- 1School of Zoology, Tel Aviv University, Tel-Aviv, Israel
- 2Department of Natural and Life Sciences, The Open University of Israel, Ra’anana, Israel
- 3Sagol School of Neuroscience, Tel Aviv University, Tel-Aviv, Israel
It is crucial for living organisms to be in synchrony with their environment and to anticipate circadian and annual changes. The circadian clock is responsible for entraining organisms’ activity to the day-night rhythmicity. Artificial light at night (ALAN) was shown to obstruct the natural light cycle, leading to desynchronized behavioral patterns. Our knowledge of the mechanisms behind these adverse effects of ALAN, however, is far from complete. Here we monitored the stridulation and locomotion behavior of male field crickets (Gryllus bimaculatus), raised under light:dark conditions, before, during, and after exposure to a nocturnal 3-h pulse of different ALAN intensities. The experimental insects were then placed under a constant light regime (of different intensities); their behavior was continuously monitored; and the period of their daily activity rhythms was calculated. The light pulse treatment induced a simultaneous negative (suppressing stridulation) and positive (inducing locomotion) effect, manifested in significant changes in the average level of the specific activity on the night of the pulse compared to the preceding and the following nights. The transition to constant light conditions led to significant changes in the period of the circadian rhythms. Both effects were light-intensity-dependent, indicating the importance of dark nights for both individual and population synchronization.
1 Introduction
The entrainment of a living organism’s circadian clock is mostly accomplished by means of synchronization to light (Aschoff, 1960; Saunders et al., 2002; Merrow et al., 2005; Tomioka and Matsumoto, 2019; Helfrich-Förster, 2020), which is the most reliable environmental signal of the Earth’s cycle. Most animals depend on perceiving the light’s rhythmicity for the anticipation and regulation of their activities: for choosing their foraging and sleep times, and for predator avoidance, as well as for the regulation of internal, hormonal, and molecular processes (Saunders et al., 2002; Kronfeld-Schor et al., 2013; Saunders, 2013; Kuhlman et al., 2018). In accordance with the species’ diurnal, nocturnal, or crepuscular nature, the same input may trigger different internal, and behavioral reactions (Mrosovsky, 1999; Helm et al., 2017).
Any obstruction of the light-dark cycle (or its perception) may affect daily activity rhythms, manifested in either entrainment to the new conditions, free-running behavior (an endogenous internal rhythm, that is not synchronized to the environment), or arrhythmic behavior. Yet another effect may be that of masking (see Aschoff, 1960; Mrosovsky, 1999; Kronfeld-Schor et al., 2017): i.e., an instantaneous reaction to an external signal that does not entrain the rhythm but overrides the circadian clock circuits. Such triggered interference may lead to an increase in activity (i.e., positive masking) or to a decrease in activity (i.e., negative masking), depending on the threshold and susceptibility of the relevant species and specific behavior to the stimuli (Mrosovsky, 1999). For example, light may trigger activity in diurnal species while eliciting an urge to rest in nocturnal species (Helm et al., 2017), a phenomenon that has been described in various species (Aschoff and von Goetz, 1988; Germ and Tomioka, 1998; Cohen et al., 2010; Rotics et al., 2011; Erkert et al., 2012; Spoelstra et al., 2018).
An important and constantly increasing source of obstruction of the light-dark cycle is that of artificial light at night (Hölker et al., 2010; Falchi et al., 2016; Falchi et al., 2019). ALAN reduces (even eliminates) the nocturnal darkness and thus disturbs the circadian entrainment to the daily periodicity. It modifies the time, duration, and intensity of the surrounding lighting conditions, as well as the light spectrum. Each of these parameters may distinctly affect a susceptible animal, depending on the species’ photoreceptive properties and sensitivity. The reported negative impacts of ALAN on the natural behavior of various animals include sleep disruption (Bedrosian et al., 2013; Raap et al., 2015), changes in foraging activity and predation (Sanders et al., 2018; Sanders et al., 2021), loss of spatial orientation (Eisenbeis, 2006; Foster et al., 2021), and disturbance to temporal partitioning and synchronization of activity (Amichai and Kronfeld-Schor, 2019; Levy et al., 2021).
Crickets (Gryllidae) have been used as insect models for behavioral research, neuroethology and, specifically, studies of circadian activity (Huber et al., 1989; Tomioka and Chiba, 1989; Horch et al., 2017). The nocturnal field cricket Gryllus bimaculatus expresses temporal shifts in its locomotor activity, stridulatory activity, or circadian gene expression following exposure to changes in illumination patterns (Loher, 1972; Loher, 1989; Abe et al., 1997; Uryu, 2014; Kutaragi et al., 2018; Tomioka and Matsumoto, 2019). Moreover, in constant darkness the compound eyes of G. bimaculatus display a clear circadian rhythm in their response to stimuli, with a diurnal minimum and a nocturnal maximum (Tomioka and Chiba, 1982), as well as a circadian sensitivity of visual interneurons and serotonin levels (Tomioka et al., 1993). We recently demonstrated the negative impact of ALAN on male field crickets (Levy et al., 2021). Lifelong exposure to even dim ALAN intensity had a desynchronizing effect on the crickets’ stridulation and locomotion behaviors. Recently, we also found tissue-, genes- and light-intensity-dependent changes in circadian gene expression in various body parts of the cricket, including the brain and optic lobes (Levy et al., 2022).
Here we studied the effects of exposing male G. bimaculatus to a pulse of ALAN of different intensities on stridulation and locomotion behavior. We further investigated the effects of a transition from light-dark conditions to constant light on the two behaviors in the same individual. We describe a light-intensity-dependent behavioral reaction, presenting simultaneous changes in distinct activities’ levels, or simultaneous negative and positive masking effects on stridulation and locomotion behavior, respectively. We also demonstrate light-intensity-dependent changes in the period of daily activity rhythms following transition from light:dark (LD) to light:ALAN (LA) or constant light (LL) treatments. The findings from this work emphasize the importance of utilizing a multi-behavioral approach in order to obtain a more complete understanding of the impact of ALAN, and its related mechanisms.
2 Materials and methods
2.1 Cricket rearing conditions
G. bimaculatus crickets were reared in plastic containers equipped with an egg carton shelter under a constant temperature of 26°C ± 1°C and a 12 h light:12 h dark (LD) cycle [compact fluorescent light (CFL, 40W, NeptOn, 6500K)]. Daylight intensities measured above the containers ranged from 250 to 350 lux, while the actual daylight intensities under the shelter ranged from 20 to 60 lux. The crickets were fed three times a week with dog-food pellets and vegetables. The rearing boxes contained water flasks with absorbent cotton wool.
