- 1Immunology Department, Noguchi Memorial Institute for Medical Research, College of Health Sciences, University of Ghana, Accra, Ghana
- 2West Africa Centre for Cell Biology of Infectious Pathogens, Department of Biochemistry, Cell and Molecular Biology, University of Ghana, Accra, Ghana
- 3Centre for Medical Parasitology, Department of Immunology and Microbiology, Faculty of Health and Medical Sciences, University of Copenhagen, Copenhagen, Denmark
- 4Department of Biochemistry and Molecular Biology, Faculty of Biosciences, University for Development Studies, Nyankpala, Ghana
- 5Department of Infectious Diseases, Rigshospitalet, Copenhagen, Denmark
- 6Centre for Tropical Clinical Pharmacology and Therapeutics, University of Ghana Medical School, College of Health Sciences, University of Ghana, Korle-Bu, Ghana
Background: The protective effect of certain haemoglobinopathies, such as HbS, HbC, and α-thalassaemia, against severe malaria has long been established; however, there is only limited and equivocal evidence regarding their impact on asymptomatic parasitaemia. Here, we investigated the effect of HbS, HbC, and α-thalassaemia on asymptomatic P. falciparum parasitaemia and acquired immunity among children in Northern Ghana.
Materials and methods: A cross-sectional study was conducted among 1,017 healthy children (1-17 years) in 13 malaria-endemic communities in Northern Ghana. The children were screened for structural Hb phenotypes using SickleSCAN, for P. falciparum infection using anti-HRP2 malaria RDT and subsequently confirmed by capillary electrophoresis and PCR, respectively. α-thalassaemia genotyping was done using PCR. Levels of IgG specific for six recombinant malaria antigens (PfCSP, GLURP, MSP3, Pfs230, HB3VAR06, and IT4VAR60) and crude asexual blood-stage antigens were evaluated by ELISA.
Results: 266 out of the 1,017 participants had either HbAC (18%) or HbAS (8.4%), whereas 35% had α‐thalassaemia. Twenty-five percent and 6% HbAC individuals co-inherited heterozygous and homozygous α-thalassaemia respectively. Similarly, 25% and 10.5% of HbAS co-inherited heterozygous and homozygous α-thalassaemia. Asymptomatic parasitaemia rates were 23%, 24%, and 19% in those with HbAA, HbAC and HbAS, respectively. The overall parasite carriage rates in heterozygous (21%) and homozygous α-thalassaemia (25%) individuals were similar to that of individuals without α-thalassaemia (23%). P. falciparum parasite carriage risk was about three times higher among homozygous α-thalassaemia individuals with HbAC (OR = 2.97; 95% CI 0.83-10.62) and heterozygous carriers with HbAS variants (OR = 2.86; 95% CI 0.85-9.60) compared to the wildtype. In HbAS individuals, IgG levels to IT4VAR60 and HB3VAR06 were significantly lower, whereas anti-CSP levels were higher than in HbAA and HbAC.
Conclusions: Co-inheritance of HbAS and HbAC with α-thalassaemia increased the risk of asymptomatic parasitaemia, an indication of a negative epistatic effect between these Hb variants. Antibody levels against non-PfEMP1 antigens were slightly higher among HbAS children, but quite similar in all study groups, indicating differences in parasite exposure.
Introduction
Haemoglobinopathies are highly prevalent in malaria-endemic communities (1–3). Haldane in 1949 (4) and Allison in 1954 (5) first speculated about the protective effect of these, giving rise to the ‘malaria hypothesis’. HbS, HbC, and HbE result from single amino acid substitutions in the β-globin gene in adult haemoglobin (HbA), whereas the thalassaemias are the consequence of reduced production of globins (6).
HbC (7, 8) HbS (9), HbE (10), α-thalassemia, and β-thalassaemia protect against severe malaria (1, 11) and are hence common in malaria-endemic areas (12). The protective effect against severe forms of malaria is most pronounced for HbS (1), with the highest level of protection in HbAS individuals (91%). The homozygous HbCC provides up to 73% protection, and 37% for homozygous and heterozygous α-thalassaemia, as well as 20% for the HbAC phenotypes (1). HbS is widely distributed across Africa, whereas HbC is restricted to parts of West Africa (13–15). In Ghana, HbC is most frequent in the northern part of the country (19.7% to 20.7%) (16, 17). α-thalassemia is highly prevalent in the sub-Saharan region (up to 50%) (18, 19) and in Southeast Asia (40%) (20).
