- Yale University School of Medicine and the Connecticut Mental Health Center, New Haven, CT, United States
Psychotic episodes are debilitating disease states that can cause extreme distress and impair functioning. There are sex differences that drive the onset of these episodes. One difference is that, in addition to a risk period in adolescence and early adulthood, women approaching the menopause transition experience a second period of risk for new-onset psychosis. One leading hypothesis explaining this menopause-associated psychosis (MAP) is that estrogen decline in menopause removes a protective factor against processes that contribute to psychotic symptoms. However, the neural mechanisms connecting estrogen decline to these symptoms are still not well understood. Using the tools of computational psychiatry, links have been proposed between symptom presentation and potential algorithmic and biological correlates. These models connect changes in signaling with symptom formation by evaluating changes in information processing that are not easily observable (latent states). In this manuscript, we contextualize the observed effects of estrogen (decline) on neural pathways implicated in psychosis. We then propose how estrogen could drive changes in latent states giving rise to cognitive and psychotic symptoms associated with psychosis. Using computational frameworks to inform research in MAP may provide a systematic method for identifying patient-specific pathways driving symptoms and simultaneously refine models describing the pathogenesis of psychosis across all age groups.
Introduction
Schizophrenia is a debilitating disorder associated with adverse social, psychological, and biological effects. Most individuals experience their first psychotic episode in their third or fourth decade of life 20–30s (1, 2). During early adulthood, men are 40% more likely to experience their first episode of psychosis (3, 4). However, as women approach menopause, there is an uptick in first-episode psychosis and hospital admissions not seen in men of the same age (5, 6). This menopause-associated psychosis (MAP) is widely recognized; however, very little work has focused on the underlying mechanisms that connect the menopausal transition to psychosis.
It has been proposed that the sudden decline in reproductive hormones during the menopause transition may trigger MAP. The Estrogen Protective Hypothesis posits that estrogen protects against psychotic symptom emergence (7). Evidence for this hypothesis comes from research demonstrating an inverse relationship between estrogen levels and psychotic symptoms: women with schizophrenia have lower estrogen levels (8); hospital admissions for psychosis increase during periods associated with low estrogen [luteal phase of menstruation, menopause, and post-partum (9–15)]; further, there is evidence, albeit less consistent, that estrogen treatments and contraceptives supplement antipsychotics in reducing symptoms (16–18). While there is evidence linking low estrogen levels with psychosis, there has been limited focus on how estrogen may alter the underlying processes that contribute to psychotic states.
Understanding MAP requires the same multifaceted approach as other complex medical disorders, which have signs and symptoms that arise as the result of underlying pathophysiological processes that are not directly observable. Take, for instance, hypothyroidism. Patients with this disorder may first present with symptoms like difficulty with exercising and breathing issues (19). These observable symptoms arise as the result of processes that are only observed on specific testing: a cascade of abnormalities starting with low thyroid hormone T4 and progressing to decreased T3 levels, downregulation of ATPase, low cytosolic calcium levels, and finally, effects on muscle tissues leading to weakness and the difficulty breathing that lead to presenting symptoms (Figure 1A) (19–22).
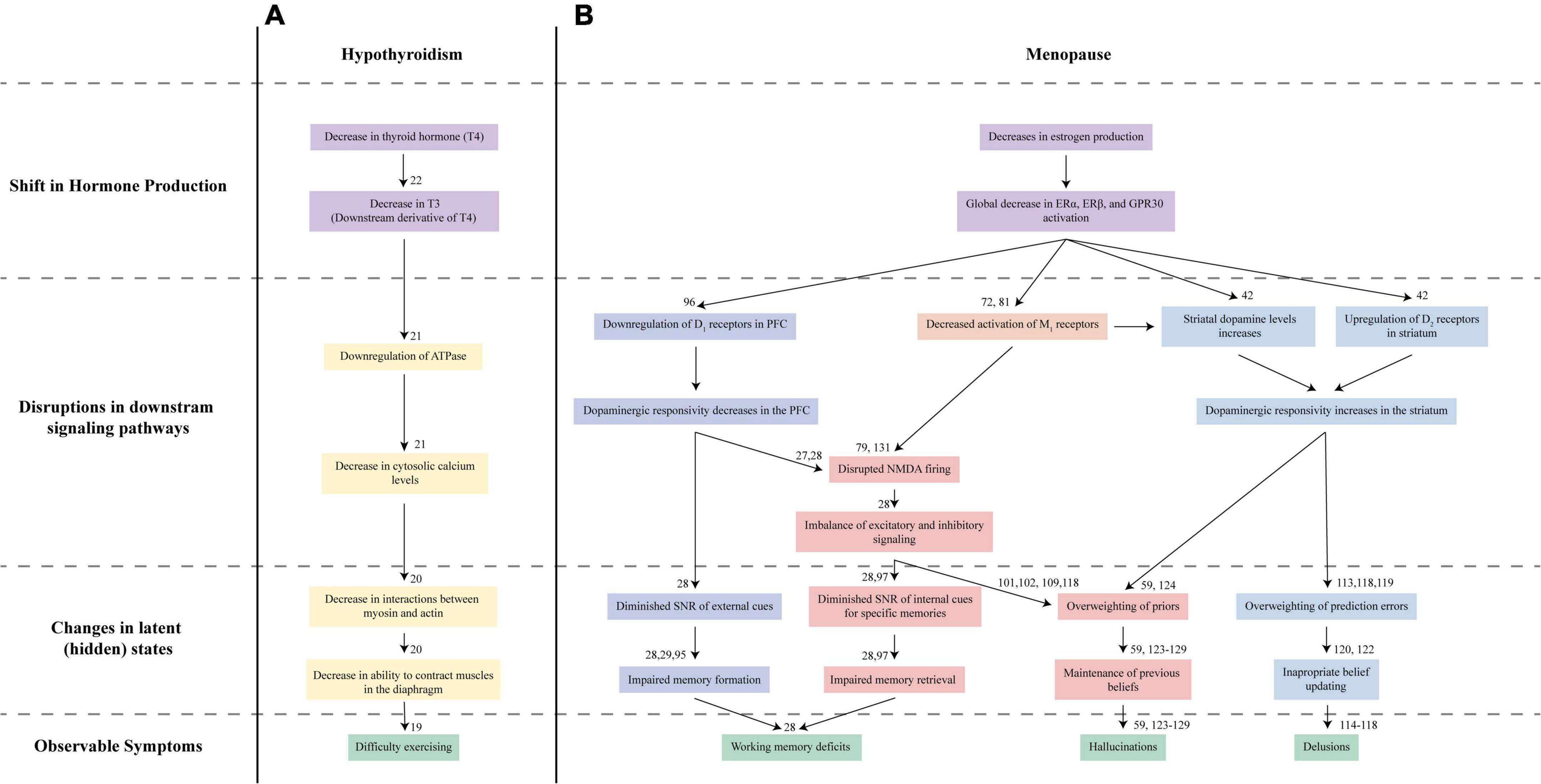
Figure 1. Proposed Nosology of Estrogen-Mediated Psychosis. Understanding hypothyroidism-mediated exercise difficulties (A) and MAP (B) requires looking at multiple levels of changes. (A) In hypothyroidism, the initial decrease in thyroid hormone T4 (purple) and its derivative T3 leads to a series of disruptions in the downstream signaling pathways (yellow). These disruptions ultimately lead to impaired global muscle contractions in the diaphragm resulting in symptoms like irregular breathing and difficulty exercising (green). (B) Our proposed mechanism for MAP reflects this multilevel approach to understanding disorders. Diminished estrogen (purple) signaling in the brain affects bottom-up (blue) and top-down (red/orange) mechanisms that contribute to the latent states driving working memory issues, hallucinations, and delusions (green) characteristic of psychosis. Estrogen decline may disrupt signaling across several pathways: dopaminergic D1 receptor activation in the prefrontal cortex (dark blue), D2 receptor activation in the striatum (light blue), cholinergic M1 activation (orange), and glutamatergic NMDA receptor activation (red). Disruptions to these symptoms, in turn, lead to altered latent states, driving psychosis development.
