- 1Department of Developmental Biology, Washington University School of Medicine, St. Louis, MO, United States
- 2Department of Biological Sciences, Missouri University of Science and Technology, Rolla, MO, United States
- 3Department of Medicine, Washington University School of Medicine, St. Louis, MO, United States
The free-living, non-parasitic nematode Caenorhabditis elegans is a premier model organism for the study of aging and longevity due to its short lifespan, powerful genetic tools, and conservation of fundamental mechanisms with mammals. Approximately 70 percent of human genes have homologs in C. elegans, including many that encode proteins in pathways that influence aging. Numerous genetic pathways have been identified in C. elegans that affect lifespan, including the dietary restriction pathway, the insulin/insulin-like growth factor (IGF) signaling pathway, and the disruption of components of the mitochondrial electron transport chain. C. elegans is also a powerful system for performing drug screens, and many lifespan-extending compounds have been reported; notably, several FDA-approved medications extend the lifespan in C. elegans, raising the possibility that they can also extend the lifespan in humans. The renin–angiotensin system (RAS) in mammals is an endocrine system that regulates blood pressure and a paracrine system that acts in a wide range of tissues to control physiological processes; it is a popular target for drugs that reduce blood pressure, including angiotensin-converting enzyme (ACE) inhibitors and angiotensin II receptor blockers (ARBs). Emerging evidence indicates that this system influences aging. In C. elegans, decreasing the activity of the ACE homolog acn-1 or treatment with the ACE-inhibitor Captopril significantly extends the lifespan. In Drosophila, treatment with ACE inhibitors extends the lifespan. In rodents, manipulating the RAS with genetic or pharmacological interventions can extend the lifespan. In humans, polymorphisms in the ACE gene are associated with extreme longevity. These results suggest the RAS plays a conserved role in controlling longevity. Here, we review studies of the RAS and aging, emphasizing the potential of C. elegans as a model for understanding the mechanism of lifespan control.
Introduction
Caenorhabditis elegans is a powerful model system for aging pharmacology
Age-related degenerative changes are a major issue for human health (Vijg and Campisi, 2008). A wide variety of systems are affected including the reproductive, central and peripheral nervous, musculoskeletal, immune, cardiac, renal, and respiratory. The age-related reduction of muscular strength, or sarcopenia, is a serious problem for many elderly people. Although the quest for methods to delay aging is a longstanding human endeavor, no pharmacological agents have yet been demonstrated to delay human aging. One of the biggest challenges to understanding and treating human aging is the fact that humans live so long and age so slowly. Thus, a critical need in aging research is model organisms that are short-lived, amenable to experimentation, and relevant to humans.
The analysis of genetically tractable model organisms with short lifespans, such as yeast, worms, and flies, has resulted in the identification of genes that can modulate longevity (Guarente and Kenyon, 2000). Studies of mice have provided substantial evidence that at least some mechanisms that affect the rate of age-related degeneration have been conserved during animal evolution (reviewed in Blagosklonny (2008), Vijg and Campisi (2008)). Thus, short-lived animals may provide meaningful guides to the biology of human aging and serve as the proving grounds where interventions that delay age-related degeneration can be identified and characterized. The report by the intervention testing program (ITP) of the National Institutes of Aging that Rapamycin extends the lifespan of mice is a compelling example, since Rapamycin was first demonstrated to influence the lifespan in yeast (Kaeberlein et al., 2005; Powers et al., 2006; Harrison et al., 2009).
C. elegans is a powerful and relevant experimental system that is excellent for the identification of genes and drugs that modulate the rate of aging. C. elegans is a free-living, hermaphroditic nematode that displays an extensive conservation of fundamental biological processes with other animals. Pioneering studies by Sydney Brenner and others established C. elegans as a powerful experimental model system for forward genetics, reverse genetics, and molecular analysis supported by a fully sequenced genome (C. elegans Sequencing Consortium, 1998; Brenner, 1974). C. elegans has been used to characterize fundamental and highly conserved biological processes such as RNA interference (RNAi) and apoptosis (Sulston and Horvitz, 1977; Fire et al., 1998). It has been used to develop innovative experimental techniques such as the in vivo expression of green fluorescent protein (GFP) (Chalfie et al., 1994); these and other discoveries have had a major impact on understanding mammalian biology. C. elegans is extremely well understood at the cellular anatomical level, since the entire cell lineage of the 959 somatic nuclei has been determined (Sulston and Horvitz, 1977).
C. elegans is excellent for studies of aging because the adults display the progressive, degenerative changes that are typical of aging in larger animals, but the adult lifespan is only about 15 days (Johnson, 1987). Age-related degenerative changes in C. elegans have been characterized extensively, and the genetic analysis of C. elegans has resulted in the discovery of genes and pathways that modulate longevity (Guarente and Kenyon, 2000; Kenyon, 2001). Many well-characterized mutations that influence aging by affecting known pathways are reagents that can be used to investigate the mechanism of action of newly discovered genes and drugs that influence aging. C. elegans has been used successfully to analyze the molecular targets of drugs (Carroll et al., 2003; Jones et al., 2005). Since nearly 75% of all C. elegans genes have human counterparts, there is a good chance that the molecular target of a drug in the worm will be similar to that in humans (C. elegans Sequencing Consortium, 1998).
The first of what is now a large class of ACE inhibitors, Captopril is an oligopeptide derivative developed in 1975 based on a peptide found in the venom of a snake, Bothrops jararaca, the Brazilian pit viper (Ondetti et al., 1977). ACE inhibitors modulate the renin–angiotensin system (RAS), a mechanism by which the body adapts to hypotension (Basso and Terragno, 2001; Igic and Igic, 2009). In this review, we focus on the emerging evidence from C. elegans, Drosophila, and mammals that the RAS controls longevity and drugs that target this system might be useful agents in the quest to extend human lifespan.
The RAS is an endocrine system that controls blood pressure and a paracrine system that mediates a wide range of physiological processes
High blood pressure, or hypertension, is defined as a systolic blood pressure over 140 mmHg and/or diastolic blood pressure over 90 mmHg (Mills et al., 2020). It is a common malady throughout the world, affecting more than 31% of the adult population (∼1.4 billion people); the percentage of the population suffering from hypertension continues to increase, creating an accelerating health crisis (Mills et al., 2020). High blood pressure is associated with and a likely cause of many cardiovascular and renal diseases (Mills et al., 2020) and is estimated to result in more than 10 million deaths per year worldwide (Forouzanfar et al., 2017). What causes hypertension? Dietary factors such as sodium are one cause; genetics can be a second cause (Singh et al., 2012). Age has been identified as a major risk factor for hypertension, with incidences rising in aging populations (Singh et al., 2012). Understanding the interaction between age and hypertension is important for maintaining the health of an aging population.
The RAS was first identified as a crucial regulator of blood pressure in the mid-20th century ((Skeggs et al., 1956), reviewed in Basso and Terragno (2001), Igic and Igic (2009)). The classic understanding was that the RAS is an endocrine system. In response to low blood pressure, the kidney secretes renin, an aspartyl protease. Renin cleaves the peptide angiotensinogen (AGT), which the liver releases into the blood stream, into angiotensin I (Ang I), a 10 amino acid peptide. Ang I is cleaved into angiotensin II (Ang II), an eight-amino acid peptide, by the angiotensin-converting enzyme (ACE), a metalloprotease secreted by the lungs. Ang II binds to the angiotensin II type 1 receptor (AGT1R) on vascular endothelial cells. AGT1R is a G-protein-coupled receptor that initiates a signal transduction pathway resulting in vasoconstriction and increased blood pressure (Figure 1A) (reviewed in Peach (1977), Reid et al. (1978), Fyhrquist and Saijonmaa (2008), and Santos et al. (2019)). In the last 30 years, exciting new discoveries have shown that the RAS is significantly more complicated in two dimensions: the endocrine system has many additional components, including some that promote vasodilation, and the RAS functions as a paracrine/autocrine system in many organs to mediate a wide range of physiological responses.
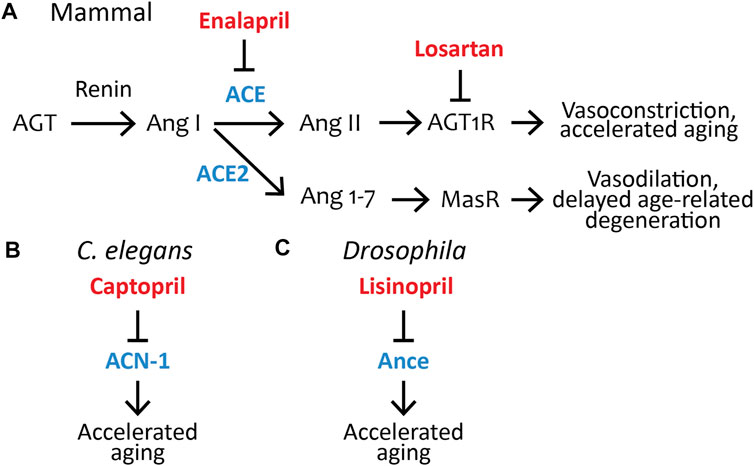
FIGURE 1. Pharmacological inhibition of the renin–angiotensin system influences aging in C. elegans, Drosophila, and mammals. (A) The RAS pathway in mammals; the ACE inhibitor Enalapril and angiotensin II type-1 receptor blocker Losartan are FDA-approved drugs that control aging in non-human mammals (see Table 1). AGT, Angiotensinogen; Ang I, Angiotensin I; ACE, Angiotensin-converting enzyme; Ang II, Angiotensin II; AGT1R, Angiotensin II type-1 receptor; ACE2, Angiotensin-converting enzyme 2; Ang 1-7, Angiotensin (1-7); MasR, Mas Receptor. (B) The FDA-approved ACE-inhibitor drug Captopril inhibits acn-1, the only C. elegans ACE homolog, to control aging (Kumar et al., 2016). ACN-1, Angiotensin-converting enzyme-like non-peptidase. (C) The FDA-approved ACE inhibitor drug Lisinopril inhibits Ance, the Drosophila ACE homolog, to control aging (Gabrawy et al., 2019). Ance, ANgiotensin-converting enzyme. Blue, ACE and its homologs ACE2, Ance, and ACN-1; red, ACE inhibitors (Captopril, Enalapril, Lisinopril) or ARBs (Losartan), which have been shown to influence aging.
In 2000, a second isoform of ACE was described in mammals, called ACE2 (Lavoie and Sigmund, 2003). ACE2 is a transmembrane protein that cleaves Ang I or Ang II into the heptapeptide angiotensin (1-7) (Ang(1-7)). Furthermore, the receptor for Ang(1-7) was discovered, called the Mas receptor (MasR). Activation of the Mas receptor results in vasodilation (Figure 1A) (Santos et al., 2003). The recent discovery that ACE2 appears to be the main entry point into cells for the SARS-CoV-2 coronavirus, the cause of the COVID-19 pandemic, has resulted in intense scrutiny (Wrapp et al., 2019). Additionally, ACE2 has been proposed to influence aging since inhibition accelerates age-related degenerative changes (Takeshita et al., 2018; Nozato et al., 2019; Takeshita et al., 2020). Other discoveries include the (pro)renin receptor [(P)RR], which binds and activates renin in tissues, and the angiotensin II type 2 receptor (AGT2R) on vascular endothelial cells. AGT2R is a G-protein-coupled receptor that initiates a signal transduction pathway resulting in vasodilation and reduced blood pressure. Thus, Ang II can increase blood pressure by acting through the AGT1R receptor and reduce blood pressure by acting through the AGT2R receptor, and the expression levels of the two receptors play an important role in the overall response (Figure 1A).
The receptors for angiotensin peptides are expressed in a wide range of tissues, indicating that they function in paracrine/autocrine systems controlled by the local generation of peptide agonists (reviewed in Lavoie and Sigmund, 2003; Bader, 2010; Nehme et al., 2019). The brain expresses the AGT1R and AGT2R receptors, which influence the autonomic nervous system, the hypothalamus–pituitary axis, vasopressin release, baroreflex sensitivity, and thirst and salt appetite. The overall effects of brain activation are to increase blood volume and blood pressure, but are also implicated in higher brain functions such as anxiety and stress (Abiodun and Ola, 2020; Balthazar et al., 2021). In the kidney, local RAS signaling plays a role in kidney development and renal function in adults. In the heart, Ang II acts through the AGT1R to induce cardiac hypertrophy and fibrosis and through the AGT2R to cause the opposite effects. In the gastrointestinal system, the RAS regulates intestinal physiological functions including electrolyte homeostasis, digestion, peptide transport, glucose, sodium and water absorption, and gastrointestinal motility (Jaworska et al., 2021). The RAS is important during pregnancy and acts locally in the uteroplacental unit to mediate angiogenesis, trophoblastic invasion, and adequate placentation (Leal et al., 2022). It is possible that the tissue-specific functions of the RAS represent primordial functions and are the target of interventions that modulate aging.
