- 1Department of Pharmacology, Faculty of Medicine, Universiti Kebangsaan Malaysia, Kuala Lumpur, Malaysia
- 2State Key Laboratory of Oncogenes and Related Genes, Renji-Med X Clinical Stem Cell Research Center, Department of Urology, Ren Ji Hospital, School of Medicine, Shanghai Jiao Tong University, Shanghai, China
Lithium, the lightest natural-occurring alkali metal with an atomic number of three, stabilizes the mood to prevent episodes of acute manic and depression. Multiple lines of evidence point to lithium as an anti-suicidal, anti-viral, anti-cancer, immunomodulatory, neuroprotective and osteoprotective agent. This review article provides a comprehensive review of studies investigating the bone-enhancing effects of lithium and its possible underlying molecular mechanisms. Most of the animal experimental studies reported the beneficial effects of lithium in defective bones but not in healthy bones. In humans, the effects of lithium on bones remain heterogeneous. Mechanistically, lithium promotes osteoblastic activities by activating canonical Wingless (Wnt)/beta (β)-catenin, phosphatidylinositol 3-kinase (PI3K)/protein kinase B (Akt) and bone morphogenetic protein-2 (BMP-2) transduction pathways but suppresses osteoclastic activities by inhibiting the receptor activator of nuclear factor-kappa B (RANK)/receptor activator of nuclear factor-kappa B ligand (RANKL)/osteoprotegerin (OPG) system, nuclear factor-kappa B (NF-κB), mitogen-activated protein kinase (MAPK), and calcium signaling cascades. In conclusion, lithium confers protection to the skeleton but its clinical utility awaits further validation from human clinical trials.
Introduction
Bone-related conditions, such as osteoporosis, fracture and bone defect, impose an enormous burden on individuals and society in terms of incidence, prevalence and healthcare cost. The key to maintaining healthy bones is to attain an optimal peak bone mass during growth and reduce the rate of bone loss during adulthood (Bonjour et al., 2009). Some best practices for maintaining healthy bones include adopting a well-balanced diet, ensuring optimal intakes of calcium and vitamin D and performing weight-bearing physical activities. In terms of treatment for skeletal diseases, research on developing novel therapeutics and repurposing drugs are currently underway to identify drugs with greater efficacy but lesser side effects.
Lithium is a recognised treatment for bipolar disorder (Tondo et al., 2019). It helps to reduce the severity and frequency of acute mania and depression and stabilize mood between episodes (Gelenberg and Hopkins, 1993). Apart from that, maintenance lithium acts as a prophylaxis of recurrent mood disorders (Davis et al., 1999). Multiple assets of lithium have been explored beyond the mood-stabilizing effect such as anti-suicidal (Lewitzka et al., 2015), anti-viral (Zhai et al., 2019), anti-cancer (Li et al., 2014), immunomodulatory (Yang et al., 2019), neuroprotective (Forlenza et al., 2014), and skeletal-promoting properties (Zamani et al., 2009; Li et al., 2017a), which further substantiate the clinical usefulness of lithium. The latter effect is the main focus of this review.
Lithium is a specific inhibitor of glycogen synthase kinase-3 beta (GSK3β) via two important mechanisms whereby it directly inhibits GSK3β by competition with magnesium ions and indirectly inhibits GSK3β via serine phosphorylation (Eldar-Finkelman and Martinez, 2011). Since its discovery four decades ago as a protein kinase that phosphorylates and inhibits glycogen synthase, GSK3β has been demonstrated to be a point of convergence for multiple cell signaling pathways involved in physiological processes (Embi et al., 1980; Wang et al., 2011). For instance, GSK3β plays a functional role in Wingless (Wnt)/beta (β)-catenin, phosphatidylinositol 3-kinase (PI3K), and nuclear factor-kappa B (NF-κB) signaling pathways (Wang et al., 2011). Intriguingly, these signal transduction pathways have been implicated in the regulation of bone metabolism and homeostasis thus suggesting the concept of lithium as a potential osteoprotective agent.
The purpose of the current review is to provide data showing the bone-protecting effects of lithium in animals and humans. The potential mechanisms of action underlying its bone-sparing effects are also described. We hope to provide an overview of the effectiveness and efficacy of lithium against bone-related disorders to encourage its greater use of lithium apart from the established anti-manic property.
Evidence Acquisition
The literature search was performed from November 15, 2019 until December 15, 2019 with PubMed and Medline electronic databases using query string “lithium AND (bone OR osteoporosis OR fracture OR osteoblast OR osteoclast OR osteocyte)”. The titles and abstracts were screened and relevant full-text articles were retrieved. A total of 40 original research articles inclusive of preclinical experimental evidence and human epidemiological data were included in this review.
Effects of Lithium on Bone: Evidence From In Vivo Studies
The effects of lithium on bone have been widely established in various types of animals, including rodents, goats, rabbits, dogs, and chickens. The in vivo models utilised by investigators vary between studies, including the use of animals subjected to surgical castration, chemical castration, bone defects, and/or fractures, genetically senescence animals, knockout animals, as well as normal healthy animals (Table 1).
The bone-protecting effects of lithium have been evaluated in bone fracture models. Loiselle et al. (2013) created a closed femur fracture by three-point bending in two strains of mice, namely female wild-type mice (Col1-Cre- Cx43flox/flox) and Connexin 43 (Cx43) conditional knockout mice (Col1-Cre+ Cx43flox/flox). Connexin 43, the most abundant gap junction protein in bone, is highly expressed during fracture healing as it helps in osteoblastic proliferation, differentiation and survival. The lack of Cx43 results in impaired bone formation and healing. The fractured animals were orally treated with lithium chloride (LiCl) at the dose of 200 mg/kg starting from day 4 post-fracture. They reported lower mineral apposition rate (MAR), bone formation rate (BFR), bone volume (BV)/total volume (TV), collagen type I alpha 1 (Col1α1), bone morphogenetic protein-2 (BMP-2), and osteocalcin (OCN) expressions in the Cx43 conditional knockout fractured mice compared to the wild-type fractured mice. Impaired osteoclastogenesis and functional deficits in healing were observed in the Cx43 conditional knockout fractured mice. Supplementation with LiCl rescued Cx43 conditional knockout fractures by increasing BV/TV, ultimate torque at failure and torsional rigidity (Loiselle et al., 2013). In another study, female Sprague-Dawley rats underwent closed midshaft unilateral femoral fractures using a blunt guillotine driven by a dropped weight. Fracture healing was maximized with oral administration of lithium (20 mg/kg) initiated seven days after fracture for 2 weeks. There was a 46% increment in maximum yield torque in the lithium-treated group compared to the non-treated controls (Bernick et al., 2014). In a recent study, closed diaphyseal fractures were performed on female Sprague-Dawley rats by dropping a ~300 g load on the right femur perpendicular to its longitudinal axis at the mid-shaft, followed by oral administration of LiCl for two weeks. The primary finding of this study was the higher maximum yield torque in the LiCl-treated animals than the vehicle-treated controls (Vachhani et al., 2018a).
Several investigators have demonstrated the protective effects of lithium on bone defects. By definition, bone defect refers to the structural loss of bone tissue where bone should normally exist. It is often sequelae of trauma, tumour or infection (Stewart et al., 2015). In an in vivo study, a bone defect (5 mm in length, 1.5 mm in width and 1 mm in depth) was made 6 mm below the knee joint of male Wistar rats and filled with BD Matrigel™ basement membrane matrix with lithium carbonate (Li2CO3, 10 mM) for 14 days. Micro-computed tomography (Micro-CT) analysis and bone histomorphometry were performed in the intracortical- and the endocortical-formation area. The osteoclast number (Oc.N) was significantly decreased but the percentage of lamellar BV was significantly increased, reiterating the acceleration of bone regeneration in promoting high-intensity bone formation (Arioka et al., 2014). In adult male goats, a symmetrical 10 mm round bone defect was introduced to the tibial facies medialis and the defect was filled with lithium-incorporated deproteinized bovine bone (Li-DBB) scaffold. Qualitatively, it was found that callus was formed in the defect region with dense and normal morphology of trabeculae in the Li-DBB group after 12 weeks. Quantitatively, it was noted that the mean gray values, mean pixel value, calcified callus BV, trabecular thickness (Tb.Th) and mean osteogenic area were significantly higher in bone defects filled with Li-DBB scaffold compared to those filled with deproteinized bovine bone (DBB) scaffold without lithium (Guo et al., 2018). In the same year, Li et al. (2018) examined the bone defect repairing effects of nano-lithium-hydroxyapatite (Li-nHA) scaffold in glucocorticoid-induced osteonecrosis of the femoral head in adult male Japanese white rabbits. Briefly, the rabbits were intravenously injected with lipopolysaccharide (LPS) followed by three intramuscularly injections of methylprednisolone acetate (20 mg/kg, time interval of 24 hours) into the right gluteus medius muscle after 24 hours. The femoral head defect was created and filled with Li-nHA scaffold. Micro-CT analysis showed that the Li-nHA group showed moderate defect repair, confirmed by the quantitative analysis expressed as higher values of bone volume and bone density as compared to the controls. Findings from histological detection also showed that the Li-nHA group presented a larger new bone area than the control animals (Li et al., 2018). More recently, lithium-doped calcium polyphosphate (Li-CPP) was fabricated by Ma and co-researchers to investigate its osteogenic potential. A bilateral tibial bone defect (5 mm in diameter and 3 mm in thickness) was built in adult male Japanese white rabbits and Li-CPP scaffold was implanted. Li-CPP scaffold was shown to enhance the formation of bone area. Histologically, there were mature interconnected new bones and irregular bone trabecular formed in the Li-CPP group (Ma et al., 2019).
Apart from that, the anti-osteoporotic effects of lithium have been evaluated in vivo. Ovariectomized rat model (the removal of both ovaries in female rat) remains the best validated animal model to mimic the clinical characteristics of postmenopausal osteoporosis in humans (Kalu, 1991). Using an osteoporosis rat model induced by ovariectomy, LiCl alone or in combination with LY294002 (a strong inhibitor of PI3K) was locally injected to the distal femoral condyle at the first and 30th days post-surgery. Serum level of bone alkaline phosphatase (BALP) was raised but the levels of procollagen type I N-terminal propeptide (PINP), tartrate-resistant acid phosphatase 5b (TRACP-5b) and C-terminal telopeptide of type 1 collagen (CTX) were lowered in the ovariectomized rats treated with LiCl alone. The combination of LiCl and LY294002 provided beneficial effects at a greater extent compared to LiCl alone. Similar outcomes were seen in the trabecular microarchitectural quantitative analysis of the femur in the ovariectomized rats, whereby the combination was better than the treatment alone (Bai et al., 2019). The ovariectomized rat model with fracture or bone defect was also used to assess the bone-sparing effects of lithium. Vachhani et al. (2018b) performed bilateral ovariectomy and a closed right femoral diaphyseal fracture in female Sprague-Dawley rats. Treatment with lithium at 20 mg/kg was initiated at day 10. The results indicated that lithium significantly improved healing at week 6 after fracture, as evidenced by higher maximum yield torque and torsional stiffness. Qualitatively, LiCl-treated femur displayed better periosteal and mineralized callus bridging (Vachhani et al., 2018b). In another study, a bone defect (diameter: 2.5 mm; depth: 4 mm) at the medial aspect to the tibial shaft was created after bilateral ovariectomy and it was filled with 50 or 100 mM LiCl doped to calcium phosphate cement (CPC). Data obtained from micro-CT showed more extensive newly-formed bone occurred and higher BV/TV at 8 weeks after the implantation of CPC with LiCl as compared to those without LiCl, indicating a better capacity of LiCl for bone regeneration (Li et al., 2017a). LiCl was also found to enhance implant osseointegration, implant fixation and bone regeneration in osteoporotic condition. In this study, female Sprague-Dawley rats underwent ovariectomy to induce osteoporosis and titanium was implanted in the tibia after 3 months. LiCl (150 mg/kg) was given to the rats every 2 days for 3 months. The positive outcomes observed in this study were increases in number and distribution of trabeculae, bone-implant contact (BIC), BV/TV, trabecular number (Tb.N), Tb.Th, connectivity density (Conn.D), osseointegration (OI), maximum push-out force, implant-bone interface shear strength, as well as a decrease in trabecular separation (Tb.Sp) in the LiCl-treated animals (Jin et al., 2017).