Placing the action of estrogen and other reproductive hormones within a computational framework may help elucidate how the menopausal transition leads to positive and cognitive symptoms (Figure 1B). Computational psychiatry has been instrumental to uncovering the links between biological mechanisms and behavioral abnormalities observed in psychiatric disorders (23–25) via the identification of latent (unobserved) states driving behavior and instantiated by specific neural circuits (26–29). This feature is a major advantage of the approach, allowing for the generation of falsifiable hypotheses about symptom development and driving iterative refinement of models of psychiatric disease. As applied to our present question, computational models facilitate hypothesis generation about biological mechanisms that may drive latent states linked to psychotic symptom development during the menopause transition.
In this perspective, we aim to critically evaluate the current literature on estrogen and psychosis and how computational frameworks may help to explain psychosis onset in light of modulations in estrogen. We begin with a discussion of how estrogen signaling in the brain influences neurotransmitter signaling abnormalities present in schizophrenia. We then discuss how these systems correspond to elements of computational frameworks accounting for positive and cognitive symptoms that precede and define psychosis. Lastly, we propose future work testing hypotheses directly arising from our framework.
Estrogen and neurotransmitter systems
Estrogen signaling in the brain
Historically, it was thought that estrogen predominantly affected gene expression; however, research has highlighted estrogen has far more diverse effects on a myriad of neurotransmitter systems and functions (30–32). Estrogen interacts with the central nervous system through three primary receptors: ERα (33), ERβ (34, 35), and GPR30 (36). Of particular relevance to schizophrenia is estrogen activity at ERα and ERβ, which are located in the prefrontal cortex (PFC), dorsal striatum, nucleus accumbens, and hippocampus (30). Disrupted signaling in these areas is implicated in cognitive and positive symptoms that characterize psychosis (37–39). In this section, we will explore how modulation by estrogen affects neurotransmitter signaling associated with psychosis.
Dopamine
The dopamine hypothesis posits that positive symptoms of schizophrenia are associated with hyperactive dopaminergic signaling, specifically D2-receptor networks in the striatum. Estrogen receptors are also highly expressed in the striatum (30); however, the role of estrogen in regulating dopamine levels is not clear. Evidence suggests that estrogen increases levels of striatal dopamine in female rats (40) likely through the inhibition of dopamine reuptake proteins (41). Conversely, ovariectomized (i.e., estrogen-free) rats display downregulated expression of dopamine reuptake proteins and increased D2 receptors in the striatum and nucleus accumbens (42), indicating that estrogen would typically result in lower dopamine levels. Typical receptor expression is restored after treatment with 17β-estradiol (42).
The dopamine hypothesis additionally posits that diminished activation of D1 receptors in the PFC contributes to the cognitive deficits commonly seen in psychotic spectrum disorders (43). Cognitive symptoms are the most significant predictors of disease prognosis in schizophrenia (44). Estrogen has a strong preventative effect on cognitive decline, substantially affecting verbal memory (45, 46). Estrogen therapy has even been found to diminish cognitive decline associated with both aging and schizophrenia (47–49) [but see (50–53) for conflicting data]. Importantly, estrogen’s effects on cognition also appear to be dopamine-dependent as studies in women have shown that increases in estrogen (either by natural fluctuation or treatments) predominantly help women with inherently low levels of dopamine (54, 55).
Acetylcholine
Acetylcholine has also been identified as a potential modulator of cognitive and psychotic symptoms. Acetylcholine targets two primary receptors families: muscarinic (mAChRs) (56) and nicotinic (nAChRs) (57). Individuals with schizophrenia display altered muscarinic and nicotinic signaling (58). However, our discussion of acetylcholine and its relation to MAP will be limited to mAChRs, as muscarinic receptor modulation is more predominantly featured in computational models (59, 60), and mAChRs are targets of new antipsychotics (61, 62).
Positive symptoms are associated with reduced activation of mAChRs. Reduced muscarinic activity induces a psychosis-like state and may worsen pre-existing symptoms in schizophrenia (58). The relationship between mAChR and positive symptoms has explicitly been linked with a reduction in M1 and M4 receptors. Post-mortem studies in individuals with schizophrenia demonstrate reduced M1 and M4 receptor density in the hippocampus and striatum (58, 63). M1 and M4 agonists have been shown to reduce positive and negative symptoms in schizophrenia (63–66). Rodent models suggest this relationship may be partly attributed to the inverse correlation between M1 receptor density and striatal dopamine levels (67) and that M4 receptor activation mediates dopaminergic release in the striatum (65, 68).
Estrogen’s protection against positive symptoms may be partially due to its influence on muscarinic receptor expression and activation. Estrogen has been shown to enhance acetylcholine release and decrease uptake in rodents (69, 70) and women (71). Women with surgically induced menopause have lower global M1 and M4 receptor density (72). Estradiol treatments increased global expression of these receptors with significant increases observed in the thalamus, lateral frontal cortex, and notably the hippocampus and left striatum (72). However, these effects run contrary to those seen in rodent models after ovariectomy, after which either down-regulation or no effect on mAChRs in the hippocampus was observed (73–75). While differences may be attributed to the timing of estrogen treatment and age at ovariectomy, more research is needed to clarify the exact relationship between estrogen and acetylcholine.
Estrogen may also protect against cognitive deficits through cholinergic pathways (76). Reduced mAChR activation disrupts memory functioning and attention, and substantial disruption can even shut down cognitive processes entirely (77). The influence of acetylcholine on cognition may be mediated by its modulatory effect on glutamatergic NMDA receptor activation. Reduced activation of NMDA receptors in the hippocampus is associated with cognitive impairment (39, 78). Rodent models suggest that muscarinic receptors colocalize with NMDA and increase the potentiation of NMDA networks (79).
Estrogen influences cognition through muscarinic receptors. Ovariectomized rats display diminished cognitive functioning, which is accompanied by disruptions in typical acetylcholine synthesis and reuptake (80, 81). However, these cognitive deficits and disruptions in acetylcholine maintenance can be improved after estrogen treatments (82). Estrogen has been found to improve cognition after inhibition of M1 receptors (83–88). Mechanisms for the effect of estrogen on cognition may be that ERα promotes neuronal growth and acetylcholine synthesis in the basal forebrain, as seen in mice (89). Rodent models also demonstrate that estrogen improves NMDA potentiation in the hippocampus and overcomes cognitive deficits induced by NMDA antagonists (90).
Computational frameworks
Estrogen affects dopaminergic, cholinergic, and glutamatergic neural signaling implicated in schizophrenia. However, the overlap in these pathways is not sufficient to determine how estrogen loss during menopause may induce MAP. Understanding the mechanisms by which estrogen deficits may cause cognitive and positive symptoms may illustrate how estrogen decline leads to psychosis. Thus, to address this gap, we will explore how a sudden decline in estrogen may affect the neural processes implicated in the generation of cognitive and positive symptoms in schizophrenia. These are also summarized in Figure 1.
Cognition
Cognitive impairments are pervasive in schizophrenia, with measures of cognitive functioning as one of the strongest predictors of psychosis conversion and everyday functioning. In particular, working memory is substantially impaired across phases of illness, from those at high risk of conversion to those with chronic schizophrenia (91). Estrogen has also been shown to improve working memory in post-menopausal women (92). Indicating that psychosis development during menopause may be explained through underlying latent states that drive working memory deficits. Research in computational psychiatry has identified diminished signal-to-noise ratio (SNR) as a primary contributor to working memory deficits (28). We will explore how estrogen decline may impact SNR, which may contribute to working memory deficits in MAP.
