- 1Neuroscience Interdepartmental Program, University of California Los Angeles, Los Angeles, CA, United States
- 2Department of Psychology, Harvard Medical School, Boston, MA, United States
- 3Department of Psychiatry and Biobehavioral Sciences, University of California Los Angeles, Los Angeles, CA, United States
- 4Jane and Terry Semel Institute for Neuroscience and Human Behavior, University of California Los Angeles, Los Angeles, CA, United States
- 5Department of Psychology and Neuroscience, The University of North Carolina at Chapel Hill, Chapel Hill, NC, United States
The cerebellum has been consistently shown to be atypical in autism spectrum disorder (ASD). However, despite its known role in sensorimotor function, there is limited research on its association with sensory over-responsivity (SOR), a common and impairing feature of ASD. Thus, this study sought to examine functional connectivity of the sensorimotor cerebellum in ASD compared to typically developing (TD) youth and investigate whether cerebellar connectivity is associated with SOR. Resting-state functional connectivity of the sensorimotor cerebellum was examined in 54 ASD and 43 TD youth aged 8-18 years. Using a seed-based approach, connectivity of each sensorimotor cerebellar region (defined as lobules I-IV, V-VI and VIIIA&B) with the whole brain was examined in ASD compared to TD youth, and correlated with parent-reported SOR severity. Across all participants, the sensorimotor cerebellum was functionally connected with sensorimotor and visual regions, though the three seed regions showed distinct connectivity with limbic and higher-order sensory regions. ASD youth showed differences in connectivity including atypical connectivity within the cerebellum and increased connectivity with hippocampus and thalamus compared to TD youth. More severe SOR was associated with stronger connectivity with cortical regions involved in sensory and motor processes and weaker connectivity with cognitive and socio-emotional regions, particularly prefrontal cortex. These results suggest that atypical cerebellum function in ASD may play a role in sensory challenges in autism.
1 Introduction
Autism spectrum disorder (ASD) is a neurodevelopmental disorder characterized by socio-emotional and communicative difficulties, repetitive behaviors, and altered sensory processing (1). While the neurobiology underlying the etiology of ASD is quite complex, reflecting the considerable heterogeneity that characterizes this disorder, emerging research has consistently implicated cerebellar atypicalities in ASD (2–7). The cerebellum shows differences in structure, such as reduced Purkinje cell size and numbers, as well as function, such as atypical activation in the anterior cerebellum during motor tasks, in ASD (8–14). However, how these cerebellar atypicalities contribute to ASD features remains unclear. In particular, despite the recognized role of the cerebellum in sensorimotor processing (15), there are almost no studies that investigate the relationship between cerebellar function and sensory processing difficulties in ASD, which are known to be both extremely prevalent and a barrier to quality of life (16, 17).
More than 90% of children with ASD experience sensory processing atypicalities, and in particular at least 56-70% of youth with ASD experience sensory over-responsivity (SOR; 16, 18–20). SOR, characterized by an extreme discomfort in response to sensory stimulation, can be particularly impairing for children with ASD and has been previously linked to challenges with emotion regulation, communication, and daily-life adaptive skills (17, 21–23). Functional magnetic resonance imaging (fMRI) studies in the past decade have shown that SOR is associated with greater neural sensitivity and reduced neural habituation in sensory-limbic regions, such as the amygdala and primary sensory cortices during sensory stimulation (24, 25). Similar to other complex features of ASD, SOR involves multiple neural networks, including altered patterns in fronto-amygdala (24, 25), thalamus (26), and salience network connectivity (27, 28). Thus far, cerebellar circuits have not been investigated as a contributing factor to SOR in ASD. However, given the known role of the cerebellum in both sensorimotor processing and in ASD, the lack of cerebellum research in relation to SOR leaves a significant gap in our understanding of the neural mechanisms underlying this impairing feature of ASD.
While no studies to date have specifically investigated the link between the cerebellum and SOR, recent research has found evidence for atypical function of the cerebellum in sensorimotor processing in ASD. Specifically, a resting-state functional connectivity (rsFC) study has shown that the cerebellum is functionally more connected with cortical sensorimotor networks in youth with autism, suggesting a role for the cerebellum in atypical sensorimotor processing in ASD (11). Additionally, altered cerebellar functional connectivity was recently linked to broad sensory processing issues, as well as to socio-communicative signs of autism and restricted interests (29). Oldehinkel et al. (29) found that elevated connectivity between the cerebellum and somatosensory and motor cortices in ASD was associated with more severe sensory symptoms, further suggesting that atypicalities in the sensorimotor cerebellum may contribute to altered sensory processing in ASD. However, this particular study did not distinguish between different types of sensory processing symptoms in ASD, which vary widely and can, for example, include sensory under-responsivity and atypical sensation seeking in addition to SOR (30). Thus, the contribution of the cerebellum to specific sensory symptoms, particularly SOR (given its impairing nature) requires further study.
In the current study, we investigated differences in rsFC of the sensorimotor cerebellum in children and adolescents with ASD compared to typically developing peers (TD), as well as associations between cerebellar functional connectivity and SOR symptoms in ASD. We focused on the regions of the cerebellum that have previously been shown to play a key role in sensorimotor processing, namely lobules I-VI, VIIIA and VIIIB (9, 11, 15, 31–35). The anterior lobe of the cerebellum (lobules I-V and parts of VI) and lobules VIIIA and VIIIB are functionally connected with sensorimotor cortical regions (11, 36, 37) and house sensorimotor homonculi (15) – body maps analogous to that on the cerebral cortex. Within the anterior lobe, lobules V and VI have been reported to be particularly functionally connected with visual and auditory cortices (34, 36); accordingly, we distinguished lobules V-VI from lobules I-IV in our study. We also differentiated lobule VIII (i.e., lobules VIIIA and VIIIB) from lobules I-VI due to anatomical distance (i.e., anterior versus posterior cerebellum; 9). For each of the three sensorimotor cerebellar seed regions (lobules I-IV, V-VI, and VIII), we aimed to examine the differences in connectivity in ASD compared to TD youth, and the association between connectivity and SOR severity within youth with ASD.
2 Materials and methods
2.1 Participants
Participants were 54 ASD (16F) and 43 TD (10F) children and adolescents, aged 8.3 - 18.0 years. Written informed consent was obtained from all parents and from children who were 13 years or older; written assent was given by participants who were younger than 13 years. ASD diagnosis was confirmed with the Autism Diagnostic Interview-Revised (ADI-R; 38), Autism Diagnostic Observation Schedule - second edition (ADOS-2; 39), and best clinical judgment. Groups did not differ significantly in age, sex, ethnicity, race, or head motion (see Table 1). IQ was assessed using the Wechsler Abbreviated Scales of Intelligence (WASI; 40), and participants had a full-scale IQ of 75 or above. ASD participants had significantly lower full-scale IQ than TD participants (see Table 1), and thus, IQ was entered as a covariate in between-group analyses. Data were initially collected for 56 ASD and 47 TD participants. Participants with maximum absolute head motion (i.e., maximum head motion with respect to the reference volume) greater than 4mm and who were significant motion outliers for both maximum and mean absolute motion (i.e., mean head motion with respect to the reference volume) were excluded from our analysis (2 ASD and 1 TD). 3 TD participants were additionally excluded due to brain anomalies (2 TD) and elevated SOR severity (1 TD). All study procedures were approved by the University of California, Los Angeles, Institutional Review Board.
2.2 Measures
Sensory Processing 3-Dimensions Scale (SP3D) Inventory (41) was used to assess SOR where parents indicated sensory experiences listed on the SP3D checklist that bother their child. The number of items (auditory, tactile or visual) were summed to calculate a total SOR score as in previous research (e.g. 42, 43), with a higher score denoting more severe SOR. Anxiety was measured using parent report on the Screen for Child Anxiety Related Emotional Disorder (i.e., SCARED) questionnaire (44) and included as a covariate in SOR analyses due to the high correlation between anxiety and SOR symptoms (e.g., 45).
2.3 MRI data acquisition
fMRI data were acquired on a Siemens Prisma 3-Tesla scanner with a 64-channel head coil. During the resting-state scan, participants fixed their gaze on a white crosshair on a black background, displayed using a pair of 800x640 resolution magnet-compatible 3D goggles under computer control (Resonance Technologies, Inc.). The resting-state scan was the first functional scan completed as part of a larger protocol. Scans were acquired using an EPI multiband acquisition lasting 8 minutes and covering the entire cerebral volume (TR=720ms, FOV=208 mm, TE=37ms, flip angle=52°, in-plane voxel size=2mm², 72 slices, multi-band acceleration factor=8).
2.4 Data preprocessing and analysis
2.4.1 Behavioral data analysis
Group differences in SOR and anxiety were investigated with independent-samples t-tests using R Version 4.1.2. Because SOR is commonly reported as being correlated with anxiety (e.g., 45), we also examined the association between SOR and anxiety in the current study sample.
2.4.2 Neuroimaging data analysis
The fMRI data were analyzed using the FMRIB Software Library (FSL)1, Version 5.0.11. Preprocessing steps included spatial smoothing (Gaussian kernel full width at half maximum=5mm) and the regression of mean white matter, cerebrospinal fluid, and global signal times series. To remove potential confounds resulting from head motion, Independent Component Analysis - Automatic Removal of Motion Artifacts (ICA-AROMA) was used, and single-subject components identified as motion or noise were regressed out (46, 47). Single-subject functional data were then registered to the MNI152 T1 2-mm template brain (12 degrees of freedom) using the registration matrix estimated prior to spatial smoothing.
