Advances in graphene-based electrochemical biosensors for on-site pesticide detection
- 1Electronics Materials Lab, College of Science and Engineering, James Cook University, Townsville, QLD, Australia
- 2Nanomaterials and Devices Laboratory, Department of Physics, University of Houston, Houston, TX, United States
- 3Texas Center for Superconductivity, University of Houston, Houston, TX, United States
The infiltration of pesticides into agricultural soils has emerged as a critical concern, posing substantial threats to the agriculture industry due to soil and water contamination. The detection of these contaminants is critical towards implementing effective environmental remediation strategies and achieving ecosystem sustainability. Electrochemical sensor technology has been demonstrated to be highly promising for this application. Graphene and its derivatives and composites are widely used as modifying materials in these sensors to enhance their analytical performance. This short review discusses recent progress in the application of graphene-based electrochemical sensors in three-electrode and field-effect transistor configurations for the detection of pesticides posing significant risks to the agricultural sector. It highlights the growing significance of graphene-based sensors in mitigating pesticide-related environmental challenges and underscores their role in ensuring the health and diversity of agricultural ecosystems.
1 Introduction
Ensuring safety of water and food supplies and preserving environmental integrity are at the forefront of the serious challenges faced by world today. Highly toxic pesticides that cause short- or long-term adverse impacts on human health and ecosystem are common contaminants of water sources and agricultural products. Pesticides are infamous for their resistance to natural degradation, large residence time in the environment, and potential for accumulation in the food chain. Detection and monitoring of pesticides in soil, food and ground water have become critical tasks in the fields of agriculture, food safety, and environmental protection (Zhou et al., 2020). Traditional analytical techniques like colorimetry, spectrometry, and optical sensing methods, while effective, suffer from drawbacks such as high costs, time-intensive procedures, and limited portability for real-time pesticide detection (Zulkifli et al., 2018; Zhang and Li, 2021). Additionally, regarding pesticide detection, optical and mechanical sensors demonstrate high sensitivity and selectivity, but often require complex and bulky equipment, limiting their suitability for real time on-site applications (Hulanicki et al., 1991).Chemical sensors were developed as a suitable alternative to overcome these challenges.
According to the International Union of Pure and Applied Chemistry (IUPAC), a chemical sensor is a device that transforms chemical information, into an analytically useful signal (Ramnani et al., 2016). An electrochemical sensor belongs to this category, and it transforms the analyte-electrode electrochemical interaction into a useful signal. The electrochemical sensors are subdivided into voltametric, potentiometric, and chemically sensitized field effect transistors (FET). In chemically sensitized FETs the effect of interaction between analyte and an active coating is transformed into a change in the source-drain current. In the literature, it is common to find all types of FETs in the class of electrochemical sensors, though generally, the operating principle can differ (Cao et al., 2016; Benjamin and Miranda Ribeiro Júnior, 2022). The conventional FET sensors that operate based on variations in conductance of the channel is an example of other types of devices (Elli et al., 2022). In this review, we discuss the electrochemical sensors in the three-electrode geometry and the FET devices working on electrochemical principles.
The conventional three-electrode electrochemical system comprises of a working electrode (WE), a reference electrode (RE), and a counter electrode (CE) (Kimmel et al., 2012). The interaction of the sensor with target analyte generates an electrical signal that is a measure of the concentration of the analyte. This process entails allowing charged molecules to move through a thin electrolyte layer. This classical method offers several benefits, including high sensitivity and selectivity, linearity of response in a broad range of analyte concentrations, minimal space and power needs, onsite detection capability, and affordable instrumentation (Wang, 2007). Nevertheless, it has its own challenges, such as reliance on an electrolyte, which introduces pH working range limitations, susceptibility to pH-related interferences that reduce stability, electrode degradation, and the need for frequent maintenance and calibration. Additionally, sensitivity can be hampered by non-specific reactions between electroactive impurities on the electrode surface and the sample (Sang et al., 2013).
The FET type, on the other hand, consists of a semiconductor with a source, drain, and gate regions. In a conventional FET, the source and drain are two p-n junctions with source supplying the electrons or holes and the drain receiving them. The gate electrode is formed on a thin insulating layer applied on the semiconductor in the region between the source and the drain (Sedki et al., 2021). Metal oxide semiconductor field effect transistor (MOSFET) is one of the earliest designs of FETs, fabricated with an oxide, SiO2, for example, as the gate insulator. In recent years, new FET configurations have emerged. Thin film transistors (TFTs), which employ typically non-silicon semiconductors as substrates, is an example. Their configurations include top-gate TFT, electrolyte-gate FET (EG-FET), electrochemical transistor (ECT), ion-sensitive FET (ISFET), and chemically sensitive FETs (ChemFETs) (Hulanicki et al., 1991; Kaisti, 2017; Bobrinetskiy and Knezevic, 2018; Benjamin and Miranda Ribeiro Júnior, 2022). Despite these advancements, the MOSFET design is still one of the most used configurations in pesticide detection.