Recently, three groups of researchers delineated the effects of lithium on osteogenic integration using male New Zealand white rabbits and male beagle dogs. In an earlier study, Liu et al. (2018) fabricated an entangled titanium wire porous scaffold with a lithium-containing nanoporous coating (0.01 or 0.02 mol/L) by micro-arc oxidation (Li-MAO-ETP). Two holes (3 mm) were generated at the femoral shaft of rabbits and Li-MAO-ETP implants were inserted. The results indicated that the bone with Li-MAO-ETP implants had a higher push-out load, bone ingrowth and bone mineralization than the bone with MAO-ETP implants after 12 weeks of implantation (Liu et al., 2018). Huang et al. (2019) implanted lithium-incorporated sandblasted, large-grit, and acid-etched (Li-SLA) titanium discs into the trabecular bone of femur condyle in rabbits. In their study, osseointegration and new bone formation were assessed using micro-CT after 2, 4, and 8 weeks of implantation. More osteoids and new bone were covering the implants and higher BV/TV and BIC were detected in the Li-SLA group (Huang et al., 2019). Using a bigger animal model, Zhang and colleagues assessed the effects of lithium-doped silica nanospheres and poly(dopamine) composite coating on polyetheretherketone (LPPK) implantation on bone integration. The amount of newly formed bone tissues, BV/TV, Tb.N, Tb.Th, failure load and BIC in the LPPK group were higher compared to their respective controls (Zhang et al., 2018b).
In addition, the effects of lithium on distraction osteogenesis after osteotomy have been also evaluated. Distraction osteogenesis refers to a surgical process to stimulate new bone formation by cutting and gradually separating two bony fragments, allowing bone healing process between the gap (Neelakandan and Bhargava, 2012). Wang and co-researchers treated the rats subjected to osteotomy and distraction osteogenesis with LiCl (200 mg/kg/day) intragastrically. The LiCl-treated animals displayed higher OCN, BV/TV, Tb.N, Tb.Th, Tb.Sp, bone mineral density (BMD) and stiffness with more mature new bone tissue and bone fragments were completely fused in the distracted tibia (Wang et al., 2015). Using a mouse model of titanium particle-induced calvarial osteolysis, Hu et al. (2017) found that intraperitoneal (i.p.) injection of 50 or 200 mg/kg LiCl for 2 weeks increased BMD, BV/TV as well as decreased eroded surface (ES), Oc.N, pore number and area of porosity in mice calvaria (Hu et al., 2017).
In an in vivo study by Clement-Lacroix et al. (2005), LiCl was gavage-fed to three different strains of 8-week-old mice [low-density lipoprotein receptor-related protein 5 (Lrp5)-knockout (Lrp5-/-), senescence-accelerated mouse prone 6 (SAMP6) and C57BL/6 mice] and the effects of LiCl on bone metabolism were assessed after 4 weeks of therapy. The animals lacking the Lrp5 without LiCl treatment had reduced bone quality and treatment with LiCl improved the condition [evidenced by increased BV/TV, Tb.N, osteoid thickness (O.Th), osteoblast number (Ob.N), MAR, BFR, and reduced adipocyte number (Ad.N)]. Similar outcomes were detected in the other two mice strains treated with LiCl, whereby bone microstructure was improved and Ob.N was increased (Clement-Lacroix et al., 2005).
On the contrary, several groups of researchers reported negative or negligible effects of lithium on bone in healthy animals. In female Wistar rats, the animal receiving 45 mg/kg Li2CO3 in their drinking water for 3 months showed no significant differences in the serum levels of calcium, phosphorus and alkaline phosphatase (ALP). In comparison to the control animals, bone histomorphometric analysis indicated the reductions in BV/TV, Tb.N, and lining cell surface (Lc.S) as well as increases in Tb.Sp and ES in the Li2CO3-treated animals (Lewicki et al., 2006). Comparable outcomes were identified using healthy female Holtzman rats. Daily administration of LiCl (4 meq/kg, i.p.) for 4 weeks resulted in significant decreases in periosteal mineralization rate and volume of osteoid present in the tibia relative to the control animals. Levels of calcium, phosphorus, ALP, parathyroid hormone (PTH), and creatinine in serum were similar among the two experimental groups (Baran et al., 1978). In another study using growing broiler chickens as the animal model, oral supplementation of 20 mg/kg LiCl were initiated at 1 week or 3 weeks of age. The animals were euthanized at 6 weeks of age and bones were harvested for further analysis. Three-point bending test revealed that the LiCl-treated chickens had lower stiffness in the femur and lower energy to fracture in the tibia as compared to the controls. There were no alterations in parameters measured by micro-CT (Harvey et al., 2015). In one of the earlier studies, Henneman and Zimmerberg found that LiCl-supplemented drinking water (1.43 ± 0.13 meq/day) did not cause any changes in the serum electrolytes (total calcium and inorganic phosphorus), metaphyseal bone composition (calcium, collagen and mineral salts contents), and bone metabolism (amount of neutral-salt-soluble collagen, resorption rate of collagen, formation rate of collagen and total incorporation of proline into bone matrix) in female Sprague Dawley rats (Henneman and Zimmerberg, 1974). Moreover, Bellwinkel et al. (1975) reported no significant deviations in serum calcium, magnesium, and phosphorus levels of female albino rats after daily subcutaneous (s.c.) injection of a lithium-containing solution (0.1 or 0.5 mM) for 6 weeks. The calcium and magnesium content in liver, skeletal muscle and femur were also unaltered in rats by chronic lithium administration (Bellwinkel et al., 1975).
In summary, the accumulated in vivo evidence suggests the promising osteoprotective effects of lithium in defective bones and osteoporotic condition. However, lithium seems to have negligible or detrimental effects to healthy bones. Generally, lithium is administered to the animals via oral gavage, injections (i.p. or s.c.) or fabricated into scaffolds to be filled into the bone defects. Since lithium potentially helps in protecting bone in vivo, we would like to further look into the impact of lithium on bone in humans.
Effects of Lithium on Bone: Evidence From Human Studies
The effects of lithium on bone have been investigated by researchers since decades ago but the findings are inconclusive. Some studies described the negative, negligible or positive effects of lithium on the skeletal system (Table 2). These human studies can be divided into epidemiology studies on the effects of lithium treatment in psychiatric patients, in which parameters related to bone were measured concomitantly and clinical trials specifically to study the effects of lithium on bone. Eren et al. (2006) pinpointed that treatment with Li2CO3 reduced BMD of the L2–L4 vertebrae and femur neck in the patients with bipolar disorder (n=15; aged 38.67 ± 8.23 years). Meanwhile, no changes were observed in the BMD of Ward’s triangle and femur trochanter in the Li2CO3-treated patients (Eren et al., 2006). Plenge and Rafaelsen (1982) assessed the effects of Li2CO3 treatment on calcium, magnesium, and phosphate balance as well as bone mineral content (BMC) in manic-melancholic patients. Two groups of patients were enrolled: (a) patients who were to start a Li2CO3 treatment and (b) patients who were to terminate a long-term Li2CO3 treatment. The excretion of calcium and phosphate were decreased but the excretion of magnesium was increased when treatment was initiated. Paradoxical outcomes were seen in patients who have terminated the treatment. However, the BMC of patients was found to decrease during the first 6 months of treatment. At that point, the authors could not clarify the positive balance of bone mineral and the slight demineralization observed in the patients (Plenge and Rafaelsen, 1982). In another study, 23 patients with affective disorders (women: aged 25–47 years; men: aged >25 years) were involved in the study conducted by Cohen et al. (1998). This study aimed to assess the short-term (0.4–1.0 year) or long-term (> 3 years) effects of Li2CO3 therapy on parameters of bone metabolism. There was no significant difference in all the bone-related blood and urine biochemical parameters as well as BMD between the two groups of subjects. The limitation of this study was the lack of longitudinal data, thus the comparison of bone metabolism parameters before and after treatment was not performed (Cohen et al., 1998). Another two-year prospective longitudinal study involving patients with bipolar affective disorder in manic phase or depressive phase and major depression (n=53, aged 16–63 years) showed that serum PTH level was significantly higher but creatinine clearance, phosphate excretion (24UPO4E), serum creatinine, ALP, albumin, inorganic phosphate (PO4), serum total calcium concentration adjusted for albumin (alb-adj Ca), and maximum tubular reabsorption of phosphate in relation to glomerular filtration rate (TmP/GFR) levels were not significantly different after 24 months of lithium therapy. However, the BMC and BMD of the subjects were not evaluated in this study (Mak et al., 1998). A nationwide population-based cohort study in Taiwan also found that bipolar disorder was associated with increased risk of fracture and the use of lithium [≥28 defined daily dose (DDD)] did not further increase the risk of fracture [hazard ratio (HR)=0.83; 95% CI 0.56–1.25] among subjects with bipolar disorder (n=3705; aged ≥16 years) (Su et al., 2017).
The protective effects of lithium on skeleton have also been demonstrated by researchers. A cross-sectional study performed by Nordenstrom et al. (1994) aimed to investigate the effects of long term lithium treatment (≥10 years) on PTH, ionized and total calcium levels in patients with manic-depressive psychosis (n=26; women: aged 53 ± 10 years, men: aged 48 ± 9 years). Higher ionized calcium level, BMD for the whole body, lumbar spine, and femoral neck but not significantly higher PTH level were detected in lithium-treated patients in relative to the normal healthy subjects (Nordenstrom et al., 1994). Vestergaard et al. (2005) designed a case-control study to examine fracture risk among users of lithium. Subjects with any fracture sustained during the year 2000 (n=124,655; aged 1–100 years) and their matching controls (n=373,962; aged 1–100 years) were recruited. Findings from the study found that lithium treatment was associated with decreased risk of any fracture [individual with 250–849 DDD of Li: odd ratio (OR)=0.74; 95% confident interval (95% CI): 0.60–0.92; individual with ≥850 DDD of Li: OR=0.67; 95% CI: 0.55–0.81]. Significant decreases in Colles’ (OR=0.57; 95% CI: 0.35–0.94) and spine fractures (OR=0.32; 95% CI: 0.11–0.95) were also observed in individuals with ≥850 DDD of lithium (Vestergaard et al., 2005). Another case-control study was performed within the United Kingdom General Practice Research Database to compare the use of lithium among patients with a sustained fracture and their matched controls. The results indicated that the current users of lithium (defined as those who received a lithium prescription within 2 months before the index date) had a decreased risk of fractures. Meanwhile, past users of lithium (defined as those who received the last lithium prescription more than 12 months prior to index date) had an increased risk of fractures, increasing with time since discontinuation. However, there was no association between fracture risk and cumulative duration of lithium use (Wilting et al., 2007). Subsequently, a case-control study reported that current lithium use was associated with a lower likelihood of osteoporotic fracture (OR=0.63; 95% CI 0.43–0.93) among individuals aged 50 years and above (n=15,792) (Bolton et al., 2008). In a study conducted by Zamani et al. (2009), patients on lithium maintenance therapy for more than one year (n=75; aged 37 ± 9.6 years) were enrolled to assess the effects of lithium on bone mass. The compliance of lithium therapy was assured by serum lithium level in the recommended therapeutic range (0.6–1.2 mmol/L). Results obtained from this study showed that bone density at the spine, femoral neck and trochanter of lithium-treated patients was higher than those in the controls. The serum ALP, CTX, and OCN were significantly lower in the lithium-treated patients as compared to the controls whereas the total calcium, PTH and urinary calcium excretion were not statistically different between the two groups (Zamani et al., 2009). In Canada, a cohort study involving 68730 individuals (62275 women and 6445 men; aged 64.2 ± 11.2 years) showed that lithium medication was associated with reduced risk of major osteoporotic fracture (HR=0.82; 95% CI 0.46–1.44) (Bolton et al., 2017).