We used seed-based connectivity analyses to examine functional connectivity between three regions of the sensorimotor cerebellum and the whole brain. Lobules I-IV, lobules V-VI and lobule VIII (including VIIIA and VIIIB) were defined as the three seed regions due to their association with sensorimotor processing (e.g., 9, 11, 15, 34). Cerebellar seeds were created with the Spatially Unbiased Infra-tentorial Template (SUIT; 48) probabilistic cerebellar atlas and thresholded at 75%, keeping only voxels with at least 0.75 probability of belonging to a certain lobule (i.e., belonging to a certain lobule in at least 75% of individuals included in the probabilistic atlas; Supplementary Figure 1). The 75% threshold was chosen for most complete lobule representation with the least overlap among lobules.
FSL’s fMRI Expert Analysis Tool (FEAT, version 6.00) was utilized to run a fixed-effects model for each subject, and FSL’s Local Analysis of Mixed Effects State (FLAME 1 + 2; 49–51) was used for higher-level group analyses. For each cerebellar seed, the time-series from the cerebellar region were isolated in individual subject space and correlated with neural activity in every other voxel in the brain to create single-subject connectivity maps. Prior to performing group level analyses, z-statistic maps were generated using Fischer’s r-to-z transformation. All whole-brain contrasts were corrected for multiple comparisons using Gaussian random-field theory (i.e., a type of Family-Wise Error (FWE) rate correction) in FSL with a voxel-wise threshold of z>3.1 (within-group contrasts) or z>2.3 (between-group contrasts and correlations with SOR) and a cluster-corrected threshold of p<0.05. Supplemental between-group and SOR correlation analyses were conducted at a more stringent voxel-wise threshold of z>2.7 and included in the Supplementary Information (Supplementary Figures 2 and 3).
To assess SOR correlations with cerebellar rsFC in ASD, SOR was entered as a bottom-up regressor in each whole-brain analysis (one for each of the three cerebellar seed regions). Anxiety severity was included as a covariate in these analyses to examine the unique effect of SOR over and above anxiety, due to previously reported high correlations between SOR and anxiety symptoms (e.g., 45, 52). Parameter estimates indexing connectivity strength were extracted from each cluster showing a significant correlation with SOR and plotted to identify potential outliers. Supplemental analyses were conducted to assess the effect of anxiety on cerebellar connectivity over and above the effect of SOR severity (see Supplementary Information).
All analyses (i.e., within-group, between-group and SOR correlations) were repeated with age as a covariate of no interest to ensure that our findings were not due to age variability in the sample (see Supplementary Information).
3 Results
3.1 Behavioral measures
To examine group differences in SOR and anxiety, we performed independent-samples t-tests. The ASD group had significantly higher SOR [t (59.17)=7.92, p<0.001, 95% confidence interval= (6.19, 10.38); Table 1) and anxiety symptoms [t (84.82)=6.12, p<0.001, 95% confidence interval= (7.94, 15.57)] compared to the TD group. SOR was positively correlated with anxiety in the ASD sample (r=0.32, p=0.02), but not in the TD sample (r=0.06, p=0.71).
3.2 Cerebellar connectivity
Within-group analyses. Across lobules, the sensorimotor cerebellum showed functional connectivity with sensorimotor and visual regions, although the specific regions and extent of connectivity varied depending on the seed region (see Figure 1 and Table 2). More specifically, all lobules showed extensive functional connectivity within the cerebellum (including with the vermis), and with the sensorimotor cortex, primary visual regions (i.e., intracalcarine and supracalcarine cortices), fusiform cortex, lingual gyrus, precuneus and cuneal cortex, and the brainstem.
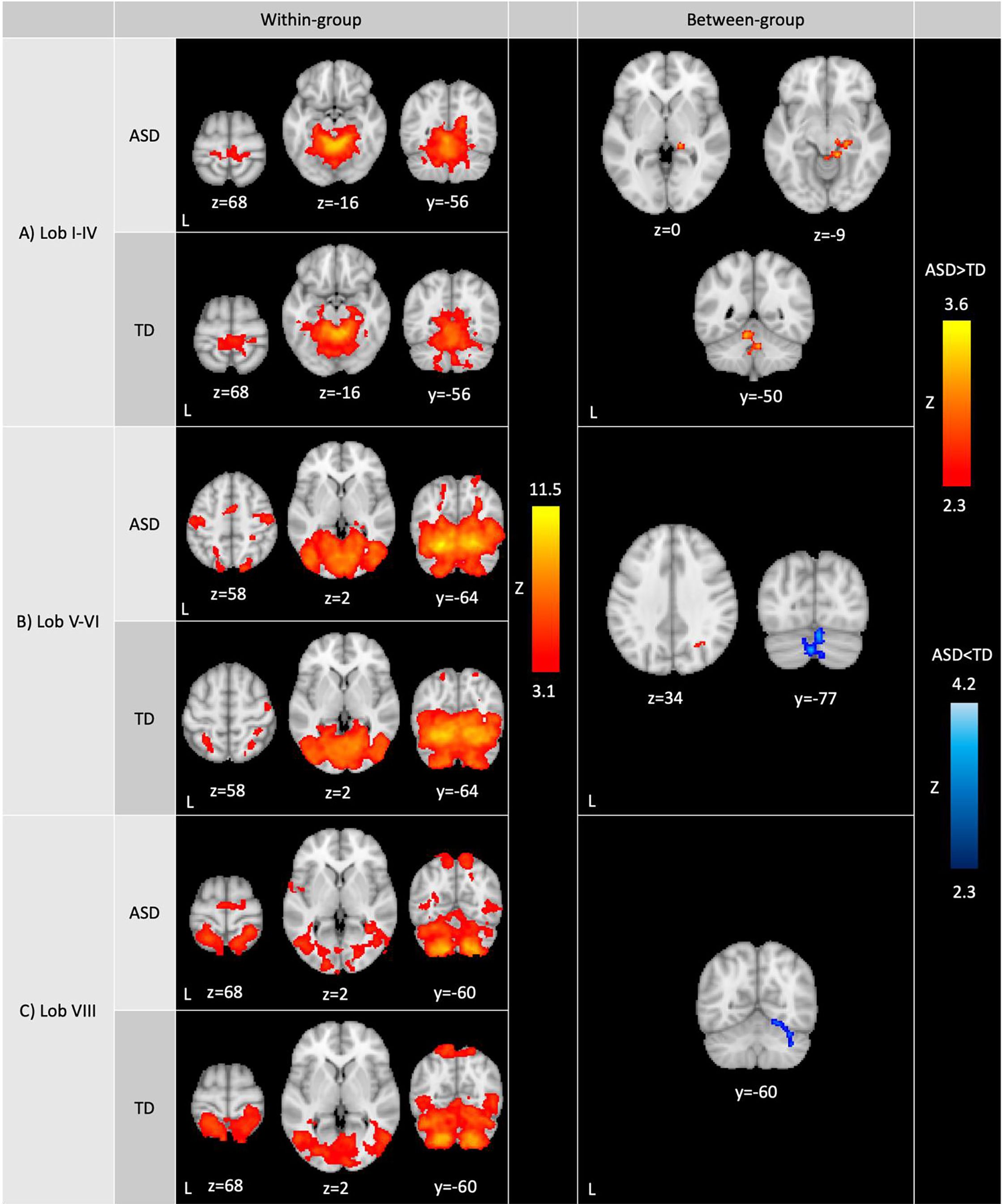
Figure 1 Whole-brain resting-state functional connectivity of the sensorimotor cerebellum. Within-group (left): Within-group ASD and TD functional contrasts were thresholded at Z>3.1, cluster corrected at p<0.05. Between-group (right): Between-group functional contrasts were thresholded at Z>2.3, cluster corrected at p<0.05. Full-scale IQ was included as a covariate in between-group analyses. Cerebellar lobules showed differences in connectivity between ASD and TD groups (red: ASD>TD; blue: ASD<TD). ASD>TD was masked by the ASD within-group contrast to display clusters that show greater positive connectivity in ASD compared to TD. Similarly, ASD<TD was masked by the TD within-group contrast to display clusters that show reduced positive connectivity in ASD compared to TD. ASD: autism spectrum disorder; TD: typically developing youth.
In both ASD and TD groups, lobules I-IV were additionally functionally connected with posterior and anterior parahippocampal gyrus, posterior cingulate gyrus and bilateral hippocampus (Figure 1A, left; Table 2). Lobules V-VI showed additional connectivity with the posterior parahippocampal gyrus, posterior cingulate gyrus, lateral occipital cortex, occipital pole, temporal cortex, higher-order sensory regions (i.e., superior parietal lobule, anterior supramarginal gyrus and operculum), and right hippocampus. Lobule VIII showed further functional connectivity with the lateral occipital cortex, occipital pole, higher-order sensory regions (i.e., superior parietal lobule, anterior supramarginal gyrus, operculum and planum temporale) and temporal cortex.
Between-group analyses. In representing between-group analyses (Figure 1), ASD>TD contrasts were masked posthoc with the within-group contrast representing positive functional connectivity in ASD at z>2.3 to display clusters that show greater positive connectivity in ASD compared to TD. Similarly, ASD<TD contrasts were masked by the within-group contrast demonstrating positive functional connectivity in TD at z>2.3 to display clusters that show greater positive connectivity in TD compared to ASD.