The introduction of graphene and its derivatives has significantly advanced the application of electrochemical sensors for real-time detection and monitoring of pesticides. In the three electrode electrochemical sensors, graphene’s large surface area and exceptional electrical conductivity make it an ideal candidate for the working electrode (WE) (Zafar et al., 2023a). Its high surface to volume ratio enhances the interaction between analytes and the electrode surface resulting into improved sensitivity and detection limits. Additionally, its excellent electron transfer properties facilitate rapid and efficient redox reactions, crucial for electrochemical sensing applications. In FET sensors, graphene serves as a key component in the channel. Its outstanding electron mobility and high carrier mobility enable precise and responsive detection of analytes. Functionalized graphene, graphene oxide (GO) and reduced graphene oxide (rGO) can also provide binding sites for biorecognition elements, enhancing selectivity (Karadurmus et al., 2022). Graphene, with its extensive delocalized π-electron system and substantial theoretical specific surface area (approximately 2,630 m2g−1), exhibits excellent adsorption capabilities for organic compounds (Gao et al., 2021). Furthermore, graphene’s unique properties, combined with the ability to customize its composition and morphology, have opened new avenues for the creation of highly efficient sensing interfaces. These interfaces hold immense potential for on-site, real-time monitoring, offering not only enhanced sensing capabilities but also empowering proactive interventions to minimize risks within the agricultural sector (Zafar et al., 2023a).
In this article, we highlight recent advancements in graphene-based three-electrode and FET electrochemical sensors and provide insights into the future of this technology. Figure 1 presents the thematic overview of our discussion. The three-electrode and FET configurations were selected for discussion due to their outstanding selectivity, sensitivity, and stability in detecting pesticides relevant to the agricultural sector (Koo et al., 2019).
2 Electrochemical detection of pesticides
2.1 Three electrodes system
Pesticides are categorized into various groups depending on their structure. The nitro group containing pesticides is known as nitro pesticides. During the electrochemical reaction, the nitro groups reduce to form hydroxylamine. In organophosphate pesticides, the C=C bond structure undergoes reduction. In organochlorine pesticides, the removal of one electron from chlorine is responsible for the peak. It has also been reported that pesticides with non-aromatic rings provide a higher limit of detection in comparison with pesticides containing aromatic structures (Gonçalves-Filho et al., 2020). The electrochemical detection of pesticides highly relies on their oxidation and reduction reactions at the working electrode. Consequently, the surface of the working electrode is modified with various materials, including nanoparticles and graphene, to enhance its performance. Graphene, with its tremendous specific surface area (m2/g), surpasses metal nanoparticles in its exceptional adsorption capabilities. This property has made it a preferred and extensively researched material for the detection of various pesticides (Tanwar and Mathur, 2021). Figure 2 illustrates the overall electrochemical detection scheme of graphene within a three-electrode system.
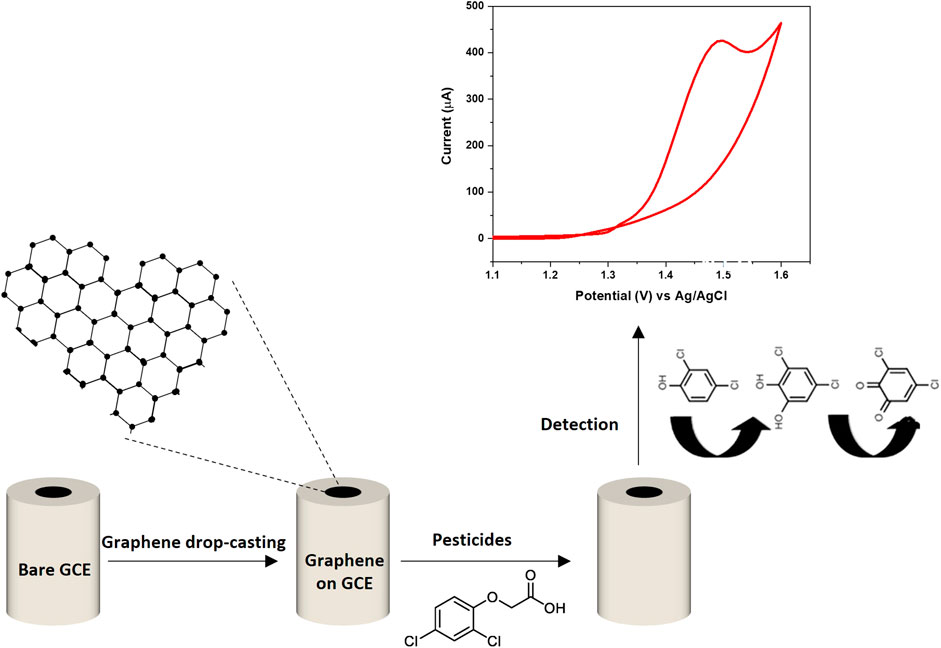
FIGURE 2. An illustration of the electrochemical detection scheme of graphene within a three-electrode system.
Carbofuran, owing to its significant toxicity to human central nervous system, has been the subject of research for its detection in soil and water samples. For instance, Tan et al. (Tan et al., 2015) utilized a reduced graphene oxide-gold-molecularly imprinted polymer (methyl acrylic acid as a functional monomer) to sense carbofuran pesticide. Their findings revealed a significant reduction in the charge-transfer resistance when bare glassy carbon electrode (GCE) was replaced with nanocomposite-modified electrode. As a result, the lower detection limit and linear range of the electrode were significantly improved in comparison. However, underlying mechanisms responsible for the observed enhancements was not clear from their study.