The proposed protective mechanisms for these erythrocyte polymorphisms include reduction in parasite growth (21–23), parasite invasion (24, 25), and in the adhesion of parasitised erythrocytes to endothelial vessels and uninfected erythrocytes (26, 27). These last two phenotypes are mediated by PfEMP1 on the parasitised erythrocyte surface (28). However, the exact mechanism of protection is unknown, and little is known about the impact of these variants on uncomplicated malaria and asymptomatic infections (29, 30). In addition, HbS could have an indirect effect on malaria susceptibility because it enhances phagocytosis and activates inflammatory cytokines (31–33). Overall, the impact of these haemoglobinopathies on parasite carriage appears to be minimal (1), but little is known about how co-inheritance of these haemoglobinopathies affects parasite carriage, malaria pathogenesis, and naturally acquired immunity. We assessed the impact of HbS and HbC, α-thalassaemia and their co-inheritance in P. falciparum parasite carriage and acquired immune response in Ghanaian children.
Materials and methods
Ethics statement
This study was approved by the Ethics Review Committee of the Ghana Health Service (GHS; GHS-ERC 008/07/19) and by the Noguchi Memorial Institute for Medical Research (NMIMR) Institutional Review Board (Federal-wide Assurance FWA 00001824, NMIMR-IRB CPN 006/19). A declaration of free willingness to participate in the study and written informed consent were obtained from all participants or guardians before enrolment.
Study design and study site
A cross-sectional study was conducted between August and September 2020 in 13 rural communities in the Kumbugu, Nanton, and Tolon Municipalities, Northern Region, Ghana (Figure 1), to assess the prevalence of the above haemoglobinopathies and their association with the risk of P. falciparum infection among children. Detailed information on the study is given elsewhere (Seidu et al. submitted for publication). The Northern Region is the largest in Ghana, and situated in the savannah woodland zone (34). The region is relatively dry, with a single rainy season from April to September or October, a dry season from November to March/April, and severe harmattan winds between December and early February (35). The population of the municipalities is approximately 300,000.
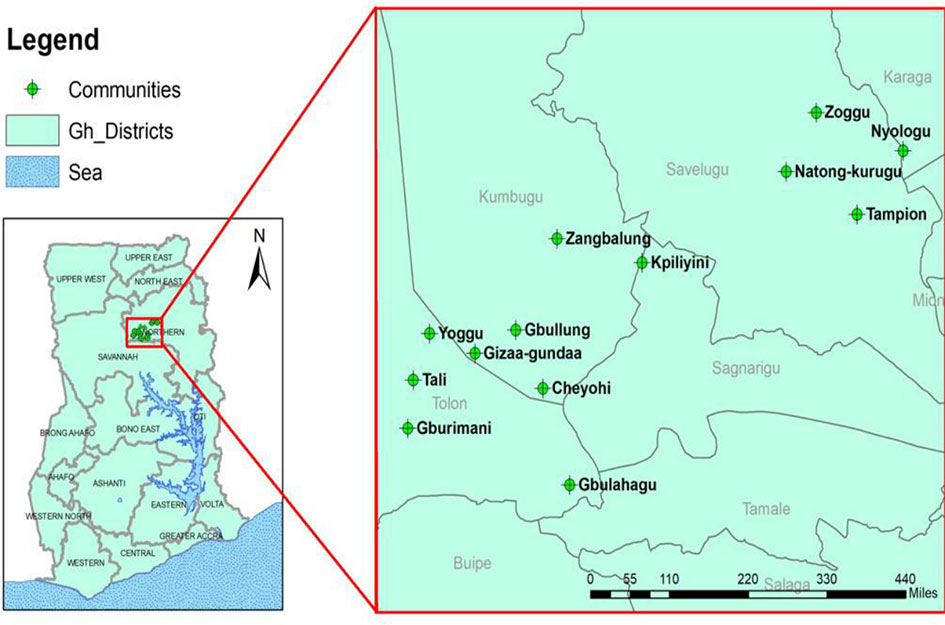
Figure 1 Location of the study sites. Ghana is shown on the left. The location of the study site in the Northern Region and the study communities are shown in the enlarged map. The GIS coordinates of the thirteen study communities were used to construct a graphical plot using the ArcMap tool employed in ArcGIS v10.1. (ESRI, Redlands, California, USA). The shapefiles used for constructing the map are publicly available at https://data.humdata.org/dataset/cod-ab-gha.
