Distinct glycosylation and functional profile of typhoid vaccine-induced antibodies in a UK challenge study and Nepalese children
- 1Oxford Vaccine Group, Department of Paediatrics, NIHR Oxford Biomedical Research Centre, University of Oxford, Oxford, United Kingdom
- 2The Jenner Institute, University of Oxford, Oxford, United Kingdom
- 3Department of Cellular and Molecular Medicine, Faculty of Health and Medical Sciences, Copenhagen Center for Glycomics, University of Copenhagen, Copenhagen, Denmark
- 4Center for Proteomics and Metabolomics, Leiden University Medical Center, Leiden, Netherlands
- 5Oxford University Clinical Research Unit, Patan Academy of Health Sciences, Kathmandu, Nepal
Vaccines against typhoid fever have been shown to be safe and effective in field trials. The mechanism through which the vaccines protect remains elusive. Recent data have implicated antibody glycosylation, and specifically afucosylated antibodies, as an important factor in vaccine-induced effector function for a range of viral infections, however this has not been evaluated for vaccines against bacterial infections such as Salmonella typhi. Here, we studied antibody glycosylation after either Vi-conjugate or Vi-polysaccharide vaccine in a UK cohort who were then challenged with virulent S. typhi, and compared findings to antibody glycosylation after Vi-conjugate vaccine in Nepalese children living in a typhoid endemic region. We compared vaccine-induced responses and correlated these measures with antibody-dependent function. Robust antigen-specific antibody galactosylation and sialylation modifications were induced by both vaccines in UK adults, with Vi-conjugate vaccine inducing Vi-specific glycan changes of higher magnitude than Vi-polysaccharide. Among those individuals diagnosed with typhoid fever after challenge, a distinct glycan profile was correlated with disease severity. Elevated galactosylation and sialylation was correlated with increased antibody-dependent phagocytosis by macrophages and neutrophils among UK adults. While bulk IgG glycosylation differed between Nepalese children and UK adults, vaccination with the Vi-conjugate vaccine overcame these differences to result in similar Vi-specific antibody glycosylation profiles 28 days after vaccination in both cohorts.
Introduction
Typhoid fever is caused by infection with Salmonella Typhi and particularly affects regions of the world with inadequate water supply and poor sanitation. An estimated 11 million people suffer from typhoid fever each year and in 2017 approximately 136,000 people died of the disease (Stanaway et al., 2019). Currently licensed typhoid vaccines have significant limitations. One of the licensed vaccines is Ty21a, an oral live attenuated vaccine, which is unsuitable for administration in young children because the capsular formulation makes it difficult to swallow. The other is an injectable polysaccharide vaccine based on the main immunogenic S. Typhi antigen, Vi (Vi-PS), which is not effective in early childhood.
In 2018 the WHO announced pre-qualification of the first protein conjugate vaccine against typhoid (Typbar TCV) following a successful safety and efficacy trial among UK participants in a controlled human infection model (CHIM) (Jin et al., 2017). This tetanus toxoid-conjugate vaccine (Vi-TCV) also utilises the main immunogenic S. Typhi antigen, Vi. High efficacy of Vi-TCV was confirmed in a Phase 3 trial in 10,005 Nepalese children, both after 1 year (81.6%; 95% CI, 58.8–91.8; (Shakya et al., 2019); and after 2 years (79.0%; 95% CI 61.9–88.5, (Shakya et al., 2021), and additionally in Bangladesh (Qadri et al., 2021) and Malawi (Patel et al., 2021).
Attempts to characterise a measurable correlate of protection which could be used to inform boosting intervals and future vaccine development, found that in healthy UK adults challenged with live S. Typhi, Vi-TCV and Vi-PS vaccinations had distinct protective signatures (Jin et al., 2021)). A combination of Vi-specific Immunoglobulin (Ig) A, avidity, IgG, and Antibody-Dependent Neutrophil Phagocytosis (ADNP) were associated with protection and reduced disease severity after vaccination, however a reliable signature with a protective threshold was not found (Jin et al., 2021). These findings led to this study where alternative characteristics of antibodies were investigated.
Unlike subclass selection, which irreversibly changes the crystallisable fragment (Fc) region of antibodies, glycosylation (the addition of sugar moieties to the CH2 domain of the Fc) represents a mechanism by which the immune system can fine-tune antibody effector function (Thomann et al., 2016). IgG glycosylation involves the post-translational modification of the conserved N-linked glycosylation site at asparagine position 297 of the Fc region of the antibody. This modification is harnessed in therapeutic monoclonal antibody development to improve the management of cancer (Pereira et al., 2018) and autoimmune diseases (Mastrangeli et al., 2019). Manipulation of antibody glycosylation can impact immunoglobulin stability, pharmacokinetics (PK) and, in particular, effector functions by modifying Fcγ receptor (FcγR) binding affinity (Irvine and Alter, 2020). Glycosylation is a dynamic process, dependent on the local availability of enzymes, sugar precursors, and cellular signals. While the majority of the glycans attached to IgG consist of diantennary glycans, large variations between individuals and with pathological conditions are found in four main glycosylation features. These features include the levels of galactosylation and sialylation, and the presence of a core fucose and a bisecting N-acetylglucosamine (GlcNAc). The best-characterised functional immunoglobulin glycan alterations include a loss of fucose which increases IgG affinity for FcyRIIIa and b by up to 17-fold (Shields et al., 2002; Dekkers et al., 2017), and Fc sialylation which plays a role in affinity maturation of antigen-specific IgG1 generated by influenza vaccination (Wang et al., 2015). Antibody glycosylation often forms part of analyses called ‘systems serology’ whereby many measures of antibody features and functions are analysed using computational methods to mine data in an unbiased way in order to better understand immune responses to vaccination and disease (Chung and Alter, 2017).
Recently, differential antibody glycosylation has been associated with differences in antibody function and severity of SARS-CoV-2 (Larsen et al., 2021), as well as between SARS-CoV2 vaccination (Van Coillie et al., 2022) and infection (Farkash et al., 2021). Given the magnitude of functional change possible via alterations of antibody glycosylation state, modifications to vaccination approaches which impact on glycoform populations, could enable fine tuning of resultant immune responses.
To further investigate the correlates of protection of different types of vaccines, we aimed to understand the similarities and differences in vaccine-induced IgG glycosylation and functionality against the same antigenic target (Vi) in two different formulations; Vi-TCV and Vi-PS. We report data from three time points of a Phase 2b S. Typhi vaccine CHIM study in the UK (Jin et al., 2017), and compare Vi-TCV-induced IgG glycosylation between a S. Typhi naïve UK population and an endemic population, using samples from a subset of Nepalese children receiving Vi-TCV in a Phase 3 efficacy study (Shakya et al., 2019). We characterise total IgG Fc glycosylation and compare those measures to Vi-specific IgG Fc glycosylation and measures of function.
Materials and methods
UK phase 2b clinical trial
Stored serum samples from a single-centre, randomised controlled, phase 2b study, using an outpatient-based human typhoid infection model, were used for this study. The clinical trial has been reported by Jin et al. (Jin et al., 2017). Briefly, healthy adult volunteers aged between 18 and 60 years, with no previous history of typhoid vaccination, infection, or prolonged residency in a typhoid-endemic region were recruited. Participants were randomly assigned to receive a single dose of Vi-conjugate (Vi-TCV; Typbar-TCV, Bharat Biotech, Hyderabad, India; n = 37), Vi-polysaccharide (Vi-PS; TYPHIM Vi, Sanofi Pasteur, Lyon, France; n = 35), or a control meningococcal vaccine. Both Vi vaccines contained 25 μg of Vi-antigen per 0.5 ml dose. Approximately 1 month post vaccination, participants ingested live S. Typhi and were assessed with daily blood culture over a 2-week period. Typhoid infection was defined as persistent fever of 38°C or higher for 12 h or longer or S. Typhi bacteraemia. Trial registered with ClinicalTrials.gov, number NCT02324751. A total of 103 participants completed follow-up; 35 in the Vi-PS group, 37 in the Vi-TCV group and 31 in the control arm. Samples used in this study were taken pre-vaccination (D0), 1-month post-vaccination (D28), and 28 days post S. Typhi challenge (PC) from the 35 Vi-PS and 37 Vi-TCV recipients. Overall, 13/35 participants who received Vi-PS and 13/37 who received Vi-TCV were diagnosed with typhoid fever.