In order to detect methyl parathion, Gong et al. (Gong et al., 2011) employed a gold-graphene modified electrode and applied the technique of square wave voltammetry (SWV). They reported an exceptionally low limit of detection (LOD) of 0.002 μM. The achievement of such a low LOD could be attributed to the synergistic effects of gold and graphene, which aided enhanced electron transfer and provided a favourable platform for the immobilization of nitroaromatic organophosphates. Govindasamy et al. (2017) developed a graphene-molybdenum disulphide nanocomposite and employed a cyclic voltametric technique for detection. Their findings revealed a linear range of 10 nM to 1.9 mM, highlighting the sensor’s ability to quantify methyl parathion over a wide concentration range. The LOD of 0.0032 μM further highlights the high sensitivity of their sensor. Li et al. (2014) focused their efforts on the detection of methyl parathion using a graphene-gadolinium Prussian blue nanocomposite. Their sensors showed a very low LOD of 1 nM and linearity of response over a wide concentration range (0.008–10 mM). The range shifted to higher concentrations compared to that reported by Govindasamy et al. (2017). The incorporation of gadolinium Prussian blue nanoparticles onto graphene surface enhanced the electrocatalytic activity, leading to improved detection capabilities. While all these devices showed promise as methyl parathion sensors, comprehensive studies to understand the selectivity and stability under long-term operation should be conducted to evaluate their practical application.
Zhang et al. (2017) developed a nitrogen-doped graphene-gold nanocomposite for the detection of dimethoate, an organophosphate pesticide. The sensor exhibited a linear range of 1 × 10−12 to 4 × 10−8 M, indicating its potential for accurate quantification over a wide concentration range. The LOD of 8.7 × 10−13 M further highlights the potential of the devise as a dimethoate sensor. However, it was not evident if the sensor would selectively detect dimethoate in presence of other contaminants.
An rGO-gold nanocomposite-modified screen-printed electrode exhibited promising results for diuron herbicide detection (Shams et al., 2016). The study employed linear sweep voltammetry (LSV) for investigations. The sensor demonstrated linear response in a broad concentration range of 0.5–30.0 μM. Additionally, the sensor showed an excellent LOD of 3.9 x 10−7 μM, highlighting its efficacy in detecting diuron herbicide. These findings suggest that the integration of rGO and gold nanocomposites can enhance the electrochemical performance of the sensor, enabling reliable and accurate detection of diuron herbicide (Shams et al., 2016). Zafar et al. (2023b) presented compelling evidence showing the potential of graphene-coated electrodes in diuron detection. The investigation provided insights into the role of pristine graphene in the detection process. The results exhibited enhanced charge-transfer kinetics and higher peak currents compared to bare electrode, which improved the lower LOD. Additionally, the differential pulse voltammetry (DPV) analysis demonstrated linearity in the response over a concentration range of 20–1,000 µM and a low LOD of 5 µM. These findings underscore the suitability of graphene-based electrodes for sensitive and dependable diuron detection, eliminating the need for additional modifications.
The combination of graphene with metals such as boron and silver has shown promise in pesticide detection. Silver-graphene modified boron-doped diamond electrodes have demonstrated simultaneous detection of paraquat and carbaryl with low LODs (Pop et al., 2018). Hashemi et al. (2019) utilized an rGO-Cu/CuO-Ag nanocomposite for detecting fenamiphos and carbaryl, achieving low LODs and wide linear ranges. Glyphosate, a widely used herbicide, poses significant risks to both the environment and human health. To address these concerns, numerous studies have employed graphene-based electrochemical sensors for the detection of glyphosate. Thanh et al. (2021) introduced a composite material on screen-printed gold electrodes, providing a sensitive platform with a low detection limit of approximately 0.08 ppb for glyphosate in river water samples. Johnson et al. (2022) presented a selective detection method using platinum-decorated laser-induced graphene (LIG) in combination with the enzyme glycine oxidase, offering a scalable and selective tool for glyphosate detection in complex matrices. In a separate study, Scandurra et al. (2022) addressed the pressing need for nanomolar-level glyphosate detection in drinking water using gold nanoelectrode arrays on graphene paper. These studies collectively emphasize the significance of developing sensitive and selective glyphosate detection methods, particularly at low concentrations, in response to the challenges posed by environmental contamination and human health risks. Table 1 compares the electrochemical performances of various graphene-based electrode.
2.2 Field-effect transistors
According to a report published by Vinay et al., the use of pesticides has increased in recent years, with the most used synthetic pesticides include organophosphates, organochlorine, carbamate and pyrethroids (Pathak et al., 2022). While different bioremediation strategies are being undertaken by many research groups, there are still grave concerns as to how efficient these solutions may be towards developing a strategy to manage pollutants in an eco-friendly manner. In the same spirit, extensive research is ongoing in the field of electrochemical sensing to supplement these bio remedies. FET biosensors involve the interaction of a biological molecule (biorecognition element) such as enzymes, antibodies, aptamers to produce a measurable signal as response to a target analyte.