Study population and data collection
One-thousand-and seventeen healthy children aged 1-17 years participated in the study (60% males and 40% females). Children from randomly selected households in the communities were recruited and assembled at community health facilities for sample collection and screening. A structured questionnaire was used for in-person interviews with children’s guardians to collect socio-demographic information. Finger-prick blood samples were collected to measure Hb levels using a URIT-12 haemoglobin instrument (URIT Medical Electronic Ltd, China), and to test for P. falciparum infection and structural Hb phenotypes by RDT. Dried blood spots (DBS) on filter paper (Whatman, USA) were used for retrospective confirmation of P. falciparum infection and α-thalassaemia genotyping by PCR. In addition, venous plasma was stored at ‐20°C to determine malaria-specific IgG levels later.
Determination of P. falciparum infection with anti-HRP2 RDT and PCR
The First Response Malaria Antigen-HRP2 Card Test (Premier Medical Corporation, Gujarat, India) was used for on-site diagnosis of P. falciparum infection, following the manufacturer’s instructions. DNA was extracted from DBS using the Chelex method (36), and P. falciparum infection was confirmed retrospectively by PCR as described (Seidu et al., submitted for publication). Children both RDT and PCR positive were classified as having asymptomatic infection.
Screening for structural Hb variants and α-thalassaemia status
The Hb phenotyping was carried out using the Sickle-SCAN (Bioline, USA), point-of-care test (37, 38) and retrospectively confirmed with iso-electric focusing (IEF) electrophoresis method, using a Multiphor II electrophoresis unit (GE Healthcare, Little Chalfont, England) as described (39). The α 3.7 deletion in the α globin gene was used for α-thalassaemia detection in this study, as described (40). Participants were categorised as wildtype, heterozygous α-thalassaemia, or as homozygous α-thalassaemia based on band size after genotyping.
Recombinant proteins
Recombinant glutamate-rich protein domain R0 (GLURP) was produced in E. coli (41), while the merozoite surface protein 3 (PfMSP3) (42) and a 230-kDa sexual stage protein (Pfs230) (43) were produced in Lactococcus lactis. The entire ectodomain of two PfEMP1 proteins, HB3VAR06 and IT4VAR60, were produced in baculovirus-transfected Sf9 insect cells, as described (44). Circumsporozoite protein (PfCSP) was purchased from IPG Technologies (Germany) and a crude asexual P. falciparum lysate was prepared from the 3D7 clone in our laboratory.
Measuring P. falciparum-specific IgG levels
Levels of IgG specific for six recombinant P. falciparum proteins and crude 3D7 antigens were measured using an indirect ELISA, as reported (45, 46). Briefly, 96-well flat-bottom microtiter plates (Nunc MaxiSorp; Thermo Fisher Scientific) were coated with 0.5 µg/mL of recombinant proteins (CSP, GLURP, PfMSP3, or Pfs230), 2µg/mL of the PfEMP1-type recombinant proteins or 5 × 105 IEs/mL of the crude antigen lysate. After blocking with 1% BSA in PBS, plasma samples (1:300) were added in duplicate, followed by horseradish peroxidase-conjugated rabbit anti-human IgG (1:3,000; Dako, Denmark). Bound plasma antibodies were detected by adding TMB (Abcam, UK), and the reaction was stopped with 0.2 M H2SO4. The optical density (OD) was read at 450 nm (Varioskan LUX; Thermofisher, USA). The specific antibody levels were calculated in arbitrary units (AU) using the equation [(ODSAMPLE - ODBLANK)/(ODPOSITIVE CONTROL - ODBLANK)] × 100. Plasma samples from malaria-unexposed Danish individuals and malaria-exposed Ghanaian children were included as negative and positive controls, respectively.
Statistical analysis
Data were analysed using R version 3.6.3 (R Core Team, 2020) in Rstudio and GraphPad Prism version 9.3.1 (GraphPad Software, San Diego, CA, USA). The data were converted into frequencies, and subgroup proportion tables were generated using the “publish” package (47). The proportions between different categories were compared by Chi-square test or Fisher’s exact test. The “glm” function in R and the “publish” package were used for multivariate analysis using a logistic regression model. P-values < 0.05 were considered statistically significant.