A major breakthrough in the treatment of hypertension was the development of Captopril, the first ACE inhibitor compound, in 1975 (Figure 2A) (Cushman and Ondetti, 1991). Observations of the hypotensive effects of venom of the snake Bothrops jararaca made it clear that ACE was a critical regulator of blood pressure and a useful target for inhibition (Cushman and Ondetti, 1991). Rational drug design techniques allowed the creation of Captopril as a peptide-analogue targeting and inhibiting the active site of ACE, with later generations of ACE inhibitors, such as Enalapril (Figure 2B) or Lisinopril (Figure 2C), improving the activity and bioavailability. Further research into the RAS led to the development of angiotensin II receptor blockers (ARBs) such as Losartan (Figure 2D) (Duncia et al., 1990). More recently, renin inhibitors such as Aliskiren were developed (Jensen et al., 2008).
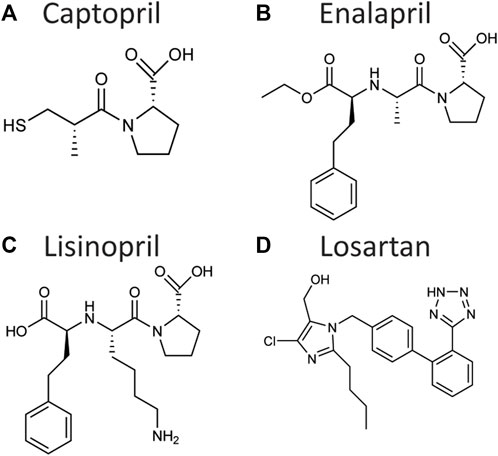
FIGURE 2. Chemical structure of the angiotensin-converting enzyme inhibitors Captopril (A), Enalapril (B), and Lisinopril (C), and of the angiotensin II receptor blocker Losartan (D). These drugs have been shown to control aging in C. elegans, Drosophila, and/or rodents.
The blood pressure regulation activity of the RAS is well characterized and has been studied in many mammalian model organisms (reviewed in (Peach, 1977; Reid et al., 1978; Igic and Igic, 2009; Santos et al., 2019). However, components of the RAS have also been discovered in non-mammalian organisms that lack closed circulatory systems. ACE homologs have been identified in organisms throughout the Bilaterian clade, including the C. elegans homolog acn-1 and the Drosophila homolog Ance (Figures 1B,C). Functional ACE homologs have also been identified in insects, crustaceans, annelids, and mollusks (reviewed in (Fournier et al., 2021)). Based on its presence in such diverse phyla, it is likely that ACE first evolved in a common ancestor roughly 600 million years ago (Dos Reis et al., 2015), prior to the evolution of the animal closed circulatory system (Simões-Costa et al., 2005). These observations raise an intriguing question: What is the ancestral function of ACE? Insight into that function can help address the role of the RAS during aging.
Emerging evidence has identified important connections between the RAS and aging. Pharmacological or genetic inhibition of members of the RAS can extend lifespan and delay age-related degenerative changes in mice and rats (Figures 3C, 4C) (reviewed in (Basso et al., 2005; de Cavanagh et al., 2011; Capettini et al., 2012; Kamo et al., 2016; Mogi, 2020; Jaworska et al., 2021; Le et al., 2021)). The study of ACE and the RAS in the context of aging is complicated in mammalian systems due to their pleiotropic effects not only on aging but also on vasoconstriction, renal, cardiovascular, and pulmonary health and function, and less understood functions in the brain (reviewed in (Le et al., 2021)). In contrast, non-mammalian organisms lacking closed circulatory systems can be used to distinguish the aging effects of the RAS from the cardiovascular functions.
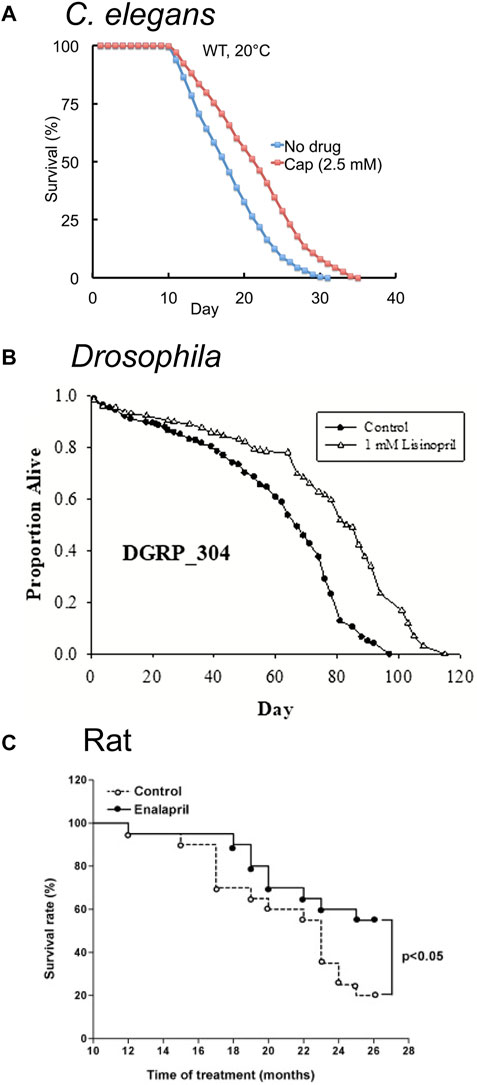
FIGURE 3. ACE inhibitors extend lifespan in model organisms. (A) The FDA-approved ACE inhibitor drug Captopril (cap) extends adult lifespan in C. elegans by about 21%. Survival curves of wild-type (WT) hermaphrodites treated with 2.5 mM Captopril from the L4 stage onward at 20°C. Adapted from (Kumar et al., 2016). (B) The FDA-approved ACE inhibitor drug Lisinopril extends lifespan in Drosophila melanogaster. Survival curve of populations of the Drosophila Genetic Reference Panel (DGRP, strain DGRP_304 is depicted) exposed to 1 mM Lisinopril. Adapted from (Gabrawy et al., 2019). (C) The FDA-approved ACE-inhibitor drug Enalapril extends lifespan in adult rats. Survival curves of adult Wistar rats exposed to 10 mg/kg Enalapril in water for 26 months. Enalapril treatment reduced mortality by 45% in the 26 month period. Adapted from (Santos et al., 2009).
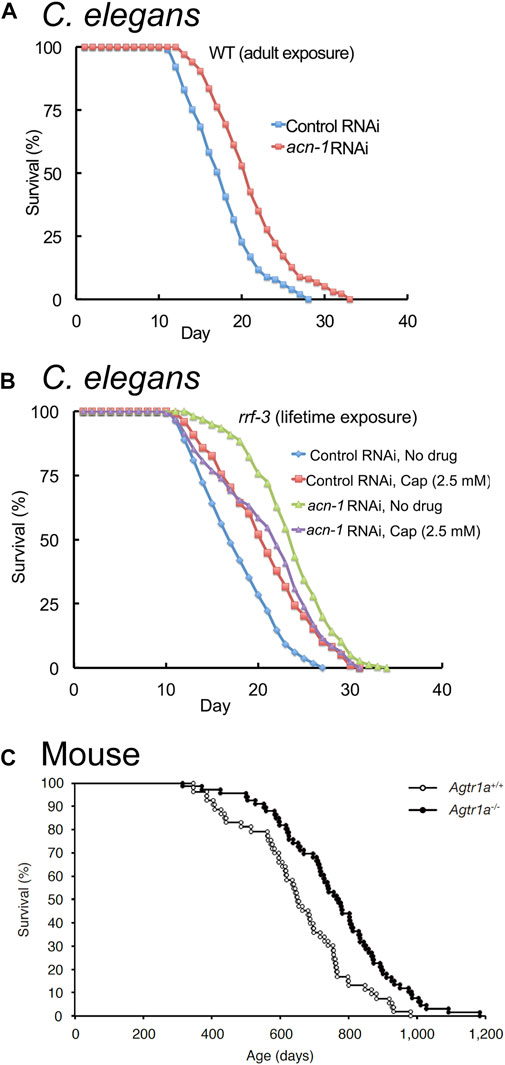
FIGURE 4. Reducing ACE expression extends lifespan in C. elegans and mice. (A) Reducing acn-1 expression via RNA-interference (RNAi) extends adult lifespan in C. elegans by about 20%. Survival curves of wild-type (WT) hermaphrodites exposed to acn-1 or control dsRNA-expressing bacteria from the L4 stage onward at 20°C. (B) Captopril and acn-1 RNAi are not additive in extending the lifespan of adult C. elegans, suggesting that Captopril inhibits acn-1 to control aging. Survival curves of RNAi-hypersensitive strain rrf-3(pk1426) exposed to E. coli bacteria expressing control or acn-1 dsRNA from the embryonic stage and to 2.5 mM Captopril from L4 stage at 20°C. (A,B) Adapted from (Kumar et al., 2016). (C) Knock-out of AGT1R extends adult lifespan in mice by about 17%. Survival curves of Agtr1a−/− and Agtr1a+/+ mice (Sugaya et al., 1995) fed with a standard diet. Adapted from (Yabumoto et al., 2015).
Captopril and the acn-1 gene influence aging in Caenorhabditis elegans
The nematode ACE homolog acn-1 was first identified by Brooks et al. (2003) as necessary for larval development and adult morphogenesis. Green fluorescent protein (GFP)-tagged ACN-1 driven by an acn-1 promoter was expressed in the embryo hypodermis, hypodermal seam cells, and vulva of the L4 hermaphrodite, and the ray papillae in the L4 male tail. acn-1 RNAi-mediated knockdown resulted in larval molting defects, characterized by a failure to shed the cuticle. Gonad injection of dsRNA resulted in larval arrest at L2 and an early mortality phenotype, indicating that it causes a strong reduction of function and acn-1 is necessary for larval survival; feeding of dsRNA-expressing bacteria allows many animals to survive the larval stage, indicating a less severe reduction of function, and animals display cuticle shedding defects in the L3/L4 and L4/adult molts. RNAi-mediated knockdown of nhr-23 and nhr-25 reduced the expression of ACN-1, suggesting that these genes regulate acn-1. Loss of function of these two genes was previously reported to induce cuticle shedding defects similar to acn-1 loss of function (Gissendanner and Sluder, 2000). Indeed, a comprehensive RNAi screen by Frand et al. (2005) identified acn-1, along with nhr-23 and nhr-25, as inducing molting defects. Based on these results, acn-1 appears to act in larval animals to regulate molting downstream of nhr-23 and nhr-25.
Oskouian et al. (2005) replicated the finding of Brooks et al. (2003) that the injection of worms with acn-1 dsRNA resulted in the arrest of larval development. Kumar et al. (2016) did not observe significant larval arrest after acn-1 RNAi feeding treatment. The difference may be due to the method of administration (feeding in the case of Kumar et al. (2016), and injection into the gonad in the cases of Oskouian et al. (2005), Brooks et al. (2003).
The heterochronic pathway controls the timing of molting and other development events by time-dependent expression of genes including several microRNAs. Knockdown of heterochronic pathway components disrupts crucial timing in cell fate decisions, leading to precocious or retarded cell fate phenotypes. Knockdown of acn-1 suppresses the ectopic phenotypes associated with the knockdown of the heterochronic pathway microRNA let-7, and acn-1 expression is modulated throughout the L1 stage in a manner dependent on lin-41 and hbl-1, both targets of let-7 (Metheetrairut et al., 2017). Thus, acn-1 genetically interacts with several components of the heterochronic pathway. Ahn et al. (2006) found that acn-1 transcription was downregulated in tax-6(jh107) mutants. tax-6 is the nematode homolog of the mammalian calcineurin A subunit, and the jh107 allele represents a constitutively active gain-of-function mutation. This suggests a role for tax-6 in the regulation of acn-1 protein expression. Dubois et al. (2019) found that ACN-1 protein expression was affected by exposure to ionizing gamma radiation; protein expression was upregulated following chronic exposure and down-regulated following acute exposure.