Two computational models that formalize the relationship between SNR aberrations and working memory deficits are connectionist and attractor network models. Connectionist models use networks of computational units (representing neurons) to form artificial neural networks (93). Aberrations in neural processing can be introduced to artificial networks by altering the properties of these units. These alterations can mimic abnormalities observed biologically, such as responsiveness to neurotransmitter signals (gain) (28, 93, 94). Studies using in silico connectionist models have found that reducing gain from dopaminergic signaling in the PFC (i.e., lowering SNR) led to cognitive deficits akin to those observed in schizophrenia (28) and decreased maintenance of important contextual information, often associated with working memory deficits (28, 29, 95).
Estrogen modulation of D1 receptor activation in the PFC may increase SNR, preventing working memory decline and, subsequently, psychosis. Jacobs and D’Esposito found that estrogen increased dopaminergic signaling in the PFC when needed for cognitive tasks (54). However, this effect was only helpful in women who had a genetic propensity toward low dopamine levels, indicating that estrogen is important in normalizing dopaminergic signaling specifically in hypodopaminergic states. In addition to direct action at dopamine receptors, estrogen may upregulate dopamine receptor expression. Research in ovariectomized rats found that stimulating ERβ increased D1 receptor expression in the PFC (96). Thus, estrogen decline during the menopause transition may diminish SNR via decreased PFC responsivity to dopamine, spurring working memory deficits that precede psychosis. However, further research is needed to confirm this pathway toward illness.
Evidence from attractor network models is consistent with findings from connectionist models. Attractor networks are a group of neurons that form a stable firing pattern due to excitatory modulation within the network (97). Attractor networks are composed of neural units designed to reflect memory formation and retrieval using attractor models (98, 99). Additionally, low SNR can be introduced to these computational models by decreasing the probability of excitatory (NMDA) neurons firing, which limits the memory retrieval process (28, 100). Research in attractor networks found that D1 stimulation modulated both excitatory NMDA and inhibitory GABA signaling within attractor networks, increasing SNR and improving memory retrieval (27, 28), replicating findings using NMDA antagonists (101, 102). Thus, illustrating that modulation of dopaminergic, glutamatergic, and gabaergic signaling may disrupt working memory leading individuals to a pre-psychotic state.
Estrogen decline during menopause may disrupt excitatory signaling at NMDA receptors needed for memory retrieval, leading to working memory issues. As explored previously, a decrease in estrogen may impair working memory by decreasing the SNR of D1-modulated neural networks in the prefrontal cortex to dopamine, which in turn destabilizes NMDA and GABA firing (28). Another potential route by which estrogen affects working memory is through cholinergic modulation of NMDA receptors. Estrogen has been shown to increase NMDA receptor binding in the hippocampus (103). This may be due to its ability to counteract inhibition of M1 receptors (81), which co-localize with NMDA receptors and induce NMDA firing (79). Estrogen’s effect on the M1-NMDA/GABA network mimics the actions of newly developed antipsychotics, which aim to improve cognition by increasing activation of M1 receptors (104, 105). This illustrates that if a system is reliant on estrogen to maintain functionality within this network, a sudden decline in estrogen may lead to diminished excitatory signals and disruptions in working memory (28).
Positive symptoms
Diminished estrogen signaling in the brain may contribute to the development of positive symptoms, such as hallucinations and delusions. The mechanisms underlying hallucination and delusion formation have been explored extensively through the lens of predictive coding theory. In this Bayesian framework, individuals build and update an internal model of the world using incoming sensory evidence (106–108). Within schizophrenia, disruptions in both model updating and model-based inference are associated with positive symptoms (60, 109).
In predictive coding, internal models are constantly being altered via belief updating to account for changes in the world. Belief updating occurs due to a discrepancy between the expectation based on the internal model (prediction) and the incoming sensory information, termed a prediction error (PE). Not all PEs contribute equally to model updating, which is driven not only by the magnitude of PEs, but the weight (or precision) they are afforded (110–112). However, there is evidence that mechanisms for appropriate weighting of PEs are disrupted in schizophrenia (113). This disruption leads to inappropriate belief updating, which may result in delusions and hallucinations (114–118).
Aberrations in dopaminergic signaling in the striatum contribute to delusions and hallucinations. Dopamine is believed to increase the weighting of reward prediction errors (113, 118, 119): D2 signaling in the striatum strengthens associations between stimuli that reliably predict reward (120, 121). Similarly, hyperdopaminergic signaling in the striatum may lead to increased precision of PEs, promoting inappropriate belief formation (120, 122). Fittingly, overly precise PEs have been tied to increased delusional ideation (60, 116).
Estrogen removal may increase D2 receptor activation leading to inappropriate model updating and delusions. Neural modulations causing sudden increases in D2 receptor activation may induce psychotic states (60, 121). Ovariectomized rats demonstrate estrogen modulated increases in D2 receptor expression and dopamine production (42). A sudden increase in D2 receptors would amplify dopaminergic signaling and increase the precision of PE, shifting internal models toward inappropriate new belief formation. Estrogen deficits may also increase striatal dopamine levels due to diminished acetylcholine signaling, which may be attributed to a reduction in M1 receptors, also observed after ovariectomy (72). However, given conflicting evidence surrounding if estrogen leads to increases or decreases in dopamine production, more research is needed to clarify the exact mechanism by which estrogen decline affects dopaminergic signaling in the striatum.
Hallucination development has also been formulated in light of the predictive processing theory. Hallucinations are thought to arise due to an overweighting of priors relative to incoming sensory evidence (59, 123–129). The processes leading to hallucinations (i.e., overweighting priors) and delusions (i.e., overweighting sensory evidence) may seem contradictory. This seeming contradiction is often resolved through appeals to hierarchy: delusions may represent aberrations at lower level processing, while hallucinations reflect aberrations at higher levels (118). Separation across the processing hierarchy may also be paired with separation over time: PE-mediated belief formation may itself lead to the solidification of inappropriate beliefs and subsequent hallucination formation, at least in a subgroup of individuals with psychosis (130).
Lastly, while there is evidence that dopamine signaling at D2 receptors leads to higher prior precision (59, 124), diminished excitatory (NMDA) signaling may be responsible for disruptions at higher levels of processing (101, 102, 109, 118). Disrupted signaling at NMDA receptors due to menopause may underscore hallucination formation. A sharp decline in M1 receptor activation can disrupt signaling at NMDA receptors (131). While the M1-modulated effects of estrogen on NMDA activation are more closely associated with cognition, these effects may also promote overweighting of prior beliefs.
Taken together, evidence suggests estrogen impacts symptom expression via multiple neurotransmitter systems. While efforts have been made to form a unifying theory underlying psychosis (118), future work should aim to link estrogen levels, neurotransmitter signaling, belief formation and updating, and phenomenology of positive psychotic symptoms. We discuss possible routes toward this future work below.
Discussion and guidance for future directions
In this perspective manuscript, we used computational and biochemical models of cognitive and psychotic symptoms to generate hypotheses about how menopause triggers psychosis. Understanding what biological changes lead to changes in observable behaviors is essential for early intervention and treatment. Identifying risk factors for psychosis has been imperative for the prevention and early treatment of traditionally recognized psychosis. Thus, the next step is to use these computational models to pinpoint what causes psychosis in women during the menopausal transition.
We can best understand the MAP transition by replicating work done on the period leading up to schizophrenia. The most influential research to characterize this period and predict psychosis onset comes from large longitudinal studies (132–136). However, the current studies did not focus on factors that may underlie MAP, such as a decline in reproductive hormones. To fill this gap, studies should track hormonal fluctuations and changes in psychotic symptoms in women as they go through menopause. Despite the insights that may be gained from this line of research, it provides a limited understanding of what elements are driving psychosis, particularly due to the substantial variability in measured hormone levels both within and between women. These limitations necessitate computational models of psychosis that incorporate biological, behavioral, and symptom changes to test hypotheses of the underlying neural mechanisms and latent states driving MAP.