The ASD and TD groups showed significant differences in connectivity across cerebellar seeds. Lobules I-IV showed stronger connectivity in ASD compared to TD with right hippocampus/thalamus (lateral pulvinar nucleus), right posterior parahippocampal gyrus, right lingual gyrus and the brainstem as well as with cerebellar lobules I-IV, left lobules V and IX, and vermis IX and X (Figure 1A, right; Table 2). In ASD, lobules V-VI showed stronger connectivity compared to the TD group with right superior lateral occipital cortex/white matter, and weaker connectivity with cerebellar bilateral crus II, bilateral crus I, right lobule VI, and vermis VI and crus II (Figure 1B, right; Table 2). The ASD group showed weaker lobule VIII functional connectivity with right crus I, right lobule VI, fusiform cortex, and lingual gyrus compared to the TD group.
3.3 SOR correlations with cerebellar connectivity
To determine whether sensorimotor cerebellar connectivity was associated with SOR in ASD, we used SOR as a regressor in whole-brain analyses, co-varying for anxiety.
SOR severity correlated negatively with lobule I-IV connectivity with supplementary motor cortex/superior frontal gyrus (Figure 2). ASD youth with higher SOR had weaker lobule V-VI connectivity with cerebellar right Crus I and II (Table 2). SOR was negatively correlated also with connectivity between lobule VIII and lateral and medial prefrontal cortex (lPFC and mPFC, respectively); and positively correlated with connectivity between lobule VIII and precentral and postcentral gyri, superior parietal lobule, superior lateral occipital cortex, paracentral lobule, and precuneus.
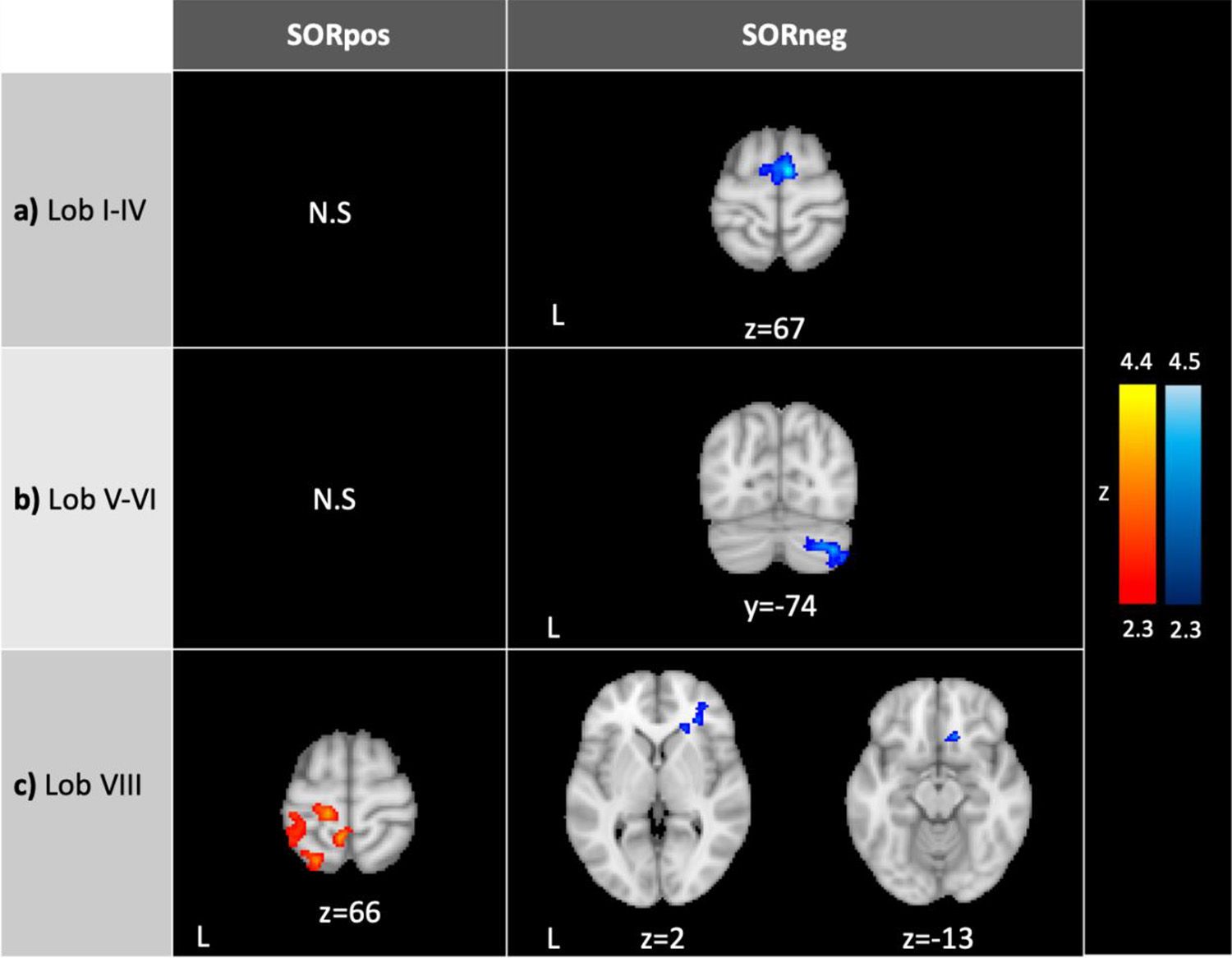
Figure 2 Connectivity of the sensorimotor cerebellum in ASD correlating with SOR. SORpos: Regions where resting-state functional connectivity with lobules I-IV, lobules V-VI and lobule VIII correlates positively with SOR severity (left, in red). SORneg: Regions where resting-state functional connectivity with the cerebellar lobules correlates negatively with SOR (right, in blue). There were no significant clusters where connectivity with lobules I-IV and V-VI correlated positively with SOR. SOR was entered as a bottom-up regressor in analyses, and anxiety was included as a regressor of no-interest. In right c), the images show the same cluster. Contrasts were thresholded at z>2.3 and cluster corrected at p<0.05. SOR, sensory over-responsivity; N.S., no significant clusters.
To ensure that correlations with SOR (e.g., Figure 3) were not driven by outliers, we extracted parameter estimates from regions where connectivity strength was significantly correlated with SOR. We evaluated the parameter estimates for outliers (i.e., ± 3 interquartile range) and detected no outliers. We additionally visually inspected parameter estimate – SOR severity scatterplots for any potential outliers. All the correlations remained significant after removing any potential connectivity-SOR outliers, indicating that these correlations were not driven by outliers.
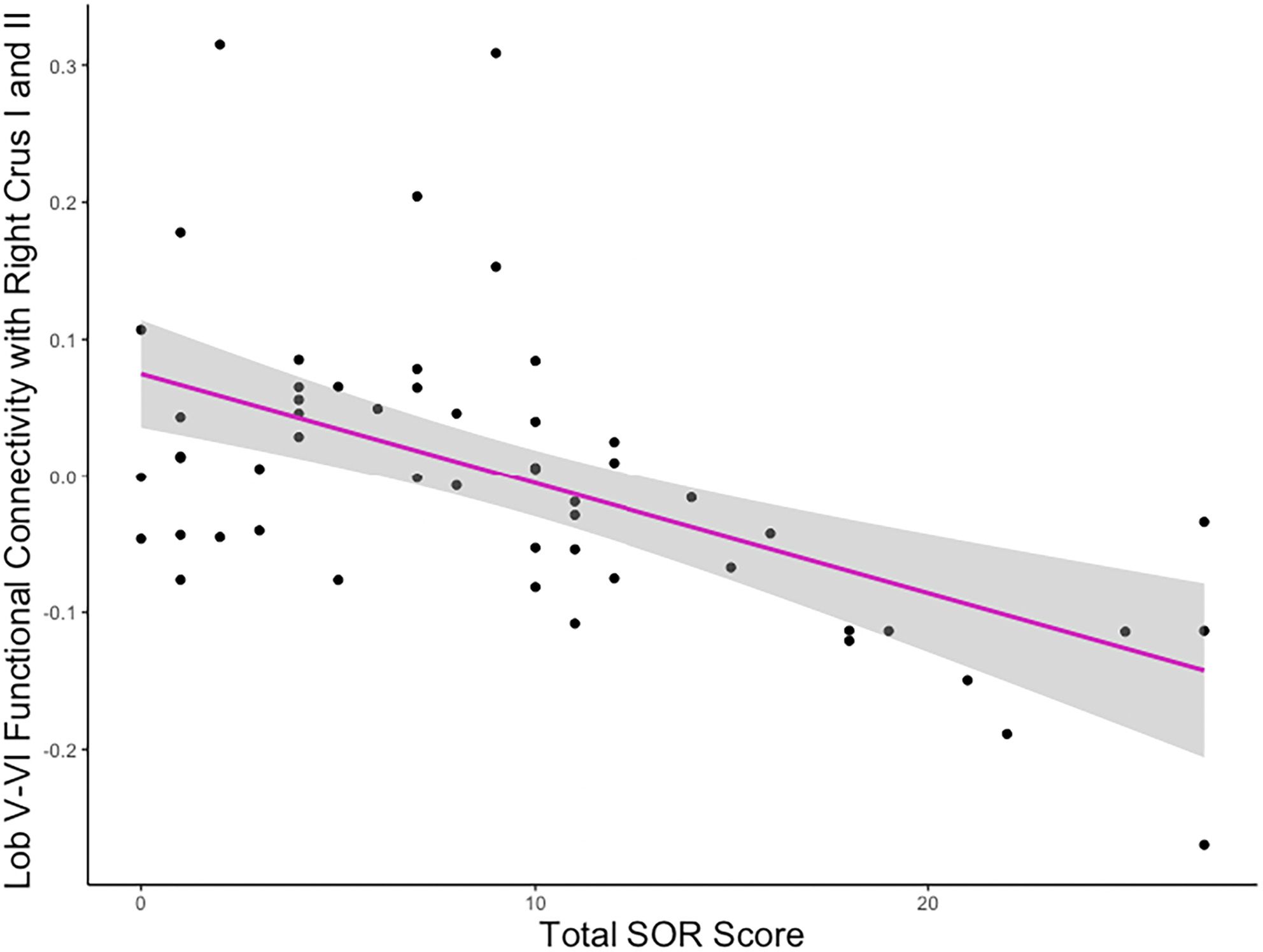
Figure 3 Association between lobule V-VI connectivity strength and SOR. Example plot demonstrating the negative correlation between SOR severity and the strength of connectivity between lobules V-VI and the cluster covering right crus I and II, also portrayed in Figure 2B (right). Y values are residuals after partialling out the effect of anxiety, which was covaried in the analyses.