FET biosensors exploit variations in the electrical properties of the channel (the active material) in presence of a target analyte (pesticide). In FET devices, the current through the channel is modulated by the local electric field, which can be altered by the physical and chemical environment. A potential difference between the drain and source controls the current between these two electrodes, while the gate voltage controls the electric field across the channel. The presence of analytes can change the electric field, hence the FET characteristics. To enhance selectivity, FET biosensors usually incorporate biorecognition elements such as enzymes, antibodies, cells, and aptamers immobilized on the sensor surface, serving as receptors for the target analyte (Benjamin and Miranda Ribeiro Júnior, 2022). Additionally, nanomaterials are integrated into FET biosensors to increase the surface area for improved analyte-sensor interaction and to enhance sensitivity through the exploitation of low material dimensions approaching the Debye length. The utilization of small bioreceptors, such as aptamers, further opens opportunities to leverage nanoscale features (Sedki et al., 2021).
Graphene based field effect transistors (GFETs) utilize graphene’s large surface area, to form a conducting channel, with sites offered for the analytes (pesticides) to get attached (Gao et al., 2021). Graphene does not readily react or bind with most materials, hence, it must be functionalized with linkers or probes (biorecognition agents) as mentioned before. This in turn is utilized to improve the selectivity of the sensors to a given pesticide. On the other hand, graphene oxide (GO) and reduced graphene oxide (rGO) – derivatives of graphene, possess carboxyl groups that can serve as linkers for direct bonding with biorecognition elements via carbodiimide chemistry (Sedki et al., 2021). Figures 3A, B show respectively the sketches of a GFET with a gate electrode formed at the top of the dielectric layer and that with a gate electrode inserted in an electrolyte. The concentration of the analyte can be correlated with a shift in the charge neutrality point or the drain-source current or the transconductance as shown in Figure 4.
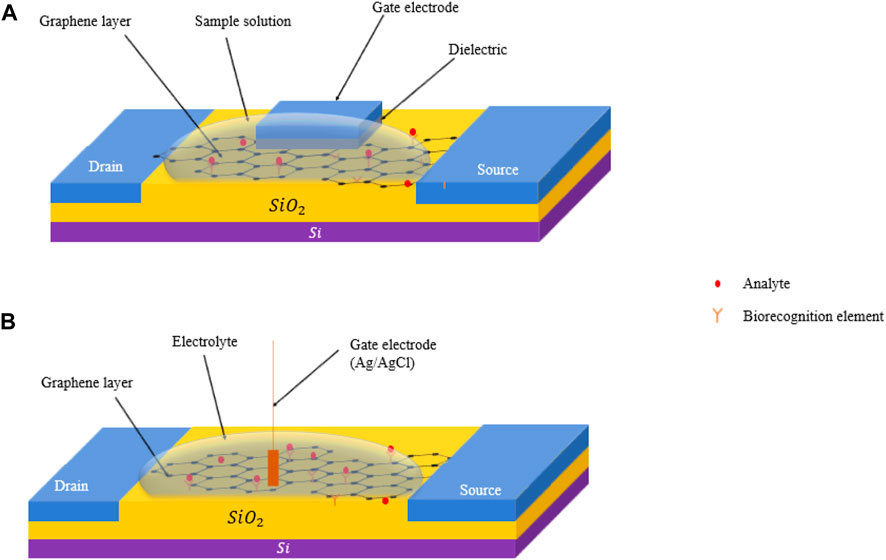
FIGURE 3. (A) A schematic of a solid top gated GFET, with an insulating layer (
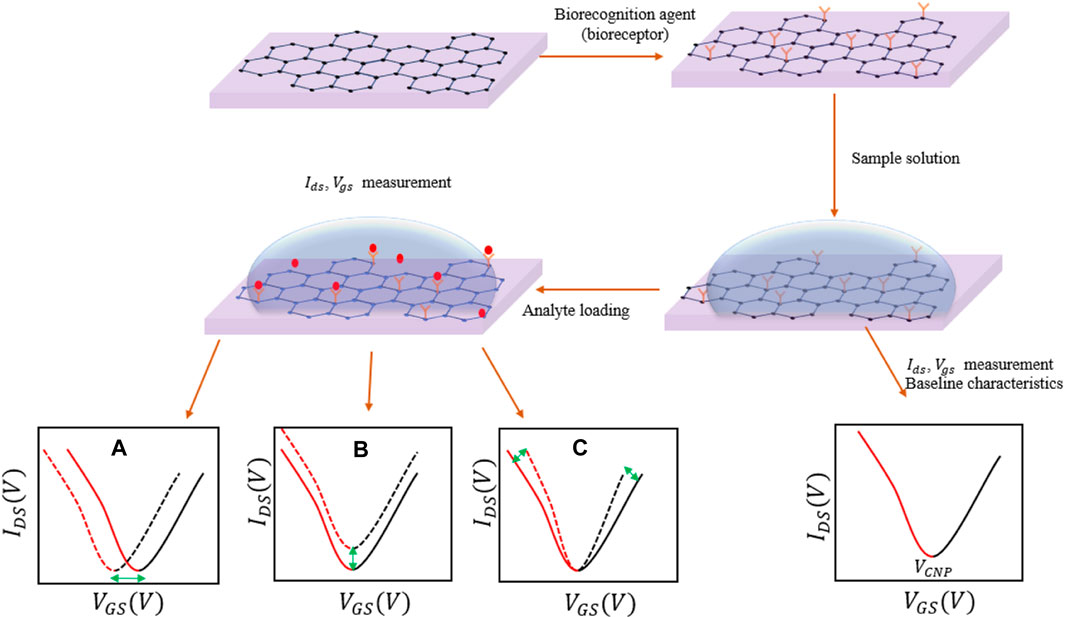
FIGURE 4. A sketch showing the change in the GFET characteristics due to interaction of the analyte with graphene. The bioreceptor is loaded on graphene by either chemical bonding or weak electrostatic interactions (Van der Waals forces). The introduction of the pesticide causes a change in the (A) charge neutrality point (VCNP), (B) drain-source current (IDS), or (C) transconductance.