Computational models provide immense benefits due to their capacity to disentangle underlying mechanisms of psychosis. Understanding these mechanisms may guide specific interventions for women going through menopause or even facilitate patient-specific treatments. Computational research on estrogen and MAP has broad implications as well. It may illustrate how estrogen contributes to psychotic episodes in individuals with illnesses associated with hormonal dysregulation (137–139). Additionally, it may illuminate the mechanisms underlying general differences between men and women with schizophrenia (140). Further, computational methods may highlight specific aspects of psychosis that estrogen alone cannot explain, thereby facilitating targeted research into how other hormones, such as androgens (141, 142) and neurosteroids (143), affect psychosis. This proposed multifaceted approach may be crucial to improving our understanding of psychosis and orienting future research.
Data availability statement
The original contributions presented in the study are included in the article/supplementary material, further inquiries can be directed to the corresponding author/s.
Author contributions
VF wrote the first draft of the main manuscript. AP provided edits and guidance on the conceptual framework. LO provided edits and guidance on Hypothyroidism. All authors contributed to the article and approved the submitted version.
Funding
AP was supported by the K23 Career Development Award and R21 from the National Institute of Mental Health (K23 MH115252-01A1 and 5R21MH122940-02), the Career Award for Medical Scientists from the Burroughs-Wellcome Fund, the Carol and Eugene Ludwig Award for Early Career Research, and the Yale Department of Psychiatry and the Yale School of Medicine. LO was supported by the Yale school of Medicine Summer Research Program.
Acknowledgments
We would like to thank laboratory members Lauren Lang, Catalina Mourgues, and Andrew Sheldon for their intellectual contributions to this work. Our discussions were invaluable in bringing these ideas to full fruition.
Conflict of interest
The authors declare that the research was conducted in the absence of any commercial or financial relationships that could be construed as a potential conflict of interest.
Publisher’s note
All claims expressed in this article are solely those of the authors and do not necessarily represent those of their affiliated organizations, or those of the publisher, the editors and the reviewers. Any product that may be evaluated in this article, or claim that may be made by its manufacturer, is not guaranteed or endorsed by the publisher.
References
1. Häfner H, Behrens S, De Vry J, Gattaz WF. Oestradiol enhances the vulnerability threshold for schizophrenia in women by an early effect on dopaminergic neurotransmission. Evidence from an epidemiological study and from animal experiments. Eur Arch Psychiatry Clin Neurosci. (1991) 241:65–8. doi: 10.1007/BF02193758
2. Munk-Jørgensen P. First-admission rates and marital status of schizophrenics. Acta Psychiatr Scand. (1987) 76:210–6. doi: 10.1111/j.1600-0447.1987.tb02886.x
3. McGrath J, Saha S, Welham J, El Saadi O, MacCauley C, Chant D. A systematic review of the incidence of schizophrenia: the distribution of rates and the influence of sex, urbanicity, migrant status and methodology. BMC Med. (2004) 2:13. doi: 10.1186/1741-7015-2-13
4. Aleman A, Kahn RS, Selten JP. Sex differences in the risk of schizophrenia: evidence from meta-analysis. Arch Gen Psychiatry. (2003) 60:565–71. doi: 10.1001/archpsyc.60.6.565
5. Häfner H, an der Heiden W, Behrens S, Gattaz WF, Hambrecht M, Löffler W, et al. Causes and consequences of the gender difference in age at onset of schizophrenia. Schizophr Bull. (1998) 24:99–113. doi: 10.1093/oxfordjournals.schbul.a033317
6. Häfner H. From onset and prodromal stage to a life-long course of schizophrenia and its symptom dimensions: how sex, age, and other risk factors influence incidence and course of illness. Psychiatry J. (2019) 2019:9804836. doi: 10.1155/2019/9804836
7. Bergemann N, Riecher-Rössler A. Estrogen Effects in Psychiatric Disorders. Berlin: Springer Science & Business Media (2005). p. 291. doi: 10.1007/b12686
8. Riecher-Rössler A, Häfner H, Stumbaum M, Maurer K, Schmidt R. Can estradiol modulate schizophrenic symptomatology? Schizophr Bull. (1994) 20:203–14. doi: 10.1093/schbul/20.1.203
9. Reilly TJ, Sagnay de la Bastida VC, Joyce DW, Cullen AE, McGuire P. Exacerbation of psychosis during the perimenstrual phase of the menstrual cycle: systematic review and meta-analysis. Schizophr Bull. (2020) 46:78–90. doi: 10.1093/schbul/sbz030
10. Brzezinski-Sinai NA, Brzezinski A. Schizophrenia and sex hormones: what is the link? Front Psychiatry. (2020) 11:693. doi: 10.3389/fpsyt.2020.00693
11. Akhondzadeh S, Mokhberi K, Amini H, Larijani B, Kashani L, Hashemi L, et al. Is there a relationship between estrogen serum level and symptom severity throughout the menstrual cycle of patients with schizophrenia? Therapy. (2005) 2:745–51. doi: 10.1586/14750708.2.5.745
12. Rubin LH, Carter CS, Drogos L, Pournajafi-Nazarloo H, Sweeney JA, Maki PM. Peripheral oxytocin is associated with reduced symptom severity in schizophrenia. Schizophr Res. (2010) 124:13–21. doi: 10.1016/j.schres.2010.09.014
13. Thompson K, Sergejew A, Kulkarni J. Estrogen affects cognition in women with psychosis. Psychiatry Res. (2000) 94:201–9. doi: 10.1016/S0165-1781(00)00161-X
14. Choi SH, Kang SB, Joe SH. Changes in premenstrual symptoms in women with schizophrenia: a prospective study. Psychosom Med. (2001) 63:822–9. doi: 10.1097/00006842-200109000-00016
15. Jones I, Chandra PS, Dazzan P, Howard LM. Bipolar disorder, affective psychosis, and schizophrenia in pregnancy and the post-partum period. Lancet. (2014) 384:1789–99. doi: 10.1016/S0140-6736(14)61278-2
16. Kulkarni J, Butler S, Riecher-Rössler A. Estrogens and SERMS as adjunctive treatments for schizophrenia. Front Neuroendocrinol. (2019) 53:100743. doi: 10.1016/j.yfrne.2019.03.002
17. Mahé V, Dumaine A. Oestrogen withdrawal associated psychoses. Acta Psychiatr Scand. (2008) 104:323–31. doi: 10.1111/j.1600-0447.2001.00288.x
18. González-Rodríguez A, Seeman MV. The association between hormones and antipsychotic use: a focus on postpartum and menopausal women. Ther Adv Psychopharmacol. (2019) 9:2045125319859973. doi: 10.1177/2045125319859973
19. Sadek SH, Khalifa WA, Azoz AM. Pulmonary consequences of hypothyroidism. Ann Thorac Med. (2017) 12:204–8. doi: 10.4103/atm.ATM_364_16
20. Szent-Györgyi AG. Calcium regulation of muscle contraction. Biophys J. (1975) 15:707–23. doi: 10.1016/S0006-3495(75)85849-8