Based on sequence alignments, the predicted ACN-1 protein lacks several histidine residues that are ligands for the zinc cofactor necessary for the metalloprotease activity of ACE, suggesting that ACN-1 protein lacks this enzyme activity (Brooks et al., 2003). Biochemical analyses of the catalytic activity of ACN-1 have not been performed, so it remains unknown if ACN-1 has the metalloprotease activity observed in ACE. It has been speculated that ACN-1 may function by binding to and sequestering its substrate, but further research is needed to determine the biochemical function of ACN-1.
While acn-1 function in larval animals is well established, Kumar et al. (2016) showed that acn-1 RNAi treatment initiated after the L4 stage, when the animal has completed all of its larval molts, extends lifespan and delays age-related degenerative changes (Figure 4A; Table 2). This suggests that acn-1 functions in adults to control aging. Further research is required to better understand the function of acn-1 in adult animals.
Kumar et al. (2016) performed a screen for FDA-approved medications that extend lifespan in C. elegans. Captopril, an ACE inhibitor, extended lifespan in hermaphrodite worms by more than 30% (Figure 3A; Table 1); additionally, Captopril treatment delayed age-related degeneration in multiple systems, including body movement and pharyngeal pumping rate. Captopril treatment increased thermotolerance and oxidative stress resistance, phenotypes associated with extended longevity (Kumar et al., 2016). The lifespan extension phenotype appeared to be independent of several known longevity pathways, including the caloric restriction, mitochondrial dysfunction, sirtuin, and TOR signaling pathways.
Kucuktepe (2021) treated animals with Captopril and replicated the lifespan extension, delayed the decline of pharyngeal pumping rate, increased thermotolerance, and increased oxidative stress resistance phenotypes reported by Kumar et al. (2016). Kucuktepe (2021) examined the effect of Captopril administration on lipid levels; triglyceride levels were significantly reduced based on oil red O-staining of L4-stage worms. The primary effect was on intestinal lipid droplets, which were reduced in diameter. Biochemical analysis indicated a reduction in triglycerides and protein content after Captopril treatment.
Kumar et al. (2016), Kucuktepe (2021) both demonstrated that the lifespan extension caused by Captopril treatment was abrogated by a daf-16 loss-of-function mutation. The DAF-16 transcription factor is the terminus of the insulin/insulin-like growth factor (IGF) signaling pathway, a well-characterized pathway that influences aging (Kimura et al., 1997; Tissenbaum and Ruvkun, 1998). Kumar et al. (2016) reported that DAF-16 does not display nuclear localization upon treatment with Captopril. Furthermore, Kucuktepe (2021) observed that the body fat reduction caused by Captopril in wild-type animals was not displayed by daf-16(lf) mutants, suggesting that both lifespan extension and body fat reduction are DAF-16-dependent. Further research is needed to determine the nature of the Captopril–DAF-16 interaction.
Kumar et al. (2016) examined interactions of the ACE inhibitor Captopril with the acn-1 gene. RNAi-mediated inhibition of acn-1 caused many phenotypes similar to Captopril administration, including lifespan extension (Figure 4A). Furthermore, RNAi-mediated knockdown of acn-1 did not further enhance the lifespan extension caused by Captopril treatment (Figure 4B), suggesting that Captopril affects aging by the inhibition of acn-1. In contrast to Kumar et al. (2016), Dalton and Curran (2018) reported that treatment with acn-1 RNAi decreased heat and oxidative stress resistance (Kumar et al., 2016; Dalton and Curran, 2018). These results may be explained by differences in methodology, as the two studies differed in the timing of temperature shift (larval day 1 or 2 in Dalton and Curran (2018) and adult day 3 in Kumar et al., (2016)) and the timing of acn-1 RNAi administration (beginning at hatch for Dalton and Curran (2018) and beginning at L4 for Kumar et al. (2016)).
The Greenwald group generated a compendium of C. elegans genes with homologs in humans, called “Ortholist” (Shaye and Greenwald, 2011; Kim et al., 2018). Based on protein homology, nematode ACN-1 has homologs throughout the Bilaterian clade: in addition to humans, non-human primates, and rodents, ACE homologs are present in Zebrafish, Xenopus, Drosophila, and various other arthropods, suggesting that ACE-like proteins have been retained throughout many animal phyla for at least six-hundred million years of evolutionary history. Moreover, this suggests that the evolution of the ACE protein predated the evolution of the animal circulatory system, implying that the ancestral function of the ACE protein was unrelated to the regulation of blood pressure, especially considering its continued retention in phyla which lack closed circulatory systems. This is further reinforced by the apparent lack of functional homologs to the primary substrate of mammalian ACE, Angiotensinogen, in worms or Drosophila, as well as the lack of functional analogs to mammalian renin or the angiotensinogen receptors in these model organisms. Thus, it is apparent that the ancestral function of ACE was unrelated to blood pressure regulation and that this activity evolved later.
Lisinopril and Ance affect aging in Drosophila melanogaster
The fruit fly Drosophila melanogaster is a valuable model organism for studies of genetics, development, behavior, and aging, having been commonly used for over a century (Castle, 1906; Morgan, 1910; Dunn, 1964; Roberts, 2006). Low maintenance costs, rapid generation time, well-developed genetic techniques, and relatively short lifespans make Drosophila one of the predominant model organisms for aging studies. Several studies have identified pathways that regulate aging including dietary restriction (DR), IGF signaling pathway, and the disruption of components of the mitochondrial electron transport chain (Broughton et al., 2005; Copeland et al., 2009; Altintas et al., 2016). Drosophila has been used to test anti-aging pharmacological interventions due to the availability of high-throughput screening techniques (Giacomotto and Ségalat, 2010; Jafari, 2010; Pandey and Nichols, 2011; Willoughby et al., 2013; Lee and Min, 2019). Compared to C. elegans, Drosophila has greater anatomical similarity to mammals (Copeland et al., 2009; Pandey and Nichols, 2011).
The Drosophila genome encodes two primary homologs of ACE as well as several that are more diverged. Ance (ANgiotensin-Converting Enzyme) is most similar to mammalian ACE (Figure 1C); Ance is expressed throughout the lifespan and plays roles in development and fertility (Houard et al., 1998; Hurst et al., 2003). Acer (angiotensin-converting enzyme-related) is most similar to mammalian ACE2 and plays roles in heart morphogenesis (Crackower et al., 2002). Several other homologous genes, Ance-2 through Ance-5, likely resulted from gene duplication events; these genes do not have established functions and are not predicted to encode catalytically active proteins based on amino acid sequence (Coates et al., 2000). Ance and Acer, in contrast to ACN-1, have highly conserved residues in the active site residues similar to mammalian ACE. These proteins have been demonstrated to be catalytically active, since they can cleave Ang I in purified extracts (Cornell et al., 1995; Coates et al., 2000). ACE inhibitors bind to and inhibit the enzymatic activity of Acer and Ance (Cornell et al., 1995; Akif et al., 2010; Lee et al., 2020).
In agreement with results in worms, RNAi-mediated knockdown of Ance, the primary ACE homolog in Drosophila, extended lifespan (Gabrawy et al., 2019) (Table 2). Lisinopril treatment failed to enhance this effect, suggesting that Lisinopril extends lifespan via the inhibition of Ance (Gabrawy et al., 2019). In contrast, RNAi-mediated knockdown of the ACE2 homolog Acer reduces lifespan. Liao et al. (2013) used tissue-specific RNAi-mediated knockdown to reduce Acer activity in mesoderm and cardiac tissue, resulting in a reduction in lifespan, an increase in heart failure rate, a reduction in heart rate and the fractional shortening of the cardiac tissue, and an increase in the systolic and diastolic diameters. In agreement with this finding, Glover et al. (2019) observed that the genetic knockout of Acer reduced lifespan when fed ad libitum, but that starvation abrogated this reduction (Table 2).
Gabrawy et al. (2019) examined the effect of the ACE inhibitor Lisinopril (Figure 2C) on several strains of Drosophila; in addition to extending lifespan (Figure 3B), Lisinopril increased the physical performance in aged flies, as measured by climbing speed, strength, and endurance (Table 1). Lisinopril treatment reduced aberrant protein aggregation in the muscles of aged flies (Gabrawy et al., 2019). Lisinopril extended lifespan in three genetic backgrounds, although the degree of extension varied. However, the physical performance and protein aggregation in aged flies varied in the degree by which they were influenced by Lisinopril, suggesting that genotype can affect phenotypes caused by Lisinopril in a complex manner.
Ederer et al. (2018) reported a genotype-specific effect of Lisinopril on metabolism; Lisinopril lowered the mitochondrial oxygen consumption in young flies, increased the number of mitochondria in aged flies, and reduced peroxide levels in young flies—however, each of these phenotypes occurred in strains with different genotypes. Genotype-specific effects were also observed in thoracic metabolite concentrations upon treatment with Lisinopril.
Crocco et al. (2022) expanded on this work using genome-wide association studies (GWAS) on 126 Drosophila strains with different genotypes treated with Lisinopril; while the majority of strains displayed extended lifespan and reduced age-related physical decline, some strains displayed reduced lifespan or increased age-related decline. GWAS implicated members of the WNT signaling pathway in the Lisinopril effect on climbing speed, and reducing the expression of some of these genes in skeletal muscle reduced Lisinopril’s beneficial impact on climbing. However, the effect of the WNT signaling pathway on Lisinopril-induced lifespan extension or other aging phenotypes was not reported.
RAS inhibitors can increase lifespan in rodents
Rodents have long been the organism of choice for studying the RAS, given their historical use in drug discovery. Their use in the study of aging is more complicated because of their relatively long lifespan (∼3 years) compared to worms and flies, making studies of the effects of drugs on lifespan more labor-intensive and expensive.
The RAS has been a popular target of study for aging in mice and rats due to its well-studied effects on blood pressure. Several rodent models displaying spontaneous hypertension are in common use; however, in comparison to the large body of evidence examining its effects on blood pressure regulation, relatively few studies have examined the effects of the inhibition of the RAS on aging, normotensive rodents (Jama et al., 2022). In aging hypertensive rodent models, the inhibition of the RAS may increase the survival rate due to alleviation of hypertension-associated morbidity; these effects cannot be distinguished from any beneficial effects on age-related degeneration itself. Therefore, the studies discussed below are only those that use normotensive rodents, which measure the effect of RAS inhibition in aged mice and that use an untreated age-matched cohort as a control.
Genetic knockout of components of the RAS has been shown to influence aging in rodents. Benigni et al. (2009) showed that the knockout of the angiotensin II type I receptor (AGT1R) increased lifespan by 25% in mice, and a similar effect was reported by Yabumoto et al. (2015) (Figure 4C; Table 2). In addition to increased lifespan, Agt1r−/− mice displayed increased late-life cardiac health and mitochondrial function, as well as reduced frailty and healthier skin aging (Benigni et al., 2009; Yabumoto et al., 2015). By contrast, the knockout of ACE2 accelerates aging in mice (Takeshita et al., 2018; Nozato et al., 2019; Takeshita et al., 2020), and the knockout of ACE resulted in detrimental effects on health (Esther et al., 1996) (Table 2); these data suggest that complete knockout of ACE is harmful, whereas complete knockout of AGT1R alone shows beneficial effects.