2.2 Experimental set-up
The experimental methods largely followed those described in Levy et al. (2021). In brief, the crickets were maintained individually in custom-made experimental anechoic chambers, preventing intraspecific communication while enabling continuous and simultaneous monitoring of stridulation and locomotion behaviors (see Levy et al., 2021; Figure 1 therein). Stridulation was recorded using a condenser microphone, an amplifier, a computer, and RavenLite2.0.0. Locomotion activity was captured from above by an infra-red (IR) surveillance camera, using motion detection (Ballon, 2015). Illumination was provided using a 5W CFL bulb (NeptOn, 6500 K, Supplementary Figure S1), emitting 40 lux, while lower illumination intensity was obtained by shading the light bulb.
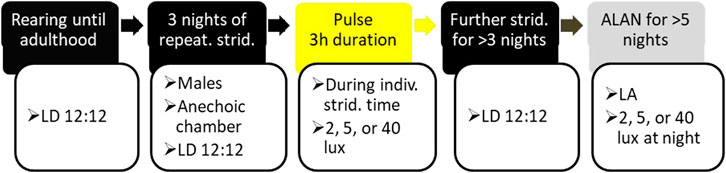
FIGURE 1. The experimental timeline: Adult, male crickets reared under LD 12:12 illumination regime (see Materials and Methods for details) were placed individually, each in an anechoic chamber, and their stridulation and locomotion monitored simultaneously (two left black boxes). On the night following three consecutive nights of consistent stridulation behavior, the experimental crickets were exposed to a light pulse (yellow box) of the duration of three hours and an intensity of 2, 5, or 40 lux. Following three to five additional nights under LD regime (right black box), the experimental animals were subjected to 12 h light:12 h ALAN (LA) or constant light (LL) conditions for at least five consecutive days and nights (grey box).
2.3 Experimental procedure
Adult males 3–7 days post adult emergence were removed from the breeding colony and placed in the anechoic chamber under similar LD conditions as in the colony (for experimental timeline, see Figure 1). Stridulation and locomotion were monitored simultaneously throughout the experiment. The crickets were monitored until reaching three consecutive nights during which consistent stridulation behavior was demonstrated. On the following night, during the same time as that of the preceding consistent stridulation, the experimental cricket was exposed to a 3-h duration light pulse at an intensity of either 2, 5, or 40 lux (Figure 1). The timing of consistent stridulation varied between individuals, and, consequently, also did the precise Zeitgeber time of the pulse. In cases where no consistent behavior could be detected over the course of a ten-night period, the experimental crickets were replaced by new males, until the experimental pre-conditions were reached. Following at least three additional nights under the same LD regime, the experimental animals were subjected to one of several treatments: 24 h cycles of 12 h 40-lux light: 12 h 0-lux dark (LD), 12 h 40-lux light: 12 h 2-lux light (LA2), 12 h 40-lux light: 12 h 5-lux light (LA5), 12 h 40-lux light: 12 h 40-lux light (LL). For each cricket, the continuous night-long ALAN was of the same intensity as that used for the pulse.
2.4 Data processing and statistical analysis
Stridulation data extraction and processing followed Levy et al. (2021), using “R”, version 3.4.1 (R Core Team, 2020), the “Rraven” open source package (Araya-Salas, 2017), and RavenPro1.5 (Bioacoustics Research Program, 2013). Data processing and statistical analyses were conducted in PYTHON v. 3.7 (PyCharm, JetBrains), SPSS version 21 (IBM Corp., Armonk, NY, United States) and PRISM 8 (GraphPad Software, San Diego, CA, United States). The number of stridulation syllables and locomotion events were assessed per animal in 10 min bouts. Values were normalized for each individual by dividing that individual’s values by its own maximum value, resulting in an activity index ranging from 0 to 1 (no activity to maximum activity). Stridulation and locomotion during the exact same hours of the pulse in the night preceding and following the pulse manipulation were averaged and compared to those of the time of the pulse. Stridulation behavior was analyzed using a one-tailed Repeated Measures ANOVA with Tukey’s multiple comparisons, while locomotion behavior was analyzed using a one-tailed non-parametric Friedman test with Dunn’s multiple comparisons. Stridulation and locomotion behaviors were also assessed and compared one hour before the pulse, one hour during the pulse, and one hour after the pulse on the exact same night of the pulse manipulation, using a one-tailed Wilcoxon Signed Ranks Test.
Periodogram analyses of the activity rhythm periods were performed using the ImageJ plugin ActogramJ (Schmid et al., 2011). Comparisons of periodogram analyses of the activity rhythm periods before and after LA or LL, as well as the changes within treatment, the absolute differences between both behaviors, and the quantitative analyses were performed using the Kruskal–Wallis test, followed by a Dunn Sidak correction. Spearman’s rank-order correlation was used to evaluate the relationship between stridulation and locomotion behavior, as well as between the absolute behavioral reaction to the pulse and to the transition to LA or LL for each behavior. Combined actograms of stridulation and locomotion behavior were created using a custom-written code in “R”, version 3.4.1. (Supplementary Material).
3 Results
3.1 Behavioral, temporal patterns under LD conditions
Confirming our previous report (Levy et al., 2021), the experimental crickets exposed to control LD conditions exhibited a synchronized activity rhythm of 24 h, with stridulation being nocturnal and locomotion activity mainly diurnal (Figure 2, top rows in all the panels).