21. Brent GA. The molecular basis of thyroid hormone action. N Engl J Med. (1994) 331:847–53. doi: 10.1056/NEJM199409293311306
22. Teixeira PFDS, Dos Santos PB, Pazos-Moura CC. The role of thyroid hormone in metabolism and metabolic syndrome. Ther Adv Endocrinol Metab. (2020) 11:2042018820917869. doi: 10.1177/2042018820917869
23. Montague PR, Dolan RJ, Friston KJ, Dayan P. Computational psychiatry. Trends Cogn Sci. (2012) 16:72–80. doi: 10.1016/j.tics.2011.11.018
24. Huys QJM, Maia TV, Frank MJ. Computational psychiatry as a bridge from neuroscience to clinical applications. Nat Neurosci. (2016) 19:404–13. doi: 10.1038/nn.4238
25. Tretter F, Gebicke-Haerter PJ, an der Heiden U, Rujescu D, Mewes HW, Turck CW. Affective disorders as complex dynamic diseases – a perspective from systems biology. Pharmacopsychiatry. (2011) 44:S2–8. doi: 10.1055/s-0031-1275278
26. Lew SE, Tseng KY. Dopamine modulation of GABAergic function enables network stability and input selectivity for sustaining working memory in a computational model of the prefrontal cortex. Neuropsychopharmacology. (2014) 39:3067–76. doi: 10.1038/npp.2014.160
27. Durstewitz D, Seamans JK. The computational role of dopamine D1 receptors in working memory. Neural Netw. (2002) 15:561–72. doi: 10.1016/S0893-6080(02)00049-7
28. Valton V, Romaniuk L, Douglas Steele J, Lawrie S, Seriès P. Comprehensive review: computational modelling of schizophrenia. Neurosci Biobehav Rev. (2017) 83:631–46. doi: 10.1016/j.neubiorev.2017.08.022
29. Cohen JD, Servan-Schreiber D. Context, cortex, and dopamine: a connectionist approach to behavior and biology in schizophrenia. Psychol Rev. (1992) 99:45–77. doi: 10.1037/0033-295X.99.1.45
30. Almey A, Milner TA, Brake WG. Estrogen receptors in the central nervous system and their implication for dopamine-dependent cognition in females. Horm Behav. (2015) 74:125–38. doi: 10.1016/j.yhbeh.2015.06.010
31. Brand BA, de Boer JN, Sommer IEC. Estrogens in schizophrenia: progress, current challenges and opportunities. Curr Opin Psychiatry. (2021) 34:228–37. doi: 10.1097/YCO.0000000000000699
32. Srivastava DP, Waters EM, Mermelstein PG, Kramár EA, Shors TJ, Liu F. Rapid estrogen signaling in the brain: implications for the fine-tuning of neuronal circuitry. J Neurosci. (2011) 31:16056–63. doi: 10.1523/JNEUROSCI.4097-11.2011
33. White R, Lees JA, Needham M, Ham J, Parker M. Structural organization and expression of the mouse estrogen receptor. Mol Endocrinol. (1987) 1:735–44. doi: 10.1210/mend-1-10-735
34. Kuiper GG, Enmark E, Pelto-Huikko M, Nilsson S, Gustafsson JA. Cloning of a novel receptor expressed in rat prostate and ovary. Proc Natl Acad Sci U.S.A. (1996) 93:5925–30. doi: 10.1073/pnas.93.12.5925
35. Tremblay GB, Tremblay A, Copeland NG, Gilbert DJ, Jenkins NA, Labrie F, et al. Cloning, chromosomal localization, and functional analysis of the murine estrogen receptor β. Mol Endocrinol. (1997) 11:353–65. doi: 10.1210/mend.11.3.9902
36. Hammond R, Nelson D, Gibbs RB. GPR30 co-localizes with cholinergic neurons in the basal forebrain and enhances potassium-stimulated acetylcholine release in the hippocampus. Psychoneuroendocrinology. (2011) 36:182–92. doi: 10.1016/j.psyneuen.2010.07.007
37. Mikell CB, McKhann GM, Segal S, McGovern RA, Wallenstein MB, Moore H. The hippocampus and nucleus accumbens as potential therapeutic targets for neurosurgical intervention in schizophrenia. Stereotact Funct Neurosurg. (2009) 87:256–65. doi: 10.1159/000225979
38. Lieberman JA, Girgis RR, Brucato G, Moore H, Provenzano F, Kegeles L, et al. Hippocampal dysfunction in the pathophysiology of schizophrenia: a selective review and hypothesis for early detection and intervention. Mol Psychiatry. (2018) 23:1764–72. doi: 10.1038/mp.2017.249
39. McCutcheon RA, Abi-Dargham A, Howes OD. Schizophrenia, dopamine and the striatum: from biology to symptoms. Trends Neurosci. (2019) 42:205–20. doi: 10.1016/j.tins.2018.12.004
40. McDermott JL, Liu B, Dluzen DE. Sex differences and effects of estrogen on dopamine and DOPAC release from the striatum of male and female CD-1 mice. Exp Neurol. (1994) 125:306–11. doi: 10.1006/exnr.1994.1034
41. Thompson TL, Certain ME. Estrogen mediated inhibition of dopamine transport in the striatum: regulation by G alpha i/o. Eur J Pharmacol. (2005) 511:121–6. doi: 10.1016/j.ejphar.2005.02.005
42. Chavez C, Hollaus M, Scarr E, Pavey G, Gogos A, van den Buuse M. The effect of estrogen on dopamine and serotonin receptor and transporter levels in the brain: an autoradiography study. Brain Res. (2010) 1321:51–9. doi: 10.1016/j.brainres.2009.12.093
43. Bora E, Yücel M, Pantelis C. Cognitive impairment in schizophrenia and affective psychoses: implications for DSM-V criteria and beyond. Schizophr Bull. (2010) 36:36–42. doi: 10.1093/schbul/sbp094
44. Bowie CR, Harvey PD. Cognitive deficits and functional outcome in schizophrenia. Neuropsychiatr Dis Treat. (2006) 2:531–6. doi: 10.2147/nedt.2006.2.4.531
45. Sherwin BB. Estrogen and cognitive aging in women. Neuroscience. (2006) 138:1021–6. doi: 10.1016/j.neuroscience.2005.07.051
46. Sinopoli KJ, Floresco SB, Galea LAM. Systemic and local administration of estradiol into the prefrontal cortex or hippocampus differentially alters working memory. Neurobiol Learn Mem. (2006) 86:293–304. doi: 10.1016/j.nlm.2006.04.003
47. Moradi F, Jahanian Sadatmahalleh S, Ziaei S. The effect of hormone replacement therapy on cognitive function in postmenopausal women: an RCT. Int J Reprod Biomed. (2018) 16:767–74. doi: 10.18502/ijrm.v16i12.3682
48. Ratka A. Menopausal hot flashes and development of cognitive impairment. Ann N Y Acad Sci. (2005) 1052:11–26. doi: 10.1196/annals.1347.002
49. Hogervorst E, Williams J, Budge M, Riedel W, Jolles J. The nature of the effect of female gonadal hormone replacement therapy on cognitive function in post-menopausal women: a meta-analysis. Neuroscience. (2000) 101:485–512. doi: 10.1016/S0306-4522(00)00410-3
50. Etgen T, Bickel H, Förstl H. Metabolic and endocrine factors in mild cognitive impairment. Ageing Res Rev. (2010) 9:280–8. doi: 10.1016/j.arr.2010.01.003
51. Maki PM, Henderson VW. Hormone therapy, dementia, and cognition: the women’s health initiative 10 years on. Climacteric. (2012) 15:256–62. doi: 10.3109/13697137.2012.660613
52. Scali J, Ryan J, Carrière I, Dartigues JF, Tavernier B, Ritchie K, et al. A prospective study of hormone therapy and depression in community-dwelling elderly women: the Three City Study. J Clin Psychiatry. (2010) 71:1673–9. doi: 10.4088/JCP.09m05188blu
53. Grady D, Yaffe K, Kristof M, Lin F, Richards C, Barrett-Connor E. Effect of postmenopausal hormone therapy on cognitive function: the heart and estrogen/progestin replacement study. Am J Med. (2002) 113:543–8. doi: 10.1016/S0002-9343(02)01270-6