Nepal phase 3 clinical trial
Stored plasma samples from a participant-masked and observer-masked individually randomised phase 3 clinical trial of Vi-TCV vaccine in Lalitpur, Nepal were used for this study. With a protective efficacy against blood culture-confirmed typhoid fever at 2 years of 79.0% (95% CI 61.9–88.5), this study enrolled 20,019 participants aged 9 months to 16 years randomised (1:1) to either receive a single dose of Vi-TCV or a control meningococcal vaccine (trial is registered at ISRCTN registry, ISRCTN43385161, (Shakya et al., 2019)). A randomly selected subset of 35 participants with samples taken for immunology were used in this study (mean age 10.1 years (range 0–15 years). Timepoints used in this study are pre-vaccination (D0) and 1 month post vaccination (D28).
Vi-specific IgG capture and glycopeptide analysis
Vi-specific IgG was captured using NeutrAvidin plates (Thermofisher), coated for 2 h at room temperature with 100 µl biotinylated Vi (commercially biotinylated WHO international Standard Vi polysaccharide of C freundii NIBSC code: 12/244) at 1.25 μg/ml in 0.5X PBS. After washing plates three times with 200 µl 0.5X PBS, 200 ul of 1:20 diluted (in 0.5X PBS) sample was added and incubated for 1 hour at room temperature, and then the sample transferred to another Vi-coated blank well for another 1-h incubation. After each incubation, wells were washed once with 200 µl 0.5X PBS and three times with 200 ul freshly made 25 mM ammonium bicarbonate (Sigma). Following the washes, 50 µl of 100 mM formic acid (Sigma) was added and plates were incubated at room temperature for 5 min with agitation. After transferring the 50 µl eluate to a clean V-bottom 96-well plate, another 50 µl 100 mM formic acid was added, incubated for 5 min with agitation and the two eluates from the same sample were pooled.
Samples were dried using a vacuum centrifuge for 2–3 h at 50/60°C, and shipped to LUMC for processing as below for total IgG.
Total IgG glycopeptide analysis
IgG was purified in duplicate using affinity bead chromatography, based on a protocol described previously (Bondt et al., 2014; Plomp et al., 2018). For IgG purification, 2 μl of Protein G Sepharose 4 Fast Flow beads (GE Healthcare) were added per well on an Orochem filter plate and washed three times with PBS. 20 μl of 1:20 diluted sample (in PBS) was added to each well. Plates were incubated for 1 h with shaking at 750 rpm. Using a vacuum manifold, the samples were washed three times by adding 400 μl PBS, followed by three times 400 μl of purified water. Antibodies were eluted from the beads by addition of 100 μl of 100 mM formic acid (Sigma) incubating for 5 min with shaking, and centrifuging at 100 × g for 2 min. Samples were dried for 2 h at 60°C in a vacuum centrifuge before resolubilisation in 20 μl 50 mM ammonium bicarbonate (Sigma) while shaking for 5 min. 20 μl of 0.05 μg/μl tosyl phenylalanyl chloromethyl ketone (TPCK)-treated trypsin (Sigma) in ice-cold purified water was added per well, and the samples were incubated at 37°C overnight.
NanoLC-ESI-QTOF-MS(/MS) analysis
Trypsin-treated IgG samples were analyzed by nanoLC-MS (Ultimate 3000 RSLCnano system; Dionex/Thermo Scientific, Breda, the Netherlands) coupled to a quadrupole-TOF-MS (MaXis HD; Bruker Daltonics, Bremen, Germany) and MS data was acquired as described previously with minor modifications (Falck et al., 2016). The LC system was equipped with an Acclaim PepMap 100 trap column (particle size 5 μm, pore size 100 Å, 100 μm × 20 mm, Dionex/Thermo Scientific) and an Acclaim PepMap C18 nano analytical column (particle size 2 μm, pore size 100 Å, 75 μm × 150 mm, Dionex/Thermo Scientific).
Glycan data processing
Glycopeptides were identified based on their m/z, retention time and literature (Falck et al., 2016). Next to an IgG1 Fc glycopeptide cluster, a joint IgG2 and IgG3 Fc glycopeptide cluster was detected, as the method does not resolve IgG2 and 3. Due to low intensity of IgG4 in the Vi-specific IgG samples, IgG4 was not quantified in the current data. Glycopeptides were quantified using LaCyTools 1.1.0-alpha (build 190207b) software as described previously (Jansen et al., 2016), applying the following quality criteria for analyte quantification: mass error between −10 and 10 ppm, IPQ above 0.35, signal-to-noise above 9 in at least 20% of the samples per cohort. Vi-specific IgG samples were excluded from the analysis when the total absolute abundance of the curated glycopeptides was below the value observed in the negative plasma controls. Total area normalization was performed to obtain relative abundancies of the individual glycoforms per IgG subclass. Supplementary Figures S7, S8 show method repeatability based on a cohort-independent standard (method standard) as well as a cohort-specific Typhoid-positive control (Positive control), showing very high method repeatability over the two cohorts. Derived glycosylation traits galactosylation (gal), sialylation (sia), bisection (bisec) and fucosylation (fuc) were calculated by summing the relative abundances of the respective individual glycans.
The mass spectrometry proteomics data have been deposited to the ProteomeXchange Consortium via the PRIDE partner repository with the dataset identifier PXD037251.
Vi-IgG and IgA ELISA
IgG and IgA Vi-specific antibody titers were measured using a commercial enzyme-linked immunosorbent assay (ELISA) kit (VaccZyme, Binding Site) according to the manufacturer’s instructions for IgG and with modifications for IgA. Data has been published previously (Jin et al., 2017; Shakya et al., 2019; Shakya et al., 2021).
Functional assays
Antibody dependent monocyte, and neutrophil phagocytosis (ADCP, ADNP), neutrophil oxidative burst (ADNOB), NK cell activation (ADNKA), complement deposition (ADCD), and serum bactericidal activity (SBA) were carried out on UK samples at all timepoints studied here and are reported separately by (Jin et al., 2021). ADNP was investigated for Nepalese samples and are reported by (Johnson et al., 2021). Methods for these assays are included in Supplementary Materials.
Serum cytokines
A custom 9-plex Merck Millipore Milliplex® human cytokine detection kit was used for analysis of inflammatory cytokines; Fractaline, IFNγ, GROa, IL10, sCD40L, IL1B, IL8, IP10, and TNFα. Analysis was carried out according to manufacturer’s instructions and bead acquisition and analysis of median fluorescence intensity was performed using Bio-Plex manager software version 6.1.
Statistical analyses
Stata version 14.1 (Stat Crop, Texas), GraphPad Prism 8, R-4.1.2 (https://www.r-project.org/) and SIMON software version 0.2.1 (https://genular.org) were used for statistical analysis, graphical representation of data and integrative analysis (Tomic et al., 2021).
Unless otherwise stated, Kruskal Wallis test was used for comparison of groups with adjustment for multiple testing using Dunn’s multiple comparisons test where appropriate. The Wilcoxon signed rank test was used to explore the differences between total and antigen-specific glycans in paired samples, and between study timepoints. The Kruskal Wallis test was used to explore differences between UK and Nepalese cohorts with respect to age, sex and anti-Vi antibody titres. The Mann-Whitney U test was used to compare the number of diagnosed individuals between the two vaccine arms among UK volunteers, and to explore missing data between independent study arms.
Pairwise correlations were calculated and visualized as a correlogram using SIMON software 0.2.1 (Tomic et al., 2021). Spearman’s rank correlation coefficient was computed and indicated on the correlogram by the heat scale. The significance test of correlation coefficients was performed, and values shown on the correlogram were adjusted for multiple testing using the Benjamini–Hochberg correction at the significance threshold FDR <0.05.
t-SNE and clustering analysis
The t-distributed stochastic neighbour embedding (t-SNE) and clustering was performed to analyse the pre-processed integrated dataset using SIMON software. Visit, study, vaccine, group, diagnosis, and sex were used as grouping variables, and thus, were excluded from the analysis. Additionally, due to the large variation between the two cohorts, age variable was excluded from the analysis.