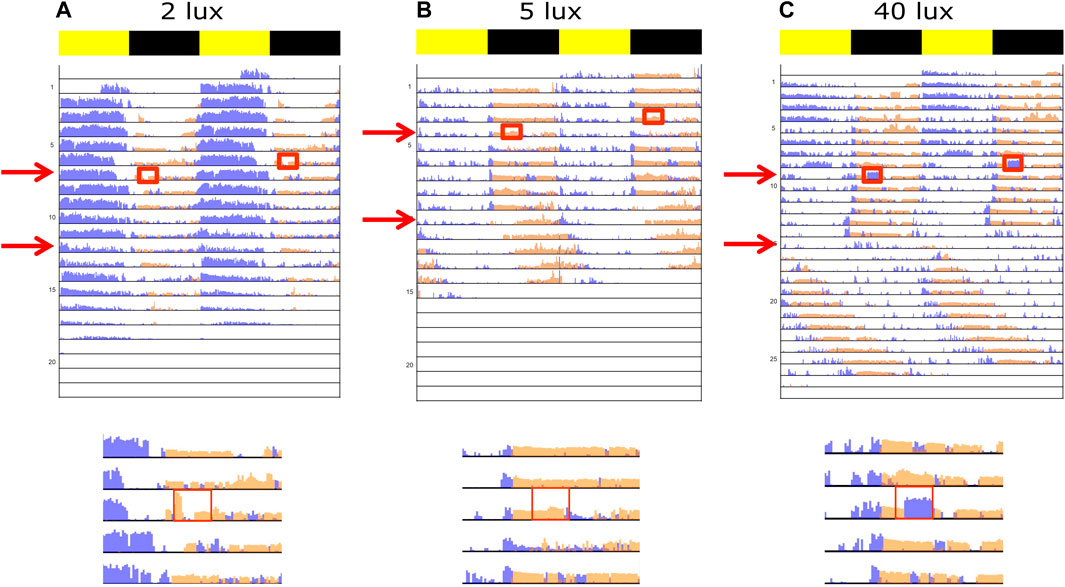
FIGURE 2. Double-plotted actograms representing light-pulse-dependent behavior of experimental crickets. Normalized activity of stridulation (orange) and locomotion (blue) are shown for each light intensity (A) 2 lux, (B) 5 lux, and (C) 40 lux. The first arrow and red rectangle represent the pulse treatment showing either a weak to no behavioral reaction (A, B), or a strong masking reaction to the light pulse (C). The general area of the plot around the time of the pulse was enlarged. The second arrow indicates the transition from LD into LA (constant, night-long ALAN) or LL (constant 40 lux), whereas nocturnal light intensity matched the pulse intensity. Yellow and black bars indicate diurnal and nocturnal phases, respectively.
3.2 A light pulse at night evoked simultaneous negative and positive changes in specific activities’ levels
The effect of a light pulse on the experimental crickets was largely intensity dependent (Figure 2): whereas a behavioral reaction was detected in the 2 lux and 5 lux treatments in only a small number of cases (Figures 3A, B, E, F), a clear simultaneous negative (for stridulation) and positive (for locomotion) masking effect was observed at 40 lux (Figures 2C, 3C, G). Stridulation activity significantly dropped during the light pulse, compared to that on the day prior to the pulse (Repeated Measures ANOVA with Tukey multiple comparisons, F7,7 = 4.257, p = 0.023, n = 8, Figure 3C), while locomotion activity increased (Friedman test with Dunn’s multiple comparisons, χ2(2) = 9.750, p = 0.037, n = 8, Figure 3G). The level of stridulation and locomotion activity during the light pulse was found to be affected by the light intensity (stridulation: between 2 and 40 lux; Kruskal–Wallis with Dunn’s multiple comparisons, χ2,24 = 5.304, p = 0.024, and for locomotion: between 2 and 40 lux, and between 5 and 40 lux; χ2,24 = 13.41, p < 0.01 Figures 3D, H, respectively). While not all individuals seemed to be affected by the pulse, the percentage of animals presenting a clear effect increased with higher light intensity. The pulse seemed to have no residual effect on behavior during the following night: in the 40 lux treatment, activity during the night of the pulse and the night following the pulse differed significantly for both stridulation and locomotion behavior (Repeated Measures ANOVA with Tukey multiple comparisons, F7,7 = 3.609, p = 0.043, n = 8, 1-tailed; Friedman test with Dunn’s multiple comparisons, χ2(2) = 9.750, p = 0.004, n = 8, 1-tailed, Figures 3C, G, respectively). A control group (no pulse treatment) revealed no differences between days (during the duration of the experiments), excluding possible time-dependent differences between the pre-pulse and post-pulse conditions (data not shown). Pre- and post-pulse behavior significantly differed only in the 5 lux stridulation group (Repeated Measures ANOVA, F7,7 = 9.084, p < 0.001, n = 8, Figure 3B).
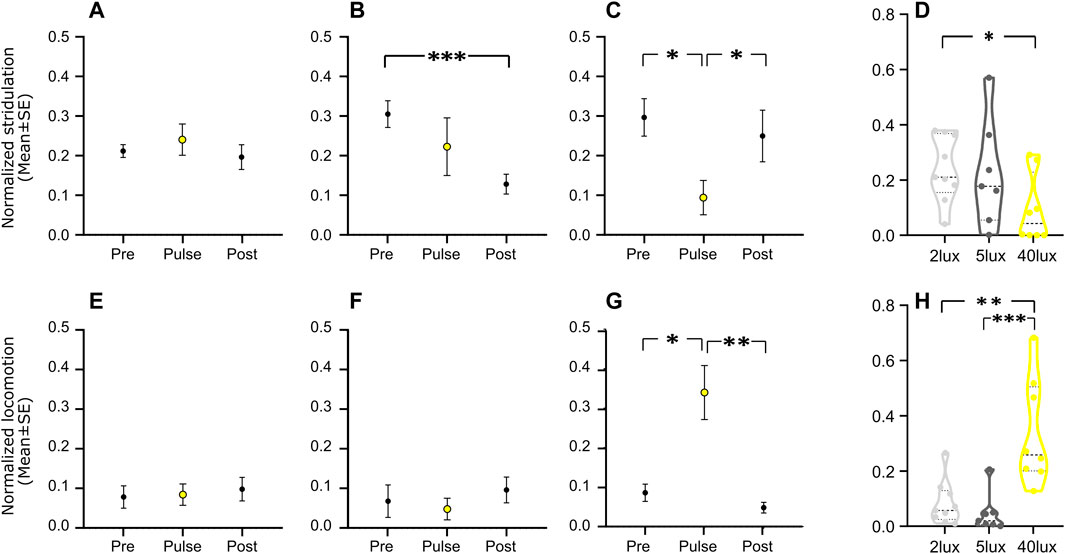
FIGURE 3. Normalized mean level (±s.e.) of stridulation (A–C) and locomotion (E–G) behavior, three nights before (‘Pre’), during the pulse treatment (‘Pulse’, yellow), and three nights after (‘Post’) a pulse of 2 lux (A, E; n = 9), 5 lux (B, F; n = 8), and 40 lux (C, G; n = 8). (D–H) The normalized mean behavior levels (D, stridulation; and H, locomotion) during the pulse treatment under the different pulse intensities. *p < 0.05, **p < 0.01, ***p < 0.001.