54. Jacobs E, D’Esposito M. Estrogen shapes dopamine-dependent cognitive processes: implications for women’s health. J Neurosci. (2011) 31:5286–93. doi: 10.1523/JNEUROSCI.6394-10.2011
55. Hidalgo-Lopez E, Pletzer B. Interactive effects of dopamine baseline levels and cycle phase on executive functions: the role of progesterone. Front Neurosci. (2017) 11:403. doi: 10.3389/fnins.2017.00403
56. Wess J. Novel insights into muscarinic acetylcholine receptor function using gene targeting technology. Trends Pharmacol Sci. (2003) 24:414–20. doi: 10.1016/S0165-6147(03)00195-0
57. Picciotto MR, Caldarone BJ, King SL, Zachariou V. Nicotinic receptors in the brain. Links between molecular biology and behavior. Neuropsychopharmacology. (2000) 22:451–65. doi: 10.1016/S0893-133X(99)00146-3
58. Higley MJ, Picciotto MR. Neuromodulation by acetylcholine: examples from schizophrenia and depression. Curr Opin Neurobiol. (2014) 29:88–95. doi: 10.1016/j.conb.2014.06.004
59. Benrimoh D, Parr T, Vincent P, Adams RA, Friston K. Active inference and auditory hallucinations. Comput Psychiatr. (2018) 2:183–204. doi: 10.1162/CPSY_a_00022
60. Adams RA, Stephan KE, Brown HR, Frith CD, Friston KJ. The computational anatomy of psychosis. Front Psychiatry. (2013) 4:47. doi: 10.3389/fpsyt.2013.00047
61. Shekhar A, Potter WZ, Lightfoot J, Lienemann J, Dubé S, Mallinckrodt C, et al. Selective muscarinic receptor agonist xanomeline as a novel treatment approach for schizophrenia. Am J Psychiatry. (2008) 165:1033–9. doi: 10.1176/appi.ajp.2008.06091591
62. Yohn SE, Conn PJ. Positive allosteric modulation of M1 and M4 muscarinic receptors as potential therapeutic treatments for schizophrenia. Neuropharmacology. (2018) 136(Pt C):438–48. doi: 10.1016/j.neuropharm.2017.09.012
63. Dean B, Scarr E. Muscarinic M1 and M4 receptors: hypothesis driven drug development for schizophrenia. Psychiatry Res. (2020) 288:112989. doi: 10.1016/j.psychres.2020.112989
64. Foster DJ, Choi DL, Conn PJ, Rook JM. Activation of M1 and M4 muscarinic receptors as potential treatments for Alzheimer’s disease and schizophrenia. Neuropsychiatr Dis Treat. (2014) 10:183–91. doi: 10.2147/NDT.S55104
65. Chan WY, McKinzie DL, Bose S, Mitchell SN, Witkin JM, Thompson RC, et al. Allosteric modulation of the muscarinic M4 receptor as an approach to treating schizophrenia. Proc Natl Acad Sci U.S.A. (2008) 105:10978–83. doi: 10.1073/pnas.0800567105
66. Foster DJ, Bryant ZK, Conn PJ. Targeting muscarinic receptors to treat schizophrenia. Behav Brain Res. (2021) 405:113201. doi: 10.1016/j.bbr.2021.113201
67. Gerber DJ, Sotnikova TD, Gainetdinov RR, Huang SY, Caron MG, Tonegawa S. Hyperactivity, elevated dopaminergic transmission, and response to amphetamine in M1 muscarinic acetylcholine receptor-deficient mice. Proc Natl Acad Sci U.S.A. (2001) 98:15312–7. doi: 10.1073/pnas.261583798
68. Tzavara ET, Bymaster FP, Davis RJ, Wade MR, Perry KW, Wess J, et al. M4 muscarinic receptors regulate the dynamics of cholinergic and dopaminergic neurotransmission: relevance to the pathophysiology and treatment of related CNS pathologies. FASEB J. (2004) 18:1410–2. doi: 10.1096/fj.04-1575fje
69. Gibbs RB, Hashash A, Johnson DA. Effects of estrogen on potassium-stimulated acetylcholine release in the hippocampus and overlying cortex of adult rats. Brain Res. (1997) 749:143–6. doi: 10.1016/S0006-8993(96)01375-3
70. O’Malley CA, Hautamaki RD, Kelley M, Meyer EM. Effects of ovariectomy and estradiol benzoate on high affinity choline uptake, ACh synthesis, and release from rat cerebral cortical synaptosomes. Brain Res. (1987) 403:389–92. doi: 10.1016/0006-8993(87)90082-5
71. Gibbs RB. Effects of estrogen on basal forebrain cholinergic neurons and cognition: implications for brain aging and dementia in women. In: M Morrison editor. Hormones, Aging, and Mental Disorders. Cambridge: Cambridge University Press (2000). p. 183–222. doi: 10.1017/CBO9780511544071.010
72. Norbury R, Travis MJ, Erlandsson K, Waddington W, Ell PJ, Murphy DGM. Estrogen therapy and brain muscarinic receptor density in healthy females: a SPET study. Horm Behav. (2007) 51:249–57. doi: 10.1016/j.yhbeh.2006.10.007
73. Cardoso CC, Ricardo VP, Frussa-Filho R, Porto CS, Abdalla FMF. Effects of 17β-estradiol on expression of muscarinic acetylcholine receptor subtypes and estrogen receptor α in rat hippocampus. Eur J Pharmacol. (2010) 634:192–200. doi: 10.1016/j.ejphar.2010.02.032
74. Cardoso CC, Pereira RTS, Koyama CA, Porto CS, Abdalla FMF. Effects of estrogen on muscarinic acetylcholine receptors in the rat hippocampus. Neuroendocrinology. (2004) 80:379–86. doi: 10.1159/000084202
75. dos Santos Pereira RT, Porto CS, Godinho RO, Abdalla FMF. Effects of estrogen on intracellular signaling pathways linked to activation of muscarinic acetylcholine receptors and on acetylcholinesterase activity in rat hippocampus. Biochem Pharmacol. (2008) 75:1827–34. doi: 10.1016/j.bcp.2008.01.016
76. Zhao LX, Ge YH, Xiong CH, Tang L, Yan YH, Law PY, et al. M1 muscarinic receptor facilitates cognitive function by interplay with AMPA receptor GluA1 subunit. FASEB J. (2018) 32:4247–57. doi: 10.1096/fj.201800029R
77. Newman EL, Gupta K, Climer JR, Monaghan CK, Hasselmo ME. Cholinergic modulation of cognitive processing: insights drawn from computational models. Front Behav Neurosci. (2012) 6:24. doi: 10.3389/fnbeh.2012.00024
78. Melancon BJ, Tarr JC, Panarese JD, Wood MR, Lindsley CW. Allosteric modulation of the M1 muscarinic acetylcholine receptor: improving cognition and a potential treatment for schizophrenia and Alzheimer’s disease. Drug Discov Today. (2013) 18:1185–99. doi: 10.1016/j.drudis.2013.09.005
79. Marino MJ, Rouse ST, Levey AI, Potter LT, Conn PJ. Activation of the genetically defined m1 muscarinic receptor potentiates N-methyl-D-aspartate (n.d.) receptor currents in hippocampal pyramidal cells. Proc Natl Acad Sci U.S.A. (1998) 95:11465–70. doi: 10.1073/pnas.95.19.11465
80. Singh M, Meyer EM, Millard WJ, Simpkins JW. Ovarian steroid deprivation results in a reversible learning impairment and compromised cholinergic function in female Sprague-Dawley rats. Brain Res. (1994) 644:305–12. doi: 10.1016/0006-8993(94)91694-2
81. Newhouse P, Dumas J. Estrogen–cholinergic interactions: implications for cognitive aging. Horm Behav. (2015) 74:173–85. doi: 10.1016/j.yhbeh.2015.06.022
82. Yamamoto H, Kitawaki J, Kikuchi N, Okubo T, Iwasa K, Kawata M, et al. Effects of estrogens on cholinergic neurons in the rat basal nucleus. J Steroid Biochem Mol Biol. (2007) 107:70–9. doi: 10.1016/j.jsbmb.2007.03.035
83. Tanabe F, Miyasaka N, Kubota T, Aso T. Estrogen and progesterone improve scopolamine-induced impairment of spatial memory. J Med Dent Sci. (2004) 51:89–98.