Before the t-SNE analysis (2,000 iterations, perplexity 30, and theta 0.5), data was pre-processed using transformation methods available in SIMON software (Tomic et al., 2021). Center (mean subtracted) and scale (standard deviation divided), and missing values were imputed based on median values (medianImpute). The generated t-SNE map was analyzed using model-based clustering algorithm (mclust; seed number 1337; 4 clusters allowed) (Fraley and Raftery, 2002). To visualize variation of measured features across the t-SNE embedding space, we performed hierarchical clustering on t-SNE maps using Euclidean distance, agglomerative hierarchical clustering with Ward.
Results
Sera from 72 UK adults with no history of S. Typhi infection were taken before (baseline; D0) and 28 days after (D28) vaccination with a single dose of Vi-PS (n = 35) or Vi-TCV (n = 37), and again 28 days after deliberate ingestion of virulent S. typhi (Post Challenge; PC). Sera from 35 randomly selected Nepalese children (with stratification for sex) from a Phase 3 clinical trial were included, with samples taken at baseline (D0) and 28 days after (D28) vaccination with a single dose of Vi-TCV (Figure 1). In the UK cohort, among the Vi-PS arm, 37% of participants were female, with a mean age of 34 years, and among the Vi-TCV arm, 49% of participants were female, with a mean age of 31.7 years (Table 1). The mean age of the Nepalese children was 10.1 years (Table 1).
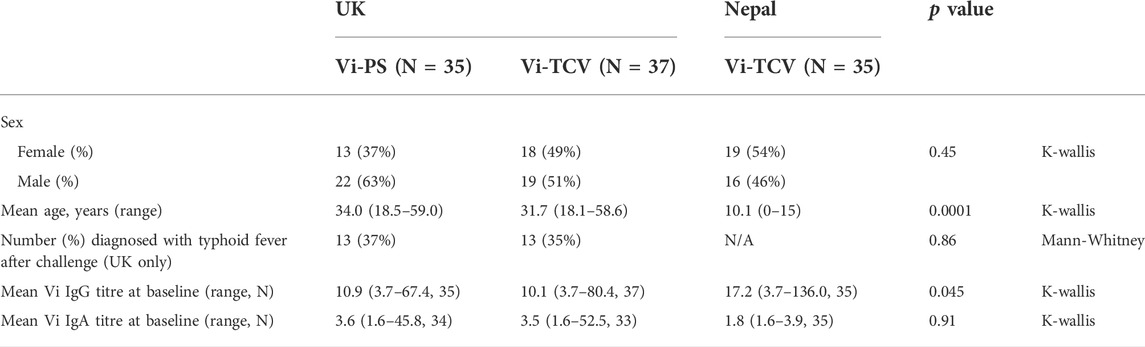
TABLE 1. Cohort demographic and baseline IgG and IgA titres for Vi-PS (UK adults) and Vi-TCV (UK adults and Nepalese children).
Table 2 shows a summary of missing data for Vi-specific IgG glycan measures. The table details the number of samples from which Vi-specific glycosylation data was successfully reported by timepoint. At baseline (D0), only 7/72 (10%) UK participants had measurable Vi-specific IgG glycosylation and this was evenly split between Vi-PS and Vi-TCV vaccine groups. At D28 and after challenge (PC), successful reporting of glycan measures increased (43% for recipients of Vi-PS at both D28 and PC, and 89% and 92% for recipients of Vi-TCV at D28 and PC respectively). Individuals with valid Vi-specific IgG glycan measures had higher Vi IgG titres as measured by Elisa for both vaccine groups (overall the median Vi-IgG titre was 7.9 EU for individuals with missing glycan data, and 423 EU for individuals with valid glycan data, p < 0.0001).
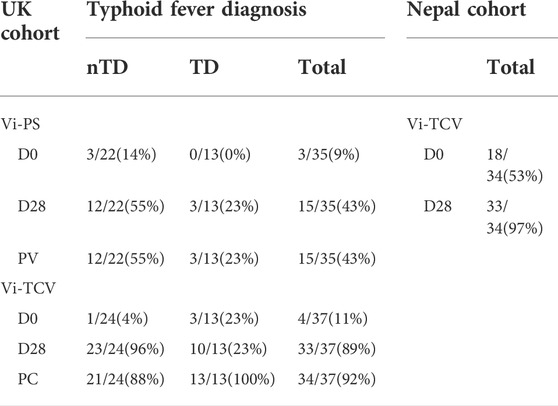
TABLE 2. Summary of missing data for Vi-specific IgG glycan measures among UK Vi-PS and Vi-TCV vaccinees split by challenge outcome, and Nepalese Vi-TCV vaccinees. Table shows the number and percentage of samples (out of total samples analysed) from which Vi-specific glycosylation data was successfully reported by timepoint. nTD - volunteers who remained free of typhoid fever after challenge, TD - participants who were diagnosed with typhoid fever after challenge with virulent S. typhi. Timepoints - D0 - Day 0, baseline (day of vaccination), D28 - Day 28 after vaccination, PC - Post Challenge, 28 days after controlled human infection.
Fifty-one percent of Nepalese infants had detectable Vi-specific IgG glycosylation at baseline (D0) and 97% at D28 post vaccination with Vi-TCV (Table 2). Again, individuals with valid Vi-specific IgG glycan measures had higher Vi igG titres as measured by Elisa (median Vi-IgG titre was 3.7 EU for individuals with missing glycan data, and 1281 EU for individuals with valid glycan data, p < 0.0001).
Partial separation of UK and nepalese cohorts by unsupervised clustering analysis
Visualisation of cohorts using t-SNE plots from integrative analysis of all timepoints for all shared parameters (Figures 2A–D) shows partial separation of Nepalese and UK study participants (Figure 2D) but no separation based on sex (Figure 2B) or vaccine received (Figure 2C). Unsupervised clustering analysis reveals 4 distinct immunophenotypic groups (Figure 2E). Cluster 1 is dominated by individuals from UK cohort having higher total IgG galactosylation (specifically, higher digalactosylated glycans (G2) and a reciprocal decreased level of agalactosylated glycans (G0); Figure 2F). Cluster 2 and 3 are again dominated by UK adults and characterised by a preponderance of intermediate (G1) galactosylated total IgG. Cluster 4 mostly overlaps with the Nepalese cohort (Figure 2E), and the heatmap (Figure 2F) reveals that this cluster is characterised by highly agalactosylated total IgG glycoforms, a high number of sialic acids per galactose residue (for total IgG), and pronounced differences in galactosylation between Vi-specific and total IgG.
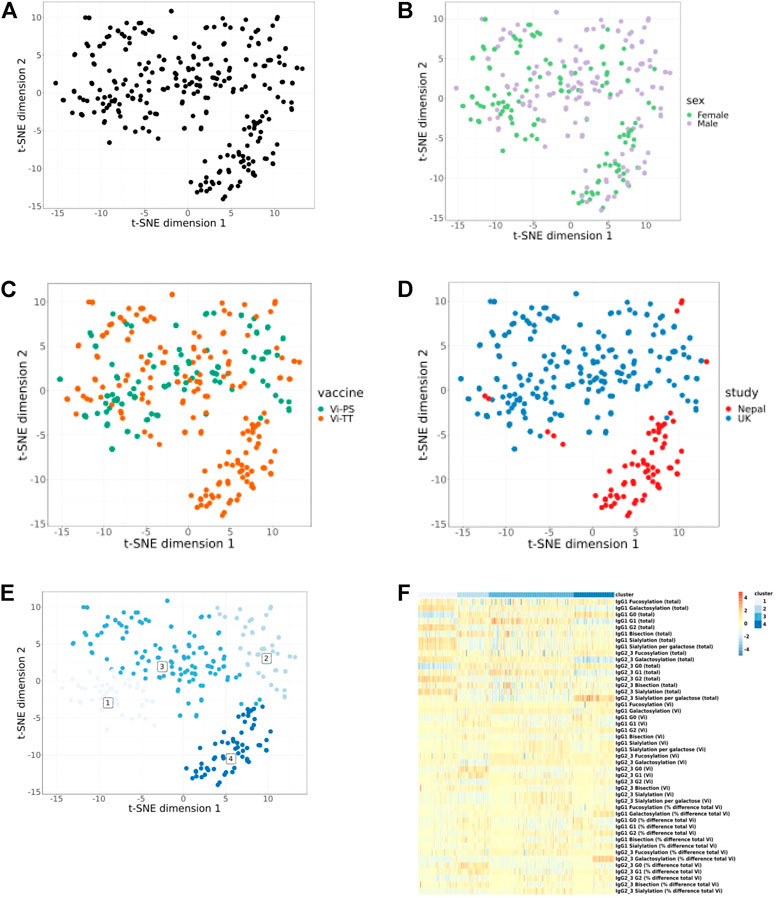
FIGURE 2. Integrative analysis of only shared glycan and immunological parameters for UK and Nepalese cohorts. All timepoints are included. Each dot represents one sample and samples are closer together if they are more similar. All samples (A). No clear separation can be seen when data are split by sex (B) or vaccine type (C). Separation of the UK and Nepalese cohorts can be seen in (D). Unsupervised clustering analysis shows 4 clusters (E) and (F) shows a heat map of cluster attributes. Heat map colour scale bar depicts values from −5 to 5.