Among the human studies included in this review, seven studies reported positive effects of lithium whereas other studies showed negative or negligible outcomes on the bone. The distinct outcomes observed in these human studies might be due to variations of study design, study population, study duration, population size, treatment duration and measurement of bone parameters. In most of the studies included, the dose of lithium prescribed to the patients was not mentioned. Therefore, it is challenging to speculate the optimum dose of lithium on bone whether it is higher, same or lower than the dose for psychiatric disorders. Recently, a comprehensive systematic review and meta-analysis was done to explore the effects of lithium on BMD and fracture risk using seven observational studies (Nordenstrom et al., 1994; Vestergaard et al., 2005; Wilting et al., 2007; Bolton et al., 2008; Zamani et al., 2009; Bolton et al., 2017; Su et al., 2017). The results indicated that lithium medication was significantly associated with a 20% decrease in the risk of fractures (Liu et al., 2019). In view of the beneficial effects of lithium on bone in the majority of the studies with the exception in few studies, further discussion pertaining the effects of lithium on bone cells in vitro and the understanding of its mechanisms of action is indispensable.
The Underlying Molecular Mechanisms of Lithium in Protecting Bone Health
The integrity of the skeleton is maintained by the balanced activities of new bone formation by osteoblasts and resorption of mineralized bone matrix by osteoclasts. Bone formation (also known as osteogenesis or ossification) is a multi-step process beginning with the proliferation of progenitor cells into osteoblastic lines, followed by osteoblast differentiation, matrix maturation and mineralization (Setiawati and Rahardjo, 2018). Markers that are associated with osteoblast differentiation and bone formation include type I collagen (COL1), ALP, OCN, bone sialoprotein (BSP), osterix (OSX), and runt-related transcription factor 2 (Runx-2) (Chapurlat and Confavreux, 2016; Wong et al., 2019). On the other hand, bone resorption is a process of breaking down bone tissues by osteoclasts releasing minerals into the bloodstream. Osteoclastogenesis (osteoclast formation) is a complex sequential process that requires the commitment of pluripotent haematopoietic stem cells and its proliferation towards osteoclast precursors mediated by macrophage-colony stimulating factor (M-CSF) as well as the differentiation of osteoclast precursors into multinucleated and mature cells mediated by receptor activator of nuclear factor-kappa B ligand (RANKL) (Yavropoulou and Yovos, 2008). Mature osteoclasts attach themselves to the bone surfaces, followed by secretion of hydrochloric acid and cathepsin K (CTSK, a matrix-degrading collagenase) to resorb bone effectively (Boyce et al., 2009). Osteoclast number, resorption pit area, and tartrate-resistant acid phosphatase (TRAP) activity are often used to assess the bone resorption ability of osteoclasts (Bai et al., 2019). In vitro studies on the effects of lithium on bone cells have focused on its capability in modulating osteoblastogenesis and osteoclastogenesis (Table 3).
Effects of Lithium on Osteoblastogenesis
Bone marrow-derived mesenchymal stem cells (MSCs) are progenitor cells capable of proliferation and differentiation into mature osteoblasts (Zomorodian and Baghaban Eslaminejad, 2012; Dai et al., 2015). Incubation of osteoblast precursor cells or mature osteoblasts with LiCl stimulated markers of osteoblast differentiation (as shown by increased ALP, COL1, and Runx-2) and enhanced calcium deposition (as shown by increased Alizarin red staining) (Clement-Lacroix et al., 2005; Arioka et al., 2014; Li et al., 2018; Bai et al., 2019; Yang et al., 2019). Two studies measured the osteogenic effects of Li-SLA treated titanium discs by seeding them with murine bone marrow mesenchymal stem cells (MSCs) or osteoblastic MC3T3 cells. As compared to the SLA group, Li-SLA treated titanium discs was beneficial for osteoblastic proliferation (assessed by higher expression of ALP, OCN, COL1, and Runx-2) (Galli et al., 2013; Huang et al., 2019). In another study, Zhang J. et al. (2018a) cultured rat bone marrow MSCs with lithium-doped mesoporous silica nanospheres. The release of lithium ions from lithium-doped mesoporous silica nanospheres enhanced the expression of osteogenesis-related genes, such as Runx-2, ALP, OPN, and OCN (Zhang et al., 2018a). The same group of researchers also reported significant stimulatory effects of LPPK on cell proliferation and ALP activity of rat bone marrow stromal cells (Zhang et al., 2018b). A trace amount of lithium doped onto CPC has demonstrated the characteristics of enhanced osteoblastic proliferation, and differentiation, shown by increased ALP activity, osteogenic markers (COL1α1, OCN, OPG, and Runx-2), and mineralization (Li et al., 2017a). Using human osteosarcoma (MG63) cells, several studies in recent years also reported that lithium had promising effects on osteoblast proliferation and differentiation. Lithium-doped CPC had high biocompatibility, supported osteoblast attachment and growth, and promoted cell proliferation and differentiation in MG-63 cells (Li et al., 2017b). It was noteworthy that MG-63 cells cultured on bone substitute coated with lithium had higher cell viability, cell proliferation, cell differentiation, calcium deposition, and expression of osteogenesis-related genes (Guo et al., 2018; Liu et al., 2018; Ma et al., 2019). Besides, the percentage of apoptotic calvarial osteoblast cells derived from Lrp5-knockout mice was significantly decreased after LiCl treatment (Clement-Lacroix et al., 2005).
Effects of Lithium on Osteoclastogenesis
Osteoclasts are highly specialized cells derived from the monocyte or macrophage lineage of the bone marrow (Tevlin et al., 2014). Several groups of researchers investigated the effects of lithium on osteoclastogenesis using bone marrow macrophages induced with M-CSF and RANKL. The bone resorption pit area, Oc.N, and TRAP activity were decreased in response to LiCl (Hu et al., 2017; Bai et al., 2019). Similarly, the number of osteoclasts was also reduced in bone marrow macrophages seeded on Li-SLA coated surface (Huang et al., 2019). Using murine osteoclast precursor (RAW-D) cells incubated with RANKL, LiCl significantly decreased the number of TRAP-positive multinucleated cells and TRAP activity (Arioka et al., 2014).
In an earlier study by Pepersack et al. (1994), the researchers found that lithium at the dose of 3 mM exerted different effects on resorption stimulated by PTH, prostaglandin E2 (PGE2), interleukin (IL)-1, or 1,25-dihydroxyvitamin D3 [1,25(OH)2D3] in foetal long bones. Lithium exhibited no inhibitory effect on resorption stimulated by IL-1, followed by slight and moderate inhibitory effects on resorption by PTH and PGE2, respectively. Meanwhile, complete inhibition of resorption induced by 1,25(OH)2D3 after addition of lithium to the bone cultures. The distinct outcomes observed in this study compared to other recent studies might be due to the different approach used for the determination of bone resorption, i.e., measurement of the percentage of calcium release from foetal rat long bones (Pepersack et al., 1994).
Collectively, the potential positive outcomes of lithium observed in preclinical settings prompt a need to look into the underlying molecular action of lithium.
The Canonical Wingless (Wnt) Signaling Pathway
The canonical Wnt signal transduction pathway has been identified for its central regulatory role in bone metabolism (Houschyar et al., 2019). Activation of this signaling pathway results in the translocation of β-catenin into the nucleus for transcription of genes that belong to the T-cell factor (TCF)/lymphoid enhancer-binding factor (LEF) family. This is an osteogenic signaling cascade which is associated with the upregulation of Runx-2 expression, an essential transcription factor for osteoblast differentiation (Ling et al., 2017). In the presence of Wnt ligand, it binds to transmembrane Frizzled receptor and Lrp5/6 co-receptors. The function of multiprotein destruction complex made of axis inhibition protein 2 (Axin2), adenomatosis polyposis coli (APC), GSK3β, and casein kinase 1 alpha (CK1α) is disrupted. Eventually, the degradation of β-catenin is inhibited. The accumulation of β-catenin in cytoplasm facilitates its translocation and subsequent gene transcription. In the case whereby Wnt ligand is absent, β-catenin is sequestered into the multiprotein destruction complex, phosphorylated, ubiquitinylated, followed by the degradation by the proteasome (Houschyar et al., 2019).
In particular, the activity of Wnt signaling is constitutively suppressed by the active form of GSK3β under basal condition (Muneer, 2017). The inactivation (phosphorylation) of GSK3β leads to dephosphorylation of β-catenin and activation of Wnt signaling to promote bone anabolism (Bertacchini et al., 2018). This pathway has been regarded as the most implicated signaling mechanism in the regulation of osteoblastogenesis and bone formation by lithium due to its well-established anti-GSK3β property (Kim et al., 2013). A study by Arioka et al. (2014) examined whether GSK3β inhibitors (LiCl and SB216763) were effective in modulating osteoblastogenesis of mesenchymal progenitor C3H10T1/2 cells. Both inhibitors promoted osteoblast differentiation and caused dephosphorylation of β-catenin (Arioka et al., 2014). For bone-repairing effects, Li et al. (2018) fabricated a composited scaffold using lithium (Li-nHA) to activate Wnt signaling pathway and consequently improve osteogenic effects in bone marrow MSCs. The co-cultures of bone marrow MSCs and Li-nHA had lower expression of GSK3β and peroxisome proliferator-activated receptor-gamma (PPAR-γ) but higher expression of β-catenin (Li et al., 2018). Likewise, Western analysis revealed that β-catenin was upregulated in bone marrow MSCs seeded on titanium discs coated with Li-SLA (Huang et al., 2019). Another group of investigators also conducted in vitro experiment to examine the fracture-healing effects of lithium-doped CPC on MC3T3-E1 cells. The release of lithium ions from CPC increased the amount of phosphorylated GSK3β but decreased the amount of phosphorylated β-catenin (Li et al., 2017a). A more comprehensive study was performed to investigate the involvement of Wnt signaling. Human osteosarcoma (MG-63) cells were seeded on Li-MAO-ETP scaffold and the expression of all signaling molecules downstream the Wnt signaling was evaluated. The expressions of Lrp5, Lrp6, Axin2, β-catenin, and phosphorylated GSK3β were increased whereas the expression of phosphorylated β-catenin was decreased (Liu et al., 2018). A recent study showed similar outcomes when human osteosarcoma (MG-63) cells were cultured on Li-CPP. Cell immunofluorescence staining and Western analysis showed lower expression of GSK3β but higher expression of β-catenin. Higher content of lithium caused a further decrease in GSK3β expression and increase in β-catenin expression (Ma et al., 2019). Mechanistically, LiCl inhibited GSK3β and caused the accumulation of β-catenin in cytoplasm leading to its translocation into the nucleus and expression of Runx-2 (one of the TCF/LEF target gene products essential for osteoblast differentiation) (Arioka et al., 2014; Li et al., 2017a; Li et al., 2018; Liu et al., 2018; Ma et al., 2019).