We additionally investigated the effect of anxiety on cerebellar functional connectivity over and above SOR severity, and found no significant associations between anxiety and cerebellar connectivity.
We replicated all our analyses controlling for age to account for age variability in our sample and found comparable results with age as a covariate (Supplementary Figures 4, 5).
4 Discussion
The focus of this study was to investigate resting-state functional connectivity of the sensorimotor cerebellum in ASD compared to TD youth. Additionally, we examined the association between cerebellar connectivity and sensory over-responsivity within ASD youth. The sensorimotor cerebellum overall showed widespread connections within the cerebellum and with sensorimotor and visual areas, brainstem, precuneus and cuneus. Each of the three seeds also displayed distinct areas of connectivity with the limbic system (lobules I-IV and lobules V-VI) and higher-order sensory regions (lobules V-VI and lobule VIII). These connectivity patterns were, for the most part, consistent across ASD and TD participants. However, the ASD participants did show some significant connectivity differences, particularly within the cerebellum, and between the sensorimotor cerebellum and the hippocampus, thalamus, visual regions, and the brainstem. In addition, within youth with ASD, SOR was associated with sensorimotor cerebellar connectivity with both sensorimotor and visual regions and regions implicated in cognitive and socio-emotional processing. To our knowledge, this is the first study that examined cerebellar atypicalities in the context of sensory over-responsivity in autism.
4.1 Functional connectivity of the sensorimotor cerebellum
For the purposes of the current study, we delineated the sensorimotor cerebellum as three seeds involving lobules I-IV, lobules V-VI and lobule VIII, due to the extensive literature indicating their involvement in sensorimotor processing (e.g., 9, 11, 15, 34). Our results indicated that for both the ASD and TD groups, all three seeds (i.e., lobules I-IV, V-VI and VIII) showed connectivity within the cerebellum, including within the lobule in consistence with Bernard et al. (53), and widespread connectivity with sensorimotor regions, especially visual, somatosensory and motor cortices. Thus, in contrast to prior studies (36), we found connectivity with visual regions across all examined lobules, although lobules V-VI and lobule VIII showed more extensive functional connectivity with the occipital lobe, including the lateral occipital cortex and occipital pole. In terms of sensorimotor cortex, lobules I-IV were also predominantly functionally connected with primary sensory and motor cortices, whereas lobules V-VI and VIII showed more extensive connectivity with higher-order sensory regions. Taken together, our results suggest that processing of sensory signals is integrated across multiple cerebellar lobules, though certain lobules may play a more distinct role in higher-order processing of sensory information.
In addition to connectivity with sensory and motor cortical regions, the sensorimotor cerebellum was functionally connected with limbic regions. We found functional connectivity between the limbic system (i.e., hippocampus, parahippocampal gyrus and posterior cingulate gyrus) and lobules I-IV and V-VI across both ASD and TD participants. These findings are consistent with the growing literature that reports functional connectivity between the cerebellum and the hippocampus (see 54 for a review). While the type of information encoded in this circuitry cannot be determined from the current resting-state study, cerebellar-limbic connectivity may reflect that sensory processing is interrelated with cognitive and emotional processing. Additionally, these results demonstrating connectivity between limbic regions and cerebellar lobules associated with sensory processing also challenge the idea that cerebellar subregions can be divided into specialized sensorimotor and supramodal cognitive zones as initially proposed (11, 36, 55). These findings are thus also consistent with research showing that sensorimotor cerebellar lobules are activated during cognitive tasks (56, 57) and contain representations of non-sensorimotor cerebral networks (e.g., 32, 55).
4.2 Diagnostic group differences in sensorimotor cerebellum connectivity
While the cerebellar networks identified here were overall consistent across ASD and TD groups, there were some notable regions of diagnostic group differences, particularly with sensory regions and hippocampus as well as within the cerebellum.
Visual networks showed distinct patterns of cerebellar connectivity in ASD compared to TD. We found enhanced connectivity between lobules I-IV and lingual gyrus and between lobules V-VI and lateral occipital cortex, while lobule VIII displayed weaker connectivity with lingual gyrus and the fusiform cortex in ASD. Differences in cerebellar connectivity with these visual regions involved in functions such as object recognition and face processing might contribute to atypical processing of visual information in ASD (e.g., 58–60). Furthermore, the sensorimotor cerebellum, particularly lobules I-IV, displayed stronger functional connectivity with the right thalamus in ASD. The thalamus plays an important role in relaying and integrating sensory information (61) and connects cerebello-cortical loops (62). Critically, thalamocortical networks show distinct connectivity patterns in autism (63–67), and thalamic connectivity has been implicated in sensory challenges in ASD youth (26, 68) and in infants with high familial likelihood for ASD (69). Greater connectivity between thalamus and sensorimotor cerebellum therefore may be another indicator of increased processing of sensory information and atypical sensory error signals (see discussion of prediction models below).
Additionally, we found stronger connectivity in ASD between the sensorimotor cerebellum (i.e., lobule I-IV) and the hippocampus compared to in TD youth. Along with the cerebellum, the hippocampus shows structural and functional differences in ASD (70–73), and is also implicated in autistic features, including challenges in social behavior and memory processing as well as strengths in visuo-spatial tasks (see 74 for a review). Importantly, recent literature on hippocampal function suggests that the hippocampus is involved not only in spatial memory, but also in the organization of different kinds of information (i.e., cognitive mapping (74, 75); to help us adapt to changes in our environment. Greater connectivity between the hippocampus and the sensorimotor cerebellum could thus be related to greater neural resources devoted to the organizational mapping of sensory information in ASD, which might contribute to both sensory strengths and challenges seen in ASD. This idea is consistent with previous findings demonstrating stronger reactivity in response sensory stimulation in both the hippocampus and cerebellum in autistic youth (24).
Finally, compared to TD, the ASD group showed greater within-cerebellum connectivity of lobules I-IV, and weaker within-cerebellum connectivity of lobules V-VI and VIII, including with the cerebellar crus I and II. These results are in alignment with previous research showing differences in local functional connectivity of the cerebellum in autism (76, 77), and may indicate distinct functional organization of the cerebellum in ASD. Particularly, a decreased connectivity between the sensorimotor cerebellum and crus I and II, which are functionally connected with the PFC, may indicate reduced integration of processing across functionally distinct cerebellar networks (78). More research is needed to determine how such organizational differences might lead to differences in information processing.
4.3 Sensorimotor cerebellum connectivity and SOR
Given the role of the cerebellum in sensorimotor processing and its demonstrated functional connectivity differences with sensory processing regions in ASD, we further examined whether sensorimotor cerebellar connectivity was associated with SOR symptoms in ASD. We found that more severe SOR symptoms were related to heightened cerebellar connectivity with the primary motor cortex and primary and higher-order sensory regions in ASD, providing a link between atypical cerebellar function in ASD and SOR. This finding is in alignment with Oldehinkel et al. (29) who found stronger cerebellum-sensorimotor network connectivity to be associated with more severe sensory symptoms. Previous research has shown that more severe SOR is associated with reduced habituation in sensory regions (25), suggesting that individuals with ASD and SOR might not adapt to sensory stimuli in the environment. The cerebellum is involved in error-based learning and maintains prediction models of sensory consequences of actions (79). Atypicalities in cerebellar connectivity with the sensorimotor network could be a sign of altered cerebellar signaling of sensory predictions to the sensorimotor network in ASD. This alteration in signaling could indicate lowered predictability of sensorimotor signals and consequently of the external world in ASD (5, 80), leading to reduced habituation and heightened reaction to sensory information as seen in SOR. In addition to alterations in habituation, more severe SOR has previously been linked to lower PFC-amygdala connectivity during sensory stimulation (24, 25), indicating that top-down emotion regulation through the PFC may be reduced in ASD youth with severe SOR. In the current study, more severe SOR in ASD was also associated with weaker sensorimotor cerebellum-PFC connectivity, such that youth with higher SOR showed lower lobule VIII connectivity with mPFC and lPFC. Moreover, more severe SOR was additionally associated with weaker functional connectivity between the sensorimotor cerebellum and crus I and II, the part of the cerebellum that is particularly implicated in socio-emotional function (34, 81, 82). Taken together, these findings suggest that reduced sensorimotor cerebellum connectivity with supramodal regions – important to cognition and emotional processing – as well as increased connectivity with sensorimotor cortex, may contribute to SOR experiences in ASD, and are in line with research demonstrating heightened allocation of mental resources to sensory information, sometimes at the expense of processing other types of information, in autism (e.g., 83, 84).