Vaccination with Vi-TCV induces vi-specific glycan changes of higher magnitude than Vi-PS among UK vaccinees
Among UK adults, Vi-TCV and Vi-PS vaccines both gave rise to altered glycan traits in Vi-specific IgG compared to those for total IgG at 28 days post-vaccination, with similar directions of effect for the two vaccines (Figure 3). Vi-specific IgG1 is more fucosylated than total IgG1, Vi-specific IgG1 and IgG2/3 are more galactosylated and sialylated than total IgG1 and IgG2/3, and Vi-specific IgG1 and IgG2/3 are less bisected than total IgG1 and IgG2/3 (Figure 3, Supplementary Figure S1). While the direction of the glycosylation effects between total and Vi-specific IgG is the same for Vi-PS and Vi-TCV recipients, Vi-TCV elicits a greater deviation than Vi-PS vaccination for IgG2/3 galactosylation (p = 0.020), IgG1 and IgG2/3 sialylation and bisection (IgG1 p = 0.002 and p = 0.038 and IgG2/3 p = 0.0005 and p = 0.0002 for sialylation and bisection respectively; Figure 3).
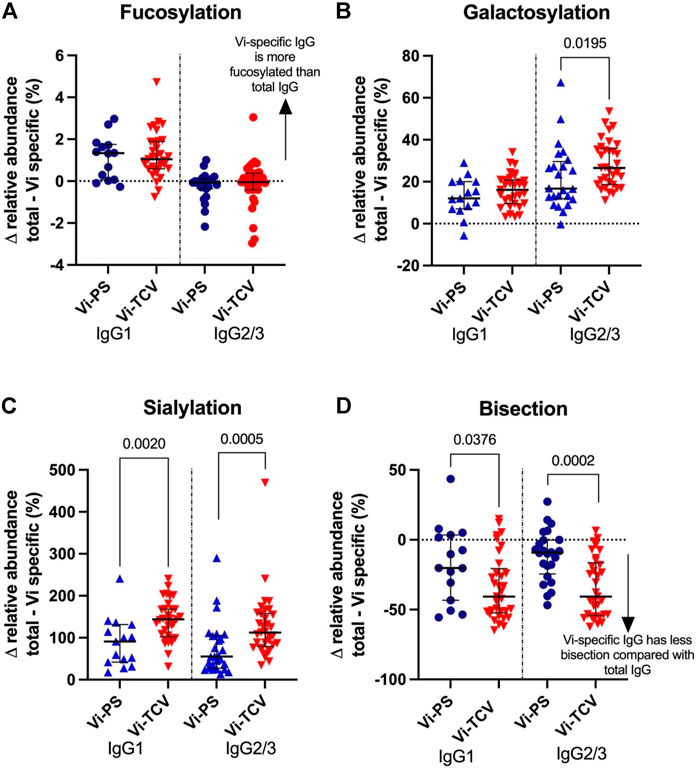
FIGURE 3. Percentage difference in glycan relative abundance between total IgG and Vi-Specific IgG for fucosylation (A), galactosylation (B), sialylation (C) and bisection (D) 28 days post vaccination. Triangles denote a significant difference between total and Vi-specific (blue–Vi-PS (n = 15) and red-TCV (n = 33) vaccinees). Circles denote no significant difference between total and Vi-specific (blue–Vi-PS and red–TCV vaccinees).
Disease severity correlates with a distinct Vi-specific IgG glycosylation profile among UK adults challenged with virulent S.typhi
While there were no differences in Vi-specific antibody glycan relative abundance between individuals who were diagnosed (Vi-TCV 13/37 and Vi-PS 13/35) or not with typhoid fever (Supplementary Figure S2) nor any differential deviation between Vi-specific IgG compared to total IgG (Supplementary Figure S3), there were specific glycan profiles that associated with disease severity among diagnosed individuals.
Glycan profiles of Vi-specific antibodies measured 28 days after vaccination (which was also the day of S. Typhi challenge (D28)) were correlated with measures of disease severity for the UK CHIM participants who were diagnosed with typhoid fever (Figure 4A). Disease severity, as measured by CRP or peak temperature (which were positively correlated with each other; rho = 0.43, p = 0.02) were themselves positively correlated with Vi-specific bisection (IgG1 rho = 0.45 and 0.65, IgG2/3 rho = 0.47 and 0.38, for CRP and peak temperature respectively) and negatively correlated with IgG1 Vi-specific galactosylation and sialylation (rho = −0.30 and −0.38 for galactosylation and rho = −0.38 and −0.42 for sialylation, for CRP and peak temperature respectively; Figure 4A). Bacterial burden at the time of diagnosis (as measured by the number of colony forming units (CFU) cultured from clinical samples) was positively correlated with Vi-specific IgG1 fucosylation (rho = 0.44), galactosylation (IgG1 rho = 0.74, IgG2/3 rho = 0.44)) and sialylation (IgG1 rho = 0.63, IgG2/3 rho = 0.39) and negatively correlated with bisection (IgG1 rho = −0.55, IgG2/3 rho = −0.44). Where correlations were statistically significant for all measures, the direction of correlation of glycan traits with measures of inflammation (CRP and peak temperature) were the opposite of the correlations between glycans and the ability of the pathogen to replicate (measured by CFU/ml; Figure 4A despite neither CRP and CFU, nor peak temperature and CFU having significant correlations (0.3127 p = 0.1463 and rho = 0.019 p = 0.9315 respectively).
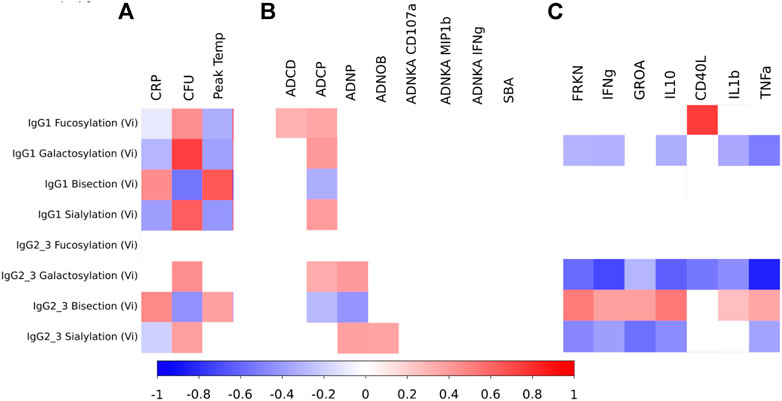
FIGURE 4. (A) Heat map showing correlations between measures of disease severity and Vi-specific IgG1 and IgG2/3 glycosylation as measured 28 days after vaccination among the UK individuals that were diagnosed with typhoid fever after challenge (and for whom glycosylation data was available at D28) (n = 13; 3 for Vi-PS and 10 for Vi-TCV). (B) Heat map showing correlations between measures of antibody functionality and Vi-specific IgG1 and IgG2/3 glycosylation as measured 28 days after vaccination for all UK individuals (whether diagnosed or not with typhoid fever) for whom glycosylation data was available at D28 post vaccination (n = 15 for Vi-PS and n = 33 for Vi-TCV; total n = 48). (C) Heat map showing correlations between circulating cytokines and Vi-specific IgG1 and IgG2/3 glycosylation as measured 28 days after vaccination for all UK individuals for whom both cytokine and glycosylation data was available at D28 post vaccination (n = 20 for IgG1 measures and n = 24 for IgG2/3 measures). The scale shows spearman rho values ranging from -1 to 1. Only significant (p < 0.05) findings are coloured.