Results
Demographic and clinical characteristics of the study population
The study included 1,017 children aged 1-17 years, most of them with HbAA (wild type; 73%), whereas 18% and 8.4% had HbAC and HbAS, respectively (Table 1). Thirty percent had heterozygous α‐thalassaemia, while 6% were homozygous. Additionally, 25% (45/181) and 6.1% (11/181) of HbAC individuals co-inherited heterozygous and homozygous α-thalassaemia respectively. Similarly, 25% (21/85) and 10.5% (11/85) of the HbAS individuals co-inherited heterozygous and homozygous α thalassaemia respectively. Overall, the mean Hb level was 10.9 g/dL. Children with homozygous α‐thalassaemia had the lowest Hb levels (p = 0.04).
Association of haemoglobinopathies with P. falciparum parasite carriage
The association of the Hb phenotypes and α-thalassaemia with P. falciparum infection was determined by comparing parasite carriage by PCR among the different haemoglobinopathies groups. The parasite carriage rates were similar among individuals with HbAA (23%), HbAC (24%), and HbAS (19%; p = 0.73; Table 1). With respect to α-thalassaemia, parasite carriage rates were similar among homozygous (25%) and heterozygous (21%), and wildtype individuals (23%) (p = 0.75; Figure 2B). However, using a logistic regression model with interaction, it was observed that parasite carriage was about three times higher among homozygous α-thalassaemia HbAC individuals (OR = 2.97, p = 0.09) and among heterozygous HbAS individuals (OR = 2.86, p = 0.09; Table 2). However, for the HbAS individuals carrying the homozygous α-thalassaemia phenotype, no significant difference was observed with the risk of parasite carriage (OR = 0.71, p = 0.77; Table 2).