4.4 Limitations and future directions
The current study has multiple strengths, including examining shared and distinct connectivity of three different sensorimotor cerebellar regions. We also investigated for the first time the role of the cerebellum in SOR in ASD youth, with a relatively large sample compared to prior studies. Nevertheless, our investigation has some limitations. First, while there are many dimensions of sensorimotor function such as sensory acquisition, discrimination and integration, the current study focused on sensory modulation (i.e., how to regulate and respond to incoming sensory information), and specifically SOR, in ASD. Previous research in neurotypical populations examined the role of the cerebellum in other aspects of sensory processing (85), such as in sensory discrimination and acquisition (86, 87) as well as with tasks that involve biological motion (88) and pain stimuli (89). Moreover, atypicalities in multiple aspects of sensorimotor processing have been reported in autism, including differences in integration of sensory feedback (e.g., 90, 91), altered activation and connectivity during motor tasks (e.g., 12) and atypical updating of sensory prediction models (e.g., 92). While SOR warrants particular attention from researchers due to its impairing nature and its developmental progression across adolescence in ASD, future research should characterize the involvement of the cerebellum in the full range of sensory processing atypicalities seen in ASD, including other sensory modulation atypicalities (i.e., sensory under-responsivity and sensory seeking).
Second, in the current study, we assessed SOR severity with parent-report. While parent-report (along with self-report) is one of the most common methods to assess sensory features in ASD samples (93, 94), it may not capture all aspects of one’s sensory experiences. In fact, parent-reported, self-reported and observed sensory assessments may tap into different aspects of sensory processing and complement one another (42, 95). For example, while parent-report might involve insights into behavioral responses to sensory stimuli and past history of sensory challenges, self-report could better represent internal experience, especially in older participants, and observed assessments (in the presence of an experimenter) may capture ability to regulate sensory responses rather than purely sensory experience (42, 95–97). In investigating the link between SOR and cerebellar function, future studies could utilize an integrated (i.e., self-reported, parent-reported and observed SOR) approach to measure SOR comprehensively and also interrogate how cerebellar connectivity relates to different aspects of sensory processing.
Third, while our sample included a relatively large number of females compared to many autism studies, the current study was underpowered to examine sex differences in cerebellar connectivity and its relationship with SOR. Emerging evidence shows that that sensory symptoms and restricted and repetitive behaviors (RRBs) may have different underlying neurobiology in girls versus boys with ASD (e.g., 27, 98), and thus future studies should examine sex differences in cerebellar connectivity and their differential relationship to SOR. Fourth, the sample in the current study is a pediatric sample including youth in middle childhood and adolescence. The development of the cerebellum continues through adolescence, with different parts of the cerebellum reaching their mature state at distinct times during development (99–101). Cerebellar responses to aversive sensory information may also change across development (43). Thus, future studies should investigate how connectivity of the sensorimotor cerebellum evolves during development, especially during adolescence, and how these developmental changes relate to SOR severity. Fifth, in the current study, the sensorimotor regions within the cerebellum were defined based on the neuroanatomy of the brain region. However, recent research has shown that anatomical cerebellar regions do not map on to functional subdivisions (56), indicating that the sensorimotor cerebellum might be more accurately defined by functional masks. In fact, sensorimotor cerebellar lobules encompass functional regions that are activated during non-sensorimotor processes, such as theory of mind, working memory and verb generation tasks (56, 57). Lobule VI, in particular, is involved in cognition and executive function (102). Thus, anatomical sensorimotor seeds might include non-sensorimotor subsections of the cerebellum as well as exclude sensorimotor functional regions outside of the anatomically defined lobules. Hence, future studies should use functionally defined sensorimotor cerebellum masks to assess the connectivity of the sensorimotor regions within the cerebellum. Furthermore, while we conducted analyses with cerebellar seeds, alternative analytic approaches to examine cerebellar connectivity (e.g., investigating cerebellar connectivity with cerebral seeds or with smaller seed regions) could be explored in future studies. Finally, while SOR is particularly prevalent in the autistic population, TD individuals also experience SOR symptoms. In fact, our original sample included one TD participant with elevated SOR severity, who was excluded from our final sample. Future research should also investigate SOR variability in other populations and whether altered cerebellar functional connectivity is involved in neural mechanisms of SOR in typical development or other non-autistic populations.
5 Conclusion
In the current study, we found that, across both diagnostic groups, the three sensorimotor cerebellar seeds that were examined, namely lobules I-IV, V-VI and VIII, were all functionally connected with sensorimotor and visual areas, brainstem, precuneus and cuneus, but showed distinctions in their connectivity with limbic and higher-order sensory regions. Youth with ASD had atypical connectivity of the cerebellum with the thalamus, hippocampus, parahippocampal gyrus, brainstem, and the visual cortex as well as within the cerebellum. In relation to SOR, we showed that more severe SOR is associated with stronger connectivity between the sensorimotor cerebellum and cerebral sensorimotor regions and precuneus, and weaker connectivity between the cerebellum and cognitive and socio-emotional regions, particularly the prefrontal cortex. These findings provide evidence for a link between functional cerebellar atypicalities in ASD and SOR for the first time. The current study adds to the recent literature indicating the involvement of cerebellar atypicalities in the differences in perception and behavior seen in ASD. Taken together with past research that described a role for the cerebellum in socio-communicative symptoms of ASD, our findings suggest that the cerebellum should be considered in the study of ASD and as well as the study of atypical sensory processing in other populations.
Data availability statement
The raw data supporting the conclusions of this article will be made available by the authors, without undue reservation.
Ethics statement
The studies involving humans were approved by University of California, Los Angeles, Institutional Review Board. The studies were conducted in accordance with the local legislation and institutional requirements. Written informed consent for participation in this study was provided by the participants’ legal guardians/next of kin.
Author contributions
MC: Writing – review & editing, Writing – original draft, Visualization, Formal analysis, Data curation, Conceptualization. NO: Writing – review & editing, Methodology, Investigation, Data curation. KC: Writing – review & editing, Investigation, Data curation. JJ: Writing – review & editing, Investigation, Data curation. SB: Writing – review & editing, Supervision, Methodology, Funding acquisition, Conceptualization. MD: Writing – review & editing, Supervision, Resources, Methodology. SG: Writing – review & editing, Supervision, Resources, Project administration, Methodology, Funding acquisition, Conceptualization.
Funding
The author(s) declare that financial support was received for the research, authorship, and/or publication of this article. This work was supported by grants from the Simons Foundation Autism Research Initiative (Grant Number 345389), National Institute of Child Health and Human Development (P50 HD055784), and the National Institute of Mental Health (R01 MH100028; R01 MH117982; K08 MH112871; R01 MH124977). For generous support, the authors also wish to thank the Brain Mapping Medical Research Organization, Brain Mapping Support Foundation, Pierson-Lovelace Foundation, The Ahmanson Foundation, William M. and Linda R. Dietel Philanthropic Fund at the Northern Piedmont Community Foundation, Tamkin Foundation, Jennifer Jones-Simon Foundation, Capital Group Companies Charitable Foundation, Robson Family and Northstar Fund. The project described was supported by Grant Numbers RR12169, RR13642, and RR00865 from the National Center for Research Resources (NCRR), a component of the National Institutes of Health (NIH); its contents are solely the responsibility of the authors and do not necessarily represent the official views of NCR or NIH. The funding sources and organizations listed above had no role in the design and conduct of the study; collection, management, analysis, and interpretation of the data; preparation, review, or approval of the manuscript; and decision to submit the manuscript for publication. These research efforts were conducted in part under the auspices of The Help Group-UCLA Autism Research Alliance, which contributed to participant recruitment.
Conflict of interest
The authors declare that the research was conducted in the absence of any commercial or financial relationships that could be construed as a potential conflict of interest.
Publisher’s note
All claims expressed in this article are solely those of the authors and do not necessarily represent those of their affiliated organizations, or those of the publisher, the editors and the reviewers. Any product that may be evaluated in this article, or claim that may be made by its manufacturer, is not guaranteed or endorsed by the publisher.