Despite of the general absence of behavioral changes on the night following the night of the pulse, we further explored the possibility of pulse-induced effects exceeding the limited period of the pulse duration itself. We therefore compared the behavior of the crickets one hour immediately prior to the pulse treatment, and one hour immediately after the pulse treatment. Figure 4 reveals an overall consistent decrease in stridulation (Figure 4A) and increase in locomotion (Figure 4B) activity during the hour following the pulse termination. The effect was significant for all experimental groups (light intensities) for both observed behaviors (one-tailed Wilcoxon signed ranks test; p < 0.05 for all). The light pulse, thus also induced some residual behavioral effect (Figure 4); even when this had been insignificant during the pulse itself (as in the case of the 2 and 5 lux treatments, Figure 3).
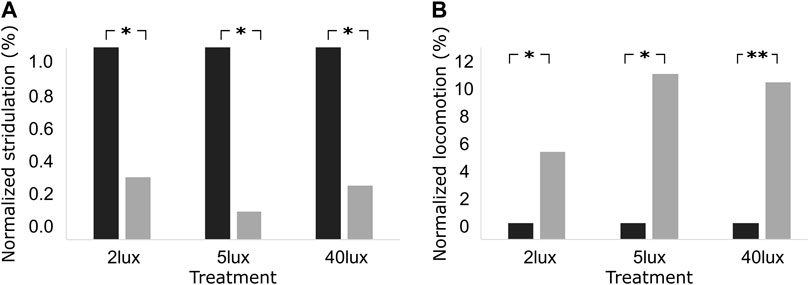
FIGURE 4. Percentage of normalized level of (A) stridulation, and (B) locomotion behavior, one hour before (black), and one hour after (grey) the light pulse treatment of 2 lux, 5 lux, and 40 lux. *p < 0.05, **p < 0.01, representing one-tailed p-values.
3.3 Transition to LA/LL evoked changes in temporal patterns of stridulation and locomotion behaviors
A transition of the experimental conditions from LD to LA and LL affected the behavior of all individuals, as reflected in the high percentage of induced free-run behavior (Figure 5). This differed between the stridulation and locomotion behaviors (94.4%, and 68.75%, respectively; n = 20). For both behaviors, however, the period of the behavioral cycle was found to be light-intensity-dependent (Figure 5): the median period of stridulation behavior increased in a light-intensity-dependent manner, while the median of locomotion behavior increased only at 40 lux (Table 1). The period of stridulation under LD conditions differed significantly from that of the 5 and 40 lux treatments (Kruskal–Wallis with Dunn’s post hoc test, p < 0.005, and p < 0.001, respectively, Figure 5A); while for locomotion behavior, only the 40 lux treatment significantly differed from that of LD (Kruskal–Wallis with Dunn’s post hoc test, p < 0.001, Figure 5B). Thus, even an ALAN of 2 lux leads to free running rhythms of stridulation.
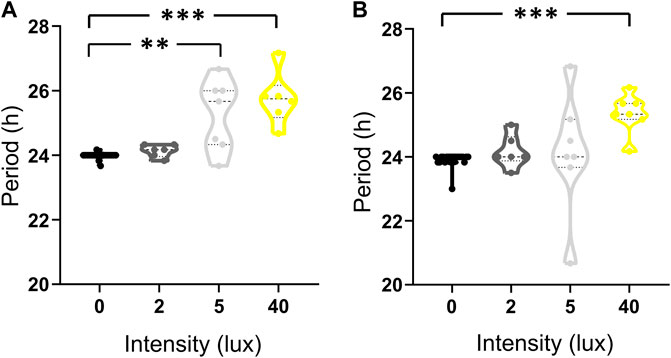
FIGURE 5. The transition from LD to LA or LL affects the individual daily activity periods of stridulation (A) and locomotion (B) in a light intensity dependent manner. LD (before transition, black, n = 20), LA2 lux (dark grey, n = 7), LA5 lux (light grey, n = 7) and LL40 lux (yellow, n = 6, n = 7 for stridulation and locomotion, respectively) treatments. *p < 0.05, **p < 0.01, ***p < 0.001.
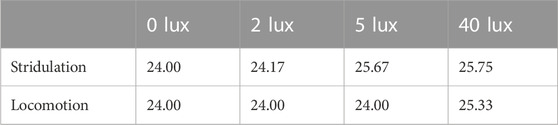
TABLE 1. The median values of stridulation and locomotion daily activity cycle periods in the experimental groups under LD (0 lux, n = 20), LA of 2 lux (n = 6), 5 lux (n = 7), and 40 lux (LL, n = 6, n = 7 for stridulation and locomotion behavior, respectively).
A quantitative comparison of the overall normalized diurnal and nocturnal stridulation activity levels prior to and following the transition to LA and LL revealed a significant change in the diurnal 5 lux and higher activity under 40 lux in both diurnal and nocturnal stridulation (p < 0.05 for all, Kruskal–Wallis test with Dunn’s multiple comparisons). However, no significant quantitative differences were detected in locomotion behavior (p > 0.05 for all, Kruskal–Wallis test). Moreover, no correlation was found between the calculated LA or LL activity periods of stridulation and locomotion (Spearman’s rank-order correlation, r (12) = 0.30, p = 0.29), emphasizing the differential impacts of the treatment on both examined behaviors and its effect on de-synchronization among the two behavioral rhythms.
3.4 No correlation was found between the responses to the pulse and to the transition to LA/LL
To investigate a probable connection between stridulation and locomotion behavior, as well as a possible individual susceptibility to ALAN intensity, the correlation among the different behavioral parameters were calculated. No correlation was found between the absolute effect of the pulse treatment and the absolute change in activity periods following transition to LA or LL (Spearman’s rank-order correlation, r (12) = −0.26, p = 0.36; and r (12) = 0.39, p = 0.17 for stridulation and locomotion, respectively).
4 Discussion
In this study, in order to provide novel insights into the adverse effects of ALAN and into the mechanisms of these effects on animal behavior, we investigated the impact of a pulse of light at night on stridulation and locomotion behavior in the field cricket G. bimaculatus.