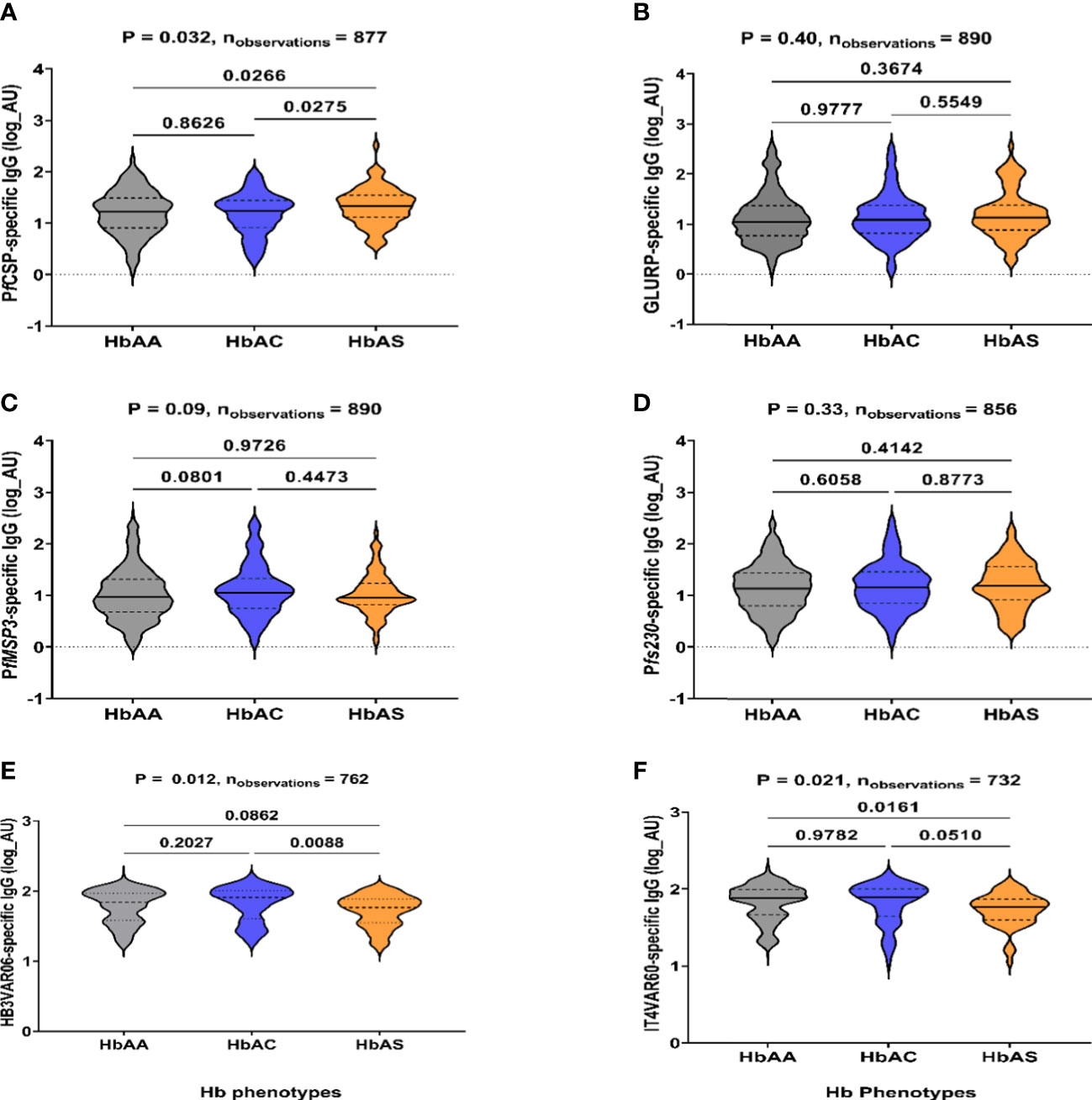
Figure 2 IgG-specific levels to P. falciparum antigens in HbAA, HbAS, and HbAC individuals. IgG levels to (A) PfCSP, (B) GLURP, (C) PfMSP3, (D) Pfs230, (E) HB3VAR06, and (F) IT4VAR60 determined by ELISA, respectively. The x-axis is the Hb phenotypes (HbAA, HbAC and HbAS). IgG levels are expressed in arbitrary log units (AU) on the y-axis. P-values using Kruskal-Wallis test followed by Dunnett’s test for multiple comparisons are shown.
Levels of P. falciparum antigen-specific IgG as a measure of malaria exposure
To further explore the effect of the haemoglobinopathies on malaria parasite exposure, IgG specific levels were measured against recombinant P. falciparum proteins from several parasite stages, sporozoite (PfCSP), merozoite (GLURP, MSP3), gametocyte (Pfs230), and infected erythrocytes (PfEMP1) (Figure 2) and crude antigens (Supplementary Figure 1). Children with HbAS had significantly higher IgG levels to PfCSP than in HbAA (p = 0.03) and HbAC (p = 0.03; Figure 2A). In contrast, levels to HB3VAR06 (p = 0.09) and IT4VAR60 (p = 0.02) were lower in HbAS compared to HbAA (Figures 2E, F). No significant differences in IgG levels to GLURP, MSP3, and Pfs230 (non-PfEMP1-type) antigens were observed among the HbAA, HbAC, and HbAS individuals.
The IgG specific levels to all the antigens except Pfs230 and IT4VAR60 positively correlated with age in the HbAA individuals (Figure 3A). There was no correlation between age and the IgG levels to HB3VAR06, IT4VAR60, PfS230 and PfMSP3 in HbAC individuals (Figure 3B). Additionally, among the HbAS individuals, there was no correlation between age and the IgG levels to all antigens (Figure 3C).