Antibody-dependent phagocytosis correlates with increased Vi-specific antibody galactosylation and sialylation and coincides with lower circulating inflammatory cytokines
Pair-wise correlations between Vi-specific IgG glycosylation and measures of antibody function were investigated by correlating the glycan profile at 28 days post vaccination, and antibody function at 28 days post vaccination (Figure 4B). Among UK participants receiving either Vi-PS or Vi-TCV vaccines, Vi-specific IgG fucosylation, galactosylation and sialylation were positively correlated with functional properties involving phagocytic cells. Specifically, fucosylation of IgG1, galactosylation of both IgG1 and IgG2/3 and sialylation of IgG1 were positively associated with phagocytosis by macrophages (ADCP). Similarly, galactosylation and sialylation of IgG2/3 was positively correlated with neutrophil phagocytosis (ADNP) and IgG2/3 sialylation with neutrophil oxidative burst (ADNOB). IgG1 fucosylation was also positively associated with antibody-dependent complement deposition (ADCD). Conversely, Vi-specific IgG bisection negatively correlated with phagocytosis by macrophages (ADCP) and neutrophils (ADNP; Figure 4B). No glycan state showed an association with any measures of NK cell activation (ADNKA) or serum bactericidal activity (SBA) (Figure 4B).
To investigate the impact of Vi antibody titres upon the relationship between glycan and functionality, a multivariable regression model was run for all significant findings in Figure 4B, to include Vi IgG titre tertile (low; mean 76.23 EU, medium; mean 333.23EU and high; mean 1427.15EU). After adjusting for levels of Vi IgG, all associations between glycan and function remained statistically significant however the regression coefficients were reduced. Therefore, the association between glycan and functionality was partially, but not completely explained by Vi antibody level.
Among UK volunteers for whom both glycan and cytokine measures were available at 28 days post vaccination, all cytokines investigated were negatively correlated with Vi-specific IgG2/3 galactosylation, and all except GROA (CXCL1) and CD40L were negatively correlated with Vi-specific IgG1 galactosylation (Figure 4C). All except CD40L were positively correlated with Vi-specific IgG2/3 bisection and all except CD40L and IL1b were negatively associated with Vi-specific IgG2/3 sialylation (Figure 4C). CD40L was positively associated with Vi-specific IgG1 fucosylation.
Vi-TCV vaccination induces Vi-specific glycan profiles among nepalese children
Aside from the different ages of participants in the UK (n = 72, mean age 32.8 (range 18.1–59.0)) and Nepal (n = 35, 10.1 (range 0–15); p < 0.001; Table 1), the cohorts were comparable in terms of sex and baseline Vi-specific IgA antibody titre. Vi-specific IgG antibody titre was slightly higher in the Nepal participants (Table 1). In terms of glycosylation, the Nepalese cohort displayed lower baseline levels of total IgG galactosylation (IgG1 median Nepal (n = 35) 71.4%, median UK (n = 72) 79.4%, p < 0.0001, IgG2/3 median Nepal (n = 35) 42.9%, median UK (n = 72) 69.1%, p < 0.0001, (Supplementary Figure S4), consistent with previous observations between IgG Fc galactosylation and life circumstances, urbanization and economic development (De Jong et al., 2016). Furthermore, a minor difference in total IgG fucosylation (higher in Nepalese children) was observed, an effect that can be explained by the age difference between the cohorts (De Haan et al., 2016).
Vi-TCV vaccine gave rise to altered glycan traits in Vi-specific IgG compared to those for total IgG at 28 days post-vaccination in the same direction for both UK adults and Nepalese children. Vi-specific IgG1 is more fucosylated than total IgG1, Vi-specific IgG1 and IgG2/3 are more galactosylated and sialylated than total IgG1 and IgG2/3, and Vi-specific IgG1 and IgG2/3 are less bisected than total IgG1 and IgG2/3 (Figure 5). The magnitude of difference between Vi-specific IgG and total IgG was similar between the UK and Nepalese cohorts with the notable exception of galactosylation. Nepalese children had a greater difference between Vi-specific and total IgG galactosylation compared with UK adults (Figure 5) due to the lower levels of total IgG galactosylation among Nepalese children. At 28 days after vaccination with Vi-TCV, there were no differences in the levels of Vi-specific IgG1 or 2/3 galactosylation between the UK and Nepalese cohorts (Supplementary Figure S5).
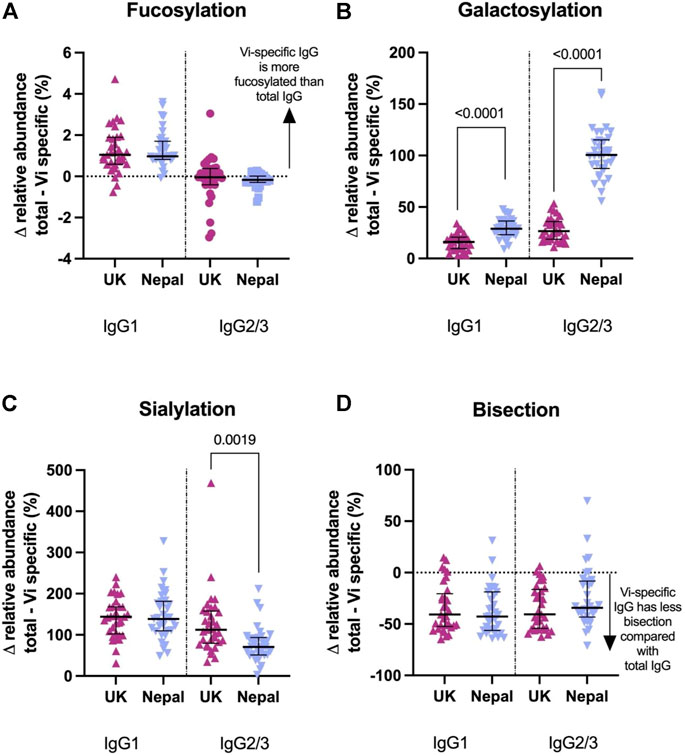
FIGURE 5. Percentage difference in glycan relative abundance between total IgG and Vi-Specific IgG among UK Vi-TCV recipients (n = 33) and Nepalese Vi-TCV recipients (n = 33), 28 days post vaccination. Fucosylation (A), galactosylation (B), sialylation (C) and bisection (D). Triangles denote a significant difference between total and Vi-specific glycan trait, Circles denote no significant difference between total and Vi-specific (Blue–Nepal. Purple—UK).
The only measure of function that was shared between UK adults and Nepalese children is ADNP (Supplementary Figure S5). An association has previously been reported between a lower percentage Vi-specific IgG1 and IgG2/3 sialylation and galactosylation, with increased induction of ADNP 28 days after Vi-TCV vaccination in this Nepalese cohort (Johnson et al., 2021). Here, we do not see any similar associations between sialylation/galactosylation and ADNP among the UK Vi-TCV recipients (Supplementary Figure S6).
Discussion
Here, in samples from vaccine clinical trials run in the UK and Nepal (including controlled human challenge with virulent S. typhi in UK volunteers), we saw no changes in the glycosylation profile of total circulating IgG for either cohort after vaccination with Vi-PS (UK) or Vi-TCV (UK and Nepal). This finding is in line with data from other vaccines (Selman et al., 2012; Vestrheim et al., 2014). Nor do we see changes in total IgG after challenge with virulent S. Typhi in the UK volunteers. Since the antibodies produced in response to vaccines likely constitute a tiny fraction of the bulk circulating immunoglobulins, and exposure to S. Typhi in a challenge model does not result in induction of Vi-specific IgG (Waddington et al., 2014), this finding is unsurprising but nonetheless an important observation, and the first such following a controlled human challenge.