Under exposure to 40 lux we identified simultaneous changes in specific activity levels in the same individual crickets; simultaneous negative and positive masking effects on the cricket’s stridulation and locomotion behavior, respectively. Such findings, to the best of our knowledge, have never been described in an insect. The masking phenomenon reflects a flexible, immediate reaction to a stimulus without affecting the animal’s endogenous rhythm (Kuhlman et al., 2018). Aschoff (1960) suggested that light increases diurnal activity in light-active animals and decreases it in dark-active animals. In accord with the Aschoff’s rule, the light pulse evoked a negative masking effect in the crickets’ nocturnal behavior, reflected in a decrease and even cessation of stridulation; while the diurnal behavior (locomotion) increased (positive masking) under the light pulse. Similarly, in rodents, exposure to ALAN was reported to result in negative masking in a nocturnal rodent, while no effect was observed in a diurnal rodent (Rotics et al., 2011). In addition, the presented increase in crickets’ diurnal locomotion activity during the light pulse is in agreement with the previously described increase in burst and intensity of locomotion during a pulse of light (Germ and Tomioka, 1998; though this was demonstrated under constant darkness). The inter-individual variations and light-intensity dependent percentage of crickets presenting a masking response in the current work (as seen in Figure 3), may indicate both an individual and a population-related illumination-sensitivity threshold.
It should be noted, that we had a preference in the current study to present the ALAN-pulse 1–2 h after lights off, as the early and late subjective night were reported to be more affected by a pulse of light (Kutaragi et al., 2016). However, the pulse was not submitted at a specific Zeitgeber time, but rather at the individual’s timing of previous consistent stridulation. These differences may have affected the responsiveness of the experimental individuals, leading to an increased variance.
The reactions of animals to different light stimuli are affected by many factors, such as the species’ way of life (i.e., nocturnal, diurnal; Helm et al., 2017; Kronfeld-Schor and Dayan, 2003; Mrosovsky, 1999), the specific properties of the animal’s visual system (Warrant and Nilsson, 2006; Land and Nilsson, 2012), visual sensory processing (Blum and Labhart, 2000; Okamoto et al., 2001; Mappes and Homberg, 2004), and of course the nature of the specific stimuli. G. bimaculatus is a nocturnal species, which possesses three types of visual receptors: UV (peak: 332 nm), blue (peak: 445 nm) and green (peak: 515 nm) (Zufall et al., 1989), and whose visual system is adapted for signal processing in dim light (Zufall et al., 1989; Sakura et al., 2003; Frolov et al., 2014). While the blue receptor has been reported to be responsible for polarization vision (Labhart et al., 1984; Herzmann and Labhart, 1989), the green-sensitive opsin-long wavelength (OpLW) was described to be the major circadian photoreceptor molecule, responsible for photic entrainment (Komada et al., 2015). Both, the visual and the circadian pathways start at the compound eye. Information from the eye is then transmitted to the optic lobe, where the circadian clock is assumed to reside, and processed in parts of the lamina, medulla and lobula of the optic lobe (Tomioka and Chiba, 1982; Tomioka and Chiba, 1985; Tomioka and Chiba, 1989; Tomioka and Chiba, 1992).
Insects, such as crickets, locusts, and cockroaches, may have several parallel neuronal pathways reaching from the compound eye to the brain, serving for similar or different functions (Okamoto et al., 2001; Homberg et al., 2003; Homberg et al., 2011; Homberg, 2004; Helfrich-Förster, 2020). Whether masking and entrainment use the same neuronal pathway or different ones, is still far from fully resolved. However, the current findings together with available other data suggest a common pathway for both: here we induced masking by a single light pulse, shown previously to affect circadian clock related transcriptional responses in the cricket one hour following the pulse (Levy et al., 2022). Our observations also revealed some residual effects of the pulse, expressed in a change in the percentage of normalized behavioral level one hour after pulse termination. This persisted even under light intensities for which the pulse itself seemed to have no effect. Such a lingering effect has not been described for rodents (Rotics et al., 2011). In a previous study on crickets, repetitive 15 min pulses (at 4, or 8 h intervals) under constant darkness evoked rhythm synchronization to the timing of the light pulses (Germ and Tomioka, 1998). It is therefore probable that in addition to the observed transient change (masking), a 3 h pulse of light at night triggers additional effects, which may be involved in the observed residual changes and maybe also in the entrainment to repetitive stimuli. These results are in line with Aschoff (1960) who suggested a connection between masking and entrainment, which may depend on the frequency and intensity of the stimuli.
The transition to LA or LL evoked a reaction in at least one of the two tested behaviors in all the experimental crickets, including in individuals that seemed unaffected by the earlier pulse. Our findings suggest, therefore, a light-intensity as well as a light-duration-dependent effect of ALAN on cricket behavior. This is in agreement with Germ and Tomioka’s (1998) findings, showing that in crickets frequent light pulses modulated the free-running period, depending on the interval of the pulses. The herein described differences in the median of the period of stridulation and locomotion activity patterns are in agreement with our previous experimental findings regarding lifelong exposure to ALAN illumination (Levy et al., 2021). These differences and the lack of correlation among the two behaviors could indicate a different susceptibility of stridulation and locomotion behaviors to the same light signals. It may also be that the differences reflect a more pronounced effect on the nocturnal than the diurnal behavior, simply since the light signal was presented at night. Our findings indicate no correlation between the stridulation and locomotion activity periods. No correlation was also found between the change during the pulse treatment and the LA/LL-induced change in activity periods for both behaviors. These findings are in accord with those of Levy et al. (2021), suggesting that the desynchronization of stridulation and locomotion activity rhythms reflect control by several peripheral clocks. We suggest that these different results for stridulation and locomotion indicate that the crickets’ behavioral reactions to light may rely on several mechanisms, rather than just one, but remain unclear to date and require further study.
Interestingly, in our previous study (Levy et al., 2021), lifelong illumination patterns evoked a high percentage of arrhythmic stridulation and locomotion behavior (29%, and 42.8%, respectively. In contrast, here, only one individual (14%) subjected to 40 lux (LL) presented arrhythmic behavior. This may be due to one or more of several reasons: First, the complexity of the experiments described in the current report have resulted in a relative small sample size, and it may be possible that a larger sample size would have included some additional arrhythmic individuals. Second, the experiments were conducted only with crickets which demonstrated consistent stridulation behavior during three consecutive nights. This pre-requisite may have filtered out individuals with a stronger tendency for arrhythmicity. Third, the crickets in (Levy et al., 2021) were submitted to lifelong ALAN, whereas the crickets in this study were raised under LD and transferred to ALAN as adults. It may be that the exposure to lifelong ALAN resulted in more arrhythmic crickets.