In this section, we provide an overview of the advancements in GFET biosensors designed for the detection of common pesticides. GFET biosensors have several advantages over the traditional three electrode electrochemical counterparts. The GFETs are known for their simple design, potential for high sensitivity and lower LOD (Benjamin and Miranda Ribeiro Júnior, 2022), which make them suitable for detection of pesticides in agriculture (Wadhera et al., 2019). In addition, they can be operated as label-free biosensors, reducing the cost and risk of interference from electrochemical tags. Liquid state measurements are usually more desirable than a dry state FET based sensors (Islam et al., 2019). Below, we highlight some of the relevant studies involving the use of graphene-based bio-FETs for detection of commonly used pesticides in agriculture. Table 2 shows a summary of these FET devices, and their corresponding limits of detection for common pesticides.
Zhu et al. (2022) introduced a solution-gate GFET for the detection of isocarbophos. A common organophosphate (OP) used in insecticides to control various pests. Si/SiO2 was used a substrate with graphene as the sensing element, functionalized with an acetylcholinesterase enzymatic complex using 3-mercaptoproponoic acid (3-MPA) as a linker. Under experimental conditions of
Islam et al. (2019) presented a graphene-FET-based immunosensor tailored for the detection of chlorpyrifos pesticide. The graphene FET was fabricated through exfoliation technique on Si/SiO2 substrate, with Cr/Au electrodes lithographically attached to form source-drain electrodes. The Chlorpyrifos antibody (Chl-Ab) was labelled with graphene and characterized using scanning electron microscopy and atomic force microscopy. The device’s graphene channel resistance was continuously measured to monitor its response to varying concentrations of Chl antigens (Chl-Ag). This approach yielded a lower limit of detection of
Selective detection of chiral molecules using surface modified graphene-based FET was demonstrated by Zhang et al. (2019). In this study, they utilized acetylcholinesterase for sensor surface modification. They demonstrated sensitive discrimination between positive and negative methamidophos by analysing the resistance changes upon exposure to these molecules. With an operating voltage of 1 V, they achieved a sensitivity of 0.34 and 0.32
Tao et al. (2021) fabricated a ZrO2/rGO nanocomposite functionalized gate for electrochemical detection of the methyl parathion (MP), and obtained an LOD of
Recently, Cao et al. (2016) fabricated a carbaryl ion selective field effect transistor (ISFET) sensor using graphene. The graphene was first prepared on polycrystalline copper and then transferred onto the interdigitated electrodes of the sensor. The sensor utilized the inhibition enzyme urease (biorecognition element) towards carbaryl. The sensor demonstrated a LOD of
A large proportion of FET based biosensors incorporate graphene and its derivatives. It is also worth noting that some non-graphene FET biosensors also were reported to have promisisng sensitivity and selectivity. For instance, a pH-based FET biosensor employing enzyme based biorecognition agents developed by Simonian et al., could detect organophosphate compounds up to micromolar concentrations (Simonian et al., 2004). Compared to these devices, the outstanding advantage of the graphene-based biosensors is the lower LOD. GFET based biosensors still have limitations that must be addressed. An example is the need for careful surface functionalization and potential for signal drift after extended periods. In conclusion, bioFETS have an enormous potential for use in pesticide detection with the use of biorecognition agents to improve selectivity and use of nanomaterials to enhance sensitivity. However, there are still challenges that must be solved for the advancement of the technology and these include use of a biorecognition agent that often causes degradation of performance in certain pH and temperatures, long fabrication time and low shelf life.
3 Conclusion and outlook
This review highlights the importance of monitoring pesticides across diverse contexts, given their adverse impacts on both human health and the environment. Conventional pesticide detection methods come with inherent drawbacks, including cost constraints and portability issues. Graphene-based electrochemical sensors, particularly those designed with three electrode and FET principles, hold great promise due to their notable advantages, including high sensitivity, selectivity, and the potential for on-site detection. Graphene’s extensive delocalized π-electron system and theoretical specific surface area make it an ideal material for adsorbing organic compounds, rendering it an exceptionally promising choice for pesticide detection. This review highlights successful pesticide detection using graphene-based sensors applied on chemicals such as carbofuran, methyl parathion, dimethoate, and diuron. These sensors have consistently demonstrated high sensitivity and wide linear detection ranges.