Clement-Lacroix et al. (2005) provided strong evidence on the activation of Wnt signaling by LiCl in increasing bone formation and bone mass using Lrp5-knockout mice. The lack of Lrp5 in mice caused the failure of Wnt signaling via the canonical pathway, leading to reduced bone mass. LiCl was predicted to act downstream of this receptor to express Wnt-responsive genes and restore bone mass. In vitro, LiCl was able to increase β-catenin stabilization and induce β-catenin nuclear translocation in calvaria cells derived from Lrp5-knockout mice. The ALP activity and COL1α1 expression were also stimulated in MSCs obtained from Lrp5-knockout mice (Clement-Lacroix et al., 2005). The study of Kurgan et al. (2019) evaluated the effects of LiCl treatment (10 mg/kg) on GSK3β phosphorylation status within the bone in male C57BL/6J mice. They pointed out that lithium supplementation caused phosphorylation of GSK3β, rendering it inactive and dephosphorylation of β-catenin (Kurgan et al., 2019). Other important players in Wnt signaling include Dickkopf-related protein 1 (DKK-1) and sclerostin (SOST). They are osteocyte-derived Wnt antagonist function as putative inhibitors of Wnt signaling to block the engagement of Wnt ligand to Frizzled receptor and Lrp5/6 co-partners (Mason and Williams, 2010). In a fractured mouse model, researchers demonstrated that the expression of β-catenin was attenuated whereas the expression of SOST and GSK3β were enhanced along with the impairments in fracture healing. These alterations in fracture healing in mice were rescued by administering LiCl (Loiselle et al., 2013).
The role of Wnt signaling in the regulation of osteoblastogenesis has been generally accepted but the involvement of this pathway in osteoclastogenesis remains controversial based on several lines of evidence. The inactivation of GSK3β by RANKL is crucial for osteoclast differentiation, confirming the negative role of GSK3β in osteoclast formation (Jang et al., 2011). On the contrary, some studies reported that constitutive activation of β-catenin prevents osteoclast differentiation (Wei et al., 2011; Albers et al., 2013). The exact role of Wnt signaling in osteoclastogenesis awaits further investigation. The effects of lithium on osteoclastogenesis did not seem to mediate through Wnt signaling. A study by Arioka et al. (2014) examined whether LiCl and SB216763 were effective in modulating osteoclastogenesis of osteoclast precursor RAW-D cells in the presence of RANKL. Their findings revealed that GSK3β inhibitors suppressed osteoclast differentiation [assessed by reduced TRAP activity, number of multinucleated cells and nuclear factor of activated T-cells cytoplasmic 1 (NFATc1) expression] independently of Wnt pathway. This was mainly because Wnt3a stimulated osteoclast formation from their precursor RAW-D cells (Arioka et al., 2014). These findings were supported by another study whereby LiCl-treated bone marrow macrophages in the presence of M-CSF and RANKL displayed a reduction in osteoclast formation but treatment with a selective inhibitor of GSK3β (IM-12) showed vice versa (Hu et al., 2017). Taken together, these studies suggested that LiCl-induced inhibition of osteoclastogenesis was not affected by the Wnt signaling pathway.
The Phosphatidylinositol 3-Kinase (PI3K)/Protein Kinase B (Akt) Signaling Pathway
The PI3K/Akt signaling pathway is a pathway essential for cell survival, growth and proliferation (Shi et al., 2019). This pathway is activated by the binding of ligands (such as hormones, growth factors and extracellular matrix components) with receptor tyrosine kinase (RTK) resulting in dimerization and autophosphorylation of tyrosine residues in the cytoplasmic domain. The phosphorylated tyrosine residues recruit PI3K (consists of a regulatory p85 subunit and a catalytic p110 subunit) to convert phosphatidylinositol-4,5-diphosphate (PIP2) into phosphatidylinositol-3,4,5-triphosphate (PIP3). Next, PIP3 acts as a secondary messenger to recruit and bind to phosphoinositide-dependent kinase-1 (PDK1) and Akt. Upon binding, PDK1 phosphorylates Akt causing full activation of Akt (McGonnell et al., 2012). GSK3β is a major effector of Akt and NFATc1 is a direct substrate of GSK3β, thus activated Akt subsequently inhibits GSK3β and downregulates NFATc1 expression (Moon et al., 2012).
Bai et al. (2019) examined the role of lithium on Akt/GSK3β/β-catenin/NFATc1 signaling pathway in osteoblasts and osteoclasts. They harvested primary osteoblasts from the calvariae of 3-day-old rats and generated osteoclasts using bone marrow-derived macrophages in the presence of M-CSF and RANKL. Both osteoblasts and osteoclasts were administered with LiCl and LY294002 (a known PI3K inhibitor) alone or in combination. Treatment with LiCl or LY294002 alone caused phosphorylation of Akt and GSK3β but dephosphorylation of β-catenin in osteoblasts and osteoclasts along with other findings (the increases in ALP activity and mineralized nodules in osteoblast as well as reductions of the resorption pit area, TRAP activity and NFATc1 expression). The trend became more evident when the combination of LiCl and LY294002 was administered (Bai et al., 2019). Li and colleagues also demonstrated the role of Li-nHA in the differentiation of MSCs into osteoblasts via PI3K/Akt signaling pathway. Their mechanistic study showed that Li-nHA scaffold co-cultured with bone marrow MSCs had more Akt expression as compared to the negative control group (Li et al., 2018). The activation of PI3K/Akt pathway has direct and indirect effects on osteogenic stimulation. The indirect effect is mediated via intersection with canonical Wnt signaling.
The Bone Morphogenetic Protein-2 (BMP-2) Signaling Pathway
Bone morphogenetic proteins (BMPs), belong to transforming growth factor-beta (TGF-β) gene superfamily, are found abundantly in bone and cartilage thus important for skeletal development and tissue regeneration (Wu et al., 2016). They signal through binding with tetrameric transmembrane serine/threonine kinase receptor (BMPR) expressed on the surface of osteoblast and osteoclast precursors. The downstream intracellular signaling events are mediated through canonical suppressor of mothers against decapentaplegic (SMAD)-dependent and non-canonical SMAD-independent pathways, such as mitogen-activated protein kinase (MAPK) signaling cascade. Both of these signal transduction pathways direct the transcription of osteogenic genes (Wu et al., 2016).
The findings from studies by two groups of researchers suggested that lithium inhibited osteogenic commitment of MSCs but enhanced osteogenic differentiation at the later stage, whereby both actions were mediated via BMP-2 signaling (Li et al., 2011; Huang et al., 2019). In an earlier study by Li and colleagues, LiCl attenuated BMP-2 signaling and inhibited early stages of osteogenic differentiation. The presence of LiCl reduced mineral deposition in von Kossa staining and ALP activity in MC3T3-E1 pre-osteoblast cultures as early as day 2. The expression of osteoblast-specific transcription factors (Runx-2, OSX, Atf4, Dlx5, Alpl, Col1α1, Ibsp, Spp1, and OCN) in mouse primary osteoblasts was also reduced at day 3 but these inhibitory effects of LiCl were diminished at day 6 and completely abolished at day 9. Comparably, lithium treatment also downregulated osteogenic markers (Runx-2, OSX, Alpl, and OCN) in murine pluripotent mesenchymal (C2C12) cells (Li et al., 2011). In a recent study, it was found that bone marrow MSCs incubated on Li-SLA coated titanium discs displayed upregulation of BMP-2 after 7 days (Huang et al., 2019).
The Receptor Activator of Nuclear Factor-Kappa B (RANK)/Receptor Activator of Nuclear Factor-Kappa B (RANKL)/Osteoprotegerin (OPG) System
The RANK/RANKL/OPG signaling axis, identified in the mid-to-late 1990s, shows an essential role of osteoblasts in modulating osteoclast formation and differentiation (Boyce and Xing, 2007). The binding of RANKL to its receptor, RANK on osteoclast precursors or osteoclasts initiates signals mediated by tumour necrosis factor receptor-associated factor 6 (TRAF6) adaptor protein and promptly causes activation of various downstream signaling events, including NF-κB, MAPK, PI3K/Akt, and NFATc1 signaling cascades (Boyle et al., 2003; Lee and Kim, 2003; Luo et al., 2016). OPG is a protein secreted by osteoblasts and it acts as a decoy receptor by binding with RANKL to negatively regulate the RANK/RANKL/OPG system thus suppressing the formation, fusion, activity, and survival of osteoclasts. Several groups of researchers delineated the differential expression of OPG and RANKL (characterized by raised OPG and unchanged RANKL) in murine mesenchymal and calvaria cells after plated on Li-SLA-coated titanium discs (Galli et al., 2013; Huang et al., 2019). A similar trend was observed, whereby there was an increase in OPG but RANKL level was unaltered in the femur of male C57BL/6J mice after treated with LiCl (10 mg/kg) (Kurgan et al., 2019). Furthermore, lithium was shown to suppress TRAP activity and TRAP-positive cell, indicating the reduction of osteoclast formation in osteoclast precursor cells cultured in the presence of M-CSF and RANKL (Arioka et al., 2014; Hu et al., 2017; Bai et al., 2019; Huang et al., 2019).
NFATc1 has been characterized as a master regulator during osteoclastogenesis and it can be induced in three different stages of osteoclastogenesis (Park et al., 2017). In the early stage, the activated NF-κB in the RANK/RANKL/OPG system induces the expression of NFATc1. In the intermediate stage, NFATc1 can be further activated via calcium signaling. The cooperation between RANKL-RANK signaling and co-stimulatory receptors [triggering receptors expressed on myeloid cells 2 (TREM-2)/DNAX-activating protein of 12 kDa (DAP12) and osteoclast-associated receptor (Oscar)/Fc receptor gamma (FcRγ)] activate phospholipase Cγ2 (PLCγ2) to facilitate robust amplification of NFATc1. At the late stage of osteoclastogenesis, NFATc1 translocates into the nucleus to induce osteoclast-specific target genes for osteoclast fusion and function (Park et al., 2017). The effects of LiCl on the expression of NFATc1 were also assessed. Bone marrow macrophages stimulated by M-CSF and RANKL expressed higher levels of NFATc1 and the expression was suppressed by LiCl in dose-dependent and time-dependent manner (Bai et al., 2019).