84. Dohanich GP, Fader AJ, Javorsky DJ. Estrogen and estrogen-progesterone treatments counteract the effect of scopolamine on reinforced T-maze alternation in female rats. Behav Neurosci. (1994) 108:988–92. doi: 10.1037/0735-7044.108.5.988
85. Fader AJ, Johnson PEM, Dohanich GP. Estrogen improves working but not reference memory and prevents amnestic effects of scopolamine on a radial-arm maze. Pharmacol Biochem Behav. (1999) 62:711–7. doi: 10.1016/S0091-3057(98)00219-6
86. Gibbs RB. Estrogen replacement enhances acquisition of a spatial memory task and reduces deficits associated with hippocampal muscarinic receptor inhibition. Horm Behav. (1999) 36:222–33. doi: 10.1006/hbeh.1999.1541
87. Markowska AL, Savonenko AV. Effectiveness of estrogen replacement in restoration of cognitive function after long-term estrogen withdrawal in aging rats. J Neurosci. (2002) 22:10985–95. doi: 10.1523/JNEUROSCI.22-24-10985.2002
88. Dumas J, Hancur-Bucci C, Naylor M, Sites C, Newhouse P. Estrogen treatment effects on anticholinergic-induced cognitive dysfunction in normal postmenopausal women. Neuropsychopharmacology. (2006) 31:2065–78. doi: 10.1038/sj.npp.1301042
89. Szegő ÉM, Barabás K, Balog J, Szilágyi N, Korach KS, Juhász G, et al. Estrogen induces estrogen receptor α-dependent cAMP response element-binding protein phosphorylation via mitogen activated protein kinase pathway in basal forebrain cholinergic neurons in vivo. J Neurosci. (2006) 26:4104–10. doi: 10.1523/JNEUROSCI.0222-06.2006
90. Gureviciene I, Puoliväli J, Pussinen R, Wang J, Tanila H, Ylinen A. Estrogen treatment alleviates NMDA-antagonist induced hippocampal LTP blockade and cognitive deficits in ovariectomized mice. Neurobiol Learn Mem. (2003) 79:72–80. doi: 10.1016/S1074-7427(02)00012-6
91. Dauvermann MR, Whalley HC, Schmidt A, Lee GL, Romaniuk L, Roberts N, et al. Computational neuropsychiatry – schizophrenia as a cognitive brain network disorder. Front Psychiatry. (2014) 5:30. doi: 10.3389/fpsyt.2014.00030
92. Duff SJ, Hampson E. A beneficial effect of estrogen on working memory in postmenopausal women taking hormone replacement therapy. Horm Behav. (2000) 38:262–76. doi: 10.1006/hbeh.2000.1625
93. Aakerlund L, Hemmingsen R. Neural networks as models of psychopathology. Biol Psychiatry. (1998) 43:471–82. doi: 10.1016/S0006-3223(97)00489-7
94. Freeman WJ. Nonlinear gain mediating cortical stimulus-response relations. Biol Cybern. (1979) 33:237–47. doi: 10.1007/BF00337412
95. Cohen JD, Servan-Schreiber D. A theory of dopamine function and its role in cognitive deficits in schizophrenia. Schizophr Bull. (1993) 19:85–104. doi: 10.1093/schbul/19.1.85
96. Sárvári M, Deli L, Kocsis P, Márk L, Maász G, Hrabovszky E, et al. Estradiol and isotype-selective estrogen receptor agonists modulate the mesocortical dopaminergic system in gonadectomized female rats. Brain Res. (2014) 1583:1–11. doi: 10.1016/j.brainres.2014.06.020
97. Rolls ET. Attractor networks. Wiley Interdiscip Rev Cogn Sci. (2010) 1:119–34. doi: 10.1002/wcs.1
98. Hopfield JJ. Neural networks and physical systems with emergent collective computational abilities. Proc Natl Acad Sci U.S.A. (1982) 79:2554–8. doi: 10.1073/pnas.79.8.2554
99. Hopfield JJ. Neurons with graded response have collective computational properties like those of two-state neurons. Proc Natl Acad Sci U.S.A. (1984) 81:3088–92. doi: 10.1073/pnas.81.10.3088
100. Rolls ET, Loh M, Deco G, Winterer G. Computational models of schizophrenia and dopamine modulation in the prefrontal cortex. Nat Rev Neurosci. (2008) 9:696–709. doi: 10.1038/nrn2462
101. Murray JD, Anticevic A, Gancsos M, Ichinose M, Corlett PR, Krystal JH, et al. Linking microcircuit dysfunction to cognitive impairment: effects of disinhibition associated with schizophrenia in a cortical working memory model. Cereb Cortex. (2014) 24:859–72. doi: 10.1093/cercor/bhs370
102. Anticevic A, Gancsos M, Murray JD, Repovs G, Driesen NR, Ennis DJ, et al. NMDA receptor function in large-scale anticorrelated neural systems with implications for cognition and schizophrenia. Proc Natl Acad Sci U.S.A. (2012) 109:16720–5. doi: 10.1073/pnas.1208494109
103. Woolley CS, Weiland NG, McEwen BS, Schwartzkroin PA. Estradiol increases the sensitivity of hippocampal CA1 pyramidal cells to NMDA receptor-mediated synaptic input: correlation with dendritic spine density. J Neurosci. (1997) 17:1848–59. doi: 10.1523/JNEUROSCI.17-05-01848.1997
104. Bakker G, Vingerhoets C, Boucherie D, Caan M, Bloemen O, Eersels J, et al. Relationship between muscarinic M1 receptor binding and cognition in medication-free subjects with psychosis. Neuroimage Clin. (2018) 18:713–9. doi: 10.1016/j.nicl.2018.02.030
105. Sellin AK, Shad M, Tamminga C. Muscarinic agonists for the treatment of cognition in schizophrenia. CNS Spectr. (2008) 13:985–96. doi: 10.1017/S1092852900014048
106. Spratling MW. A review of predictive coding algorithms. Brain Cogn. (2017) 112:92–7. doi: 10.1016/j.bandc.2015.11.003
107. Rao RP, Ballard DH. Predictive coding in the visual cortex: a functional interpretation of some extra-classical receptive-field effects. Nat Neurosci. (1999) 2:79–87. doi: 10.1038/4580
108. Friston K. A theory of cortical responses. Philos Trans R Soc Lond B Biol Sci. (2005) 360:815–36. doi: 10.1098/rstb.2005.1622
109. Corlett PR, Horga G, Fletcher PC, Alderson-Day B, Schmack K, Powers AR III. Hallucinations and strong priors. Trends Cogn Sci. (2019) 23:114–27. doi: 10.1016/j.tics.2018.12.001
110. Mathys C, Daunizeau J, Friston KJ, Stephan KE. A Bayesian foundation for individual learning under uncertainty. Front Hum Neurosci. (2011) 5:39. doi: 10.3389/fnhum.2011.00039
111. Friston K. The free-energy principle: a rough guide to the brain? Trends Cogn Sci. (2009) 13:293–301. doi: 10.1016/j.tics.2009.04.005
112. Bastos AM, Usrey WM, Adams RA, Mangun GR, Fries P, Friston KJ. Canonical microcircuits for predictive coding. Neuron. (2012) 76:695–711. doi: 10.1016/j.neuron.2012.10.038
113. Haarsma J, Fletcher PC, Griffin JD, Taverne HJ, Ziauddeen H, Spencer TJ, et al. Precision weighting of cortical unsigned prediction error signals benefits learning, is mediated by dopamine, and is impaired in psychosis. Mol Psychiatry. (2021) 26:5320–33. doi: 10.1038/s41380-020-0803-8
114. Erdmann T, Mathys C. A generative framework for the study of delusions. Schizophr Res. (2021). 245:42–49. doi: 10.1016/j.schres.2020.11.048
115. Fletcher PC, Frith CD. Perceiving is believing: a Bayesian approach to explaining the positive symptoms of schizophrenia. Nat Rev Neurosci. (2009) 10:48–58. doi: 10.1038/nrn2536