In summary, to date the response to a light pulse has been mostly studied in relation to phase shifts or entrainment under constant darkness. Here, we present instantaneous masking effects in two key insect behaviors. We describe for the first time a simultaneous pulse-induced negative and positive reaction, as well as a residual effect. We also demonstrate a change in behavior generated by transition to LA or LL conditions. This study and its findings add to the alarming effects of ALAN on living organisms, both individuals and populations. Our findings indicate the importance of darkness for timekeeping in nocturnal insects, and present the harsh, and fast effects of a pulse of light or chronic dim-ALAN on activity rhythms and courtship behavior of the crickets. The findings indicate that not only can life-long or night-long exposure to ALAN alter animal behavior (Rich and Longcore, 2006; Levy et al., 2021; Borges, 2022; Dominoni et al., 2022), but so too can a transient light exposure, such as a single pulse of ALAN (Rotics et al., 2011; Spoelstra et al., 2018; Levy et al., 2022). This aspect should be added to the other possible effects of ALAN, as it may become a stressor when frequently experienced, or even entrain individual behaviors, as shown for the cricket (Germ and Tomioka, 1998). The findings from this study provide further new insights into the importance of a multi-modal approach in order to more fully uncover the effects of ALAN on animal behavior and populations.
Data availability statement
The original contributions presented in the study are included in the article/Supplementary Materials, further inquiries can be directed to the corresponding author.
Author contributions
AA, AB, and KL conceived the study and wrote the manuscript; KL carried out experiments and conducted the data analysis. AB and AA secured the funding.
Funding
This research was funded by The Open University of Israel Research Fund.
Acknowledgments
We thank Yoav Gothilf, Noga Kronfeld-Schor, and Michal Segoli for their seminal idea and contribution to the conceptualization and analysis of the study. We are grateful to Yoav Wegrzyn for his assistance with the analysis and Ronny Efronny for developing the code for the actograms.
Conflict of interest
The authors declare that the research was conducted in the absence of any commercial or financial relationships that could be construed as a potential conflict of interest.
Publisher’s note
All claims expressed in this article are solely those of the authors and do not necessarily represent those of their affiliated organizations, or those of the publisher, the editors and the reviewers. Any product that may be evaluated in this article, or claim that may be made by its manufacturer, is not guaranteed or endorsed by the publisher.
Supplementary material
The Supplementary Material for this article can be found online at: https://www.frontiersin.org/articles/10.3389/fphys.2023.1151570/full#supplementary-material
SUPPLEMENTARY FIGURE S1 | Spectrograms of the light bulb used in the anechoic chamber for all experimental groups (LD, LA2, LA5, and LL). (A) 40 lux, (B) 5 lux, (C) 2 lux. The light spectra were recorded using the Sekonic Sprectromaster C-700 (North White Plans, NY, United States). https://doi.org/10.6084/m9.figshare.22060328.v1 for the code for the actograms Levy, Keren (2023): Actogram two behaviors code. figshare. Journal contribution.
References
Abe, Y., Ushirogawa, H., and Tomioka, K. (1997). Circadian locomotor rhythms in the cricket, Gryllodes sigillatus I. Localization of the pacemaker and the photoreceptor. Zoological Sci. 14 (5), 719–727. doi:10.2108/zsj.14.719
Amichai, E., and Kronfeld-Schor, N. (2019). Artificial light at night promotes activity throughout the night in nesting common swifts (Apus apus). Sci. Rep. 9 (1), 11052–11058. doi:10.1038/s41598-019-47544-3
Araya-Salas, M. (2017). Rraven: Connecting R and raven sound analysis software. Available at: https://cran.r-project.org/web/packages/Rraven/vignettes/Rraven.html.
Aschoff, J. (1960). Exogenous and endogenous components in circadian rhythms. Cold Spring Harb. Symposia Quantitative Biol. 25, 11–28. doi:10.1101/sqb.1960.025.01.004
Aschoff, J., and von Goetz, C. (1988). Masking of circadian activity rhythms in male golden hamsters by the presence of females. Behav. Ecol. Sociobiol. 22 (6), 409–412. doi:10.1007/bf00294978
Ballon, Y. (2015). The effects of different light regimes on activity rhythms of the eastern spadefoot (Pelobates syriacus). Master Thesis (Israel: Tel-Aviv University).
Bedrosian, T. A., Vaughn, C. A., Weil, Z. M., and Nelson, R. J. (2013). Behaviour of laboratory mice is altered by light pollution within the housing environment. Anim. Welf. 22 (4), 483–487. doi:10.7120/09627286.22.4.483
Bioacoustics Research Program (2013). Raven pro: Interactive sound analysis software (version 1.5). Ithaca, NY: The cornell lab of ornithology. Available from: http://www.birds.cornell.edu/raven.2014.
Blum, M., and Labhart, T. (2000). Photoreceptor visual fields, ommatidial array, and receptor axon projections in the polarisation-sensitive dorsal rim area of the cricket compound eye. J. Comp. Physiology. A, Sens. Neural, Behav. Physiology 186 (2), 119–128. doi:10.1007/s003590050012
Borges, R. M. (2022). Impacts of artificial light at night on nocturnal and diurnal insect biology and diversity. Indian J. Entomology 84 (2), 483–492. doi:10.55446/ije.2022.182
Cohen, R., Smale, L., and Kronfeld-Schor, N. (2010). Masking and temporal niche switches in spiny mice. J. Biol. Rhythms 25 (1), 47–52. doi:10.1177/0748730409351672
Dominoni, D. M., de Jong, M., van Oers, K., O’Shaughnessy, P., Blackburn, G. J., Atema, E., et al. (2022). Integrated molecular and behavioural data reveal deep circadian disruption in response to artificial light at night in male Great tits (Parus major). Sci. Rep. 12 (1), 1553. doi:10.1038/s41598-022-05059-4
Eisenbeis, G. (2006). “Artificial night lighting and insects: Attraction of insects to streetlamps in a rural setting in Germany,” in Ecological consequences of artificial night lighting. Editors C. Rich, and T. Longcore (Washington, DC: Island Press), 281–304.
Erkert, H. G., Fernandez-Duque, E., Rotundo, M., and Scheideler, A. (2012). Seasonal variation of temporal niche in wild owl monkeys (Aotus azarai azarai) of the argentinean chaco: A matter of masking? Chronobiology Int. 29 (6), 702–714. doi:10.3109/07420528.2012.673190
Falchi, F., Cinzano, P., Duriscoe, D., Kyba, C. C. M., Elvidge, C. D., Baugh, K., et al. (2016). The new world atlas of artificial night sky brightness. GFZ data services. Sci. Adv. 2, 1–26.