Changes in antibody glycosylation are associated with a variety of physiological states, including pregnancy and autoimmune diseases such as rheumatoid arthritis (Arnold et al., 2007). Factors which shape Fc-glycan profiles are not well understood. However, as an important regulator of humoral immune activity, manipulation of glycosylation has been used to fine tune monoclonal antibody function for increased cellular cytotoxicity, extended half-life and a range of other pharmacokinetic features (Reichert et al., 2016). Most is known about functional attributes associated with a lack of core fucose on IgG which are known to cause elevated antibody dependent cellular cytotoxicity (ADCC) via increased IgG-Fc receptor IIIa (FcγRIIIa) affinity (Shields et al., 2002; Ferrara et al., 2011) but also elevated galactosylation and sialylation, independent of fucosylation changes, are associated with increased C1q binding and downstream complement deposition (Dekkers et al., 2017). Specifically, it has been found that galactosylation induces hexamerization of human IgG1 which enhances the classical complement cascade (van Osch et al., 2021). While findings from in vitro studies of glycoengineered monoclonal antibodies are critical to understanding the mechanism by which glycan changes can affect function, less is known about in vivo alterations in an infection or vaccination setting.
While most serum IgG antibodies are highly fucosylated at birth (∼98%) and slightly decrease to ∼94% fucosylation in adulthood (De Haan et al., 2016), some antibodies, such as alloantibodies against foetal red blood cells and platelets, show very low (down to 10%) IgG-Fc-fucosylation (Wuhrer et al., 2009; Kapur et al., 2014). A growing body of data points towards induction of afucosylated antibodies produced in response to infection or vaccination specifically with surface epitopes of enveloped viruses, but not for non-surface exposed antigens or for non-enveloped viruses and protein subunit vaccines (Larsen et al., 2021). In this study, antibodies specific to the Vi vaccine antigen have a different glycosylation profile to bulk IgG antibodies 1 month after vaccination. The same antigen administered in different contexts (unmodified polysaccharide or conjugated to a protein) gives rise to antigen-specific IgG Fc-glycan profiles skewed from bulk IgG to different degrees, despite both vaccines eliciting similar protection following experimental infection in the UK cohort, and robust vaccine-specific antibody responses (Jin et al., 2017). Here we saw high levels (∼98%) of total IgG1 fucosylation, and even higher levels (∼99%) of vaccine-specific IgG1 fucosylation after vaccination using the surface-exposed bacterial polysaccharide Vi antigen in two different formulations in the UK cohort, and the same was seen after Vi-TCV in the Nepalese cohort. This finding is in keeping with the observation that highly afucosylated antibodies were not seen in response to inactivated influenza, pneumococcal, meningococcal or tetanus vaccines (Selman et al., 2012; Vestrheim et al., 2014). Vaccination with mRNA SARS-CoV19 elicited higher levels of afucosylated anti-spike IgG in infection-naïve individuals than virus-exposed people, and levels of afucosylation correlated with post-boost anti-spike IgG levels (Van Coillie et al., 2022). Here, we did not see a significant alteration of the glycan/functionality relationship when Vi antibody titre was added into regression analyses.
The same Vi-TCV vaccine administered to two populations with different ages, and pre-vaccination pathogen exposure (UK adults and Nepalese children) gave rise to similar Vi-specific glycosylation relative abundance 28 days post vaccination despite very different baseline total IgG galactosylation profile. The low total IgG galactosylation among Nepalese children is likely due to generally increased immune activation, and specifically, prevalence of parasitic infections (de Jong et al., 2021). The dynamic immune environment provided by circulating cytokines at the time of antibody production is likely to be influential in the control of antibody glycosylation in vivo. Although we do not have data on inflammatory cytokines for the Nepalese cohort, for the UK cohort, we see an overwhelmingly positive association between cytokines associated with chemoattraction and immune activation measured 28 days post vaccination, with decreased Vi-specific IgG1 and IgG2/3 galactosylation. In turn, these cytokines are also negatively correlated with IgG2/3 sialyation which is unsurprising, given that galactosylation is a prerequisite for sialylation. A major caveat in the cytokine/glycan relationship is that the half-life of IgG is approximately 21 days (Vidarsson et al., 2014), and so the cytokines circulating at the time of glycoform measurement is likely not representative of the immune environment when the antibodies being measured were actually produced by plasma cells. A detailed time course would be able to distinguish any temporal differences, but that was beyond the scope of this study.
In this study, we did not see a link between Vi-specific antibody glycosylation and development of typhoid fever following experimental challenge. For individuals diagnosed with typhoid fever, we did however see a negative correlation between disease severity (as measured by CRP and peak temperature) and galactosylation and sialylation, and a positive correlation between disease severity and bisection. This finding was the opposite of what was seen for the ability of the bacteria to replicate (as measured by CFU/ml). With many infectious diseases a fine balance exists between sufficient magnitude of host immune response required to clear a pathogen, and too much response which can lead to host immune-pathology and pathogen escape. The high levels of sialylation of intravenous immunoglobulin (IVIG) are the determinant of Fc-type II FcR binding (CD23 and DC-SIGN) and are the reason for the anti-inflammatory qualities of IVIG (Anthony et al., 2008a; Anthony et al., 2008b; Anthony and Ravetch, 2010). In keeping with this, here, we see sialylation to be associated with lower CRP and peak temperature but also with poorer control of bacteria among UK individuals who developed disease after infection. Additionally, we see a consistently clear co-dependent profile of galactosylation and sialylation, due to the dependence of terminal sialylic acid upon the presence of galactose in the glycan structure (Reily et al., 2019).
Associations seen between antibody-specific glycoforms and antibody-dependent neutrophil phagocytosis (ADNP), reported as an important component of a protective signature among UK vaccine participants who underwent controlled infection with virulent S. Typhi (Jin et al., 2021), were different between UK and Nepalese cohorts. A causal link between glycan alterations and neutrophil function is plausible; addition of galactose to a monoclonal antibody increased affinity for all FcγRII (CD32) which are highly expressed on neutrophils (Subedi and Barb, 2016). Many possible confounders such as antibody affinity, subclass and subtype were not investigated here. Additionally, the ADNP assay was not carried out on all samples concurrently. Since this assay uses fresh human cells as the source of neutrophils, the fact that different donor cells were used is likely a significant source of variation.
Human challenge models, while useful controlled experimental settings for evaluating correlates of vaccine-induced protection, and for intensive collection of clinical data, are not able to recapitulate many aspects of natural S. Typhi infection. The important differences between the cohorts studied here, including age, pre-existing pathogen exposure, and the baseline total IgG galactosylation already mentioned, are compounded by the lack of consistency in the time interval between vaccination and infection and the dose of challenge or natural inoculum. Given this, it is difficult is disentangle the differences seen between the two cohorts with respect to their glycosylation profile and antibody function. Further investigation of these antibody characteristics as a nested case control study of Nepalese children who were diagnosed, or not, with typhoid fever as part of the Phase 3 clinical trial would be ideal to tease these apart.
In summary, while antibody glycosylation in response to viral infections and vaccines has been studied extensively, far less is known about bacterial infections and vaccines. While this study does not find a robust correlate of vaccine-induced protection in IgG antibody glycosylation, it adds important data to the literature in this field. This study is the first to show a greater magnitude vaccine-specific glycosylation skewing for the same vaccine antigen in a protein-conjugated form compared to that of a polysaccharide formulation. It is also the first report of antibody glycosylation in a controlled human challenge model; we show that infection with virulent S. typhi does not alter vaccine-specific IgG glycosylation. Vi-specific IgG glycosylation at challenge is not clearly associated with disease outcome but a clear profile is associated with severity if diagnosed with typhoid fever in the UK cohort. Finally, we show a consistent vaccine-induced skewing when two very different cohorts were vaccinated with the conjugated Vi vaccine, and the vaccine was able to overcome the large differences in total antibody galactosylation between the UK and Nepalese cohorts. The role of Fc glycoform modulation during evolution of humoral immune responses is an exciting prospect for vaccine development. The highly complex relationships between IgG glycoforms and functionality and how to manipulate those, require further research but are likely influenced by a range of factors including cohort demographics, baseline exposure and immunity, vaccine type and adjuvant, as well as the inflammatory environment in which plasma cells mature. Controlled human challenge studies, paired with natural infection studies in pathogen-endemic regions could be useful going forward to tease apart these highly complex interactions.