Supplementary material
The Supplementary Material for this article can be found online at: https://www.frontiersin.org/articles/10.3389/fpsyt.2024.1337921/full#supplementary-material
Footnotes
References
1. American Psychiatric Association. Diagnostic and statistical manual of mental disorders. Fifth Edition. Washington, D.C.: American Psychiatric Association (2013). doi: 10.1176/appi.books.9780890425596
2. Wang SSH, Kloth AD, Badura A. The cerebellum, sensitive periods, and autism. Neuron. (2014) 83:518–32. doi: 10.1016/j.neuron.2014.07.016
3. Stoodley CJ, D’Mello AM, Ellegood J, Jakkamsetti V, Liu P, Nebel MB, et al. Altered cerebellar connectivity in autism and cerebellar-mediated rescue of autism-related behaviors in mice. Nat Neurosci. (2017) 20:1744–51. doi: 10.1038/s41593-017-0004-1
4. Kelly E, Meng F, Fujita H, Morgado F, Kazemi Y, Rice LC, et al. Regulation of autism-relevant behaviors by cerebellar–prefrontal cortical circuits. Nat Neurosci. (2020) 23:1102–10. doi: 10.1038/s41593-020-0665-z
5. Kelly E, Escamilla CO, Tsai PT. Cerebellar dysfunction in autism spectrum disorders: deriving mechanistic insights from an internal model framework. Neuroscience. (2021) 462:274–87. doi: 10.1016/j.neuroscience.2020.11.012
6. Su LD, Xu FX, Wang XT, Cai XY, Shen Y. Cerebellar dysfunction, cerebro-cerebellar connectivity and autism spectrum disorders. Neuroscience. (2021) 462:320–7. doi: 10.1016/j.neuroscience.2020.05.028
7. King M. The big role of the ‘little brain’: exploring the developing cerebellum and its role in cognition. Curr Opin Behav Sci. (2023) 54:101301. doi: 10.1016/j.cobeha.2023.101301
8. Allen G, Müller RA, Courchesne E. Cerebellar function in autism: functional magnetic resonance image activation during a simple motor task. Biol Psychiatry. (2004) 56:269–78. doi: 10.1016/j.biopsych.2004.06.005
9. D’Mello AM, Stoodley CJ. Cerebro-cerebellar circuits in autism spectrum disorder. Front Neurosci. (2015) 9:408/full. doi: 10.3389/fnins.2015.00408/full
10. Fatemi SH, Halt AR, Realmuto G, Earle J, Kist DA, Thuras P, et al. Purkinje cell size is reduced in cerebellum of patients with autism. Cell Mol Neurobiol. (2002) 22:171–5. doi: 10.1023/A:1019861721160
11. Khan AJ, Nair A, Keown CL, Datko MC, Lincoln AJ, Müller RA. Cerebro-cerebellar resting-state functional connectivity in children and adolescents with autism spectrum disorder. Biol Psychiatry. (2015) 78:625–34. doi: 10.1016/j.biopsych.2015.03.024
12. Mostofsky SH, Powell SK, Simmonds DJ, Goldberg MC, Caffo B, Pekar JJ. Decreased connectivity and cerebellar activity in autism during motor task performance. Brain. (2009) 132:2413–25. doi: 10.1093/brain/awp088
13. Rogers TD, McKimm E, Dickson PE, Goldowitz D, Blaha CD, Mittleman G. Is autism a disease of the cerebellum? An integration of clinical and pre-clinical research. Front Syst Neurosci. (2013) 7. https://www.ncbi.nlm.nih.gov/pmc/articles/PMC3650713/. doi: 10.3389/fnsys.2013.00015
14. Takarae Y, Minshew NJ, Luna B, Sweeney JA. Atypical involvement of frontostriatal systems during sensorimotor control in autism. Psychiatry Res. (2007) 156:117–27. doi: 10.1016/j.pscychresns.2007.03.008
15. Stoodley CJ, Schmahmann JD. Chapter 4 - Functional topography of the human cerebellum. In: Manto M, Huisman TAGM, editors. The cerebellum: from embryology to diagnostic investigations, vol. 154 . Amsterdam, Netherlands: Elsevier (2018). p. 59–70. Available at: http://www.sciencedirect.com/science/article/pii/B9780444639561000047.
16. Ben-Sasson A, Cermak SA, Orsmond GI, Tager-Flusberg H, Carter AS, Kadlec MB, et al. Extreme sensory modulation behaviors in toddlers with autism spectrum disorders. Am J Occup Ther. (2007) 61:584–92. doi: 10.5014/ajot.61.5.584
17. Ben-Sasson A, Carter AS, Briggs-Gowan MJ. Sensory over-responsivity in elementary school: prevalence and social-emotional correlates. J Abnorm Child Psychol. (2009) 37:705–16. doi: 10.1007/s10802-008-9295-8
18. Baranek GT, David FJ, Poe MD, Stone WL, Watson LR. Sensory Experiences Questionnaire: discriminating sensory features in young children with autism, developmental delays, and typical development. J Child Psychol Psychiatry. (2006) 47:591–601. doi: 10.1111/j.1469-7610.2005.01546.x
19. Carson TB, Valente MJ, Wilkes BJ, Richard L. Brief report: prevalence and severity of auditory sensory over-responsivity in autism as reported by parents and caregivers. J Autism Dev Disord. (2021) 52:1395–402. doi: 10.1007/s10803-021-04991-0
20. Leekam SR, Nieto C, Libby SJ, Wing L, Gould J. Describing the sensory abnormalities of children and adults with autism. J Autism Dev Disord. (2007) 37:894–910. doi: 10.1007/s10803-006-0218-7
21. Cermak SA, Curtin C, Bandini LG. Food selectivity and sensory sensitivity in children with autism spectrum disorders. J Am Diet Assoc. (2010) 110:238–46. doi: 10.1016/j.jada.2009.10.032
22. Schaaf RC, Benevides TW, Blanche E, Brett-Green BA, Burke J, Cohn E, et al. Parasympathetic functions in children with sensory processing disorder. Front Integr Neurosci. (2010) 4:4/full. doi: 10.3389/fnint.2010.00004/full
23. Stein LI, Polido JC, Cermak SA. Oral care and sensory over-responsivity in children with autism spectrum disorders. Pediatr Dentistry. (2013) 35:230–5.
24. Green SA, Hernandez L, Tottenham N, Krasileva K, Bookheimer SY, Dapretto M. Neurobiology of sensory overresponsivity in youth with autism spectrum disorders. JAMA Psychiatry. (2015) 72:778. doi: 10.1001/jamapsychiatry.2015.0737
25. Green SA, Hernandez L, Lawrence KE, Liu J, Tsang T, Yeargin J, et al. Distinct patterns of neural habituation and generalization in children and adolescents with autism with low and high sensory overresponsivity. Am J Psychiatry. (2019) 176:1010–20. doi: 10.1176/appi.ajp.2019.18121333
26. Green SA, Hernandez L, Bookheimer SY, Dapretto M. Reduced modulation of thalamocortical connectivity during exposure to sensory stimuli in ASD. Autism Res. (2017) 10:801–9. doi: 10.1002/aur.1726
27. Cummings KK, Lawrence KE, Hernandez LM, Wood ET, Bookheimer SY, Dapretto M, et al. Sex differences in salience network connectivity and its relationship to sensory over-responsivity in youth with autism spectrum disorder. Autism Res. (2020) 13:1489–500. doi: 10.1002/aur.2351
28. Green SA, Hernandez L, Bookheimer SY, Dapretto M. Salience network connectivity in autism is related to brain and behavioral markers of sensory overresponsivity. J Am Acad Child Adolesc Psychiatry. (2016) 55:618–626.e1. doi: 10.1016/j.jaac.2016.04.013
29. Oldehinkel M, Mennes M, Marquand A, Charman T, Tillmann J, Ecker C, et al. Altered connectivity between cerebellum, visual, and sensory-motor networks in autism spectrum disorder: results from the EU-AIMS longitudinal European autism project. Biol Psychiatry: Cogn Neurosci Neuroimaging. (2019) 4:260–70. doi: 10.1016/j.bpsc.2018.11.010
30. Ben-Sasson A, Gal E, Fluss R, Katz-Zetler N, Cermak SA. Update of a meta-analysis of sensory symptoms in ASD: A new decade of research. J Autism Dev Disord. (2019) 49:4974–96. doi: 10.1007/s10803-019-04180-0
31. Stoodley CJ, Schmahmann JD. Evidence for topographic organization in the cerebellum of motor control versus cognitive and affective processing. Cortex. (2010) 46:831–44. doi: 10.1016/j.cortex.2009.11.008
32. Buckner RL, Krienen FM, Castellanos A, Diaz JC, Yeo BTT. The organization of the human cerebellum estimated by intrinsic functional connectivity. J Neurophysiol. (2011) 106:2322–45. doi: 10.1152/jn.00339.2011
33. Kipping JA, Grodd W, Kumar V, Taubert M, Villringer A, Margulies DS. Overlapping and parallel cerebello-cerebral networks contributing to sensorimotor control: An intrinsic functional connectivity study. NeuroImage. (2013) 83:837–48. doi: 10.1016/j.neuroimage.2013.07.027
34. Leggio M, Olivito G. Chapter 5 - Topography of the cerebellum in relation to social brain regions and emotions. In: Manto M, Huisman TAGM, editors. The cerebellum: from embryology to diagnostic investigations, vol. 154. Amsterdam, Netherlands:Elsevier (2018). p. 71–84. Available at: http://www.sciencedirect.com/science/article/pii/B9780444639561000059.