For advancing the sensor technology for pesticide detection, several essential measures should be taken. These include the following: i) conducting rigorous validation studies using actual samples collected from agricultural and environmental settings; ii) making comparisons with traditional analytical methods to ascertain the reliability of graphene-based sensors; iii) integrating the sensors with smartphone technology for the development of portable devices; iv) investigating the effects of defects, morphology, and nanoparticle size on sensor performance. Nevertheless, the current rapid developments in the semiconductor and graphene technologies are expected to make these targets achievable in the near future.
Author contributions
MZ: Data curation, Validation, Writing–original draft. DW: Data curation, Formal Analysis, Writing–original draft. OV: Conceptualization, Writing–review and editing. MJ: Writing–review and editing.
Funding
The authors declare that no financial support was received for the research, authorship, and/or publication of this article.
Acknowledgments
MZ acknowledges support of PG research scholarship received.
Conflict of interest
The authors declare that the research was conducted in the absence of any commercial or financial relationships that could be construed as a potential conflict of interest.
Publisher’s note
All claims expressed in this article are solely those of the authors and do not necessarily represent those of their affiliated organizations, or those of the publisher, the editors and the reviewers. Any product that may be evaluated in this article, or claim that may be made by its manufacturer, is not guaranteed or endorsed by the publisher.
References
Benjamin, S. R., and Miranda Ribeiro Júnior, E. J. (2022). Graphene based electrochemical sensors for detection of environmental pollutants. Curr. Opin. Environ. Sci. Health 29, 100381. doi:10.1016/j.coesh.2022.100381
Bobrinetskiy, I. I., and Knezevic, N. Z. (2018). Graphene-based biosensors for on-site detection of contaminants in food. Anal. Methods 10 (42), 5061–5070. doi:10.1039/c8ay01913d
Cao, T. T., Nguyen, V. C., Nguyen, H. B., Bui, H. T., Vu, T. T., Phan, N. H., et al. (2016). Fabrication of few-layer graphene film based field effect transistor and its application for trace-detection of herbicide atrazine. Adv. Nat. Sci. Nanosci. Nanotechnol. 7 (035007), 035007. doi:10.1088/2043-6262/7/3/035007
Elli, G., Hamed, S., Petrelli, M., Ibba, P., Ciocca, M., Lugli, P., et al. (2022). Field-effect transistor-based biosensors for environmental and agricultural monitoring. Sensors 22 (11), 4178. doi:10.3390/s22114178
Gao, N., Tan, R., Cai, Z., Zhao, H., Chang, G., and He, Y. (2021). A novel electrochemical sensor via Zr-based metal organic framework–graphene for pesticide detection. J. Mater. Sci. 56 (34), 19060–19074. doi:10.1007/s10853-021-06436-6
Gonçalves-Filho, D., Silva, C. C. G., and De Souza, D. (2020). Pesticides determination in foods and natural waters using solid amalgam-based electrodes: challenges and trends. Talanta 212, 120756. doi:10.1016/j.talanta.2020.120756
Gong, J., Miao, X., Zhou, T., and Zhang, L. (2011). An enzymeless organophosphate pesticide sensor using Au nanoparticle-decorated graphene hybrid nanosheet as solid-phase extraction. Talanta 85 (3), 1344–1349. doi:10.1016/j.talanta.2011.06.016
Govindasamy, M., Chen, S. M., Mani, V., Akilarasan, M., Kogularasu, S., and Subramani, B. (2017). Nanocomposites composed of layered molybdenum disulfide and graphene for highly sensitive amperometric determination of methyl parathion. Microchim. Acta 184, 725–733. doi:10.1007/s00604-016-2062-6
Hashemi, P., Karimian, N., Khoshsafar, H., Arduini, F., Mesri, M., Afkhami, A., et al. (2019). Reduced graphene oxide decorated on Cu/CuO-Ag nanocomposite as a high-performance material for the construction of a non-enzymatic sensor: application to the determination of carbaryl and fenamiphos pesticides. Mater. Sci. Eng. C 102, 764–772. doi:10.1016/j.msec.2019.05.010
Hasnan, M. M. I. M., Lim, G. P., Nayan, N., Soon, C. F., Halim, A. A. A., Ahmad, M. K., et al. (2023). The investigation of chlorpyrifos (Cpy) detection of PEDOT: PSS-MXene (Ti2CT X)-BSA-GO composite using P-ISFET reduction method. Polym. Bull. 80 (2), 1243–1264. doi:10.1007/s00289-022-04105-5
Hulanicki, A., Glab, S., and Ingman, F. (1991). Chemical sensors: definitions and classification. Pure Appl. Chem. 63 (9), 1247–1250. doi:10.1351/pac199163091247