116. Corlett PR, Taylor JR, Wang XJ, Fletcher PC, Krystal JH. Toward a neurobiology of delusions. Prog Neurobiol. (2010) 92:345–69. doi: 10.1016/j.pneurobio.2010.06.007
117. Corlett PR, Honey GD, Fletcher PC. Prediction error, ketamine and psychosis: an updated model. J Psychopharmacol. (2016) 30:1145–55. doi: 10.1177/0269881116650087
118. Sterzer P, Adams RA, Fletcher P, Frith C, Lawrie SM, Muckli L, et al. The predictive coding account of psychosis. Biol Psychiatry. (2018) 84:634–43. doi: 10.1016/j.biopsych.2018.05.015
119. Schultz W. Dopamine reward prediction error coding. Dialogues Clin Neurosci. (2016) 18:23–32. doi: 10.31887/DCNS.2016.18.1/wschultz
120. Kapur S, Seeman P. Ketamine has equal affinity for NMDA receptors and the high-affinity state of the dopamine D2 receptor. Biol Psychiatry. (2001) 49:954–7. doi: 10.1016/S0006-3223(01)01110-6
121. Howes OD, Kapur S. The dopamine hypothesis of schizophrenia: version III–the final common pathway. Schizophr Bull. (2009) 35:549–62. doi: 10.1093/schbul/sbp006
122. Corlett PR, Frith CD, Fletcher PC. From drugs to deprivation: a Bayesian framework for understanding models of psychosis. Psychopharmacology. (2009) 206:515–30. doi: 10.1007/s00213-009-1561-0
123. Adams RA, Vincent P, Benrimoh D, Friston KJ, Parr T. Everything is connected: inference and attractors in delusions. Schizophr Res. (2021) 245:5–22. doi: 10.1016/j.schres.2021.07.032
124. Benrimoh D, Parr T, Adams RA, Friston K. Hallucinations both in and out of context: an active inference account. PLoS One. (2019) 14:e0212379. doi: 10.1371/journal.pone.0212379
125. Vercammen A, Aleman A. Semantic expectations can induce false perceptions in hallucination-prone individuals. Schizophr Bull. (2010) 36:151–6. doi: 10.1093/schbul/sbn063
126. Powers AR, Mathys C, Corlett PR. Pavlovian conditioning–induced hallucinations result from overweighting of perceptual priors. Science. (2017) 357:596–600. doi: 10.1126/science.aan3458
127. Teufel C, Subramaniam N, Dobler V, Perez J, Finnemann J, Mehta PR, et al. Shift toward prior knowledge confers a perceptual advantage in early psychosis and psychosis-prone healthy individuals. Proc Natl Acad Sci U.S.A. (2015) 112:13401–6. doi: 10.1073/pnas.1503916112
128. Kafadar E, Fisher V, Quagan B, Hammer A, Jaeger H, Mourgues C, et al. Conditioned hallucinations and prior over-weighting are state-sensitive markers of hallucination susceptibility. Biol Psychiatry. (2022). doi: 10.1016/j.biopsych.2022.05.007 [Epub ahead of print].
129. Kafadar E, Mittal VA, Strauss GP, Chapman HC, Ellman LM, Bansal S, et al. Modeling perception and behavior in individuals at clinical high risk for psychosis: support for the predictive processing framework. Schizophr Res. (2020) 226:167–75. doi: 10.1016/j.schres.2020.04.017
130. Sheldon AD, Kafadar E, Fisher V, Greenwald MS, Aitken F, Negreira AM, et al. Perceptual pathways to hallucinogenesis. Schizophr Res. (2022) 245:77–89. doi: 10.1016/j.schres.2022.02.002
131. Galvin VC, Yang S, Lowet AS, Datta D, Duque A, Arnsten AF, et al. M1 receptors interacting with NMDAR enhance delay-related neuronal firing and improve working memory performance. Curr Res Neurobiol. (2021) 2:100016. doi: 10.1016/j.crneur.2021.100016
132. Hafner H, Maurer K, an der Heiden W. ABC schizophrenia study: an overview of results since 1996. Soc Psychiatry Psychiatr Epidemiol. (2013) 48:1021–31. doi: 10.1007/s00127-013-0700-4
133. Hafner H, Maurer K, Loffler W, an der Heiden W, Munk-Jorgensen P, Hambrecht M, et al. The ABC schizophrenia study: a preliminary overview of the results. Soc Psychiatry Psychiatr Epidemiol. (1998) 33:380–6. doi: 10.1007/s001270050069
134. Addington J, Cadenhead KS, Cannon TD, Cornblatt B, McGlashan TH, Perkins DO, et al. North American prodrome longitudinal study: a collaborative multisite approach to prodromal schizophrenia research. Schizophr Bull. (2007) 33:665–72. doi: 10.1093/schbul/sbl075
135. Addington J, Cadenhead KS, Cornblatt BA, Mathalon DH, McGlashan TH, Perkins DO, et al. North American prodrome longitudinal study (NAPLS 2): overview and recruitment. Schizophr Res. (2012) 142:77–82. doi: 10.1016/j.schres.2012.09.012
136. Addington J, Liu L, Brummitt K, Bearden CE, Cadenhead KS, Cornblatt BA, et al. North American prodrome longitudinal study (NAPLS 3): methods and baseline description. Schizophr Res. (2020) 33:665–72.
137. Doretto L, Mari FC, Chaves AC. Polycystic ovary syndrome and psychotic disorder. Front Psychiatry. (2020) 11:543. doi: 10.3389/fpsyt.2020.00543
138. Jung SY, Park JW, Kim DH, Jun YH, Lee JS, Lee JE. Mosaic Turner syndrome associated with schizophrenia. Ann Pediatr Endocrinol Metab. (2014) 19:42–4. doi: 10.6065/apem.2014.19.1.42
139. Catinari S, Vass A, Heresco-Levy U. Psychiatric manifestations in turner syndrome: a brief survey. Isr J Psychiatry Relat Sci. (2006) 43:293–5.
140. Zhang B, Han M, Tan S, De Yang F, Tan Y, Jiang S, et al. Gender differences measured by the MATRICS consensus cognitive battery in chronic schizophrenia patients. Sci Rep. (2017) 7:11821. doi: 10.1038/s41598-017-12027-w
141. Du X, McCarthny CR, Notaras M, van den Buuse M, Hill RA. Effect of adolescent androgen manipulation on psychosis-like behaviour in adulthood in BDNF heterozygous and control mice. Horm Behav. (2019) 112:32–41. doi: 10.1016/j.yhbeh.2019.03.005
142. Howard JM. Testosterone and psychosis. Br J Psychiatry. (2004) 185:173. doi: 10.1192/bjp.185.2.173
Keywords: menopause, psychosis, computational psychiatry, predictive coding, estrogen
Citation: Fisher VL, Ortiz LS and Powers AR III (2022) A computational lens on menopause-associated psychosis. Front. Psychiatry 13:906796. doi: 10.3389/fpsyt.2022.906796
Received: 29 March 2022; Accepted: 07 July 2022;
Published: 03 August 2022.
Edited by:
Qiyong Gong, Sichuan University, ChinaReviewed by:
Mary V. Seeman, University of Toronto, CanadaSarah Sullivan, University of Bristol, United Kingdom
Copyright © 2022 Fisher, Ortiz and Powers. This is an open-access article distributed under the terms of the Creative Commons Attribution License (CC BY). The use, distribution or reproduction in other forums is permitted, provided the original author(s) and the copyright owner(s) are credited and that the original publication in this journal is cited, in accordance with accepted academic practice. No use, distribution or reproduction is permitted which does not comply with these terms.
*Correspondence: Albert R. Powers III, albert.powers@yale.edu