The effect of Enalapril on aging rodents is well studied. Santos et al. (2009) showed that Enalapril treatment extends the lifespan of rats by 45% on either standard or high-fat diet (Figures 1A, 3C; Table 1) (Santos et al., 2009). It has been noted that many age-related degenerative phenotypes displayed by the cardiovascular and renal systems of normotensive mammals are similar to phenotypes observed in younger hypertensive mammals (Benetos et al., 1993; Pinaud et al., 2007). Thus, high blood pressure may accelerate the aging of the cardiovascular system. Alternatively, high blood pressure may cause pathologies that resemble age-related degeneration but are actually distinct. Treatment with anti-hypertensive medications in the absence of hypertension may have beneficial anti-aging properties (Benetos et al., 1993; Assayag et al., 1997). Cardiovascular health declines with age (Miller and Arnold, 2022) and treatment with Enalapril (González Bosc et al., 2000; de Cavanagh et al., 2003; Carter et al., 2004; Basso et al., 2007; de Cavanagh et al., 2008; Inserra et al., 2009; Carter et al., 2011) or Losartan (González Bosc et al., 2000; de Cavanagh et al., 2003; Basso et al., 2007; de Cavanagh et al., 2008; Inserra et al., 2009; Carter et al., 2011) improve many aspects of the health of aged rodents (Figure 1A; Table 1). Improvements were observed in cardiac (Inserra et al., 1995; González Bosc et al., 2000; Basso et al., 2007) and renal health (Ferder et al., 1994; de Cavanagh et al., 2003; de Cavanagh et al., 2008; Inserra et al., 2009), reduced tumor incidence (Carter et al., 2011), reduced frailty (Keller et al., 2019; de Cavanagh et al., 2008; Carter et al., 2011), and reduced age-associated hypertension (González Bosc et al., 2000; de Cavanagh et al., 2003; Carter et al., 2004; Basso et al., 2007; de Cavanagh et al., 2008; Keller et al., 2019). Enalapril, but not Losartan, reduced body weight in old age, suggesting that RAS inhibition of different targets may result in distinct phenotypes (Ferder et al., 1994; Inserra et al., 1995; González Bosc et al., 2000; Basso et al., 2007; Carter et al., 2011). Body fat and food consumption were reduced, suggesting dietary restriction as a possible mechanism (Carter et al., 2004; Carter et al., 2011). Other studies have implicated mitochondria by demonstrating an increase in mitochondrial number and function late in life and a reduction of reactive oxygen species (Ferder et al., 1994; de Cavanagh et al., 2003; de Cavanagh et al., 2008).
Associations between a polymorphism in the ACE gene and longevity in humans
Human centenarians (>100 years of age) and supercentenarians (>110 years of age) are of interest for studies of aging due to their extreme longevity and extraordinarily healthy lives. In addition to extremely long lifespans, human centenarians exhibit fewer infectious diseases, lower inflammation and cancer rates, and reduced age-related comorbidities such as Alzheimer’s disease, cardiovascular disease, and hypertension (Willcox et al., 2008; Andersen et al., 2012; Hirata et al., 2020; Sato et al., 2021). Many studies, including several meta-analyses, investigate variants that are associated with the phenotype of extreme human longevity (Sebastiani et al., 2013; Revelas et al., 2018). One candidate is the ACE polymorphism rs4340 (Revelas et al., 2018). This polymorphism was discovered by Rigat et al. (1990) and is characterized by the presence or absence of a 287bp Alu repetitive element in intron 16. The allele with the Alu sequence is defined as I (insertion), and the allele without the Alu sequence is defined as D (deletion) (Rigat et al., 1990; Sayed-Tabatabaei et al., 2006). The D allele is most likely an ancestral version because the I allele insertion is absent from the genomes of non-human primates (Batzer et al., 1994; Li et al., 2011). The I and D alleles are not homogeneously distributed among the population (Batzer et al., 1994; Li et al., 2011): The D allele is more frequent in Africa and the Middle East, whereas the I allele is more frequent in East Asia (Li et al., 2011). This polymorphism is likely to have a direct phenotypic consequence on the level of ACE in the plasma since the level of ACE activity is increased in humans with the genotype D/D (Rigat et al., 1990). Cultured primary human endothelial cells with an I/I genotype exhibited lower Ang II levels and a higher cell viability compared to cells with the D/D genotype. D/D cells phenocopied I/I cells after the addition of Captopril, indicating that the phenotype is caused by alterations in ACE activity (Hamdi and Castellon, 2004).
Several studies investigated the association of the ACE I/D polymorphism with pathologies. The D allele is positively associated with hypertension, arteriosclerosis, cardiovascular disease, and diabetic microvascular disorders and is negatively associated with Alzheimer’s disease (Sayed-Tabatabaei et al., 2006). By contrast, the I allele is associated with a higher expression of ACE2, which has been shown to negatively affect health (Delanghe et al., 2020). The positive association of the D allele with extreme longevity was investigated in over 32 studies (Human Ageing Genomic Resources, 2022). Two meta-analysis included (1) 12 studies with a total of 1803 centenarians and 10,485 non-centenarian controls (Garatachea et al., 2013), and (2) eight studies with 2043 individuals over 85 years of age and 8,820 younger controls (Revelas et al., 2018). These studies identified a positive association between increased longevity and the presence of the D allele. The authors speculate that the D allele has a negative impact early in life and may grant a survival advantage in later life by effecting tissue repair and activating the immune system, thus representing a pleiotropic effect in favor of longevity (Revelas et al., 2018). It is important to note that studies with centenarians are cross-sectional rather than longitudinal, and the control group is younger individuals from a different birth cohort. In addition, associations might be caused by nearby polymorphisms that are linked to the D or I allele.
Currently available results suggest the possibility that ACE may have undefined functions in addition to its effect on blood pressure that may be responsible for the longevity effects. Humans treated with ACE inhibitors or ARBs display improvements in some measures of health and a reduction in all-cause mortality; however, no aging-focused study has been performed on normotensive humans treated with these drugs (Zoja et al., 2010; Kwang Chae et al., 2011; Abdel-Zaher et al., 2014; Faglia et al., 2014). Interestingly, reduced mortality was reported in diabetic patients treated with ACE inhibitors but not in patients treated with the cholesterol-lowering medication Statin, suggesting that the effect of ACE inhibitors on human health is at least partially independent of its effect on cardiovascular health (Faglia et al., 2014). Future studies should address the mechanistic basis of ACE-associated longevity with a focus on determining to what degree this longevity is caused by a reduction in blood pressure.
Conclusions and perspectives
The large body of research performed on the RAS over the last several decades makes it clear that the RAS can significantly influence aging. However, several important questions at the intersection of the RAS and aging remain unanswered.
What is the function of the renin–angiotensin system in general, and the angiotensin-converting enzyme in particular, in non-vertebrate animals that lack closed circulatory systems? Treating hypertensive humans with medicines that reduce blood pressure makes them live longer by alleviating the pathologies caused by high blood pressure, including strokes and heart disease. Thus, one model for how these same drugs extend lifespan is by reducing blood pressure. However, RAS inhibition extends lifespan in normotensive rodents. While this might indicate that lowering blood pressure below the normal level extends lifespan, it also hints that there is another mechanism. Furthermore, how does the inhibition of ACE affect aging in animals that lack a closed circulatory system altogether? Clearly, in these animals, the effect cannot be on blood pressure, so there must be an alternative mechanism. Although Drosophila has tissues that are analogous to the vertebrate cardiac and pulmonary systems, C. elegans lacks these systems altogether, yet ACE inhibitors extend lifespan in both of these organisms. We speculate that ACE has some ancestral function that mediates its effect on aging.
It is well established that ACE is an essential enzyme in many organisms; the knockout of ACE or its homologs in other species has severe negative effects on health in mammals and is lethal in Drosophila and C. elegans (Tatei et al., 1995; Esther et al., 1996; Brooks et al., 2003; Kumar et al., 2016; Nichols et al., 2016). ACE likely evolved in a hypothesized most recent common ancestor of the Bilaterian clade; since that time functional ACE homologs have been retained in such diverse phyla as insects (Lamango and Isaac, 1994; Cornell et al., 1995; Wijffels et al., 1996; Wijffels et al., 1997; Loeb et al., 1998; Isaac et al., 1999; Vandingenen et al., 2001; Vandingenen et al., 2002; Ekbote et al., 2003a; Ekbote et al., 2003b; Nathalie et al., 2003; Burnham et al., 2005; Lemeire et al., 2008; Wang et al., 2015; Abu Hasan et al., 2017; Nagaoka et al., 2017; Wang et al., 2019), crustaceans (Smiley and Doig, 1994; Kamech et al., 2007; Sook Chung and Webster, 2008), mollusks (Laurent et al., 1997; Riviere et al., 2011), annelids (Rivière et al., 2004), nematodes (Brooks et al., 2003; Kumar et al., 2016; Metheetrairut et al., 2017; Kucuktepe, 2021), and vertebrates (reviewed in (Lv et al., 2018)). Metalloprotease activity, predicted by the highly conserved histidine-rich HEXXH motif, is retained in all known organisms with an active ACE other than nematode ACN-1, indicating a high degree of evolutionary selective pressure to retain this motif. ACE inhibitors bind to and competitively inhibit this active site, and ACE inhibitors have been shown to function in non-vertebrate animals (Lamango and Isaac, 1994; Smiley and Doig, 1994; Wijffels et al., 1996; Wijffels et al., 1997; Isaac et al., 1999; Vandingenen et al., 2001; Vandingenen et al., 2002; Ekbote et al., 2003a; Ekbote et al., 2003b; Nathalie et al., 2003; Rivière et al., 2004; Vercruysse et al., 2004; Kamech et al., 2007; Lemeire et al., 2008; Sook Chung and Webster, 2008; Nagaoka et al., 2017). ACE has been shown to be involved in fertility in mice, Drosophila, and other arthropods (Wijffels et al., 1996; Wijffels et al., 1997; Loeb et al., 1998; Ramaraj et al., 1998; Isaac et al., 1999; Vandingenen et al., 2002; Ekbote et al., 2003a; Ekbote et al., 2003b; Hurst et al., 2003; Nathalie et al., 2003; Vercruysse et al., 2004; Kamech et al., 2007; Sook Chung and Webster, 2008; Riviere et al., 2011; Nagaoka et al., 2017), being commonly expressed in the testis or ovaries, and effecting sperm motility or progeny production in many species. This is especially interesting considering the existence of a testis-specific isoform of ACE in mammals, called tACE; this isoform has been shown to regulate male fertility and sperm function (Hagaman et al., 1998). ACE has also been implicated in ecdysis (Ekbote et al., 2003a; Ekbote et al., 2003b; Vercruysse et al., 2004; Lemeire et al., 2008; Metheetrairut et al., 2017), being most strongly expressed during larval molts in several species. Thus, ACE plays many roles in many different organisms, but it is likely that its role as a blood pressure regulator was a later development, as this activity is not observed outside of vertebrates. It is likely that ACE evolved from earlier peptidyl dipeptidases and diverged to serve many functions; one later development would be the maturation of blood pressure signaling peptides in a vertebrate ancestor, whose function was retained in modern vertebrates. It is likely, then, that any potential secondary effects on aging were retained in mammals as well, explaining the seemingly dual effects of ACE inhibitors on aging and blood pressure.
The C. elegans homolog of ACE does not conserve some amino acids necessary for the catalytic function, leading to the model that it is not an active enzyme (Brooks et al., 2003). However, it has an important function because the genetic knockout of acn-1 is lethal and it has been implicated as an essential regulator of molting and development, indicating an essential role for acn-1 despite the predicted lack of metalloprotease activity (Brooks et al., 2003; Kumar et al., 2016; Metheetrairut et al., 2017). Kumar et al. (2016) demonstrated that Captopril treatment or knockdown of acn-1 by RNAi still resulted in lifespan extension if administered after the final larval molt, once all known functions of acn-1 have presumably been completed. What is the function of acn-1 in adulthood, and why does inhibiting this function affect aging? It is possible that ACN-1 merely sequesters or is involved in the maturation of its substrate rather than acting through an enzymatic activity, or that it acts in concert with a second enzyme. Further research must be done to determine what, if any, substrate is associated with ACN-1.
The discovery of drugs that influence aging has traditionally been a strength of non-vertebrate model organisms; C. elegans in particular has been a fruitful ecosystem for repurposing well-studied human drugs into potential anti-aging treatments. Two of the most well-studied anti-aging compounds are the FDA-approved medications Metformin and Rapamycin. Metformin was first developed to treat type 2 diabetes, and Rapamycin was approved as an immunosuppressant for organ transplants. Subsequent research in C. elegans (Onken and Driscoll, 2010; Robida-Stubbs et al., 2012; Cabreiro et al., 2013) and Drosophila (Bjedov et al., 2010) demonstrated that these drugs control aging. Metformin has been shown to control aging in nematodes and mice (reviewed in (Glossmann and Lutz, 2019; Hu et al., 2021)). Additionally, the Targeting Research with MEtformin (TAME) project is currently testing the effect of Metformin on mortality in elderly humans (Justice et al., 2018). The National Institute of Aging’s Interventions Testing Program (ITP) has identified Rapamycin as a potent controller of aging, extending lifespan in both male and female mice by more than 10% (Harrison et al., 2009; Miller et al., 2011). Rapamycin has also been tested as a potential anti-aging therapy in non-human primates (Ross et al., 2015) and is currently being tested in canines (Kaeberlein et al., 2016). Captopril is currently undergoing testing by the ITP as well and is on track to become the third FDA-approved drug with potential as an anti-aging therapy. Captopril is an ideal candidate for future human studies due to its long history as a safe, effective blood pressure medication, but it has not yet been tested as an anti-aging therapy in normotensive humans. Future research in model organisms will lay the foundation for testing Captopril and other RAS inhibitors for use as an anti-aging therapy in humans.