Islam, S., Shukla, S., Bajpai, V. K., Han, Y. K., Huh, Y. S., Ghosh, A., et al. (2019). Microfluidic-based graphene field effect transistor for femtomolar detection of chlorpyrifos. Sci. Rep. 9 (1), 276. doi:10.1038/s41598-018-36746-w
Johnson, Z. T., Jared, N., Peterson, J. K., Li, J., Smith, E. A., Walper, S. A., et al. (2022). Enzymatic laser-induced graphene biosensor for electrochemical sensing of the herbicide glyphosate. Glob. Challenges 6 (9), 2200057. doi:10.1002/gch2.202200057
Kaisti, M. (2017). Detection principles of biological and chemical FET sensors. Biosens. Bioelectron. 98, 437–448. doi:10.1016/j.bios.2017.07.010
Karadurmus, L., Cetinkaya, A., Kaya, S. I., and Ozkan, S. A. (2022). Recent trends on electrochemical carbon-based nanosensors for sensitive assay of pesticides. Trends Environ. Anal. Chem. 34, e00158. doi:10.1016/j.teac.2022.e00158
Kimmel, D. W., LeBlanc, G., Meschievitz, M. E., and Cliffel, D. E. (2012). Electrochemical sensors and biosensors. Anal. Chem. 84 (2), 685–707. doi:10.1021/ac202878q
Koo, W. T., Jang, J. S., and Kim, I. D. (2019). Metal-organic frameworks for chemiresistive sensors. Chem 5 (8), 1938–1963. doi:10.1016/j.chempr.2019.04.013
Kumar, T. V., and Sundramoorthy, A. K. (2019). Electrochemical biosensor for methyl parathion based on single-walled carbon nanotube/glutaraldehyde crosslinked acetylcholinesterase-wrapped bovine serum albumin nanocomposites. Anal. Chim. Acta 1074, 131–141. doi:10.1016/j.aca.2019.05.011
Li, Y., Xu, M., Li, P., Dong, J., and Ai, S. (2014). Nonenzymatic sensing of methyl parathion based on graphene/gadolinium Prussian Blue analogue nanocomposite modified glassy carbon electrode. Anal. Methods 6 (7), 2157–2162. doi:10.1039/c3ay41820k
Pathak, V. M., Verma, V. K., and Rawat, B. S. (2022). Current status of pesticide effects on environment, human health and it’s eco-friendly management as bioremediation: a comprehensive review. Front. Microbiol. 13. doi:10.3389/fmicb.2022.962619
Pop, A., Lung, S., Orha, C., and Manea, F. (2018). Silver/graphene-modified boron doped diamond electrode for selective detection of carbaryl and paraquat from water. Int. J. Electrochem. Sci. 13, 2651–2660. doi:10.20964/2018.03.02
Ramnani, P., Saucedo, N. M., and Mulchandani, A. (2016). Carbon nanomaterial-based electrochemical biosensors for label-free sensing of environmental pollutants. Chemosphere 143, 85–98. doi:10.1016/j.chemosphere.2015.04.063
Sang, S., Zhang, W., and Zhao, Y. (2013). “Review on the design art of biosensors,” in State of the art in biosensors-general aspects, Intechopen, London, UK, 89–110.
Scandurra, A., Censabella, M., Gulino, A., Grimaldi, M. G., and Ruffino, F. (2022). Gold nanoelectrode arrays dewetted onto graphene paper for selective and direct electrochemical determination of glyphosate in drinking water. Sens. Bio-Sensing Res. 36, 100496. doi:10.1016/j.sbsr.2022.100496
Sedki, M., Shen, Y., and Mulchandani, A. (2021). Nano-FET-enabled biosensors: materials perspective and recent advances in North America. Biosens. Bioelectron. 176, 112941. doi:10.1016/j.bios.2020.112941
Shams, N., Lim, H. N., Hajian, R., Yusof, N. A., Abdullah, J., Sulaiman, Y., et al. (2016). A promising electrochemical sensor based on Au nanoparticles decorated reduced graphene oxide for selective detection of herbicide diuron in natural waters. J. Appl. Electrochem. 46, 655–666. doi:10.1007/s10800-016-0950-4
Simonian, A., Flounders, A., and Wild, J. (2004). FET-based biosensors for the direct detection of organophosphate neurotoxins. Electroanalysis 16 (22), 1896–1906. doi:10.1002/elan.200403078
Tan, X., Hu, Q., Wu, J., Li, X., Li, P., Yu, H., et al. (2015). Electrochemical sensor based on molecularly imprinted polymer reduced graphene oxide and gold nanoparticles modified electrode for detection of carbofuran. Sensors Actuators B Chem. 220, 216–221. doi:10.1016/j.snb.2015.05.048
Tanwar, S., and Mathur, D. (2021). Graphene-based nanocomposites as sensing elements for the electrochemical detection of pesticides: a review. J. Solid State Electrochem. 25 (8-9), 2145–2159. doi:10.1007/s10008-021-04990-2
Tao, T., Zhou, Y., Ma, M., He, H., Gao, N., Cai, Z., et al. (2021). Novel graphene electrochemical transistor with ZrO2/rGO nanocomposites functionalized gate electrode for ultrasensitive recognition of methyl parathion. Sensors Actuators B Chem. 328, 128936. doi:10.1016/j.snb.2020.128936