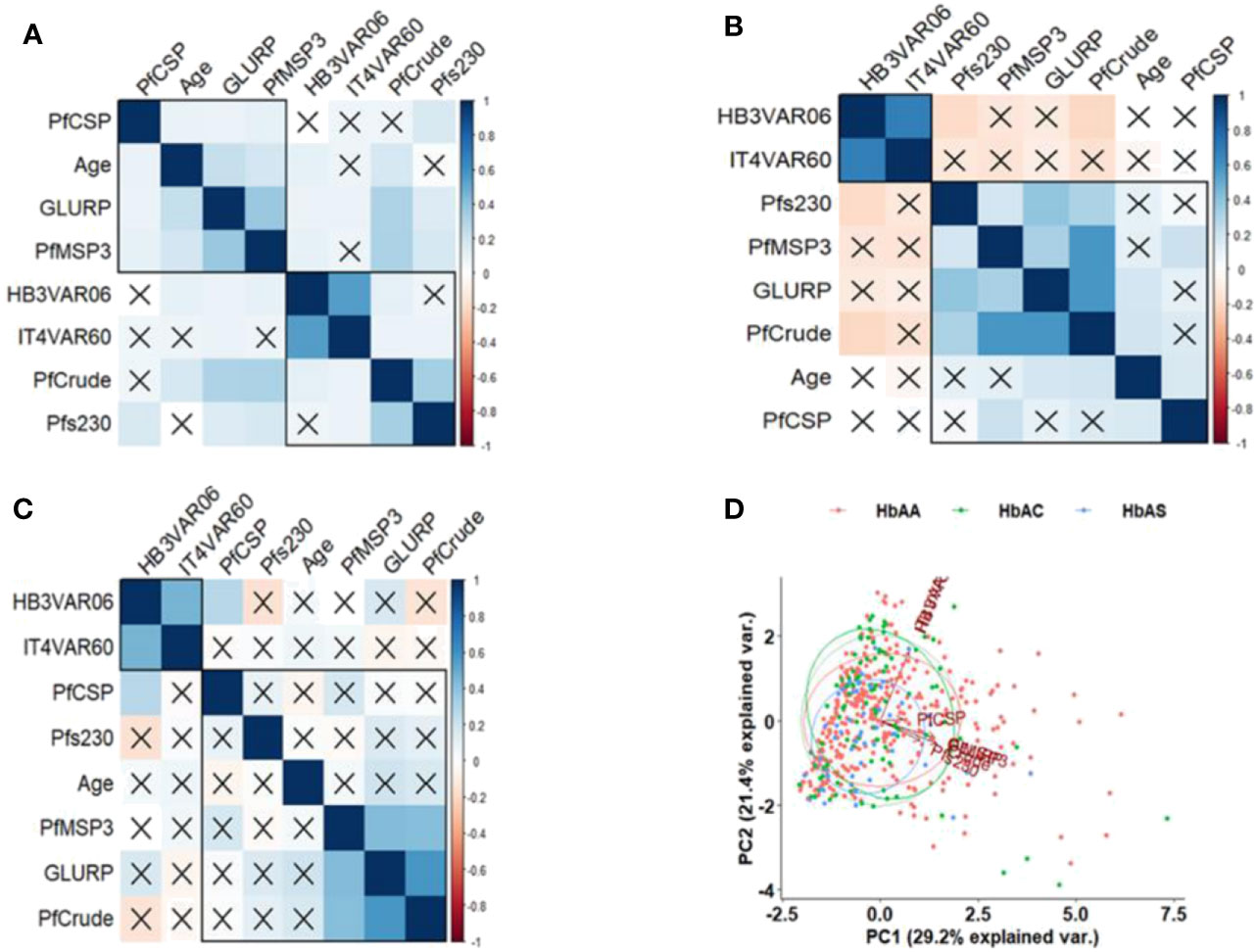
Figure 3 Correlation of IgG levels and age according to Hb phenotypes. Pearson’s correlation matrixes of specific IgG levels and age in (A) HbAA, (B) HbAC, and (C) HbAS individuals. The values displayed are r values, deep blue colour shows strength of the variation among the variables. Insignificant correlations are crossed. (D) principal component analysis (PCA) based on the IgG levels to the antigens. The x-axis and y-axis on the PCA show the proportion of variance explained by Principal Component (PC) 1 and 2, respectively.
A principal component analysis (PCA) indicated variation in the IgG levels among HbAA, HbAC, and HbAS individuals (Figure 3D) but did not differentiate the Hb phenotypes. The PCA also revealed that PfEMP1-specific IgG levels are completely different from the non-PfEMP1 antigens. Among the non-PfEMP1 antigens, levels against PfCSP, the only pre-erythrocytic stage antigen, were also different.
Individuals with homozygous α-thalassaemia had significantly higher IgG levels to PfCSP (p=0.01) and GLURP (p=0.02) and lower IgG levels to HB3VAR60 (p=0.07) and IT4VAR06 (p=0.03) than both the heterozygous mutants and wildtype individuals. They also had slightly higher IgG levels to MSP3 and Pfs230 (Figure 4).