Data availability statement
The mass spectrometry proteomics data have been deposited to the ProteomeXchange Consortium via the PRIDE partner repository with the dataset identifier PXD037251.
Ethics statement
The studies involving human participants were reviewed and approved by UK study—University of Oxford and the South Central Oxford A Ethics Committee (14/SC/1427) and the Medicines and Healthcare Products Regulatory Agency (Eudract 2014-002978-36) Nepal study—Oxford Tropical Research Ethics Committee (OxTREC 15–17) and the Nepal Health Research Council (NHRC 170/2017). Written informed consent to participate in this study was provided by the participants’ legal guardian/next of kin.
Author contributions
LS conceived the study and wrote the manuscript with input from co-authors. LS, NdH, EJ, MJ, CJ, JH, MV, JN, SP-M, and CK performed laboratory analyses under the direction of MW and AP. BB, MS, and DP were responsible for clinical sample collection in Nepal. CJ was responsible for clinical sample collection in the UK. LS and AT performed statistical analyses.
Funding
The UK CHIM study was funded by the Bill and Melinda Gates Foundation (OPP1084259) and European Commission Seventh Framework Programme grant “Advanced Immunization Technologies” with support from the National Institute for Health Research Oxford Biomedical Research Centre. The Phase 3 efficacy study in Nepal was funded by the Bill and Melinda Gates Foundation; Current Controlled Trials number, ISRCTN43385161. This work was supported by the GCRF Networks in Vaccines Research and Development which was co-funded by the MRC and BBSRC.
Conflict of interest
The authors declare that the research was conducted in the absence of any commercial or financial relationships that could be construed as a potential conflict of interest.
Publisher’s note
All claims expressed in this article are solely those of the authors and do not necessarily represent those of their affiliated organizations, or those of the publisher, the editors and the reviewers. Any product that may be evaluated in this article, or claim that may be made by its manufacturer, is not guaranteed or endorsed by the publisher.
Supplementary material
The Supplementary Material for this article can be found online at: https://www.frontiersin.org/articles/10.3389/frans.2022.1005558/full#supplementary-material.
References
Anthony, R., Nimmerjahn, F., Ashline, D., Reinhold, V., Paulson, J., and Ravetch, J. (2008). Recapitulation of IVIG anti-inflammatory activity with a recombinant IgG F. Science 320 (5874), 373–376. doi:10.1126/science.1154315
Anthony, R. M., and Ravetch, J. V. (2010). A novel role for the IgG Fc glycan: The anti-inflammatory activity of sialylated IgG fcs. J. Clin. Immunol. 30, S9–S14. doi:10.1007/s10875-010-9405-6
Anthony, R. M., Wermeling, F., Karlsson, M. C. I., and Ravetch, J. V. (2008). Identification of a receptor required for the anti-inflammatory activity of IVIG. Proc. Natl. Acad. Sci. U. S. A. 105 (50), 19571–19578. Available at: http://www.pnas.org/cgi/doi/10.1073/pnas.0810163105.
Arnold, J. N., Wormald, M. R., Rudd, P. M., Sim, R. B., and Dwek, R. A. (2007). The impact of glycosylation on the biological function and structure of human immunoglobulins. Annu. Rev. Immunol. 25 (1), 21–50. doi:10.1146/annurev.immunol.25.022106.141702
Bondt, A., Rombouts, Y., Selman, M. H. J., Hensbergen, P. J., Reiding, K. R., Hazes, J. M. W., et al. (2014). Immunoglobulin G (IgG) fab glycosylation analysis using a new mass spectrometric high-throughput profiling method reveals pregnancy-associated changes. Mol. Cell. Proteomics 13 (11), 3029–3039. doi:10.1074/mcp.M114.039537
Chung, A. W., and Alter, G. (2017). Systems serology: Profiling vaccine induced humoral immunity against HIV. Retrovirology 14 (1), 57–11. doi:10.1186/s12977-017-0380-3
De Haan, N., Reiding, K. R., Driessen, G., Van Der Burg, M., and Wuhrer, M. (2016). Changes in healthy human IgG Fc-glycosylation after birth and during early childhood. J. Proteome Res. 15 (6), 1853–1861. doi:10.1021/acs.jproteome.6b00038
De Jong, S. E., Selman, M. H. J., Adegnika, A. A., Amoah, A. S., Van Riet, E., Kruize, Y. C. M., et al. (2016). IgG1 Fc N-glycan galactosylation as a biomarker for immune activation. Sci. Rep. 6, 1–9. doi:10.1038/srep28207
de Jong, S. E., van Unen, V., Manurung, M. D., Stam, K. A., Goeman, J. J., Jochems, S. P., et al. (2021). Systems analysis and controlled malaria infection in Europeans and Africans elucidate naturally acquired immunity. Available at: http://www.nature.com/articles/s41590-021-00911-7.
Dekkers, G., Treffers, L., Plomp, R., Bentlage, A. E. H., Boer, M. de, Koeleman, C. A. M., et al. (2017). Decoding the human immunoglobulin G-glycan repertoire reveals a spectrum of Fc-receptor- and complement-mediated-effector activities. Front. Immunol. 8, 877. doi:10.3389/fimmu.2017.00877
Falck, D., Jansen, B. C., de Haan, N., and Wuhrer, M. (2016). “High-throughput analysis of IgG Fc glycopeptides by LC-MS,” in High-throughput Glycomics and glycoproteomics, 31–47.
Farkash, I., Feferman, T., Cohen-Saban, N., Avraham, Y., Morgenstern, D., Mayuni, G., et al. (2021). Anti-SARS-CoV-2 antibodies elicited by COVID-19 mRNA vaccine exhibit a unique glycosylation pattern. Cell Rep. 37 (11), 110114. doi:10.1016/j.celrep.2021.110114
Ferrara, C., Grau, S., Jäger, C., Sondermann, P., Brünker, P., Waldhauer, I., et al. (2011). Unique carbohydrate-carbohydrate interactions are required for high affinity binding between FcgammaRIII and antibodies lacking core fucose. Proc. Natl. Acad. Sci. U. S. A. 108 (31), 12669–12674. doi:10.1073/pnas.1108455108
Fraley, C., and Raftery, A. E. (2002). Model-based clustering, discriminant analysis, and density estimation. J. Am. Stat. Assoc. 97 (458), 611–631. doi:10.1198/016214502760047131
Irvine, E. B., and Alter, G. (2020). Understanding the role of antibody glycosylation through the lens of severe viral and bacterial diseases. Glycobiology 30 (4), 241–253. doi:10.1093/glycob/cwaa018
Jansen, B., Falck, D., de Haan, N., Hipgrave Ederveen, A. L., Razdorov, G., Lauc, G., et al. (2016). LaCyTools: A targeted liquid Chromatography−Mass SpectrometryData processing package for relative quantitation of glycopeptides. J. Proteome Res. 15, 2198–2210. doi:10.1021/acs.jproteome.6b00171
Jin, C., Gibani, M. M., Moore, M., Juel, H. B., Jones, E., Meiring, J., et al. (2017). Efficacy and immunogenicity of a vi-tetanus toxoid conjugate vaccine in the prevention of typhoid fever using a controlled human infection model of Salmonella typhi: A randomised controlled, phase 2b trial. Lancet 390 (10111), 2472–2480. doi:10.1016/S0140-6736(17)32149-9
Jin, C., Hill, J., Gunn, B. M., Yu, W. H., Dahora, L. C., Jones, E., et al. (2021). Vi-specific serological correlates of protection for typhoid fever. J. Exp. Med. 218 (2), e20201116. doi:10.1084/jem.20201116
Johnson, M., Stockdale, L., de Haan, N., Wuhrer, M., Nouta, J., Koeleman, C. A. M., et al. (2021). Association of antibody-dependent neutrophil phagocytosis with distinct antibody glycosylation profiles following typhoid vaccination. Front. Trop. Dis. 2, 2. doi:10.3389/fitd.2021.742804