35. Gruol DL, Koibuchi N, Manto M, Molinari M, Schmahmann JD, Shen Y eds. Essentials of cerebellum and cerebellar disorders: A primer for graduate students. Cham: Springer International Publishing (2023). doi: 10.1007/978-3-031-15070-8
36. O’Reilly JX, Beckmann CF, Tomassini V, Ramnani N, Johansen-Berg H. Distinct and overlapping functional zones in the cerebellum defined by resting state functional connectivity. Cereb Cortex. (2010) 20:953–65. doi: 10.1093/cercor/bhp157
37. Sang L, Qin W, Liu Y, Han W, Zhang Y, Jiang T, et al. Resting-state functional connectivity of the vermal and hemispheric subregions of the cerebellum with both the cerebral cortical networks and subcortical structures. NeuroImage. (2012) 61:1213–25. doi: 10.1016/j.neuroimage.2012.04.011
38. Lord C, Rutter M, Le Couteur A. Autism Diagnostic Interview-Revised: A revised version of a diagnostic interview for caregivers of individuals with possible pervasive developmental disorders. J Autism Dev Disord. (1994) 24:659–85. doi: 10.1007/BF02172145
39. Lord C, Rutter M, DiLavore P, Risi S, Gotham K, Bishop S. Autism diagnostic observation schedule–2nd edition (ADOS-2) Vol. 284. Los Angeles, CA: Western Psychological Corporation (2012).
40. Wechsler D. Wechsler abbreviated scale of intelligence (WASI). San Antonio, TX, US: Harcourt Assessment. Inc: Pearson (1999). doi: 10.1037/t15170-000
41. Miller LJ, Schoen SA, Mulligan S, Sullivan J. Identification of sensory processing and integration symptom clusters: A preliminary study. Occup Ther Int. (2017) 2017:e2876080. doi: 10.1155/2017/2876080
42. Ramappa S, Anderson A, Jung J, Chu R, Cummings KK, Patterson G, et al. An observed assessment of sensory responsivity in autism spectrum disorders: associations with diagnosis, age, and parent report. J Autism Dev Disord. (2022) 53(10):3860–72. doi: 10.1007/s10803-022-05653-5
43. Cakar ME, Cummings KK, Bookheimer SY, Dapretto M, Green SA. Age-related changes in neural responses to sensory stimulation in autism: a cross-sectional study. Mol Autism. (2023) 14:38. doi: 10.1186/s13229-023-00571-4
44. Birmaher B, Khetarpal S, Brent D, Cully M, Balach L, Kaufman J, et al. The screen for child anxiety related emotional disorders (SCARED): scale construction and psychometric characteristics. J Am Acad Child Adolesc Psychiatry. (1997) 36:545–53. doi: 10.1097/00004583-199704000-00018
45. Green SA, Ben-Sasson A. Anxiety disorders and sensory over-responsivity in children with autism spectrum disorders: is there a causal relationship? J Autism Dev Disord. (2010) 40:1495–504. doi: 10.1007/s10803-010-1007-x
46. Pruim RHR, Mennes M, Buitelaar JK, Beckmann CF. Evaluation of ICA-AROMA and alternative strategies for motion artifact removal in resting state fMRI. Neuroimage. (2015) 112:278–87. doi: 10.1016/j.neuroimage.2015.02.063
47. Pruim RHR, Mennes M, van Rooij D, Llera A, Buitelaar JK, Beckmann CF. ICA-AROMA: A robust ICA-based strategy for removing motion artifacts from fMRI data. Neuroimage. (2015) 112:267–77. doi: 10.1016/j.neuroimage.2015.02.064
48. Diedrichsen J, Balsters JH, Flavell J, Cussans E, Ramnani N. A probabilistic MR atlas of the human cerebellum. NeuroImage. (2009) 46:39–46. doi: 10.1016/j.neuroimage.2009.01.045
49. Beckmann CF, Jenkinson M, Smith SM. General multilevel linear modeling for group analysis in FMRI. Neuroimage. (2003) 20:1052–63. doi: 10.1016/S1053-8119(03)00435-X
50. Woolrich MW, Behrens TEJ, Beckmann CF, Jenkinson M, Smith SM. Multilevel linear modelling for FMRI group analysis using Bayesian inference. Neuroimage. (2004) 21:1732–47. doi: 10.1016/j.neuroimage.2003.12.023
51. Woolrich M. Robust group analysis using outlier inference. Neuroimage. (2008) 41:286–301. doi: 10.1016/j.neuroimage.2008.02.042
52. Green SA, Ben-Sasson A, Soto TW, Carter AS. Anxiety and sensory over-responsivity in toddlers with autism spectrum disorders: bidirectional effects across time. J Autism Dev Disord. (2012) 42:1112–9. doi: 10.1007/s10803-011-1361-3
53. Bernard J, Seidler R, Hassevoort K, Benson B, Welsh R, Wiggins J, et al. Resting state cortico-cerebellar functional connectivity networks: a comparison of anatomical and self-organizing map approaches. Front Neuroanat. (2012) 6:31. doi: 10.3389/fnana.2012.00031
54. Froula JM, Hastings SD, Krook-Magnuson E. The little brain and the seahorse: Cerebellar-hippocampal interactions. Front Syst Neurosci. (2023) 17:1158492. doi: 10.3389/fnsys.2023.1158492
55. Habas C, Kamdar N, Nguyen D, Prater K, Beckmann CF, Menon V, et al. Distinct cerebellar contributions to intrinsic connectivity networks. J Neurosci. (2009) 29:8586–94. doi: 10.1523/JNEUROSCI.1868-09.2009
56. King M, Hernandez-Castillo CR, Poldrack RA, Ivry RB, Diedrichsen J. Functional boundaries in the human cerebellum revealed by a multi-domain task battery. Nat Neurosci. (2019) 22:1371–8. doi: 10.1038/s41593-019-0436-x
57. Stoodley CJ, Valera EM, Schmahmann JD. Functional topography of the cerebellum for motor and cognitive tasks: An fMRI study. NeuroImage. (2012) 59:1560–70. doi: 10.1016/j.neuroimage.2011.08.065
58. Dakin S, Frith U. Vagaries of visual perception in autism. Neuron. (2005) 48:497–507. doi: 10.1016/j.neuron.2005.10.018
59. Nilsson Jobs E, Falck-Ytter T, Bölte S. Local and global visual processing in 3-year-olds with and without autism. J Autism Dev Disord. (2018) 48:2249–57. doi: 10.1007/s10803-018-3470-8
60. Robertson CE, Baron-Cohen S. Sensory perception in autism. Nat Rev Neurosci. (2017) 18:671–84. doi: 10.1038/nrn.2017.112
61. Wolff M, Morceau S, Folkard R, Martin-Cortecero J, Groh A. A thalamic bridge from sensory perception to cognition. Neurosci Biobehav Rev. (2021) 120:222–35. doi: 10.1016/j.neubiorev.2020.11.013
62. Habas C, Manto M, Cabaraux P. The cerebellar thalamus. Cerebellum. (2019) 18:635–48. doi: 10.1007/s12311-019-01019-3
63. Woodward ND, Giraldo-Chica M, Rogers B, Cascio CJ. Thalamocortical dysconnectivity in autism spectrum disorder: An analysis of the Autism Brain Imaging Data Exchange. Biol Psychiatry Cognit Neurosci Neuroimaging. (2017) 2:76–84. doi: 10.1016/j.bpsc.2016.09.002
64. Iidaka T, Kogata T, Mano Y, Komeda H. Thalamocortical hyperconnectivity and amygdala-cortical hypoconnectivity in male patients with autism spectrum disorder. Front Psychiatry. (2019) 10. doi: 10.3389/fpsyt.2019.00252
65. Ayub R, Sun KL, Flores RE, Lam VT, Jo B, Saggar M, et al. Thalamocortical connectivity is associated with autism symptoms in high-functioning adults with autism and typically developing adults. Transl Psychiatry. (2021) 11:1–9. doi: 10.1038/s41398-021-01221-0
66. Baran B, Nguyen QTH, Mylonas D, Santangelo SL, Manoach DS. Increased resting-state thalamocortical functional connectivity in children and young adults with autism spectrum disorder. Autism Res. (2023) 16:271–9. doi: 10.1002/aur.2875
67. Hwang WJ, Kwak YB, Cho KIK, Lee TY, Oh H, Ha M, et al. Thalamic connectivity system across psychiatric disorders: current status and clinical implications. Biol Psychiatry Global Open Science. (2022) 2:332–40. doi: 10.1016/j.bpsgos.2021.09.008
68. Wood ET, Cummings KK, Jung J, Patterson G, Okada N, Guo J, et al. Sensory over-responsivity is related to GABAergic inhibition in thalamocortical circuits. Transl Psychiatry. (2021) 11:39. doi: 10.1038/s41398-020-01154-0
69. Wagner L, Banchik M, Okada NJ, McDonald N, Jeste SS, Bookheimer SY, et al. Associations between thalamocortical functional connectivity and sensory over-responsivity in infants at high likelihood for ASD. Cereb Cortex. (2023) 33:8075–86. doi: 10.1093/cercor/bhad100
70. Barnea-Goraly N, Frazier TW, Piacenza L, Minshew NJ, Keshavan MS, Reiss AL, et al. A preliminary longitudinal volumetric MRI study of amygdala and hippocampal volumes in autism. Prog Neuropsychopharmacol Biol Psychiatry. (2014) 48:124–8. doi: 10.1016/j.pnpbp.2013.09.010
71. Groen W, Teluij M, Buitelaar J, Tendolkar I. Amygdala and hippocampus enlargement during adolescence in autism. J Am Acad Child Adolesc Psychiatry. (2010) 49:552–60. doi: 10.1097/00004583-201006000-00004