The Regulation of Inflammatory Response via Nuclear Factor-Kappa B (NF-κB) and Mitogen-Activated Protein Kinase (MAPK) Signaling Pathways
The NF-κB and MAPK signaling play a significant role in inflammation. NF-κB represents a set of inducible transcription factors regulating the production of cytokines involved in immune and inflammatory responses (Liu et al., 2017). NF-κB, which exists in dimers, either homodimers or heterodimers, is typically sequestered in the cytoplasm by the inhibitor of NF-κB (IκBα). NF-κB can be activated by many influencers, including the interaction between RANKL, tumour necrosis factor-alpha (TNF-α) and IL-1 with their respective receptors. Eventually, NF-κB modulates the synthesis of cytokines producing a self-amplifying cycle of cytokine release and NF-κB activation (Boyce et al., 2010). MAPKs are highly conserved protein kinases that phosphorylate their dual serine and threonine residues, also known as autophosphorylation (Soares-Silva et al., 2016). The MAPK signaling can be activated in response to pro-inflammatory stimuli, leading to activation of transcription factors such as activator protein (AP-1) and subsequent expression of inflammatory genes (Yang et al., 2014). The overwhelming inflammatory response stimulated by NF-κB and MAPK activation is closely associated with perturbation of bone metabolism, hence promoting the initiation and progression of osteoporosis and other bone-related disorders.
Hu and co-authors recently reported that LiCl reduced the levels of TNF-α, IL-1β, and IL-6 with improvement in bone microstructure in a mouse model of calvarial osteolysis, indicating that LiCl effectively alleviated inflammatory response and bone loss. Their concurrent in vitro study showed that bone marrow macrophages treated with M-CSF, RANKL, and exposed to LiCl had lower osteoclast formation and osteoclast-related gene expression [such as NFATc1, CTSK, semaphorin-4A (Sema-4A) and Oscar]. The inhibitory effects of LiCl in osteoclastogenesis were mainly regulated via the inhibition of NF-κB. The phosphorylation of IκBα and NF-κB p65, as well as translocation of p65 proteins from cytoplasm to nucleus, occurred upon stimulation with RANKL alone. These changes were diminished by LiCl treatment. LiCl also suppressed the protein expression of Fos proto-oncogene (c-Fos) and NFATc1, which are the transcription factors to promote osteoclast differentiation. For other signaling pathways downstream the interaction between RANKL and RANK, Western analysis revealed that none of the subfamilies of MAPK [including the extracellular-signal-regulated kinase (ERK), c-Jun N-terminal kinase (JNK) and p38 MAPK] and PI3K/Akt was affected by LiCl (Hu et al., 2017). In a recent study, Yang et al. (2019) showed that LiCl reduced titanium nanoparticle-stimulated inflammatory response in the RAW264.7 macrophages. Specifically, titanium particle stimulation increased production of pro-inflammatory (TNF-α and IL-6) but reduced secretion of anti-inflammatory cytokines (IL-4 and IL-10). These changes were reversed in the presence of LiCl. Western blot results also revealed that titanium particle stimulation significantly increased ERK and p38 MAPK phosphorylation. The activation of these signaling events was suppressed by LiCl treatment (Yang et al., 2019).
To sum up, most of these animal and cell culture studies pointed out that lithium promoted osteoblastogenesis and bone formation but inhibited osteoclastogenesis and bone resorption. The modulation of osteogenesis is mainly through activation of Wnt, PI3K/Akt and BMP-2 signaling axis (Figure 1). The inhibition of osteoclastogenesis by lithium is orchestrated through the RANK/RANKL/OPG system and the regulation of inflammatory response via NF-κB and MAPK signaling pathways (Figure 2).
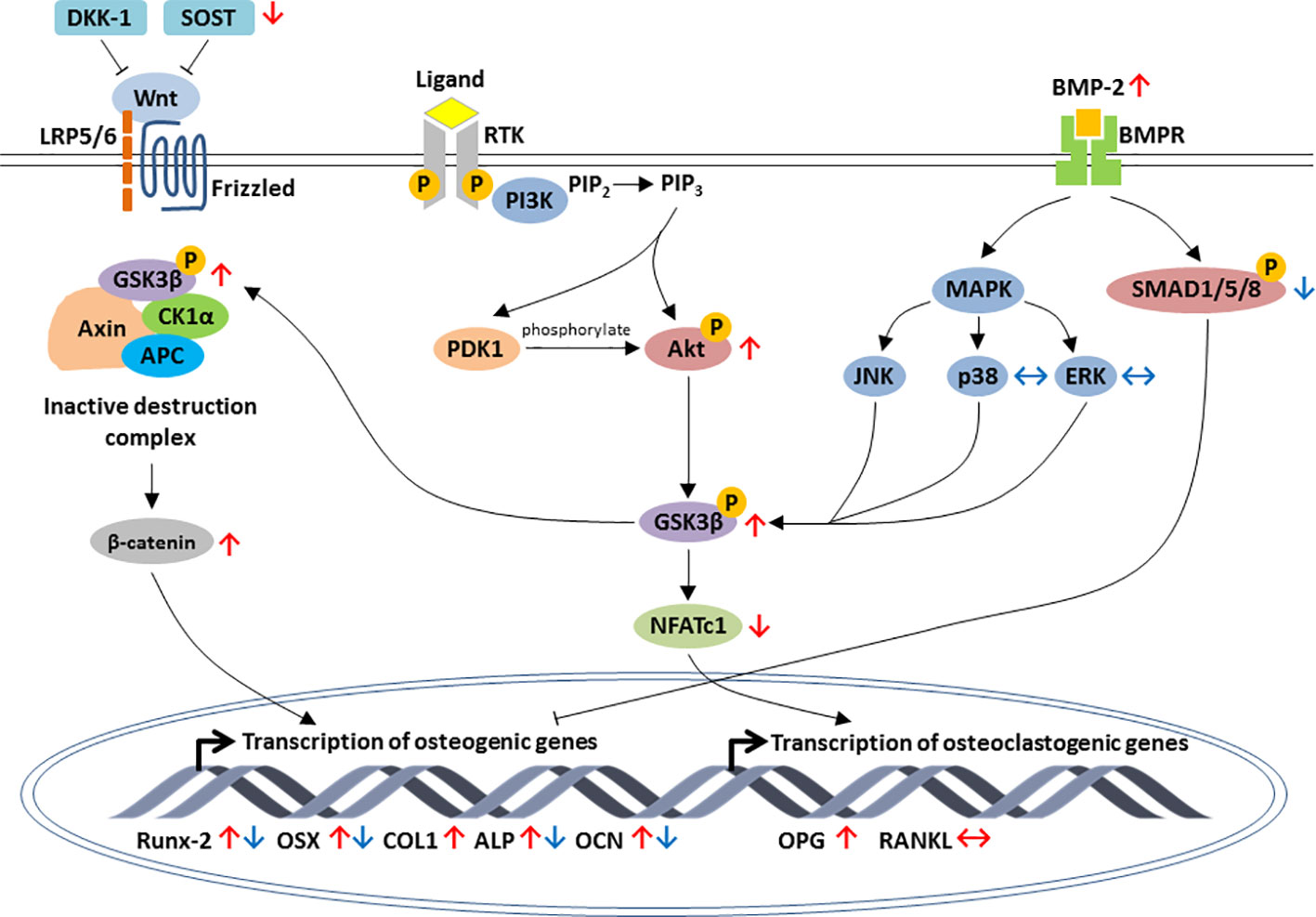
Figure 1 Schematic diagram depicting the regulation of osteoblastic-specific genes expression by lithium through activation of three major signaling pathways, including the canonical Wnt/β-catenin, PI3K/Akt and BMP-2 signaling pathways. In Wnt/β-catenin pathway, lithium inhibits the expression of sclerostin (SOST) as well as increases phosphorylation of GSK3β and accumulation of β-catenin. Through PI3K/Akt pathway, the activation of Akt by lithium leads to the inhibition of GSK3β and downregulation of NFATc1. Lithium also enhances osteogenic differentiation by increasing BMP-2 expression. The effects of lithium are indicated by red arrows. For early stage of osteogenic differentiation, lithium inhibited the expression of osteogenic markers by increasing phosphorylation of suppressor of mothers against decapentaplegics (SMADs) in BMP-2 signal transduction pathway (the effects of lithium are indicated by blue arrows). Arrow pointing upward (↑) indicates an increase and pointing downward (↓) indicates a decrease. Equivalent arrow (↔) indicates no effect.
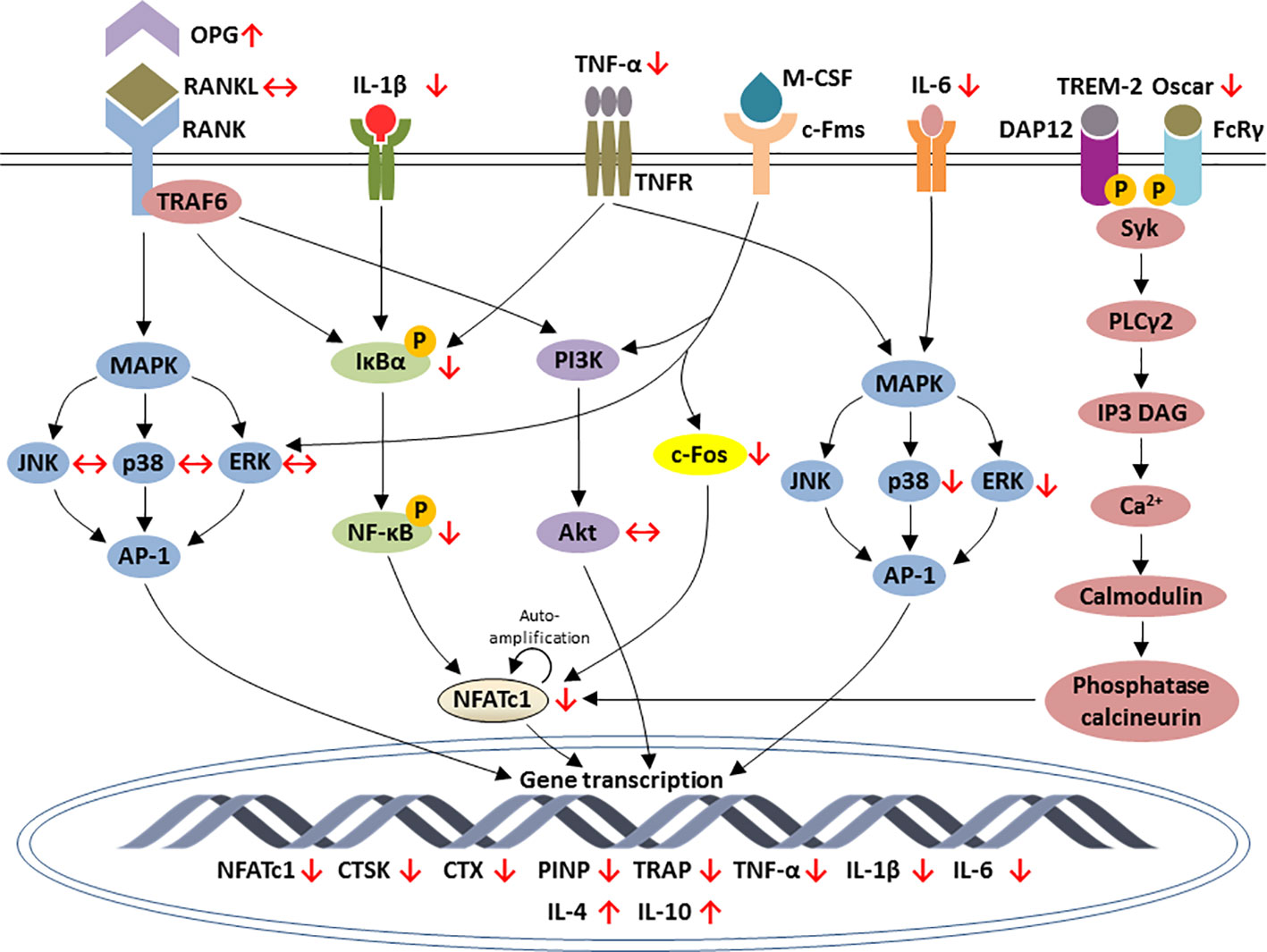
Figure 2 Schematic diagram depicting the regulation of osteoclastic-specific genes expression by lithium through macrophage-colony stimulating factor (M-CSF), nuclear factor-kappa B (RANK)/RANK ligand (RANKL)/osteoprotegerin (OPG), NF-κB, mitogen-activated protein kinase (MAPK), and calcium signaling pathways. Lithium increases the expression of OPG and reduces the inflammatory response that eventually leads to the inhibition of NF-κB and NFATc1. Lithium downregulates c-Fos, a downstream target of interaction between M-CSF and c-Fms, which is essential for osteoclast differentiation. Lithium exerts differential outcomes for MAPK signaling. The increases in TNF-α and IL-6 by lithium subsequently inhibits ERK and p38 phosphorylation but none of the subfamilies of MAPK is affected downstream the inhibition of RANK-RANKL interaction. In calcium signaling pathways, lithium inhibits Oscar which an important bone-specific regulator for osteoclast differentiation via NFATc1 amplification. The effects of lithium are indicated by red arrows. Arrow pointing upward (↑) indicates an increase and pointing downward (↓) indicates a decrease. Equivalent arrow (↔) indicates no effect.