Kapur, R., Kustiawan, I., Vestrheim, A., Koeleman, C. A. M., Visser, R., Einarsdottir, H. K., et al. (2014). A prominent lack of IgG1-Fc fucosylation of platelet alloantibodies in pregnancy. Blood 123 (4), 471–480. doi:10.1182/blood-2013-09-527978
Larsen, M. D., Lopez-Perez, M., Dickson, E. K., Ampomah, P., Tuikue Ndam, N., Nouta, J., et al. (2021). Afucosylated Plasmodium falciparum-specific IgG is induced by infection but not by subunit vaccination. Nat. Commun. 12, 5838. t. doi:10.1038/s41467-021-26118-w
Mastrangeli, R., Palinsky, W., and Bierau, H. (2019). Glycoengineered antibodies: Towards the next-generation of immunotherapeutics. Glycobiology 29 (3), 199–210. doi:10.1093/glycob/cwy092
Patel, P. D., Patel, P., Liang, Y., Meiring, J. E., Misiri, T., Mwakiseghile, F., et al. (2021). Safety and efficacy of a typhoid conjugate vaccine in Malawian children. N. Engl. J. Med. 385, 1104–1115. doi:10.1056/NEJMoa2035916
Pereira, N. A., Chan, K. F., Lin, P. C., and Song, Z. (2018). The “less-is-more” in therapeutic antibodies: Afucosylated anti-cancer antibodies with enhanced antibody-dependent cellular cytotoxicity. MAbs 10 (5), 693–711. doi:10.1080/19420862.2018.1466767
Plomp, R., De Haan, N., Bondt, A., Murli, J., Dotz, V., and Wuhrer, M. (2018). Comparative glycomics of immunoglobulin A and G from saliva and plasma reveals biomarker potential. Front. Immunol. 9, 2436. doi:10.3389/fimmu.2018.02436
Qadri, F., Khanam, F., Liu, X., Theiss-Nyland, K., Biswas, P. K., Bhuiyan, A. I., et al. (2021). Protection by vaccination of children against typhoid fever with a vi-tetanus toxoid conjugate vaccine in urban Bangladesh: A cluster-randomised trial. Lancet 398 (10301), 675–684. doi:10.1016/S0140-6736(21)01124-7
Reily, C., Stewart, T. J., Renfrow, M. B., and Novak, J. (2019). Glycosylation in health and disease. Nat. Rev. Nephrol. 15 (6), 346–366. doi:10.1038/s41581-019-0129-4
Selman, M. H. J., de Jong, S. E., Soonawala, D., Kroon, F. P., Adegnika, A. A., Deelder, A. M., et al. (2012). Changes in antigen-specific IgG1 Fc N -glycosylation upon influenza and tetanus vaccination. Mol. Cell. Proteomics 11 (4), M111014563. doi:10.1074/mcp.M111.014563
Shakya, M., Colin-Jones, R., Theiss-Nyland, K., Voysey, M., Pant, D., Smith, N., et al. (2019). Phase 3 efficacy analysis of a typhoid conjugate vaccine trial in Nepal. N. Engl. J. Med. 381 (23), 2209–2218. doi:10.1056/NEJMoa1905047
Shakya, M., Maharjan, A. M., Dahal Mph, A., Haque PharmD, N., Pradhan, A., Shrestha Mph, S., et al. (2021). Efficacy of typhoid conjugate vaccine in Nepal: Final results of a phase 3, randomised, controlled trial. Available at: www.thelancet.com/lancetghVol.
Shields, R. L., Lai, J., Keck, R., O’Connell, L. Y., Hong, K., Gloria Meng, Y., et al. (2002). Lack of fucose on human IgG1 N-linked oligosaccharide improves binding to human Fcgamma RIII and antibody-dependent cellular toxicity. J. Biol. Chem. 277 (30), 26733–26740. doi:10.1074/jbc.M202069200
Stanaway, J. D., Reiner, R. C., Blacker, B. F., Goldberg, E. M., Khalil, I. A., Troeger, C. E., et al. (2019). The global burden of typhoid and paratyphoid fevers: A systematic analysis for the global burden of disease study 2017. Lancet. Infect. Dis. 19 (4), 369–381. doi:10.1016/S1473-3099(18)30685-6
Subedi, G. P., and Barb, A. W. (2016). The immunoglobulin G1 N-glycan composition affects binding to each low affinity Fc γ receptor. MAbs 8 (8), 1512–1524. doi:10.1080/19420862.2016.1218586
Thomann, M., Reckermann, K., Reusch, D., Prasser, J., and Tejada, M. L. (2016). Fc-galactosylation modulates antibody-dependent cellular cytotoxicity of therapeutic antibodies. Mol. Immunol. 73, 69–75. doi:10.1016/j.molimm.2016.03.002
Tomic, A., Tomic, I., Waldron, L., Geistlinger, L., Kuhn, M., Spreng, R. L., et al. (2021). Simon: Open-source knowledge discovery platform. Patterns 2 (1), 100178. doi:10.1016/j.patter.2020.100178
Van Coillie, J., Pongracz, T., Rahmoller, J., Chen, H.-J., Geyer, C., van Vlught, L. A., et al. (2022). The BNT162b2 mRNA SARS-CoV-2 vaccine induces transient afucosylated IgG1 in naive but not antigen-experienced vaccinees. bioRxiv. doi:10.1101/2022.02.14.480353
van Osch, T. L. J., Nouta, J., Derksen, N. I. L., van Mierlo, G., van der Schoot, C. E., Wuhrer, M., et al. (2021). Fc galactosylation promotes hexamerization of human IgG1, leading to enhanced classical complement activation. J. Immunol. 207 (6), 1545–1554. doi:10.4049/jimmunol.2100399
Vestrheim, A. C., Moen, A., Egge-Jacobsen, W., Reubsaet, L., Halvorsen, T. G., Bratlie, D. B., et al. (2014). A pilot study showing differences in glycosylation patterns of igg subclasses induced by pneumococcal, meningococcal, and two types of influenza vaccines. Immun. Inflamm. Dis. 2 (2), 76–91. doi:10.1002/iid3.22
Vidarsson, G., Dekkers, G., and Rispens, T. (2014). IgG subclasses and allotypes: From structure to effector functions. Front. Immunol. 5, 1–17. doi:10.3389/fimmu.2014.00520
Waddington, C. S., Darton, T. C., Jones, C., Haworth, K., Peters, A., John, T., et al. (2014). An outpatient, ambulant-design, controlled human infection model using escalating doses of Salmonella Typhi challenge delivered in sodium bicarbonate solution. Clin. Infect. Dis. 58 (9), 1230–1240. doi:10.1093/cid/ciu078
Wang, T. T., Maamary, J., Tan, G. S., Bournazos, S., Davis, C. W., Krammer, F., et al. (2015). Anti-HA glycoforms drive B cell affinity selection and determine influenza vaccine efficacy. Cell 162 (1), 160–169. doi:10.1016/j.cell.2015.06.026
Keywords: typhoid, antibody glycosylation, challenge study, vaccine, enteric fever
Citation: Stockdale LK, de Haan N, Hill J, Johnson M, Tomic A, Wuhrer M, Jones E, Jin C, Nouta J, Koeleman CAM, Verheul M, Basnyat B, Shakya M, Pant D, Provstgaard-Morys S and Pollard AJ (2022) Distinct glycosylation and functional profile of typhoid vaccine-induced antibodies in a UK challenge study and Nepalese children. Front. Anal. Sci. 2:1005558. doi: 10.3389/frans.2022.1005558
Received: 28 July 2022; Accepted: 13 October 2022;
Published: 01 November 2022.
Edited by:
Liang Qiao, Fudan University, ChinaReviewed by:
Ruo-Can Qian, East China University of Science and Technology, ChinaRutan Zhang, University of Washington, United States
Ling Lin, Xiamen University Affiliated Cardiovascular Hospital, China
Copyright © 2022 Stockdale, de Haan, Hill, Johnson, Tomic, Wuhrer, Jones, Jin, Nouta, Koeleman, Verheul, Basnyat, Shakya, Pant, Provstgaard-Morys and Pollard. This is an open-access article distributed under the terms of the Creative Commons Attribution License (CC BY). The use, distribution or reproduction in other forums is permitted, provided the original author(s) and the copyright owner(s) are credited and that the original publication in this journal is cited, in accordance with accepted academic practice. No use, distribution or reproduction is permitted which does not comply with these terms.
*Correspondence: L. K. Stockdale, lisa.stockdale@ndm.ox.ac.uk