Author contributions
BE, AS, FP, and KK provided scientific input and wrote the article. All authors contributed to the article and approved the submitted version.
Funding
The work was supported by the NIH grants R56 AG072169, R21 AG058037, and RO1 AG057748 to KK, the Irving Boime Graduate Student Fellowship to BME, and by a postdoctoral fellowship from the National Ataxia Foundation to FP.
Conflict of interest
The authors declare that the research was conducted in the absence of any commercial or financial relationships that could be construed as a potential conflict of interest.
Publisher’s note
All claims expressed in this article are solely those of the authors and do not necessarily represent those of their affiliated organizations, or those of the publisher, the editors and the reviewers. Any product that may be evaluated in this article, or claim that may be made by its manufacturer, is not guaranteed or endorsed by the publisher.
Abbreviations
ACE, Angiotensin-converting enzyme; ACE2, Angiotensin-converting enzyme 2; Acer, Angiotensin-converting enzyme-related; AGT1R, Angiotensin II type-1 receptor; AGT2R, Angiotensin II type-2 receptor; Ance, ANgiotensin-converting enzyme; AGT, Angiotensinogen; Ang I, Angiotensin I; Ang II, Angiotensin II; ARB, Angiotensin II type-1 receptor blocker; DR, Dietary restriction; GWAS, Genome-wide association study; GFP, Green fluorescent protein; IGF, Insulin-like growth factor; ITP, Interventions Testing Program; RAS, Renin–angiotensin system; RNAi, RNA interference; TOR, Target of Rapamycin.
References
Abdel-Zaher, A. O., Elkoussi, A. E. A., Abudahab, L. H., Elbakry, M. H., and Elsayed, E. A. E. (2014). Effect of simvastatin on the antihypertensive activity of losartan in hypertensive hypercholesterolemic animals and patients: Role of nitric oxide, oxidative stress, and high-sensitivity C-reactive protein. Fundam. Clin. Pharmacol. 28 (3), 237–248. doi:10.1111/fcp.12020
Abiodun, O. A., and Ola, M. S. (2020). Role of brain renin angiotensin system in neurodegeneration: An update. Saudi J. Biol. Sci. 27 (3), 905–912. doi:10.1016/j.sjbs.2020.01.026
Abu Hasan, Z. I., Williams, H., Ismail, N. M., Othman, H., Cozier, G. E., Acharya, K. R., et al. (2017). The toxicity of angiotensin converting enzyme inhibitors to larvae of the disease vectors Aedes aegypti and Anopheles gambiae. Sci. Rep. 71, 45409. doi:10.1038/srep45409
Ahn, D. H., Singaravelu, G., Lee, S., Ahnn, J., and Shim, Y. H. (2006). Functional and phenotypic relevance of differentially expressed proteins in calcineurin mutants of Caenorhabditis elegans. Proteomics 6, 1340–1350. doi:10.1002/pmic.200500315
Akif, M., Georgiadis, D., Mahajan, A., Dive, V., Sturrock, E. D., Isaac, R. E., et al. (2010). High-resolution crystal structures of Drosophila melanogaster angiotensin-converting enzyme in complex with novel inhibitors and antihypertensive drugs. J. Mol. Biol. 400 (3), 502–517. doi:10.1016/j.jmb.2010.05.024
Altintas, O., Park, S., and Lee, S. J. V. (2016). The role of insulin/IGF-1 signaling in the longevity of model invertebrates, C. elegans and D. melanogaster. BMB Rep. 49 (2), 81–92. doi:10.5483/bmbrep.2016.49.2.261
Andersen, S. L., Sebastiani, P., Dworkis, D. A., Feldman, L., and Perls, T. T. (2012). Health span approximates lifespan among many supercentenarians: Compression of morbidity at the approximate limit of lifespan. J. Gerontol. A Biol. Sci. Med. Sci. 67A (4), 395–405. doi:10.1093/gerona/glr223
Assayag, P., Charlemagne, D., de Leiris, J., Boucher, F., Valere, P. E., Lortet, S., et al. (1997). Senescent heart compared with pressure overload–induced hypertrophy. Hypertension 29, 15–21. doi:10.1161/01.HYP.29.1.15
Bader, M. (2010). Tissue renin-angiotensin-aldosterone systems: Targets for pharmacological therapy. Annu. Rev. Pharmacol. Toxicol. 50, 439–465. doi:10.1146/annurev.pharmtox.010909.105610
Balthazar, L., Lages, Y. V. M., Romano, V. C., Landeira-Fernandez, J., and Krahe, T. E. (2021). The association between the renin-angiotensin system and the hypothalamic-pituitary-adrenal axis in anxiety disorders: A systematic review of animal studies. Psychoneuroendocrinology 132, 105354. doi:10.1016/j.psyneuen.2021.105354
Basso, N., Paglia, N., Stella, I., De Cavanagh, E. M. V., Ferder, L., Arnaiz, M. D. R. L., et al. (2005). Protective effect of the inhibition of the renin-angiotensin system on aging. Regul. Pept. 128 (3), 247–252. doi:10.1016/j.regpep.2004.12.027
Basso, N., Cini, R., Pietrelli, A., Ferder, L., Terragno, N. A., and Inserra, F. (2007). Protective effect of long-term angiotensin II inhibition. Am. J. Physiol. - Hear Circ. Physiol. 293, 1351–1358. doi:10.1152/ajpheart.00393.2007
Basso, N., and Terragno, N. A. (2001). History about the discovery of the renin-angiotensin system. Hypertension 38, 1246–1249. doi:10.1161/hy1201.101214
Batzer, M. A., Stoneking, M., Alegria-Hartman, M., Bazan, H., Kass, D. H., Shaikh, T. H., et al. (1994). African origin of human-specific polymorphic Alu insertions. Proc. Natl. Acad. Sci. U. S. A. 91 (25), 12288–12292. doi:10.1073/pnas.91.25.12288
Benetos, A., Laurent, S., Hoeks, A. P., Boutouyrie, P. H., and Safar, M. E. (1993). Arterial alterations with aging and high blood pressure. A noninvasive study of carotid and femoral arteries. Arterioscler. Thromb. 13, 90–97. doi:10.1161/01.ATV.13.1.90
Benigni, A., Corna, D., Zoja, C., Sonzogni, A., Latini, R., Salio, M., et al. (2009). Disruption of the Ang II type 1 receptor promotes longevity in mice. J. Clin. Invest. 119 (3), 524–530. doi:10.1172/JCI36703
Bjedov, I., Toivonen, J. M., Kerr, F., Slack, C., Jacobson, J., Foley, A., et al. (2010). Mechanisms of lifespan extension by rapamycin in the fruit fly Drosophila melanogaster. Cell Metab. 11 (1), 35–46. doi:10.1016/j.cmet.2009.11.010
Brenner, S. (1974). The genetics of Caenorhabditis elegans. Genetics 77 (1), 71–94. doi:10.1093/genetics/77.1.71
Brooks, D. R., Appleford, P. J., Murray, L., and Isaac, R. E. (2003). An essential role in molting and morphogenesis of Caenorhabditis elegans for ACN-1, a novel member of the angiotensin-converting enzyme family that lacks a metallopeptidase active site. J. Biol. Chem. 278 (52), 52340–52346. doi:10.1074/jbc.M308858200
Broughton, S. J., Piper, M. D. W., Ikeya, T., Bass, T. M., Jacobson, J., Driege, Y., et al. (2005). Longer lifespan, altered metabolism, and stress resistance in Drosophila from ablation of cells making insulin-like ligands. Proc. Natl. Acad. Sci. U. S. A. 102 (8), 3105–3110. doi:10.1073/pnas.0405775102
Burnham, S., Smith, J. A., Lee, A. J., Isaac, R. E., and Shirras, A. D. (2005). The angiotensin-converting enzyme (ACE) gene family of Anopheles gambiae. BMC Genomics 6, 172. doi:10.1186/1471-2164-6-172
Cabreiro, F., Au, C., Leung, K. Y., Vergara-Irigaray, N., Cochemé, H. M., Noori, T., et al. (2013). Metformin retards aging in C. elegans by altering microbial folate and methionine metabolism. Cell 153, 228–239. doi:10.1016/j.cell.2013.02.035
Capettini, L. S. A., Montecucco, F., Mach, F., Stergiopulos, N., Santos, R. A. S., da Silva, R. F., et al. (2012). Role of renin-angiotensin system in inflammation, immunity and aging. Curr. Pharm. Des. 18 (7), 963–970. doi:10.2174/138161212799436593
Carhan, A., Tang, K., Shirras, C. A., Shirras, A. D., and Isaac, R. E. (2011). Loss of angiotensin-converting enzyme-related (ACER) peptidase disrupts night-time sleep in adult Drosophila melanogaster. J. Exp. Biol. 214 (4), 680–686. doi:10.1242/jeb.049353
Carroll, P. M., Dougherty, B., Ross-Macdonald, P., Browman, K., and FitzGerald, K. (2003). Model systems in drug discovery: Chemical genetics meets genomics. Pharmacol. Ther. 99 (2), 183–220. doi:10.1016/s0163-7258(03)00059-7
Carter, C. S., Cesari, M., Ambrosius, W. T., Hu, N., Diz, D., Oden, S., et al. (2004). Angiotensin-converting enzyme inhibition, body composition, and physical performance in aged rats. J. Gerontol. Biol. Sci. Copyr. 59, 416–423. doi:10.1093/gerona/59.5.b416
Carter, C. S., Giovaninni, S., Seo, D-O., DuPree, J., Morgan, D., Young Chung, H., et al. (2011). Differential effects of Enalapril and losartan on body composition and indices of muscle quality in aged male Fischer 344 × Brown Norway rats. Age (Omaha) 33, 167–183. doi:10.1007/s11357-010-9196-y
Castle, W. E. (1906). Inbreeding, cross-breeding and sterility in Drosophila. Science 23 (578), 153. doi:10.1126/science.23.578.153
Chalfie, M., Tu, Y., Euskirchen, G., Ward, W. W., and Prasher, D. C. (1994). Green fluorescent protein as a marker for gene expression. Science 263, 802–805. doi:10.1126/science.8303295
Coates, D., Isaac, R. E., Cotton, J., Siviter, R., Williams, T. A., Shirras, A., et al. (2000). Functional conservation of the active sites of human and Drosophila angiotensin I-converting enzyme†. Biochemistry 39, 8963–8969. doi:10.1021/bi000593q
C. elegans Sequencing Consortium (1998). Genome sequence of the nematode C. elegans: A platform for investigating biology. Science 282 (5396), 2012–2018. doi:10.1126/science.282.5396.2012
Copeland, J. M., Cho, J., Lo, T., Hur, J. H., Bahadorani, S., Arabyan, T., et al. (2009). Extension of Drosophila lifespan by RNAi of the mitochondrial respiratory chain. Curr. Biol. 19 (19), 1591–1598. doi:10.1016/j.cub.2009.08.016
Cornell, M. J., Williams, T. A., Lamango, N. S., Coates, D., Corvol, P., Soubrier, F., et al. (1995). Cloning and expression of an evolutionary conserved single-domain angiotensin converting enzyme from Drosophila melanogaster. J. Biol. Chem. 270 (23), 13613–13619. doi:10.1074/jbc.270.23.13613
Crackower, M. A., Sarao, R., Oudit, G. Y., Yagil, C., Kozieradzki, I., Scanga, S. E., et al. (2002). Angiotensin-converting enzyme 2 is an essential regulator of heart function. Nature 417, 822–828. doi:10.1038/nature00786
Crocco, P., De Rango, F., Gabrawy, M. M., Khosravian, N., Morcos, G. S., Morozova, T. V., et al. (2022). Genome-wide analysis in Drosophila reveals the genetic basis of variation in age-specific physical performance and response to ACE inhibition. Genes (Basel) 13, 143. doi:10.3390/genes13010143
Cushman, D. W., and Ondetti, M. A. (1991). History of the design of Captopril and related inhibitors of angiotensin converting enzyme. Hypertension 17, 589–592. doi:10.1161/01.hyp.17.4.589
Dalton, H. M., and Curran, S. P. (2018). Hypodermal responses to protein synthesis inhibition induce systemic developmental arrest and AMPK-dependent survival in Caenorhabditis elegans. PLOS Genet. 14, e1007520. doi:10.1371/journal.pgen.1007520
de Cavanagh, E. M. V., Piotrkowski, B., Basso, N., Stella, I., Inserra, F., Ferder, L., et al. (2003). Enalapril and losartan attenuate mitochondrial dysfunction in aged rats. FASEB J. 17 (9), 1096–1098. doi:10.1096/fj.02-0063fje
de Cavanagh, E. M. V., Flores, I., Ferder, M., Inserra, F., and Ferder, L. (2008). Renin-angiotensin system inhibitors protect against age-related changes in rat liver mitochondrial DNA content and gene expression. Exp. Gerontol. 43 (10), 919–928. doi:10.1016/j.exger.2008.08.007
de Cavanagh, E. M. V., Inserra, F., and Ferder, L. (2011). Angiotensin II blockade: A strategy to slow ageing by protecting mitochondria? Cardiovasc. Res. 89 (1), 31–40. doi:10.1093/cvr/cvq285
Delanghe, J. R., Speekaert, M. M., and Buyzere, M. L. (2020). The host’s angiotensin-converting enzyme polymorphism may explain epidemiological findings in COVID-19 infections. Clin. Chim. Acta. 505, 192–193. doi:10.1016/j.cca.2020.03.031
Dos Reis, M., Thawornwattana, Y., Angelis, K., Telford, M. J., Donoghue, P. C. J., Yang, Z., et al. (2015). Uncertainty in the timing of origin of animals and the limits of precision in molecular timescales. Curr. Biol. 25 (22), 2939–2950. doi:10.1016/j.cub.2015.09.066
Dubois, C., Pophillat, M., Audebert, S., Fourquet, P., Lecomte, C., Dubourg, N., et al. (2019). Differential modification of the C. elegans proteome in response to acute and chronic gamma radiation: Link with reproduction decline. Sci. Total Environ. 676, 767–781. doi:10.1016/j.scitotenv.2019.04.039
Duncia, J. V., Chiu, A. T., Carini, D. J., Gregory, G. B., Johnson, A. L., Price, W. A., et al. (1990). The discovery of potent nonpeptide angiotensin II receptor antagonists: A new class of potent hypertensives. J. Med. Chem. 33, 183–201. doi:10.1021/jm00167a007
Ederer, K. A., Jin, K., Bouslog, S., Wang, L., Gorman, G. S., Rowe, G. C., et al. (2018). Age-and genotype-specific effects of the angiotensin-converting enzyme inhibitor Lisinopril on mitochondrial and metabolic parameters in drosophila melanogaster. Int. J. Mol. Sci. 19 (11), E3351. doi:10.3390/ijms19113351
Ekbote, U., Looker, M., and Isaac, R. E. (2003). ACE inhibitors reduce fecundity in the mosquito, Anopheles stephensi. Comp. Biochem. Physiol. B Biochem. Mol. Biol. 134 (4), 593–598. doi:10.1016/s1096-4959(03)00019-8
Ekbote, U. V., Weaver, R. J., and Isaac, R. E. (2003). Angiotensin I-converting enzyme (ACE) activity of the tomato moth, Lacanobia oleracea: Changes in levels of activity during development and after copulation suggest roles during metamorphosis and reproduction. Insect biochem. Mol. Biol. 33 (10), 989–998. doi:10.1016/s0965-1748(03)00105-x
Esther, C. R., Howard, T. E., Marino, E. M., Goddard, J. M., Capecchi, M. R., Bernstein, K. E., et al. (1996). Mice lacking angiotensin-converting enzyme have low blood pressure, renal pathology, and reduced male fertility. Lab. Invest. 74 (5), 953–965.
