobium infection modulates plasmodium falciparum parasite density and antimalarial antibody responses. Parasite Immunol. 42, e12702. doi: 10.1111/pim.12702
Torre D., Speranza F., Giola M., Matteelli A., Tambini R., Biondi G. (2002). Role of Th1 and Th2 cytokines in immune response to uncomplicated plasmodium falciparum malaria. Clin. Vaccine Immunol. 9, 348–351. doi: 10.1128/CDLI.9.2.348-351.2002
Tristão-Sá R., Ribeiro-Rodrigues R., Johnson L. T., Pereira F. E. L., Dietze R. (2002). Intestinal nematodes and pulmonary tuberculosis. Rev. Soc Bras. Med. Trop. 35, 533–535. doi: 10.1590/S0037-86822002000500020
Vacca F., Le Gros G. (2022). Tissue-specific immunity in helminth infections. Mucosal Immunol. 15, 1212–1223. doi: 10.1038/s41385-022-00531-w
Wahome P. K., Kiende P., Nakazea R. J., Mwasowa N. M., Nyamu G. W. (2022). Occult hepatitis b virus infections and risk factors among school-going adolescent voluntary blood donors in kwale county Kenya, January 2020-June 2021: cross sectional study. PloS One 17, e0263473. doi: 10.1371/journal.pone.0263473
Walson J. L., Stewart B. T., Sangaré L., Mbogo L. W., Otieno P. A., Piper B. K. S., et al. (2010). Prevalence and correlates of helminth Co-infection in Kenyan HIV-1 infected adults. PloS Negl. Trop. Dis. 4, e644. doi: 10.1371/journal.pntd.0000644
Wammes L. J., Hamid F., Wiria A. E., May L., Kaisar M. M. M., Prasetyani-Gieseler M. A., et al. (2016). Community deworming alleviates geohelminth-induced immune hyporesponsiveness. Proc. Natl. Acad. Sci. 113, 12526–12531. doi: 10.1073/pnas.1604570113
Weatherhead J., Cortés A. A., Sandoval C., Vaca M., Chico M., Loor S., et al. (2017). Comparison of cytokine responses in Ecuadorian children infected with giardia, ascaris, or both parasites. Am. J. Trop. Med. Hyg 96, 1394–1399. doi: 10.4269/ajtmh.16-0580
Weng M., Huntley D., Huang I.-F., Foye-Jackson O., Wang L., Sarkissian A., et al. (2007). Alternatively activated macrophages in intestinal helminth infection: effects on concurrent bacterial colitis. J. Immunol. 179, 4721–4731. doi: 10.4049/jimmunol.179.7.4721
Wescott R. B., Todd A. C. (1966). Interaction of nippostrongylus brasiliensis and influenza virus in mice. i. influence of the nematode on the virus. J. Parasitol. 52, 242–247. doi: 10.2307/3276478
Wiria A. E., Hamid F., Wammes L. J., Kaisar M. M. M., May L., Prasetyani M. A., et al. (2013). The effect of three-monthly albendazole treatment on malarial parasitemia and allergy: a household-based cluster-randomized, double-blind, placebo-controlled trial. PloS One 8, e57899. doi: 10.1371/journal.pone.0057899
Wolday D., Mayaan S., Mariam Z. G., Berhe N., Seboxa T., Britton S., et al. (2002). Treatment of intestinal worms is associated with decreased HIV plasma viral load. JAIDS J. Acquired Immune Deficiency Syndromes 31, 56. doi: 10.1097/00126334-200209010-00008
Wondmieneh A., Gedefaw G., Alemnew B., Getie A., Bimerew M., Demis A. (2020). Intestinal parasitic infections and associated factors among people living with HIV/AIDS in Ethiopia: a systematic review and meta-analysis. PloS One 15, e0244887. doi: 10.1371/journal.pone.0244887
World Health Organization (2016) Global tuberculosis report 2016 (World Health Organization). Available at: https://apps.who.int/iris/handle/10665/250441 (Accessed March 31, 2023).
World Health Organization (2022) Schistosomiasis and soil-transmitted helminthiases: progress report 2021. Available at: https://www.who.int/publications-detail-redirect/who-wer9748-621-632 (Accessed March 30, 2023).
World Health Organization (2023) Soil-transmitted helminth infections. Available at: https://www.who.int/news-room/fact-sheets/detail/soil-transmitted-helminth-infections (Accessed March 30, 2023).
Xiao P.-L., Zhou Y.-B., Chen Y., Yang Y., Shi Y., Gao J.-C., et al. (2015). Prevalence and risk factors of ascaris lumbricoides (Linnaeus 1758), trichuris trichiura (Linnaeus 1771) and HBV infections in southwestern China: a community-based cross sectional study. Parasit Vectors 8, 661. doi: 10.1186/s13071-015-1279-2
Yordanova I. A., Jürchott K., Steinfelder S., Vogt K., Krüger U., Kühl A. A., et al. (2022). The host peritoneal cavity harbors prominent memory Th2 and early recall responses to an intestinal nematode. Front. Immunol. 13. doi: 10.3389/fimmu.2022.842870
Zaini A., Good-Jacobson K. L., Zaph C. (2021). Context-dependent roles of b cells during intestinal helminth infection. PloS Negl. Trop. Dis. 15, e0009340. doi: 10.1371/journal.pntd.0009340
Zaph C., Cooper P. J., Harris N. L. (2014). Mucosal immune responses following intestinal nematode infection. Parasite Immunol. 36, 439–452. doi: 10.1111/pim.12090
Zeukeng F., Tchinda V. H. M., Bigoga J. D., Seumen C. H. T., Ndzi E. S., Abonweh G., et al. (2014). Co-Infections of malaria and geohelminthiasis in two rural communities of nkassomo and vian in the mfou health district, Cameroon. PloS Negl. Trop. Dis. 8, e3236. doi: 10.1371/journal.pntd.0003236
Keywords: Ascaris, Trichuris, hookworm, nematode, virus, bacteria, protozoa
Citation: Schlosser-Brandenburg J, Midha A, Mugo RM, Ndombi EM, Gachara G, Njomo D, Rausch S and Hartmann S (2023) Infection with soil-transmitted helminths and their impact on coinfections. Front. Parasitol. 2:1197956. doi: 10.3389/fpara.2023.1197956
Received: 31 March 2023; Accepted: 11 May 2023;
Published: 24 May 2023.
Edited by:
Sheila Donnelly, University of Technology Sydney, AustraliaReviewed by:
Subash Babu, International Centers for Excellence in Research (ICER), IndiaLisa A. Reynolds, University of Victoria, Canada
Copyright © 2023 Schlosser-Brandenburg, Midha, Mugo, Ndombi, Gachara, Njomo, Rausch and Hartmann. This is an open-access article distributed under the terms of the Creative Commons Attribution License (CC BY). The use, distribution or reproduction in other forums is permitted, provided the original author(s) and the copyright owner(s) are credited and that the original publication in this journal is cited, in accordance with accepted academic practice. No use, distribution or reproduction is permitted which does not comply with these terms.
*Correspondence: Josephine Schlosser-Brandenburg, am9zZXBoaW5lLnNjaGxvc3NlckBmdS1iZXJsaW4uZGU=