Challenge for the Use of Lithium
The major medical challenge of lithium is the underutilization of clinical application mainly attributable to the presence of unfavourable side effects (Rybakowski, 2018), despite the reported favourable effects and the necessity of trace amount of lithium (1000 mg/day for a 70 kg adult) for mental and physical health (Schrauzer, 2002). Long-term lithium therapy potentially comes at the cost of renal, endocrine and dermatological side effects. In the kidney, 4 weeks of lithium therapy caused renal tubular concentration defects (characterized by a reduction in urinary concentrating ability) in healthy volunteers with greater concern of developing polyuria, polydipsia, and nephrogenic diabetes insipidus (Walker et al., 2005). Lithium treatment was also closely associated with hyperparathyroidism and hypercalcaemia. A case report revealed that a 28-year-old female patient who receiving Li2CO3 for bipolar mood disorder for at least 2 years developed hyperparathyroidism (Nair et al., 2013). In a cohort study, it was found that bipolar patients with lithium treatment had higher odds of hypercalcaemia as compared to those not receiving lithium treatment (Meehan et al., 2018). Otherwise, the skin is adversely affected by lithium. A case report reported that a 31-year-old Caucasian woman with bipolar disorder type I manifested a cutaneous adverse reaction after treated with Li2CO3 (Pinna et al., 2017). Some clinical cohort studies also indicated the association between lithium treatment and congenital malformation, raising the concern about the possible teratogenicity of lithium exposure during the first trimester (Poels et al., 2018).
Research Gap and Future Perspectives
The efficacy of lithium in promoting bone health has been abundantly demonstrated by researchers in controlled experimental settings and human populations. This collection of articles encourages the greater use of lithium despite as a fundamental treatment for bipolar disease. To our knowledge, other commonly used medications for bipolar disease such as anti-depressants, anti-convulsants, and neuroleptics are associated with low BMD (Crews and Howes, 2012; Rady et al., 2018; Zhou et al., 2018). Lithium may be a better treatment option for bipolar disease patients with low BMD taking into consideration the side effects of lithium. Hence, the clinical utility of lithium particularly the dose and frequency of supplementation should be under careful consideration to maximize its efficacy and prevent life-threatening complications.
Lithium targets the perturbation of canonical Wnt/β-catenin, PI3K/Akt, BMP-2, RANKL/RANKL/OPG, NF-κB, MAPK, and calcium signaling responsible for bone pathologies. It becomes clear that GSK3β may be a central nexus to cross-talk in many signaling pathways and transcriptional networks controlling bone cell development to fine-tune the bone remodelling processes. The current state of knowledge suggests that GSK3β may be a potential drug target for the management of osteoporosis, fractures and bone defects. However, GSK3β is a multifaceted protein kinase involved in the regulation of many cellular activities including energy metabolism and signaling by cytokines and hormones. The inhibition of GSK3 by lithium may suggest the multiple adverse effects of lithium. Apart from suggesting lithium as a bone-protective agent and understanding the molecular action of lithium on bone, this review provides new insights into repurposing other GSK3β inhibitors as a potential adjunct therapy for bone-related conditions.
Conclusion
It is tempting to speculate lithium as a promising pharmaceutical agent toward improving bone health. Apart from exerting its beneficial properties on bone via direct administration, the application of lithium in bone tissue engineering has been attempted by doping a trace amount on the scaffold to enhance osteogenesis and inhibit osteoclastogenesis subsequently improve the physical-mechanical characteristics of bone. In term of treating bone defect and fracture, lithium seems to be a good choice of microelement for improving the shortcomings of synthetic bone substitutes for their brittleness and insufficiency to promote osteogenesis. Lithium is also applicable for bipolar disease patients with the phenotype of low bone mass who are prone to osteoporosis-related fractures. We hope this review may provide a small contribution to bring novel possibilities for the additional therapeutic use of lithium, particularly against bone-related disorders, in patients with psychiatric problems. A comprehensive understanding of the mechanism of action for lithium in bone metabolism is likely to reveal the potential molecular targets for novel bone therapies.
Author Contributions
SW performed literature search and drafted the manuscript. K-YC and SI-N provided critical review for the manuscript. SI-N gave the final approval for the publication of this manuscript.
Funding
We thank Universiti Kebangsaan Malaysia and Ministry of Education, Malaysia for supporting this work via MI-2019-006 and FRGS/1/2018/SKK10/UKM/03/1 grants.
Conflict of Interest
The authors declare that the research was conducted in the absence of any commercial or financial relationships that could be construed as a potential conflict of interest.
References
Albers, J., Keller, J., Baranowsky, A., Beil, F. T., Catala-Lehnen, P., Schulze, J., et al. (2013). Canonical Wnt signaling inhibits osteoclastogenesis independent of osteoprotegerin. J. Cell Biol. 200 (4), 537–549. doi: 10.1083/jcb.201207142
Arioka, M., Takahashi-Yanaga, F., Sasaki, M., Yoshihara, T., Morimoto, S., Hirata, M., et al. (2014). Acceleration of bone regeneration by local application of lithium: Wnt signal-mediated osteoblastogenesis and Wnt signal-independent suppression of osteoclastogenesis. Biochem. Pharmacol. 90 (4), 397–405. doi: 10.1016/j.bcp.2014.06.011
Bai, J., Xu, Y., Dieo, Y., Sun, G. (2019). Combined low-dose LiCl and LY294002 for the treatment of osteoporosis in ovariectomized rats. J. Orthop. Surg. Res. 14 (1), 177. doi: 10.1186/s13018-019-1210-1
Baran, D. T., Schwartz, M. P., Bergfeld, M. A., Teitelbaum, S. L., Slatopolsky, E., Avioli, L. V. (1978). Lithium inhibition of bone mineralization and osteoid formation. J. Clin. Invest. 61 (6), 1691–1696. doi: 10.1172/JCI109090
Bellwinkel, S., Schafer, A., Minne, H., Ziegler, R. (1975). The effect of chronic lithium application on the mineral content of rat bone and soft tissues. Int. Pharmacopsychiatry 10 (1), 9–16. doi: 10.1159/000468163
Bernick, J., Wang, Y., Sigal, I. A., Alman, B. A., Whyne, C. M., Nam, D. (2014). Parameters for lithium treatment are critical in its enhancement of fracture-healing in rodents. J. Bone Joint Surg. Am. 96 (23), 1990–1998. doi: 10.2106/JBJS.N.00057
Bertacchini, J., Magarò, M. S., Potì, F., Palumbo, C. (2018). Osteocytes Specific GSK3 Inhibition Affects In Vitro Osteogenic Differentiation. Biomedicines 6 (2), 61. doi: 10.3390/biomedicines6020061
Bolton, J. M., Metge, C., Lix, L., Prior, H., Sareen, J., Leslie, W. D. (2008). Fracture risk from psychotropic medications: a population-based analysis. J. Clin. Psychopharmacol. 28 (4), 384–391. doi: 10.1097/JCP.0b013e31817d5943
Bolton, J. M., Morin, S. N., Majumdar, S. R., Sareen, J., Lix, L. M., Johansson, H., et al. (2017). Association of Mental Disorders and Related Medication Use With Risk for Major Osteoporotic Fractures. JAMA Psychiatry 74 (6), 641–648. doi: 10.1001/jamapsychiatry.2017.0449
Bonjour, J.-P., Chevalley, T., Ferrari, S., Rizzoli, R. (2009). The importance and relevance of peak bone mass in the prevalence of osteoporosis. Salud Publica Mex. 51 (Suppl 1), S5–S17. doi: 10.1590/S0036-36342009000700004
Boyce, B. F., Xing, L. (2007). The RANKL/RANK/OPG pathway. Curr. Osteoporos Rep. 5 (3), 98–104. doi: 10.1007/s11914-007-0024-y
Boyce, B. F., Yao, Z., Xing, L. (2009). Osteoclasts have multiple roles in bone in addition to bone resorption. Crit. Rev. Eukaryot Gene Expr. 19 (3), 171–180. doi: 10.1615/CritRevEukarGeneExpr.v19.i3.10
Boyce, B. F., Yao, Z., Xing, L. (2010). Functions of nuclear factor kappaB in bone. Ann. N. Y. Acad. Sci. 1192, 367–375. doi: 10.1111/j.1749-6632.2009.05315.x
Boyle, W. J., Simonet, W. S., Lacey, D. L. (2003). Osteoclast differentiation and activation. Nature 423 (6937), 337–342. doi: 10.1038/nature01658
Chapurlat, R. D., Confavreux, C. B. (2016). Novel biological markers of bone: from bone metabolism to bone physiology. Rheumatology 55 (10), 1714–1725. doi: 10.1093/rheumatology/kev410
Clement-Lacroix, P., Ai, M., Morvan, F., Roman-Roman, S., Vayssiere, B., Belleville, C., et al. (2005). Lrp5-independent activation of Wnt signaling by lithium chloride increases bone formation and bone mass in mice. Proc. Natl. Acad. Sci. U. S. A 102 (48), 17406–17411. doi: 10.1073/pnas.0505259102
Cohen, O., Rais, T., Lepkifker, E., Vered, I. (1998). Lithium carbonate therapy is not a risk factor for osteoporosis. Horm. Metab. Res. 30 (9), 594–597. doi: 10.1055/s-2007-978939
Crews, M. P. K., Howes, O. D. (2012). Is antipsychotic treatment linked to low bone mineral density and osteoporosis? A review of the evidence and the clinical implications. Hum. Psychopharmacol. 27 (1), 15–23. doi: 10.1002/hup.1265
Dai, F., Zhang, F., Sun, D., Zhang, Z. H., Dong, S. W., Xu, J. Z. (2015). CTLA4 enhances the osteogenic differentiation of allogeneic human mesenchymal stem cells in a model of immune activation. Braz. J. Med. Biol. Res. 48 (7), 629–636. doi: 10.1590/1414-431x20154209
Davis, J. M., Janicak, P. G., Hogan, D. M. (1999). Mood stabilizers in the prevention of recurrent affective disorders: a meta-analysis. Acta Psychiatr. Scand. 100 (6), 406–417. doi: 10.1111/j.1600-0447.1999.tb10890.x
Eldar-Finkelman, H., Martinez, A. (2011). GSK-3 Inhibitors: Preclinical and Clinical Focus on CNS. Front. Mol. Neurosci. 4, 32–32. doi: 10.3389/fnmol.2011.00032
Embi, N., Rylatt, D. B., Cohen, P. (1980). Glycogen synthase kinase-3 from rabbit skeletal muscle. Separation from cyclic-AMP-dependent protein kinase and phosphorylase kinase. Eur. J. Biochem. 107 (2), 519–527. doi: 10.1111/j.1432-1033.1980.tb06059.x
Eren, I., Yildiz, M., Civi, I. (2006). The effects of lithium treatment on bone mineral density in bipolar patients. Neurol. Psychiat Br. 13 (4), 174–179.