Faglia, E., Clerici, G., Scatena, A., Caminiti, M., Curci, V., Morabito, A., et al. (2014). Effectiveness of combined therapy with angiotensin-converting enzyme inhibitors and statins in reducing mortality in diabetic patients with critical limb ischemia: An observational study. Diabetes Res. Clin. Pract. 103 (2), 292–297. doi:10.1016/j.diabres.2013.12.060
Ferder, L., Inserra, F., Romano, L., Ercole, L., and Pszenny Ferder, V. L. (1994). Decreased glomerulosclerosis in aging by angiotensin-converting enzyme inhibitors. J. Am. Soc. Nephrol. 5 (4), 1147–1152. doi:10.1681/ASN.V541147
Fire, A., Xu, S., Montgomery, M. K., Kostas, S. A., Driver, S. E., and Mello, C. C. (1998). Potent and specific genetic interference by double-stranded RNA in Caenorhabditis elegans. Nature 391, 806–811. doi:10.1038/35888
Forouzanfar, M. H., Liu, P., Roth, G. A., Ng, M., Biryukov, S., Marczak, L., et al. (2017). Global burden of hypertension and systolic blood pressure of at least 110 to 115 mm Hg, 1990-2015. JAMA 317, 165–182. doi:10.1001/jama.2016.19043
Fournier, D., Luft, F. C., Bader, M., Ganten, D., and Andrade-Navarro, M. A. (2021). Emergence and evolution of the renin-angiotensin-aldosterone system. J. Mol. Med. 90 (5), 495–508. doi:10.1007/s00109-012-0894-z
Frand, A. R., Russel, S., and Ruvkun, G. (2005). Functional genomic analysis of c. elegans molting. PLoS Biol. 3, e312. doi:10.1371/journal.pbio.0030312(10)
Fyhrquist, F., and Saijonmaa, O. (2008). Renin-angiotensin system revisited. J. Intern. Med. 264 (3), 224–236. doi:10.1111/j.1365-2796.2008.01981.x
Gabrawy, M. M., Campbell, S., Carbone, M. A., Morozova, T. V., Arya, G. H., Turlapati, L. B., et al. (2019). Lisinopril preserves physical resilience and extends lifespan in a genotype-specific manner in Drosophila melanogaster. J. Gerontol. A Biol. Sci. Med. Sci. 74 (12), 1844–1852. doi:10.1093/gerona/glz152
Garatachea, N., Marín, P. J., and Lucia, A. (2013). The ACE dd genotype and D-allele are associated with exceptional longevity: A meta-analysis. Ageing Res. Rev. 12 (4), 1079–1087. doi:10.1016/j.arr.2013.04.001
Giacomotto, J., and Ségalat, L. (2010). High-throughput screening and small animal models, where are we? Br. J. Pharmacol. 160 (2), 204–216. doi:10.1111/j.1476-5381.2010.00725.x
Gissendanner, C. R., and Sluder, A. E. (2000). nhr-25, the Caenorhabditis elegans ortholog of ftz-f1, is required for epidermal and somatic gonad development. Dev. Biol. 221 (1), 259–272. doi:10.1006/dbio.2000.9679
Glossmann, H. H., and Lutz, O. M. D. (2019). Metformin and aging: A review. Gerontology 65, 581–590. doi:10.1159/000502257
Glover, Z., Hodges, M. D., Dravecz, N., Cameron, J., Askwith, H., Shirras, A., et al. (2019). Loss of angiotensin-converting enzyme-related (ACER) peptidase disrupts behavioural and metabolic responses to diet in Drosophila melanogaster. J. Exp. Biol. 222 (8), jeb194332. doi:10.1242/jeb.194332
González Bosc, L., Kurnjek, M. L., Müller, A., Basso, N., and Muller, A. (2000). Effect of chronic angiotensin II inhibition on the cardiovascular system of the normal rat. Am. J. Hypertens. 13 (12), 1301–1307. doi:10.1016/s0895-7061(00)01209-7
Guarente, L., and Kenyon, C. (2000). Genetic pathways that regulate ageing in model organisms. Nature 408 (6809), 255–262. doi:10.1038/35041700
Hagaman, J. R., Moyer, J. S., Bachman, E. S., Sibony, M., Magyar, P. L., Welch, J. E., et al. (1998). Angiotensin-converting enzyme and male fertility. Proc. Natl. Acad. Sci. U. S. A. 95, 2552–2557. doi:10.1073/pnas.95.5.2552
Hamdi, H. K., and Castellon, R. (2004). A genetic variant of ACE increases cell survival: A new paradigm for biology and disease. Biochem. Biophys. Res. Commun. 318 (1), 187–191. doi:10.1016/j.bbrc.2004.04.004
Harrison, D. E., Strong, R., Sharp, Z. D., Nelson, J. F., Astle, C. M., Flurkey, K., et al. (2009). Rapamycin fed late in life extends lifespan in genetically heterogeneous mice. Nature 460, 392. doi:10.1038/nature08221
Hirata, T., Arai, Y., Yuasa, S., Abe, Y., Takayama, M., Sasaki, T., et al. (2020). Associations of cardiovascular biomarkers and plasma albumin with exceptional survival to the highest ages. Nat. Commun. 11 (1), 3820. doi:10.1038/s41467-020-17636-0
Houard, X., Williams, T. A., Michaud, A., Dani, P., Isaac, R. E., Shirras, A. D., et al. (1998). The Drosophila melanogaster-related angiotensin-I-converting enzymes Acer and Ance Distinct enzymic characteristics and alternative expression during pupal development. Eur. J. Biochem. 257, 599–606. doi:10.1046/j.1432-1327.1998.2570599.x
Hu, D., Xie, F., Xiao, Y., Lu, C., Zhong, J., Huang, D., et al. (2021). Metformin: A potential candidate for targeting aging mechanisms. Aging Dis. 12, 480–493. doi:10.14336/AD.2020.0702
Human Ageing Genomic Resources (2022). LongevityMap: Genetic association studies of longevity. Available from: https://genomics.senescence.info/longevity/search.php?search=ACE.
Hurst, D., Rylett, C. M., Isaac, R. E., and Shirras, A. D. (2003). The drosophila angiotensin-converting enzyme homologue Ance is required for spermiogenesis. Dev. Biol. 254 (2), 238–247. doi:10.1016/s0012-1606(02)00082-9
Igic, R., and Igic, R. (2009). A short history of the renin-angiotensin system. Acta Medica Salin. 38, 8–12. doi:10.5457/ams.v38i1.33
Inserra, F., Romano, L., Ercole, L., De Cavanagh, E. M. V., and Ferder, L. (1995). Cardiovascular changes by long-term inhibition of the renin-angiotensin system in aging. Hypertension 25 (3), 437–442. doi:10.1161/01.hyp.25.3.437
Inserra, F., Basso, N., Ferder, M., Userpater, M., Stella, I., Paglia, N., et al. (2009). Changes seen in the aging kidney and the effect of blocking the renin—Angiotensin system. Ther. Adv. Cardiovasc. Dis. 3, 341–346. doi:10.1177/1753944709339195
Isaac, R. E., Ekbote, U., Coates, D., and Shirras, A. D. (1999). Insect angiotensin-converting enzyme: A processing enzyme with broad substrate specificity and a role in reproduction. Ann. N. Y. Acad. Sci. 897, 342–347. doi:10.1111/j.1749-6632.1999.tb07904.x
Jafari, M. (2010). Drosophila melanogaster as a model system for the evaluation of anti-aging compounds. Fly 4 (3), 253–257. doi:10.4161/fly.4.3.11997
Jama, H. A., Muralitharan, R. R., Xu, C., O’Donnell, J. A., Bertagnolli, M., Broughton, B. R. S., et al. (2022). Rodent models of hypertension. Br. J. Pharmacol. 179 (5), 918–937. doi:10.1111/bph.15650
Jaworska, K., Koper, M., and Ufnal, M. (2021). Gut microbiota and renin-angiotensin system: A complex interplay at local and systemic levels. Am. J. Physiol. - Gastrointest. Liver Physiol. 321, G355–G366. doi:10.1152/ajpgi.00099.2021
Jensen, C., Herold, P., and Brunner, H. R. (2008). Aliskiren: The first renin inhibitor for clinical treatment. Nat. Rev. Drug Discov. 7, 399–410. doi:10.1038/nrd2550
Johnson, T. E. (1987). Aging can be genetically dissected into component processes using long-lived lines of Caenorhabditis elegans. Proc. Natl. Acad. Sci. U. S. A. 84 (11), 3777–3781. doi:10.1073/pnas.84.11.3777
Jones, A. K., Buckingham, S. D., and Sattelle, D. B. (2005). Chemistry-to-gene screens in Caenorhabditis elegans. Nat. Rev. Drug Discov. 44, 321–330. doi:10.1038/nrd1692
Justice, J. N., Ferrucci, L., Newman, A. B., Aroda, V. R., Bahnson, J. L., Divers, J., et al. (2018). A framework for selection of blood-based biomarkers for geroscience-guided clinical trials: Report from the TAME biomarkers workgroup. GeroScience 40, 419–436. doi:10.1007/s11357-018-0042-y
Kaeberlein, M., Creevy, K. E., and Promislow, D. E. L. (2016). The dog aging project: Translational geroscience in companion animals. Mamm. Genome 27, 279–288. doi:10.1007/s00335-016-9638-7
Kaeberlein, M., Powers, R. W., Steffen, K. K., Westman, E. A., Hu, D., Dang, N., et al. (2005). Regulation of yeast replicative lifespan by TOR and Sch9 response to nutrients. Science 310, 1193–1196. doi:10.1126/science.1115535
Kamech, N., Simunic, J., Franklin, S. J., Francis, S., Tabitsika, M., Soyez, D., et al. (2007). Evidence for an angiotensin-converting enzyme (ACE) polymorphism in the crayfish Astacus leptodactylus. Peptides 28 (7), 1368–1374. doi:10.1016/j.peptides.2007.06.009
Kamo, T., Akazawa, H., Suzuki, J. I., and Komuro, I. (2016). Roles of renin-angiotensin system and Wnt pathway in aging-related phenotypes. Inflamm. Regen. 36, 12. doi:10.1186/s41232-016-0018-1(1)
Keller, K., Kane, A., Heinze-Milne, S., Grandy, S. A., and Howlett, S. E. (2019). Chronic treatment with the ace inhibitor Enalapril attenuates the development of frailty and differentially modifies pro- and anti-inflammatory cytokines in aging male and female c57bl/6 mice. J. Gerontol. A Biol. Sci. Med. Sci. 74 (8), 1149–1157. doi:10.1093/gerona/gly219
Kenyon, C. (2001). A conserved regulatory system for aging. Cell 105 (2), 165–168. doi:10.1016/s0092-8674(01)00306-3
Kim, W., Underwood, R. S., Greenwald, I., and Shaye, D. D. (2018). OrthoList 2: A new comparative genomic analysis of human and Caenorhabditis elegans genes. Genetics 210, 445. doi:10.1534/genetics.118.301307
Kimura, K. D., Tissenbaum, H. A., Liu, Y., and Ruvkun, G. (1997). Daf-2, an insulin receptor-like gene that regulates longevity and diapause in Caenorhabditis elegans. Science 277 (5328), 942–946. doi:10.1126/science.277.5328.942
Kucuktepe, S. (2021). The anti-obesity effects of the angiotensin-converting enzyme (ACE) inhibitor Captopril – metabolomics and transcriptomics studies. Victoria, Australia: La Trobe.