72. Modi B, Pimpinella D, Pazienti A, Zacchi P, Cherubini E, Griguoli M. Possible implication of the CA2 hippocampal circuit in social cognition deficits observed in the neuroligin 3 knock-out mouse, a non-syndromic animal model of autism. Front Psychiatry. (2019) 10:513. doi: 10.3389/fpsyt.2019.00513
73. Solomon M, Ragland JD, Niendam TA, Lesh TA, Beck JS, Matter JC, et al. Atypical learning in autism spectrum disorders: A functional magnetic resonance imaging study of transitive inference. J Am Acad Child Adolesc Psychiatry. (2015) 54:947–55. doi: 10.1016/j.jaac.2015.08.010
74. Banker SM, Gu X, Schiller D, Foss-Feig JH. Hippocampal contributions to social and cognitive deficits in autism spectrum disorder. Trends Neurosciences. (2021) 44:793–807. doi: 10.1016/j.tins.2021.08.005
75. Schiller D, Eichenbaum H, Buffalo EA, Davachi L, Foster DJ, Leutgeb S, et al. Memory and space: towards an understanding of the cognitive map. J Neurosci. (2015) 35:13904–11. doi: 10.1523/JNEUROSCI.2618-15.2015
76. Dajani DR, Uddin LQ. Local brain connectivity across development in autism spectrum disorder: A cross-sectional investigation. Autism Res. (2016) 9:43–54. doi: 10.1002/aur.1494
77. Paakki JJ, Rahko J, Long X, Moilanen I, Tervonen O, Nikkinen J, et al. Alterations in regional homogeneity of resting-state brain activity in autism spectrum disorders. Brain Res. (2010) 1321:169–79. doi: 10.1016/j.brainres.2009.12.081
78. Wang Z, Wang Y, Sweeney JA, Gong Q, Lui S, Mosconi MW. Resting-state brain network dysfunctions associated with visuomotor impairments in autism spectrum disorder. Front Integr Neurosci. (2019) 13:17. doi: 10.3389/fnint.2019.00017
79. Sokolov AA, Miall RC, Ivry RB. The cerebellum: adaptive prediction for movement and cognition. Trends Cogn Sci. (2017) 21:313–32. doi: 10.1016/j.tics.2017.02.005
80. Sinha P, Kjelgaard MM, Gandhi TK, Tsourides K, Cardinaux AL, Pantazis D, et al. Autism as a disorder of prediction. Proc Natl Acad Sci USA. (2014) 111:15220–5. doi: 10.1073/pnas.1416797111
81. Van Overwalle F, Ma Q, Heleven E. The posterior crus II cerebellum is specialized for social mentalizing and emotional self-experiences: a meta-analysis. Soc Cogn Affect Neurosci. (2020) 15:905–28. doi: 10.1093/scan/nsaa124
82. Van Overwalle F, Pu M, Ma Q, Li M, Haihambo N, Baetens K, et al. The involvement of the posterior cerebellum in reconstructing and predicting social action sequences. Cerebellum. (2022) 21:733–41. doi: 10.1007/s12311-021-01333-9
83. Gong X, Li X, Wang Q, Hoi SP, Yin T, Zhao L, et al. Comparing visual preferences between autism spectrum disorder (ASD) and normal children to explore the characteristics of visual preference of ASD children by improved visual preference paradigm: a case-control study. Transl Pediatr. (2021) 10:2006–15. doi: 10.21037/tp
84. Green SA, Hernandez LM, Bowman HC, Bookheimer SY, Dapretto M. Sensory over-responsivity and social cognition in ASD: Effects of aversive sensory stimuli and attentional modulation on neural responses to social cues. Dev Cogn Neurosci. (2018) 29:127–39. doi: 10.1016/j.dcn.2017.02.005
85. Baumann O, Borra RJ, Bower JM, Cullen KE, Habas C, Ivry RB, et al. Consensus paper: the role of the cerebellum in perceptual processes. Cerebellum. (2015) 14:197–220. doi: 10.1007/s12311-014-0627-7
86. Gao JH, Parsons LM, Bower JM, Xiong J, Li J, Fox PT. Cerebellum implicated in sensory acquisition and discrimination rather than motor control. Science. (1996) 272:545–7. doi: 10.1126/science.272.5261.545
87. Baumann O, Mattingley JB. Scaling of neural responses to visual and auditory motion in the human cerebellum. J Neurosci. (2010) 30:4489–95. doi: 10.1523/JNEUROSCI.5661-09.2010
88. Sokolov AA, Erb M, Gharabaghi A, Grodd W, Tatagiba MS, Pavlova MA. Biological motion processing: The left cerebellum communicates with the right superior temporal sulcus. NeuroImage. (2012) 59:2824–30. doi: 10.1016/j.neuroimage.2011.08.039
89. Coombes SA, Misra G. Pain and motor processing in the human cerebellum. PAIN. (2016) 157:117–27. doi: 10.1097/j.pain.0000000000000337
90. Shafer RL, Wang Z, Bartolotti J, Mosconi MW. Visual and somatosensory feedback mechanisms of precision manual motor control in autism spectrum disorder. J Neurodev Disord. (2021) 13:32. doi: 10.1186/s11689-021-09381-2
91. Lepping RJ, McKinney WS, Magnon GC, Keedy SK, Wang Z, Coombes SA, et al. Visuomotor brain network activation and functional connectivity among individuals with autism spectrum disorder. Hum Brain Mapping. (2022) 43:844–59. doi: 10.1002/hbm.25692
92. Goris J, Braem S, Herck SV, Simoens J, Deschrijver E, Wiersema JR, et al. Reduced primacy bias in autism during early sensory processing. J Neurosci. (2022) 42(19):3989–99. doi: 10.1523/JNEUROSCI.3088-20.2022
93. Burns CO, Dixon DR, Novack M, Granpeesheh D. A systematic review of assessments for sensory processing abnormalities in autism spectrum disorder. Rev J Autism Dev Disord. (2017) 4:209–24. doi: 10.1007/s40489-017-0109-1
94. Gunderson J, Worthley E, Byiers B, Symons F, Wolff J. Self and caregiver report measurement of sensory features in autism spectrum disorder: a systematic review of psychometric properties. J Neurodevelop Disord. (2023) 15:5. doi: 10.1186/s11689-022-09473-7
95. Keith JM, Jamieson JP, Bennetto L. The importance of adolescent self-report in autism spectrum disorder: integration of questionnaire and autonomic measures. J Abnorm Child Psychol. (2019) 47:741–54. doi: 10.1007/s10802-018-0455-1
96. Cascio CJ, Woynaroski T, Baranek GT, Wallace MT. Toward an interdisciplinary approach to understanding sensory function in autism spectrum disorder. Autism Res. (2016) 9:920–5. doi: 10.1002/aur.1612
97. Tavassoli T, Brandes-Aitken A, Chu R, Porter L, Schoen S, Miller LJ, et al. Sensory over-responsivity: parent report, direct assessment measures, and neural architecture. Mol Autism. (2019) 10:4. doi: 10.1186/s13229-019-0255-7
98. Supekar K, Menon V. Sex differences in structural organization of motor systems and their dissociable links with repetitive/restricted behaviors in children with autism. Mol Autism. (2015) 6:50. doi: 10.1186/s13229-015-0042-z
99. Bernard JA, Leopold DR, Calhoun VD, Mittal VA. Regional cerebellar volume and cognitive function from adolescence to late middle age. Hum Brain Mapping. (2015) 36:1102–20. doi: 10.1002/hbm.22690
100. Bernard JA, Orr JM, Mittal VA. Differential motor and prefrontal cerebello-cortical network development: Evidence from multimodal neuroimaging. NeuroImage. (2016) 124:591–601. doi: 10.1016/j.neuroimage.2015.09.022
101. Tiemeier H, Lenroot RK, Greenstein DK, Tran L, Pierson R, Giedd JN. Cerebellum development during childhood and adolescence: a longitudinal morphometric MRI study. Neuroimage. (2010) 49:63–70. doi: 10.1016/j.neuroimage.2009.08.016
Keywords: cerebellum, autism spectrum disorder, sensorimotor, sensory over-responsivity, functional connectivity, fMRI
Citation: Cakar ME, Okada NJ, Cummings KK, Jung J, Bookheimer SY, Dapretto M and Green SA (2024) Functional connectivity of the sensorimotor cerebellum in autism: associations with sensory over-responsivity. Front. Psychiatry 15:1337921. doi: 10.3389/fpsyt.2024.1337921
Received: 13 November 2023; Accepted: 27 February 2024;
Published: 25 March 2024.
Edited by:
Thomas Nickl-Jockschat, The University of Iowa, United StatesReviewed by:
Reubs J. Walsh, University of Toronto, CanadaMarc Thioux, University Medical Center Groningen, Netherlands
Copyright © 2024 Cakar, Okada, Cummings, Jung, Bookheimer, Dapretto and Green. This is an open-access article distributed under the terms of the Creative Commons Attribution License (CC BY). The use, distribution or reproduction in other forums is permitted, provided the original author(s) and the copyright owner(s) are credited and that the original publication in this journal is cited, in accordance with accepted academic practice. No use, distribution or reproduction is permitted which does not comply with these terms.
*Correspondence: Melis E. Cakar, cakarm@g.ucla.edu
†ORCID: Melis E. Cakar, orcid.org/0000-0002-7012-3247