Forlenza, O. V., De-Paula, V. J. R., Diniz, B. S. O. (2014). Neuroprotective effects of lithium: implications for the treatment of Alzheimer’s disease and related neurodegenerative disorders. ACS Chem. Neurosci. 5 (6), 443–450. doi: 10.1021/cn5000309
Galli, C., Piemontese, M., Lumetti, S., Manfredi, E., Macaluso, G. M., Passeri, G. (2013). GSK3b-inhibitor lithium chloride enhances activation of Wnt canonical signaling and osteoblast differentiation on hydrophilic titanium surfaces. Clin. Implants Res. 24 (8), 921–927. doi: 10.1111/j.1600-0501.2012.02488.x
Gelenberg, A. J., Hopkins, H. S. (1993). Report on efficacy of treatments for bipolar disorder. Psychopharmacol. Bull. 29 (4), 447–456.
Guo, H., Wang, C., Wang, J., He, Y. (2018). Lithium-incorporated deproteinized bovine bone substitute improves osteogenesis in critical-sized bone defect repair. J. Biomater. Appl. 32 (10), 1421–1434. doi: 10.1177/0885328218768185
Harvey, B. M., Eschbach, M., Glynn, E. A., Kotha, S., Darre, M., Adams, D. J., et al. (2015). Effect of daily lithium chloride administration on bone mass and strength in growing broiler chickens. Poult. Sci. 94 (2), 296–301. doi: 10.3382/ps/peu079
Henneman, D., Zimmerberg, J. J. (1974). Lack of effect of chronic lithium chloride on bone composition and metabolism. Endocrinology 94 (3), 915–917. doi: 10.1210/endo-94-3-915
Houschyar, K. S., Tapking, C., Borrelli, M. R., Popp, D., Duscher, D., Maan, Z. N., et al. (2019). Wnt Pathway in Bone Repair and Regeneration - What Do We Know So Far. Front. Cell Dev. Biol. 6, 170–170. doi: 10.3389/fcell.2018.00170
Hu, X., Wang, Z., Shi, J., Guo, X., Wang, L., Ping, Z., et al. (2017). Lithium chloride inhibits titanium particle-induced osteoclastogenesis by inhibiting the NF-kappaB pathway. Oncotarget 8 (48), 83949–83961. doi: 10.18632/oncotarget.20000
Huang, T. B., Li, Y. Z., Yu, K., Yu, Z., Wang, Y., Jiang, Z. W., et al. (2019). Effect of the Wnt signal-RANKL/OPG axis on the enhanced osteogenic integration of a lithium incorporated surface. Biomater. Sci. 7 (3), 1101–1116. doi: 10.1039/C8BM01411F
Jang, H. D., Shin, J. H., Park, D. R., Hong, J. H., Yoon, K., Ko, R., et al. (2011). Inactivation of glycogen synthase kinase-3beta is required for osteoclast differentiation. J. Biol. Chem. 286 (45), 39043–39050. doi: 10.1074/jbc.M111.256768
Jin, Y., Xu, L., Hu, X., Liao, S., Pathak, J. L., Liu, J. (2017). Lithium chloride enhances bone regeneration and implant osseointegration in osteoporotic conditions. J. Bone Miner. Metab. 35 (5), 497–503. doi: 10.1007/s00774-016-0783-6
Kalu, D. N. (1991). The ovariectomized rat model of postmenopausal bone loss. Bone Miner. 15 (3), 175–191. doi: 10.1016/0169-6009(91)90124-I
Kim, J. H., Liu, X., Wang, J., Chen, X., Zhang, H., Kim, S. H., et al. (2013). Wnt signaling in bone formation and its therapeutic potential for bone diseases. Ther. Adv. Musculoskelet. Dis. 5 (1), 13–31. doi: 10.1177/1759720X12466608
Kurgan, N., Bott, K. N., Helmeczi, W. E., Roy, B. D., Brindle, I. D., Klentrou, P., et al. (2019). Low dose lithium supplementation activates Wnt/beta-catenin signalling and increases bone OPG/RANKL ratio in mice. Biochem. Biophys. Res. Commun. 511 (2), 394–397. doi: 10.1016/j.bbrc.2019.02.066
Lee, Z. H., Kim, H. H. (2003). Signal transduction by receptor activator of nuclear factor kappa B in osteoclasts. Biochem. Biophys. Res. Commun. 305 (2), 211–214. doi: 10.1016/S0006-291X(03)00695-8
Lewicki, M., Paez, H., Mandalunis, P. M. (2006). Effect of lithium carbonate on subchondral bone in sexually mature Wistar rats. Exp. Toxicol. Pathol. 58 (2-3), 197–201. doi: 10.1016/j.etp.2006.06.003
Lewitzka, U., Severus, E., Bauer, R., Ritter, P., Müller-Oerlinghausen, B., Bauer, M. (2015). The suicide prevention effect of lithium: more than 20 years of evidence-a narrative review. Int. J. Bipolar Disord. 3 (1), 32–32. doi: 10.1186/s40345-015-0032-2
Li, J., Khavandgar, Z., Lin, S. H., Murshed, M. (2011). Lithium chloride attenuates BMP-2 signaling and inhibits osteogenic differentiation through a novel WNT/GSK3- independent mechanism. Bone 48 (2), 321–331. doi: 10.1016/j.bone.2010.09.033
Li, H., Huang, K., Liu, X., Liu, J., Lu, X., Tao, K., et al. (2014). Lithium chloride suppresses colorectal cancer cell survival and proliferation through ROS/GSK-3β/NF-κB signaling pathway. Oxid. Med. Cell Longev 2014, 241864–241864. doi: 10.1155/2014/241864
Li, L., Peng, X., Qin, Y., Wang, R., Tang, J., Cui, X., et al. (2017a). Acceleration of bone regeneration by activating Wnt/β-catenin signalling pathway via lithium released from lithium chloride/calcium phosphate cement in osteoporosis. Sci. Rep. 7, 45204. doi: 10.1038/srep45204
Li, L., Wang, R., Li, B., Liang, W., Pan, H., Cui, X., et al. (2017b). Lithium doped calcium phosphate cement maintains physical mechanical properties and promotes osteoblast proliferation and differentiation. J. BioMed. Mater. Res. B. Appl. Biomater. 105 (5), 944–952. doi: 10.1002/jbm.b.33625
Li, D., Xie, X., Yang, Z., Wang, C., Wei, Z., Kang, P. (2018). Enhanced bone defect repairing effects in glucocorticoid-induced osteonecrosis of the femoral head using a porous nano-lithium-hydroxyapatite/gelatin microsphere/erythropoietin composite scaffold. Biomater. Sci. 6 (3), 519–537. doi: 10.1039/C7BM00975E
Ling, Z., Wu, L., Shi, G., Chen, L., Dong, Q. (2017). Increased Runx2 expression associated with enhanced Wnt signaling in PDLLA internal fixation for fracture treatment. Exp. Ther. Med. 13 (5), 2085–2093. doi: 10.3892/etm.2017.4216
Liu, T., Zhang, L., Joo, D., Sun, S.-C. (2017). NF-κB signaling in inflammation. Signal Transduct. Target Ther. 2, 17023. doi: 10.1038/sigtrans.2017.23
Liu, W., Chen, D., Jiang, G., Li, Q., Wang, Q., Cheng, M., et al. (2018). A lithium-containing nanoporous coating on entangled titanium scaffold can enhance osseointegration through Wnt/beta-catenin pathway. Nanomedicine 14 (1), 153–164. doi: 10.1016/j.nano.2017.09.006
Liu, B., Wu, Q., Zhang, S., Del Rosario, A. (2019). Lithium use and risk of fracture: a systematic review and meta-analysis of observational studies. Osteoporos Int. 30 (2), 257–266. doi: 10.1007/s00198-018-4745-9
Loiselle, A. E., Lloyd, S. A., Paul, E. M., Lewis, G. S., Donahue, H. J. (2013). Inhibition of GSK-3beta rescues the impairments in bone formation and mechanical properties associated with fracture healing in osteoblast selective connexin 43 deficient mice. PloS One 8 (11), e81399. doi: 10.1371/journal.pone.0081399
Luo, J., Yang, Z., Ma, Y., Yue, Z., Lin, H., Qu, G., et al. (2016). LGR4 is a receptor for RANKL and negatively regulates osteoclast differentiation and bone resorption. Nat. Med. 22 (5), 539–546. doi: 10.1038/nm.4076
Ma, Y., Li, Y., Hao, J., Ma, B., Di, T., Dong, H. (2019). Evaluation of the degradation, biocompatibility and osteogenesis behavior of lithium-doped calcium polyphosphate for bone tissue engineering. BioMed. Mater. Eng. 30 (1), 23–36. doi: 10.3233/BME-181030
Mak, T. W., Shek, C. C., Chow, C. C., Wing, Y. K., Lee, S. (1998). Effects of lithium therapy on bone mineral metabolism: a two-year prospective longitudinal study. J. Clin. Endocrinol. Metab. 83 (11), 3857–3859. doi: 10.1210/jc.83.11.3857
Mason, J. J., Williams, B. O. (2010). SOST and DKK: Antagonists of LRP Family Signaling as Targets for Treating Bone Disease. J. Osteoporos 2010, 9. doi: 10.4061/2010/460120
McGonnell, I. M., Grigoriadis, A. E., Lam, E. W. F., Price, J. S., Sunters, A. (2012). A specific role for phosphoinositide 3-kinase and AKT in osteoblasts? Front. Endocrinol. 3, 88–88. doi: 10.3389/fendo.2012.00088
Meehan, A. D., Udumyan, R., Kardell, M., Landén, M., Järhult, J., Wallin, G. (2018). Lithium-associated hypercalcemia: pathophysiology, prevalence, management. World J. Surg. 42 (2), 415–424. doi: 10.1007/s00268-017-4328-5
Moon, J. B., Kim, J. H., Kim, K., Youn, B. U., Ko, A., Lee, S. Y., et al. (2012). Akt induces osteoclast differentiation through regulating the GSK3β/NFATc1 signaling cascade. J. Immunol. 188 (1), 163–169. doi: 10.4049/jimmunol.1101254
Muneer, A. (2017). Wnt and GSK3 Signaling Pathways in Bipolar Disorder: Clinical and Therapeutic Implications. Clin. Psychopharmacol. Neurosci. 15 (2), 100–114. doi: 10.9758/cpn.2017.15.2.100
Nair, C. G., Menon, R., Jacob, P., Babu, M. (2013). Lithium-induced parathyroid dysfunction: A new case. Indian J. Endocrinol. Metab. 17 (5), 930–932. doi: 10.4103/2230-8210.117223
Neelakandan, R. S., Bhargava, D. (2012). Transport distraction osteogenesis for maxillomandibular reconstruction: current concepts and applications. J. Maxillofac. Surg. 11 (3), 291–299. doi: 10.1007/s12663-011-0329-3