Kumar, S., Dietrich, N., and Kornfeld, K. (2016). Angiotensin converting enzyme (ACE) inhibitor extends Caenorhabditis elegans lifespan. PLOS Genet. 12, e1005866. doi:10.1371/journal.pgen.1005866
Kwang Chae, Y., Valsecchi, M. E., Kim, J., Lucca Bianchi, A., Khemasuwan, D., Desai, A., et al. (2011). Cancer investigation reduced risk of breast cancer recurrence in patients using ACE inhibitors, ARBs, and/or statins causation and prevention reduced risk of breast cancer recurrence in patients using ACE inhibitors, ARBs, and/or statins. Cancer Invest. 29, 585–593.
Lamango, N. S., and Isaac, R. E. (1994). Identification and properties of a peptidyl dipeptidase in the housefly, Musca domestica, that resembles mammalian angiotensin-converting enzyme. Biochem. J. 299, 651–657. doi:10.1042/bj2990651
Laurent, V., Stefano, G., and Salzet, M. (1997). Presence and biochemical properties of a molluscan invertebrate angiotensin-converting enzyme. Regul. Pept. 69 (2), 53–61. doi:10.1016/s0167-0115(96)02121-0
Lavoie, J. L., and Sigmund, C. D. (2003). Minireview: Overview of the renin-angiotensin system—an endocrine and paracrine system. Endocrinology 144, 2179–2183. doi:10.1210/en.2003-0150
Le, D., Brown, L., Malik, K., and Murakami, S. (2021). Two opposing functions of angiotensin-converting enzyme (ACE) that links hypertension, dementia, and aging. Int. J. Mol. Sci. 22, 13178. doi:10.3390/ijms222413178
Leal, C. R. V., Costa, L. B., Ferreira, G. C., Ferreira, A. de M., Reis, F. M., Simões e Silva, A. C., et al. (2022). Renin-angiotensin system in normal pregnancy and in preeclampsia: A comprehensive review. Pregnancy Hypertens. 28, 15–20. doi:10.1016/j.preghy.2022.01.011
Lee, S. H., Gomes, S. M., Boulianne, G. L., Ghalayini, J., and Iliadi, K. G. (2020). Angiotensin converting enzyme inhibitors and angiotensin receptor blockers rescue memory defects in drosophila-expressing Alzheimer’s disease-related transgenes independently of the canonical renin angiotensin system. eNeuro 7. doi:10.1523/ENEURO.0235-20.2020
Lee, S. H., and Min, K. J. (2019). Drosophila melanogaster as a model system in the study of pharmacological interventions in aging. Transl. Med. Aging 3, 98–103. doi:10.1016/j.tma.2019.09.004
Lemeire, E., Vanholme, B., Van Leeuwen, T., Van Camp, J., and Smagghe, G. (2008). Angiotensin-converting enzyme in Spodoptera littoralis: Molecular characterization, expression and activity profile during development. Insect biochem. Mol. Biol. 38 (2), 166–175. doi:10.1016/j.ibmb.2007.10.004
Li, X., Sun, X., Li, J., and Xue, F. (2011). Worldwide spatial genetic structure of angiotensin-converting enzyme gene: A new evolutionary ecological evidence for the thrifty genotype hypothesis. Eur. J. Hum. Genet. 19, 1002–1008. doi:10.1038/ejhg.2011.66
Liao, F-T., Chang, C-Y., Su, M-T., and Kuo, W-C. (2013). Necessity of angiotensin-converting enzyme-related gene for cardiac functions and longevity of Drosophila melanogaster assessed by optical coherence tomography. J. Biomed. Opt. 19, 011014. doi:10.1117/1.JBO.19.1.011014
Loeb, M. J., De Loof, A., Schoofs, L., Isaac, E., and De Loof, A. (1998). Angiotensin II and angiotensin-converting enzyme as candidate compounds modulating the effects of testis ecdysiotropin in testes of the gypsy moth, Lymantria dispar1. Gen. Comp. Endocrinol. 112 (2), 232–239. doi:10.1006/gcen.1998.7169
Lv, Y., Li, Y., Yi, Y., Zhang, L., Shi, Q., and Yang, J. (2018). A genomic survey of angiotensin-converting enzymes provides novel insights into their molecular evolution in vertebrates. Molecules 23, 2923. doi:10.3390/molecules23112923
Metheetrairut, C., Ahuja, Y., and Slack, F. J. (2017). acn-1, a C. elegans homologue of ACE, genetically interacts with the let-7 microRNA and other heterochronic genes. Cell Cycle 16 (19), 1800–1809. doi:10.1080/15384101.2017.1344798
Miller, A. J., and Arnold, A. C. (2022). The renin-angiotensin system and cardiovascular autonomic control in aging. Peptides 150, 170733. doi:10.1016/j.peptides.2021.170733
Miller, R. A., Harrison, D. E., Astle, C. M., Baur, J. A., Boyd, A. R., De Cabo, R., et al. (2011). Rapamycin, but not resveratrol or simvastatin, extends lifespan of genetically heterogeneous mice. Journals Gerontol. Ser. A 66A, 191–201. doi:10.1093/gerona/glq178
Mills, K. T., Stefanescu, A., and He, J. (2020). The global epidemiology of hypertension. Nat. Rev. Nephrol. 16 (4), 223–237. doi:10.1038/s41581-019-0244-2
Mogi, M. (2020). Effect of renin–angiotensin system on senescence. Geriatr. Gerontol. Int. 20, 520–525. doi:10.1111/ggi.13927
Morgan, T. H. (1910). Sex limited inheritance in Drosophila. Science 32 (812), 120–122. doi:10.1126/science.32.812.120
Nagaoka, S., Kawasaki, S., Kawasaki, H., and Kamei, K. (2017). The angiotensin converting enzyme (ACE) inhibitor, Captopril disrupts the motility activation of sperm from the silkworm, Bombyx mori. J. Insect Physiol. 103, 18–28. doi:10.1016/j.jinsphys.2017.09.007
Nathalie, M., Anick, V., Constant, G., Korneel, H., Geert, B., Liliane, S., et al. (2003). Isolation of angiotensin converting enzyme from testes of Locusta migratoria (Orthoptera). Eur. J. Entomology 100, 467–474. doi:10.14411/eje.2003.070
Nehme, A., Zouein, F. A., Zayeri, Z. D., and Zibara, K. (2019). An update on the tissue renin angiotensin system and its role in physiology and pathology. J. Cardiovasc. Dev. Dis. 6, 14. doi:10.3390/jcdd6020014
Nichols, H. J., Zecherle, L., and Arbuckle, K. (2016). Patterns of philopatry and longevity contribute to the evolution of post-reproductive lifespan in mammals. Biol. Lett. 12, 20150992. doi:10.1098/rsbl.2015.0992
Nozato, S., Yamamoto, K., Takeshita, H., Nozato, Y., Imaizumi, Y., Fujimoto, T., et al. (2019). Angiotensin 1-7 alleviates aging-associated muscle weakness and bone loss, but is not associated with accelerated aging in ACE2-knockout mice. Clin. Sci. 133 (18), 2005–2018. doi:10.1042/CS20190573
Ondetti, M. A., Rubin, B., and Cushman, D. W. (1977). Design of specific inhibitors of angiotensin-converting enzyme: New class of orally active antihypertensive agents. Science 4288, 441–444. doi:10.1126/science.191908
Onken, B., and Driscoll, M. (2010). Metformin induces a dietary restriction-like state and the oxidative stress response to extend C. elegans healthspan via AMPK, LKB1, and SKN-1. PLoS One 5, e8758. doi:10.1371/journal.pone.0008758
Oskouian, B., Mendel, J., Shocron, E., Lee, M. A., Fyrst, H., Saba, J. D., et al. (2005). Regulation of sphingosine-1-phosphate lyase gene expression by members of the GATA family of transcription factors. J. Biol. Chem. 280 (18), 18403–18410. doi:10.1074/jbc.M410928200
Pandey, U. B., and Nichols, C. D. (2011). Human disease models in Drosophila melanogaster and the role of the fly in therapeutic drug discovery. Pharmacol. Rev. 63 (2), 411–436. doi:10.1124/pr.110.003293
Peach, M. J. (1977). Renin angiotensin system: Biochemistry and mechanisms of action. Physiol. Rev. 57 (2), 313–370. doi:10.1152/physrev.1977.57.2.313
Pinaud, F., Bocquet, A., Dumont, O., Retailleau, K., Baufreton, C., Andriantsitohaina, R., et al. (2007). Paradoxical role of angiotensin II type 2 receptors in resistance arteries of old rats. Hypertens 50, 96–102. (Dallas, Tex 1979). doi:10.1161/HYPERTENSIONAHA.106.085035
Powers, R. W., Kaeberlein, M., Caldwell, S. D., Kennedy, B. K., and Fields, S. (2006). Extension of chronological lifespan in yeast by decreased TOR pathway signaling. Genes Dev. 20, 174–184. doi:10.1101/gad.1381406
Ramaraj, P., Kessler, S. P., Colmenares, C., and Sen, G. C. (1998). Selective restoration of male fertility in mice lacking angiotensin-converting enzymes by sperm-specific expression of the testicular isozyme. J. Clin. Invest. 102, 371–378. doi:10.1172/JCI3545
Reid, I. A., Morris, B. J., and Ganong, W. F. (1978). The renin-angiotensin system. Ann. Rev. Physiol. 40, 377–410.