Thanh, C. T., Binh, N. H., Duoc, P. N. D., Thu, V. T., Van Trinh, P., Anh, N. N., et al. (2021). Electrochemical sensor based on reduced graphene oxide/double-walled carbon nanotubes/octahedral Fe 3 O 4/chitosan composite for glyphosate detection. Bull. Environ. Contam. Toxicol. 106, 1017–1023. doi:10.1007/s00128-021-03179-7
Wadhera, T., Kakkar, D., Wadhwa, G., and Raj, B. (2019). Recent advances and progress in development of the field effect transistor biosensor: a review. J. Electron. Mater. 48, 7635–7646. doi:10.1007/s11664-019-07705-6
Wang, H., Hou, E., Xu, N., Nie, P., Chang, L., Wu, J., et al. (2023). Graphene electrochemical transistors decorated by Ag nanoparticles exhibiting high sensitivity for the detection of paraquat over a wide concentration range. Anal. Methods 15 (7), 959–968. doi:10.1039/d2ay01728h
Wang, J. (2007). Electrochemical sensing of explosives. Electroanalysis 19 (4), 415–423. doi:10.1002/elan.200603748
Wang, N., Liu, Z., Wen, L., Zhang, B., Tao, Ca, and Wang, J. (2022). Aptamer-binding zirconium-based metal-organic framework composites prepared by two conjunction approaches with enhanced bio-sensing for detecting isocarbophos. Talanta 236, 122822. doi:10.1016/j.talanta.2021.122822
Yan, X., Deng, J., Xu, J., Li, H., Wang, L., Chen, D., et al. (2012). A novel electrochemical sensor for isocarbophos based on a glassy carbon electrode modified with electropolymerized molecularly imprinted terpolymer. Sensors Actuators B Chem. 171-172, 1087–1094. doi:10.1016/j.snb.2012.06.038
Zafar, M. A., Liu, Y., Allende, S., and Jacob, M. V. (2023a). Expeditious and Eco-friendly fabrication of Graphene-Ag nanocomposite for methyl paraben sensing. Appl. Surf. Sci. 638, 158006. doi:10.1016/j.apsusc.2023.158006
Zafar, M. A., Liu, Y., Hernandez, F. C. R., Varghese, O. K., and Jacob, M. V. (2023b). Plasma-based synthesis of freestanding graphene from a natural resource for sensing application. Adv. Mater. Interfaces 10 (11), 2202399. doi:10.1002/admi.202202399
Zhang, D., Wu, Z., Cao, M., Ni, D., Yu, Z., and Liang, P. (2023). A facile heat-treatment solid phase microextraction method for SERS detection of isocarbophos in tea using a hand-held Raman spectrometer. Food Chem. 424, 136397. doi:10.1016/j.foodchem.2023.136397
Zhang, J., and Li, S. (2021). Sensors for detection of Cr (VI) in water: a review. Int. J. Environ. Anal. Chem. 101 (8), 1051–1073. doi:10.1080/03067319.2019.1675652
Zhang, Y., Fa, Hb, He, B., Hou, Cj, Huo, Dq, Xia, Tc, et al. (2017). Electrochemical biomimetic sensor based on oxime group-functionalized gold nanoparticles and nitrogen-doped graphene composites for highly selective and sensitive dimethoate determination. J. Solid State Electrochem. 21, 2117–2128. doi:10.1007/s10008-017-3560-0
Zhang, Y., Liu, X., Qiu, S., Zhang, Q., Tang, W., Liu, H., et al. (2019). A flexible acetylcholinesterase-modified graphene for chiral pesticide sensor. J. Am. Chem. Soc. 141 (37), 14643–14649. doi:10.1021/jacs.9b05724
Zhou, Y., Zhao, W., Lai, Y., Zhang, B., and Zhang, D. (2020). Edible plant oil: global status, health issues, and perspectives. Front. Plant Sci. 11 (1315), 1315. doi:10.3389/fpls.2020.01315
Zhu, J., Feng, M., and Lian, G. (2022). Graphene based FET biosensor for organic-phosphorous sample detection and the enzymatic analysis. Crystals 12 (10), 1327. doi:10.3390/cryst12101327
Keywords: graphene, electrochemical sensor, biosensor, pesticide, graphene sensor
Citation: Zafar MA, Waligo D, Varghese OK and Jacob MV (2023) Advances in graphene-based electrochemical biosensors for on-site pesticide detection. Front. Carbon 2:1325970. doi: 10.3389/frcrb.2023.1325970
Received: 22 October 2023; Accepted: 13 November 2023;
Published: 28 November 2023.
Edited by:
Duncan John Mowbray, Yachay Tech University, EcuadorReviewed by:
Sarah Briceño, Yachay Tech University, EcuadorJulio Cesar Chacón Torres, Yachay Tech University, Ecuador
Copyright © 2023 Zafar, Waligo, Varghese and Jacob. This is an open-access article distributed under the terms of the Creative Commons Attribution License (CC BY). The use, distribution or reproduction in other forums is permitted, provided the original author(s) and the copyright owner(s) are credited and that the original publication in this journal is cited, in accordance with accepted academic practice. No use, distribution or reproduction is permitted which does not comply with these terms.
*Correspondence: Mohan V. Jacob, mohan.jacob@jcu.edu.au
‡ORCID: Oomman K. Varghese, https://orcid.org/0000-0003-2386-0153
†These authors have contributed equally to this work and share first authorship