Falchi, F., Furgoni, R., Gallaway, T. A. A., Rybnikova, N. A. A., Portnov, B. A. A., Baugh, K., et al. (2019). Light pollution in USA and Europe: The good, the bad and the ugly. J. Environ. Manag. 248, 109227. doi:10.1016/j.jenvman.2019.06.128
Foster, J. J., Tocco, C., Smolka, J., Khaldy, L., Baird, E., Byrne, M. J., et al. (2021). Light pollution forces a change in dung beetle orientation behavior. Curr. Biol. 31 (17), 3935–3942.e3. doi:10.1016/j.cub.2021.06.038
Frolov, R. V., Immonen, E. V., and Weckström, M. (2014). Performance of blue- and green-sensitive photoreceptors of the cricket Gryllus bimaculatus. J. Comp. Physiol. A Neuroethol. Sens. Neural Behav. Physiol. 200 (3), 209–219. doi:10.1007/s00359-013-0879-6
Germ, M., and Tomioka, K. (1998). Circadian period modulation and masking effects induced by repetitive light pulses in locomotor rhythms of the cricket, Gryllus bimaculatus. Zoological Sci. 15 (3), 309–316. doi:10.2108/zsj.15.309
Helfrich-Förster, C. (2020). Light input pathways to the circadian clock of insects with an emphasis on the fruit fly Drosophila melanogaster. J. Comp. Physiology A 206 (2), 259–272. doi:10.1007/s00359-019-01379-5
Helm, B., Visser, M. E., Schwartz, W., Kronfeld-Schor, N., Gerkema, M., Piersma, T., et al. (2017). Two sides of a coin: Ecological and chronobiological perspectives of timing in the wild. Philosophical Trans. R. Soc. B: Biol. Sci. 372 (1734), 20160246. doi:10.1098/rstb.2016.0246
Herzmann, D., and Labhart, T. (1989). Spectral sensitivity and absolute threshold of polarization vision in crickets: A behavioral study. J. Comp. Physiology A 165 (3), 315–319. doi:10.1007/bf00619350
Hölker, F., Moss, T., Griefahn, B., Kloas, W., Voigt, C. C., Henckel, D., et al. (2010). The dark side of light: A transdisciplinary research agenda for light pollution policy. Ecol. Soc. 15 (4), art13–224. doi:10.5751/es-03685-150413
Homberg, U., Heinze, S., Pfeiffer, K., Kinoshita, M., and El Jundi, B. (2011). Central neural coding of sky polarization in insects. Philosophical Trans. R. Soc. B: Biol. Sci. 366 (1565), 680–687. doi:10.1098/rstb.2010.0199
Homberg, U. (2004). In search of the sky compass in the insect brain. Naturwissenschaften 91 (5), 199–208. doi:10.1007/s00114-004-0525-9
Homberg, U., Reischig, T., and Stengl, M. (2003). Neural organization of the circadian system of the cockroach Leucophaea maderae. Chronobiology Int. 20 (4), 577–591. doi:10.1081/cbi-120022412
Horch, H. W., Mito, T., Popadi, A., Ohuchi, H., and Noji, S. (2017). The cricket as a model organism. Berlin, Germany: Springer.
Huber, F., Moore, T. E., and Loher, W. (1989). Cricket behavior and neurobiology. Ithaca, New York: Cornell University Press.
Komada, S., Kamae, Y., Koyanagi, M., Tatewaki, K., Hassaneen, E., Saifullah, A., et al. (2015). Green-sensitive opsin is the photoreceptor for photic entrainment of an insect circadian clock. Zool. Lett. 1 (1), 11–10. doi:10.1186/s40851-015-0011-6
Kronfeld-Schor, N., Bloch, G., and Schwartz, W. J. (2013). Animal clocks: When science meets nature. Proc. R. Soc. B: Biol. Sci. 280 (1765), 20131354–20131356. doi:10.1098/rspb.2013.1354
Kronfeld-Schor, N., and Dayan, T. (2003). Partitioning of time as an ecological resource. Annu. Rev. Ecol. Evol. Syst. 34, 153–181. doi:10.1146/annurev.ecolsys.34.011802.132435
Kronfeld-Schor, N., Visser, M. E., Salis, L., and van Gils, J. A. (2017). Chronobiology of interspecific interactions in a changing world. Philosophical Trans. R. Soc. B: Biol. Sci. 372 (1734), 20160248. doi:10.1098/rstb.2016.0248
Kuhlman, S. J., Craig, L. M., and Duffy, J. F. (2018). Introduction to chronobiology. Cold Spring Harb. Perspect. Biol. 10 (9), a033613. doi:10.1101/cshperspect.a033613
Kutaragi, Y., Miki, T., Bando, T., and Tomioka, K. (2016). Transcriptional and nontranscriptional events are involved in photic entrainment of the circadian clock in the cricket Gryllus bimaculatus. Physiol. Entomol. 41 (4), 358–368. doi:10.1111/phen.12162
Kutaragi, Y., Tokuoka, A., Tomiyama, Y., Nose, M., Watanabe, T., Bando, T., et al. (2018). A novel photic entrainment mechanism for the circadian clock in an insect: Involvement of c-fos and cryptochromes. Zool. Lett. 4 (1), 26–12. doi:10.1186/s40851-018-0109-8
Labhart, T., Hodel, B., and Valenzuela, I. (1984). The physiology of the cricket’s compound eye with particular reference to the anatomically specialized dorsal rim area. J. Comp. Physiology A 155 (3), 289–296. doi:10.1007/bf00610582
Land, M. F., and Nilsson, D.-E. (2012). in Animal eyes. Editors M. F. Land, and D.-E. Nilsson 2nd ed (Oxford, UK: Oxford University Press).
Levy, K., Fishman, B., Barnea, A., Ayali, A., and Tauber, E. (2022). Transcriptional response of circadian clock genes to an ‘artificial light at night’ pulse in the cricket Gryllus bimaculatus. Int. J. Mol. Sci. 23 (19), 11358. doi:10.3390/ijms231911358
Levy, K., Wegrzyn, Y., Efronny, R., Barnea, A., and Ayali, A. (2021). Lifelong exposure to artificial light at night impacts stridulation and locomotion activity patterns in the cricket Gryllus bimaculatus. Proc. R. Soc. B: Biol. Sci. 288, 20211626. doi:10.1098/rspb.2021.1626
Loher, W. (1972). Circadian control of stridulation in the cricket Teleogryllus commodus Walker. J. Comp. Physiology 79 (2), 173–190. doi:10.1007/bf00697770
Loher, W. (1989). “Temporal organization of reproductive behavior,” in Cricket behavior and neurobiology. Editors F. Huber, T. E. Moore, and W. Loher (Ithaca, New York: Cornell University Press), 83–113.