Revelas, M., Thalamuthu, A., Oldmeadow, C., Evans, T. J., Armstrong, N. J., Kwok, J. B., et al. (2018). Review and meta-analysis of genetic polymorphisms associated with exceptional human longevity. Mech. Ageing Dev. 175, 24–34. doi:10.1016/j.mad.2018.06.002
Rigat, B., Hubert, C., Alhenc-Gelas, F., Cambien, F., Corvol, P., Soubrier, F., et al. (1990). An insertion/deletion polymorphism in the angiotensin I-converting enzyme gene accounting for half the variance of serum enzyme levels. J. Clin. Invest. 86 (4), 1343–1346. doi:10.1172/JCI114844
Riviere, G., Fellous, A., Franco, A., Bernay, B., and Favrel, P. (2011). A crucial role in fertility for the oyster angiotensin-converting enzyme orthologue CgACE. PLoS One 12, e27833. doi:10.1371/journal.pone.0027833
Rivière, G., Michaud, A., Deloffre, L., Vandenbulcke, F., Levoye, A., Breton, C., et al. (2004). Characterization of the first non-insect invertebrate functional angiotensin-converting enzyme (ACE): Leech TtACE resembles the N-domain of mammalian ACE. Biochem. J. 382, 565–573. doi:10.1042/BJ20040522
Roberts, D. B. (2006). Drosophila melanogaster: The model organism. Entomol. Exp. Appl. 121 (2), 93–103. doi:10.1111/j.1570-8703.2006.00474.x
Robida-Stubbs, S., Glover-Cutter, K., Lamming, D. W., Mizunuma, M., Narasimhan, S. D., Neumann-Haefelin, E., et al. (2012). TOR signaling and Rapamycin influence longevity by regulating SKN-1/Nrf and DAF-16/FoxO. Cell Metab. 15 (5), 713–724. doi:10.1016/j.cmet.2012.04.007
Ross, C. N., Salmon, A., Strong, R., Fernandez, E., Javors, M., Richardson, A., et al. (2015). Metabolic consequences of long-term Rapamycin exposure on common marmoset monkeys (Callithrix jacchus) repository citation. Aging (Albany NY) 7, 964. doi:10.18632/aging.100843
Santos, R. A. S., Simoes e Silva, A. C., Maric, C., Silva, D. M. R., Machado, R. P., De Buhr, I., et al. (2003). Angiotensin-(1-7) is an endogenous ligand for the G protein-coupled receptor Mas. Proc. Natl. Acad. Sci. U. S. A. 100, 8258–8263. doi:10.1073/pnas.1432869100
Santos, E. L., de Picoli Souza, K., da Silva, E. D., Batista, E. C., Martins, P. J. F., D’Almeida, V., et al. (2009). Long term treatment with ACE inhibitor Enalapril decreases body weight gain and increases lifespan in rats. Biochem. Pharmacol. 78 (8), 951–958. doi:10.1016/j.bcp.2009.06.018
Santos, R. A. S., Oudit, G. Y., Verano-Braga, T., Canta, G., Steckelings, U. M., and Bader, M. (2019). The renin-angiotensin system: Going beyond the classical paradigms. Am. J. Physiol. - Hear Circ. Physiol. 316, H958–H970. doi:10.1152/ajpheart.00723.2018
Sato, Y., Atarashi, K., Plichta, D. R., Arai, Y., Sasajima, S., Kearney, S. M., et al. (2021). Novel bile acid biosynthetic pathways are enriched in the microbiome of centenarians. Nature 599 (7885), 458–464. doi:10.1038/s41586-021-03832-5
Sayed-Tabatabaei, F. A., Oostra, B. A., Isaacs, A., Van Duijn, C. M., and Witteman, J. C. M. (2006). ACE polymorphisms. Circ. Res. 98 (9), 1123–1133. doi:10.1161/01.RES.0000223145.74217.e7
Sebastiani, P., Bae, H., Sun, F. X., Andersen, S. L., Daw, E. W., Malovini, A., et al. (2013). Meta-analysis of genetic variants associated with human exceptional longevity. Aging (Albany NY) 5 (9), 653–661. doi:10.18632/aging.100594
Shaye, D. D., and Greenwald, I. (2011). OrthoList: A compendium of C. elegans genes with human orthologs. PLoS One 6, e20085. doi:10.1371/journal.pone.0020085
Simões-Costa, M. S., Vasconcelos, M., Sampaio, A. C., Cravo, R. M., Linhares, V. L., Hochgreb, T., et al. (2005). The evolutionary origin of cardiac chambers. Dev. Biol. 277 (1), 1–15. doi:10.1016/j.ydbio.2004.09.026
Singh, G. M., Danaei, G., Pelizzari, P. M., Lin, J. K., Cowan, M. J., Stevens, G. A., et al. (2012). The age associations of blood pressure, cholesterol, and glucose: Analysis of health examination surveys from international populations. Circulation 125, 2204–2211. doi:10.1161/circulationaha.111.058834
Skeggs, L. T., Kahn, J. R., and Shumway, N. P. (1956). The preparation and function of the hypertensin-converting enzyme. J. Exp. Med. 103, 295–299. doi:10.1084/jem.103.3.29510.1084/jem.103.3.295
Smiley, J. W., and Doig, M. T. (1994). Distribution and characterization of angiotensin-converting enzyme-like activity in tissues of the blue crab Callinectes sapidus. Comp. Biochem. Physiol. Comp. Physiol. 108 (4), 491–496. doi:10.1016/0300-9629(94)90332-8
Sook Chung, J., and Webster, S. G. (2008). Angiotensin-converting enzyme-like activity in crab gills and its putative role in degradation of crustacean hyperglycemic hormone. Arch. Insect Biochem. Physiol. 68 (3), 171–180. doi:10.1002/arch.20247
Sugaya, T., Nishimatsu, S. I., Tanimoto, K., Takimoto, E., Yamagishi, T., Imamura, K., et al. (1995). Angiotensin II type 1a receptor-deficient mice with hypotension and hyperreninemia. J. Biol. Chem. 270 (32), 18719–18722. doi:10.1074/jbc.270.32.18719
Sulston, J. E., and Horvitz, H. R. (1977). Post-embryonic cell lineages of the nematode, Caenorhabditis elegans. Dev. Biol. 56 (1), 110–156. doi:10.1016/0012-1606(77)90158-0
Takeshita, H., Yamamoto, K., Nozato, S., Takeda, M., ichiro, Fukada S., Inagaki, T., et al. (2018). Angiotensin-converting enzyme 2 deficiency accelerates and angiotensin 1-7 restores age-related muscle weakness in mice. J. Cachexia Sarcopenia Muscle 9 (5), 975–986. doi:10.1002/jcsm.12334
Takeshita, H., Yamamoto, K., Mogi, M., Nozato, S., Horiuchi, M., Rakugi, H., et al. (2020). Different effects of the deletion of angiotensin converting enzyme 2 and chronic activation of the renin-angiotensin system on muscle weakness in middle-aged mice. Hypertens. Res. 43 (4), 296–304. doi:10.1038/s41440-019-0375-7
Tatei, K., Cai, H., Ip, Y. T., and Levine, M. (1995). Race: A drosophila homologue of the angiotensin converting enzyme. Mech. Dev. 51, 157–168. doi:10.1016/0925-4773(95)00349-5
Tissenbaum, H. A., and Ruvkun, G. (1998). An insulin-like signaling pathway affects both longevity and reproduction in Caenorhabditis elegans. Genetics 148 (2), 703–717. doi:10.1093/genetics/148.2.703
Vandingenen, A., Hens, K., Macours, N., Zhu, W., Janssen, I., Breuer, M., et al. (2001). Captopril, a specific inhibitor of angiotensin converting enzyme, enhances both trypsin and vitellogenin titers in the grey fleshfly Neobellieria bullata. Arch. Insect Biochem. Physiol. 47, 161–167. doi:10.1002/arch.1047
Vandingenen, A., Hens, K., Macours, N., Schoofs, L., De Loof, A., Huybrechts, R., et al. (2002). Presence of angiotensin converting enzyme (ACE) interactive factors in ovaries of the grey fleshfly Neobellieria bullata. Comp. Biochem. Physiol. B Biochem. Mol. Biol. 132 (1), 27–35. doi:10.1016/s1096-4959(01)00529-2
Vercruysse, L., Gelman, D., Raes, E., Hooghe, B., Vermeirssen, V., Van Camp, J., et al. (2004). The angiotensin converting enzyme inhibitor Captopril reduces oviposition and ecdysteroid levels in Lepidoptera. Arch. Insect Biochem. Physiol. 57, 123–132. doi:10.1002/arch.20023
Vijg, J., and Campisi, J. (2008). Puzzles, promises and a cure for ageing. Nature 454, 1065–1071. doi:10.1038/nature07216
Wang, W., Luo, L., Lu, H., Chen, S., Kang, L., Cui, F., et al. (2015). Angiotensin-converting enzymes modulate aphid-plant interactions. Sci. Rep. 5, 8885. doi:10.1038/srep08885
Wang, X., Wang, W., Zhang, W., Li, J., Cui, F., and Qiao, L. (2019). Immune function of an angiotensin-converting enzyme against Rice stripe virus infection in a vector insect. Virology 533, 137–144. doi:10.1016/j.virol.2019.05.007
Wijffels, G., Fitzgerald, C., Gough, J., Riding, G., Elvin, C., Kemp, D., et al. (1996). Cloning and characterisation of angiotensin-converting enzyme from the dipteran species, Haematobia irritans exigua, and its expression in the maturing male reproductive system. Eur. J. Biochem. 237 (2), 414–423. doi:10.1111/j.1432-1033.1996.0414k.x
Wijffels, G., Gough, J., Muharsini, S., Donaldson, A., and Eisemann, C. (1997). Expression of angiotensin-converting enzyme-related carboxydipeptidases in the larvae of four species of fly. Insect biochem. Mol. Biol. 27 (5), 451–460. doi:10.1016/s0965-1748(97)00020-9
Willcox, D. C., Willcox, B. J., Wang, N. C., He, Q., Rosenbaum, M., Suzuki, M., et al. (2008). Life at the extreme limit: Phenotypic characteristics of supercentenarians in Okinawa. J. Gerontol. A Biol. Sci. Med. Sci. 63 (11), 1201–1208. doi:10.1093/gerona/63.11.1201
Willoughby, L. F., Schlosser, T., Manning, S. A., Parisot, J. P., Street, I. P., Richardson, H. E., et al. (2013). An in vivo large-scale chemical screening platform using Drosophila for anti-cancer drug discovery. Dis. Model. Mech. 6 (2), 521–529. doi:10.1242/dmm.009985
Wrapp, D., Wang, N., Corbett, K. S., Goldsmith, J. A., Hsieh, C-L., Abiona, O., et al. (2019). Cryo-EM structure of the 2019-nCoV spike in the prefusion conformation. Science 367, 1260. doi:10.1126/science.abb2507
Yabumoto, C., Akazawa, H., Yamamoto, R., Yano, M., Kudo-Sakamoto, Y., Sumida, T., et al. (2015). Angiotensin II receptor blockade promotes repair of skeletal muscle through down-regulation of aging-promoting C1q expression. Sci. Rep. 25, 14453. doi:10.1038/srep14453
Zoja, C., Corna, D., Gagliardini, E., Conti, S., Arnaboldi, L., Benigni, A., et al. (2010). Adding a statin to a combination of ACE inhibitor and ARB normalizes proteinuria in experimental diabetes, which translates into full renoprotection. Am. J. Physiol. - Ren. Physiol. 299, 1203–1211. doi:10.1152/ajprenal.00045.2010
Keywords: Captopril (ACE-I), longevity, acn-1, ACE-angiotensin-converting enzyme, Ance, Enalapril, Losartan, Lisinopril
Citation: Egan BM, Scharf A, Pohl F and Kornfeld K (2022) Control of aging by the renin–angiotensin system: a review of C. elegans, Drosophila, and mammals. Front. Pharmacol. 13:938650. doi: 10.3389/fphar.2022.938650
Received: 07 May 2022; Accepted: 13 July 2022;
Published: 14 September 2022.
Edited by:
Long Ma, Central South University, ChinaReviewed by:
Yidong Shen, Shanghai Institute of Biochemistry and Cell Biology (CAS), ChinaSamantha Hughes, VU Amsterdam, Netherlands
Copyright © 2022 Egan, Scharf, Pohl and Kornfeld. This is an open-access article distributed under the terms of the Creative Commons Attribution License (CC BY). The use, distribution or reproduction in other forums is permitted, provided the original author(s) and the copyright owner(s) are credited and that the original publication in this journal is cited, in accordance with accepted academic practice. No use, distribution or reproduction is permitted which does not comply with these terms.
*Correspondence: Kerry Kornfeld, kornfeld@wustl.edu