Nordenstrom, J., Elvius, M., Bagedahl-Strindlund, M., Zhao, B., Torring, O. (1994). Biochemical hyperparathyroidism and bone mineral status in patients treated long-term with lithium. Metabolism 43 (12), 1563–1567. doi: 10.1016/0026-0495(94)90017-5
Park, J. H., Lee, N. K., Lee, S. Y. (2017). Current Understanding of RANK Signaling in Osteoclast Differentiation and Maturation. Mol. Cells 40 (10), 706–713. doi: 10.14348/molcells.2017.0225
Pepersack, T., Corvilain, J., Bergmann, P. (1994). Effects of lithium on bone resorption in cultured foetal rat long-bones. Eur. J. Clin. Invest. 24 (6), 400–405. doi: 10.1111/j.1365-2362.1994.tb02183.x
Pinna, M., Manchia, M., Puddu, S., Minnai, G., Tondo, L., Salis, P. (2017). Cutaneous adverse reaction during lithium treatment: a case report and updated systematic review with meta-analysis. Int. J. Bipolar Disord. 5 (1), 20–20. doi: 10.1186/s40345-017-0091-7
Plenge, P., Rafaelsen, O. J. (1982). Lithium effects on calcium, magnesium and phosphate in man: effects on balance, bone mineral content, faecal and urinary excretion. Acta Psychiatr. Scand. 66 (5), 361–373. doi: 10.1111/j.1600-0447.1982.tb06718.x
Poels, E. M. P., Bijma, H. H., Galbally, M., Bergink, V. (2018). Lithium during pregnancy and after delivery: a review. Int. J. Bipolar Disord. 6 (1), 26–26. doi: 10.1186/s40345-018-0135-7
Rady, A., Elsheshai, A., Elkholy, O., Abouelwafa, H., Eltawil, M. (2018). Long Term Use of Antipsychotics and Adverse Effects on Bone Density. doi: 10.4172/Neuropsychiatry.1000491
Rybakowski, J. K. (2018). Challenging the Negative Perception of Lithium and Optimizing Its Long-Term Administration. Front. Mol. Neurosci. 11, 349–349. doi: 10.3389/fnmol.2018.00349
Schrauzer, G. N. (2002). Lithium: occurrence, dietary intakes, nutritional essentiality. J. Am. Coll. Nutr. 21 (1), 14–21. doi: 10.1080/07315724.2002.10719188
Setiawati, R., Rahardjo, P. (2018). Bone Development and Growth Osteogenesis and Bone Regeneration: IntechOpen. doi: 10.5772/intechopen.82452
Shi, X., Wang, J., Lei, Y., Cong, C., Tan, D., Zhou, X. (2019). Research progress on the PI3K/AKT signaling pathway in gynecological cancer (Review). Mol. Med. Rep. 19 (6), 4529–4535. doi: 10.3892/mmr.2019.10121
Soares-Silva, M., Diniz, F. F., Gomes, G. N., Bahia, D. (2016). The Mitogen-Activated Protein Kinase (MAPK) Pathway: Role in Immune Evasion by Trypanosomatids. Front. Microbiol. 7, 183–183. doi: 10.3389/fmicb.2016.00183
Stewart, S., Bryant, S. J., Ahn, J., Hankenson, K. D. (2015). “Chapter 24 - Bone Regeneration,” in Translational Regenerative Medicine. Eds. Atala, A., Allickson, J. G. (Boston: Academic Press), 313–333.
Su, J. A., Cheng, B. H., Huang, Y. C., Lee, C. P., Yang, Y. H., Lu, M. L., et al. (2017). Bipolar disorder and the risk of fracture: A nationwide population-based cohort study. J. Affect. Disord. 218, 246–252. doi: 10.1016/j.jad.2017.04.037
Tevlin, R., McArdle, A., Chan, C. K. F., Pluvinage, J., Walmsley, G. G., Wearda, T., et al. (2014). Osteoclast derivation from mouse bone marrow. J. Vis. Exp. (93), e52056–e52056. doi: 10.3791/52056
Tondo, L., Alda, M., Bauer, M., Bergink, V., Grof, P., Hajek, T., et al. (2019). Clinical use of lithium salts: guide for users and prescribers. Int. J. Bipolar Disord. 7 (1), 16–16. doi: 10.1186/s40345-019-0151-2
Vachhani, K., Pagotto, A., Wang, Y., Whyne, C., Nam, D. (2018a). Design of experiments confirms optimization of lithium administration parameters for enhanced fracture healing. J. Biomech. 66, 153–158. doi: 10.1016/j.jbiomech.2017.09.043
Vachhani, K., Whyne, C., Wang, Y., Burns, D. M., Nam, D. (2018b). Low-dose lithium regimen enhances endochondral fracture healing in osteoporotic rodent bone. J. Orthop. Res. 36 (6), 1783–1789. doi: 10.1002/jor.23799
Vestergaard, P., Rejnmark, L., Mosekilde, L. (2005). Reduced relative risk of fractures among users of lithium. Calcif. Tissue Int. 77 (1), 1–8. doi: 10.1007/s00223-004-0258-y
Walker, R. J., Weggery, S., Bedford, J. J., McDonald, F. J., Ellis, G., Leader, J. P. (2005). Lithium-induced reduction in urinary concentrating ability and urinary aquaporin 2 (AQP2) excretion in healthy volunteers. Kidney Int. 67 (1), 291–294. doi: 10.1111/j.1523-1755.2005.00081.x
Wang, H., Brown, J., Martin, M. (2011). Glycogen synthase kinase 3: a point of convergence for the host inflammatory response. Cytokine 53 (2), 130–140. doi: 10.1016/j.cyto.2010.10.009
Wang, X., Zhu, S., Jiang, X., Li, Y., Song, D., Hu, J. (2015). Systemic administration of lithium improves distracted bone regeneration in rats. Calcif. Tissue Int. 96 (6), 534–540. doi: 10.1007/s00223-015-0004-7
Wei, W., Zeve, D., Suh, J. M., Wang, X., Du, Y., Zerwekh, J. E., et al. (2011). Biphasic and dosage-dependent regulation of osteoclastogenesis by β-catenin. Mol. Cell Biol. 31 (23), 4706–4719. doi: 10.1128/MCB.05980-11
Wilting, I., de Vries, F., Thio, B. M., Cooper, C., Heerdink, E. R., Leufkens, H. G., et al. (2007). Lithium use and the risk of fractures. Bone 40 (5), 1252–1258. doi: 10.1016/j.bone.2006.12.055
Wong, S. K., Mohamad, N. V., Ibrahim, N., Chin, K. Y., Shuid, A. N., Ima-Nirwana, S. (2019). The Molecular Mechanism of Vitamin E as a Bone-Protecting Agent: A Review on Current Evidence. Int. J. Mol. Sci. 20 (6). doi: 10.3390/ijms20061453
Wu, M., Chen, G., Li, Y.-P. (2016). TGF-β and BMP signaling in osteoblast, skeletal development, and bone formation, homeostasis and disease. Bone Res. 4, 16009–16009. doi: 10.1038/boneres.2016.9
Yang, Y., Kim, S. C., Yu, T., Yi, Y.-S., Rhee, M. H., Sung, G.-H., et al. (2014). Functional roles of p38 mitogen-activated protein kinase in macrophage-mediated inflammatory responses. Mediators Inflammation 2014, 352371–352371. doi: 10.1155/2014/352371
Yang, C., Wang, W., Zhu, K., Liu, W., Luo, Y., Yuan, X., et al. (2019). Lithium chloride with immunomodulatory function for regulating titanium nanoparticle-stimulated inflammatory response and accelerating osteogenesis through suppression of MAPK signaling pathway. Int. J. Nanomed. 14, 7475–7488. doi: 10.2147/IJN.S210834
Yavropoulou, M. P., Yovos, J. G. (2008). Osteoclastogenesis–current knowledge and future perspectives. J. Musculoskelet. Neuronal Interact. 8 (3), 204–216.
Zamani, A., Omrani, G. R., Nasab, M. M. (2009). Lithium’s effect on bone mineral density. Bone 44 (2), 331–334. doi: 10.1016/j.bone.2008.10.001
Zhai, X., Wang, S., Zhu, M., He, W., Pan, Z., Su, S. (2019). Antiviral Effect of Lithium Chloride and Diammonium Glycyrrhizinate on Porcine Deltacoronavirus In Vitro. Pathogens 8 (3), 144. doi: 10.3390/pathogens8030144
Zhang, J., Cai, L., Tang, L., Zhang, X., Yang, L., Zheng, K., et al. (2018a). Highly dispersed lithium doped mesoporous silica nanospheres regulating adhesion, proliferation, morphology, ALP activity and osteogenesis related gene expressions of BMSCs. Colloids Surf. B. Biointerfaces 170, 563–571. doi: 10.1016/j.colsurfb.2018.06.038
Zhang, J., Cai, L., Wang, T., Tang, S., Li, Q., Tang, T., et al. (2018b). Lithium doped silica nanospheres/poly(dopamine) composite coating on polyetheretherketone to stimulate cell responses, improve bone formation and osseointegration. Nanomedicine 14 (3), 965–976. doi: 10.1016/j.nano.2018.01.017
Zhou, C., Fang, L., Chen, Y., Zhong, J., Wang, H., Xie, P. (2018). Effect of selective serotonin reuptake inhibitors on bone mineral density: a systematic review and meta-analysis. Osteoporos. Int. : A J. established as result cooperation between Eur. Foundation Osteoporos. Natl. Osteoporos. Foundation U.S.A. 29 (6), 1243–1251. doi: 10.1007/s00198-018-4413-0
Keywords: bone, glycogen synthase kinase-3β, lithium, osteoblast, osteoclast, osteoporosis
Citation: Wong SK, Chin K-Y and Ima-Nirwana S (2020) The Skeletal-Protecting Action and Mechanisms of Action for Mood-Stabilizing Drug Lithium Chloride: Current Evidence and Future Potential Research Areas. Front. Pharmacol. 11:430. doi: 10.3389/fphar.2020.00430
Received: 10 February 2020; Accepted: 20 March 2020;
Published: 07 April 2020.
Edited by:
Maria Angela Sortino, University of Catania, ItalyReviewed by:
Marlena Cathorina Kruger, Massey University, New ZealandJiacan Su, Second Military Medical University, China
Copyright © 2020 Wong, Chin and Ima-Nirwana. This is an open-access article distributed under the terms of the Creative Commons Attribution License (CC BY). The use, distribution or reproduction in other forums is permitted, provided the original author(s) and the copyright owner(s) are credited and that the original publication in this journal is cited, in accordance with accepted academic practice. No use, distribution or reproduction is permitted which does not comply with these terms.
*Correspondence: Soelaiman Ima-Nirwana, imasoel@ppukm.ukm.edu.my