Mappes, M., and Homberg, U. (2004). Behavioral analysis of polarization vision in tethered flying locusts. J. Comp. Physiology A 190 (1), 61–68. doi:10.1007/s00359-003-0473-4
Merrow, M., Spoelstra, K., and Roenneberg, T. (2005). The circadian cycle: Daily rhythms from behaviour to genes. EMBO Rep. 6 (10), 930–935. doi:10.1038/sj.embor.7400541
Mrosovsky, N. (1999). Masking: History, definitions, and measurement. Chronobiology Int. 16 (4), 415–429. doi:10.3109/07420529908998717
Okamoto, A., Mori, H., and Tomioka, K. (2001). The role of the optic lobe in circadian locomotor rhythm generation in the cricket, Gryllus bimaculatus, with special reference to PDH-immunoreactive neurons. J. Insect Physiology 47 (8), 889–895. doi:10.1016/s0022-1910(01)00061-0
R Core Team (2020). R: A language and environment for statistical computing. Vienna, Austria: R Foundation for Statistical Computing, 2020. 3.4.1.
Raap, T., Pinxten, R., and Eens, M. (2015). Light pollution disrupts sleep in free-living animals. Sci. Rep. 5 (1), 13557–13558. doi:10.1038/srep13557
Rich, C., and Longcore, T. (2006). Ecological consequences of artificial night lighting. Washington, D.C.: Island Press.
Rotics, S., Dayan, T., Levy, O., and Kronfeld-Schor, N. (2011). Light masking in the field: An experiment with nocturnal and diurnal spiny mice under semi-natural field conditions. Chronobiology Int. 28 (1), 70–75. doi:10.3109/07420528.2010.525674
Sakura, M., Takasuga, K., Watanabe, M., and Eguchi, E. (2003). Diurnal and circadian rhythm in compound eye of cricket (Gryllus bimaculatus): Changes in structure and photon capture efficiency. Zoological Sci. 20 (7), 833–840. doi:10.2108/zsj.20.833
Sanders, D., Frago, E., Kehoe, R., Patterson, C., and Gaston, K. J. (2021). A meta-analysis of biological impacts of artificial light at night. Nat. Ecol. Evol. 5 (1), 74–81. doi:10.1038/s41559-020-01322-x
Sanders, D., Kehoe, R., Cruse, D., van Veen, F. J. F., and Gaston, K. J. (2018). Low levels of artificial light at night strengthen top-down control in insect food web. Curr. Biol. 28 (15), 2474–2478. doi:10.1016/j.cub.2018.05.078
Saunders, D. S. (2013). Insect photoperiodism: Measuring the night. J. Insect Physiology 59 (1), 1–10. doi:10.1016/j.jinsphys.2012.11.003
Saunders, D. S., Steel, C. G. H., Vafopoulou, X., and Lewis, R. D. (2002). “Circadian rhythms of activity in individual insects,” in Insect clocks (Academic Press: Elsevier B.V), 7–42.
Schmid, B., Helfrich-Förster, C., and Yoshii, T. (2011). A new ImageJ plug-in “actogramJ” for chronobiological analyses. J. Biol. Rhythms 26 (5), 464–467. doi:10.1177/0748730411414264
Spoelstra, K., Verhagen, I., Meijer, D., and Visser, M. E. (2018). Artificial light at night shifts daily activity patterns but not the internal clock in the great tit (Parus major). Proc. R. Soc. B: Biol. Sci. 285, 20172751. doi:10.1098/rspb.2017.2751
Tomioka, K., and Chiba, Y. (1992). Characterization of an optic lobe circadian pacemaker by in situ and in vitro recording of neural activity in the cricket, Gryllus bimaculatus. J. Comp. Physiology A 171 (1), 1–7. doi:10.1007/bf00195955
Tomioka, K., and Chiba, Y. (1985). Optic lobe-compound eye system in cricket: A complete circadian system. J. Interdiscip. Cycle Res. 16 (1), 73–76. doi:10.1080/09291018509359873
Tomioka, K., and Chiba, Y. (1982). Persistence of circadian ERG rhythm in the cricket with optic tract severed cellular polarity in root epidermis of Gibasis geniculata. Naturwissenschaften 69, 395–396. doi:10.1007/bf00396696
Tomioka, K., and Chiba, Y. (1989). Photoperiodic entrainment of locomotor activity in crickets (Gryllus bimaculatus) lacking the optic lobe pacemaker. J. Insect Physiology 35 (11), 827–835. doi:10.1016/0022-1910(89)90098-x
Tomioka, K., Ikeda, M., Nagao, T., and Tamotsu, S. (1993). Involvement of serotonin in the circadian rhythm of an insect visual system. Naturwissenschaften 80 (3), 137–139. doi:10.1007/bf01131019
Tomioka, K., and Matsumoto, A. (2019). “The circadian system in insects: Cellular, molecular, and functional organization,” in Advances in insect Physiology. 1st ed. (Academic Press: Elsevier), 73–115.
Uryu, O. (2014). “Molecular analysis of the circadian clock system in the cricket,” in Doctoral dissertation: Vol. Okayama un (Japan: Okayam University).
Warrant, E., and Nilsson, D.-E. (2006). “Invertebrate vision,” in Invertebrate vision. Editors E. Warrant, and D.-E. Nilsson 1st ed. (Cambridge, UK: Cambridge University Press), 570.
Keywords: artificial light at night, ALAN, light pollution, masking, insect, circadian rhythm, Gryllus bimaculatus
Citation: Levy K, Barnea A and Ayali A (2023) Exposure to a nocturnal light pulse simultaneously and differentially affects stridulation and locomotion behaviors in crickets. Front. Physiol. 14:1151570. doi: 10.3389/fphys.2023.1151570
Received: 26 January 2023; Accepted: 03 March 2023;
Published: 16 March 2023.
Edited by:
Urs Albrecht, Université de Fribourg, SwitzerlandReviewed by:
Monika Stengl, University of Kassel, GermanyEtienne Challet, Université de Strasbourg, France
Copyright © 2023 Levy, Barnea and Ayali. This is an open-access article distributed under the terms of the Creative Commons Attribution License (CC BY). The use, distribution or reproduction in other forums is permitted, provided the original author(s) and the copyright owner(s) are credited and that the original publication in this journal is cited, in accordance with accepted academic practice. No use, distribution or reproduction is permitted which does not comply with these terms.
*Correspondence: Amir Ayali, ayali@post.tau.ac.il