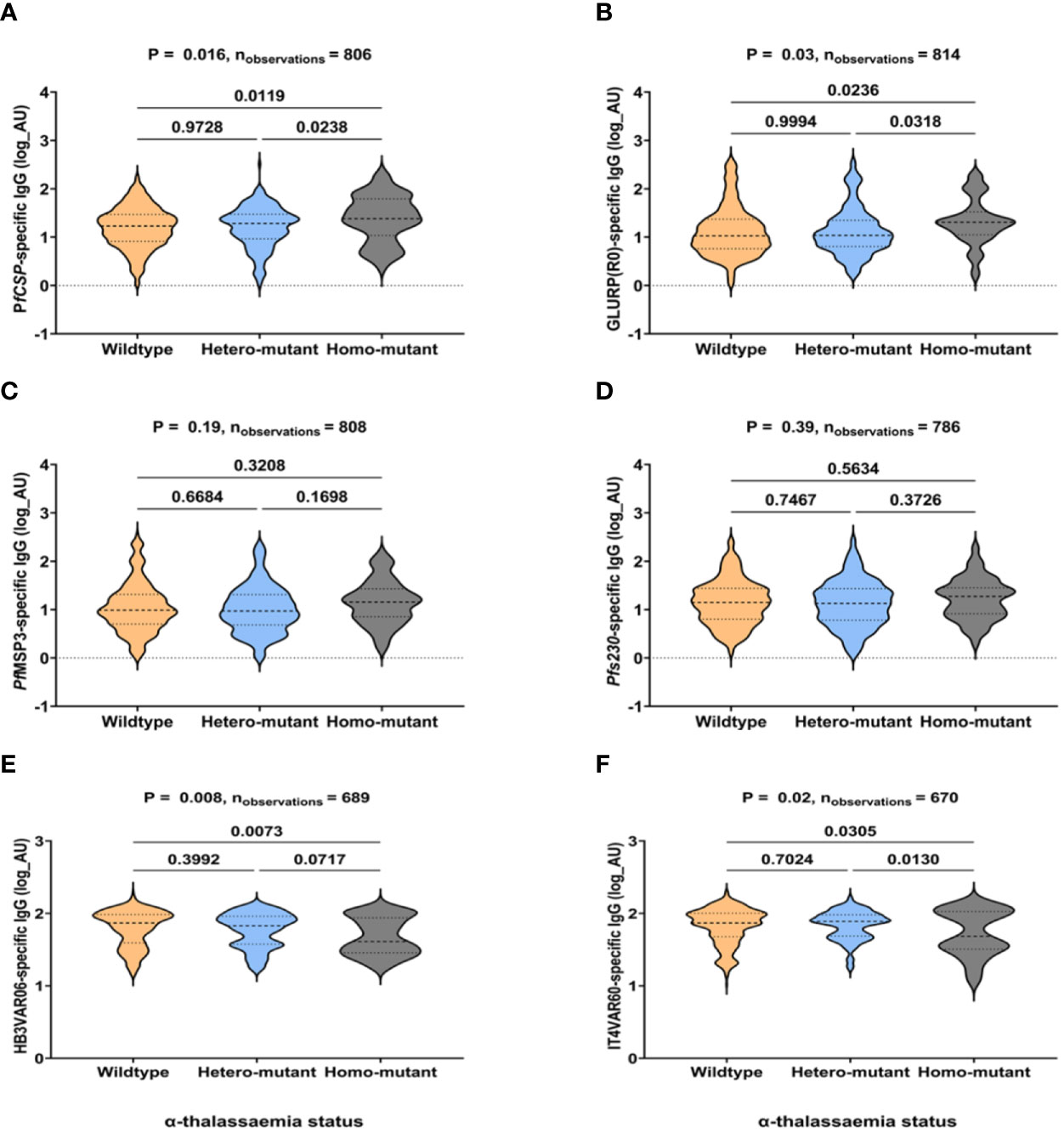
Figure 4 IgG levels to non-PfEMP1 and PfEMP1 antigens in individuals with α-thalassaemia. (A–F) shows total IgG responses to PfCSP, GLURP(R0), PfMSP3, Pfs230, HB3VAR06, and IT4VAR60, respectively. The x-axis is the α-thalassaemia status of participants (wildtype, heterozygous, and homozygous mutants). IgG levels are expressed in arbitrary log units (AU) on the y-axis. P-values were determined using Kruskal-Wallis test followed by Dunnett’s test for Multiple Comparisons.
There is virtually no correlation between age and IgG levels against all the antigens in both the wildtype α-thalassaemia individuals and homozygous mutation (Figures 5A, C). In contrast, IgG levels against GLURP, PfMSP3 and the crude antigens positively correlated with age (Figure 5B).
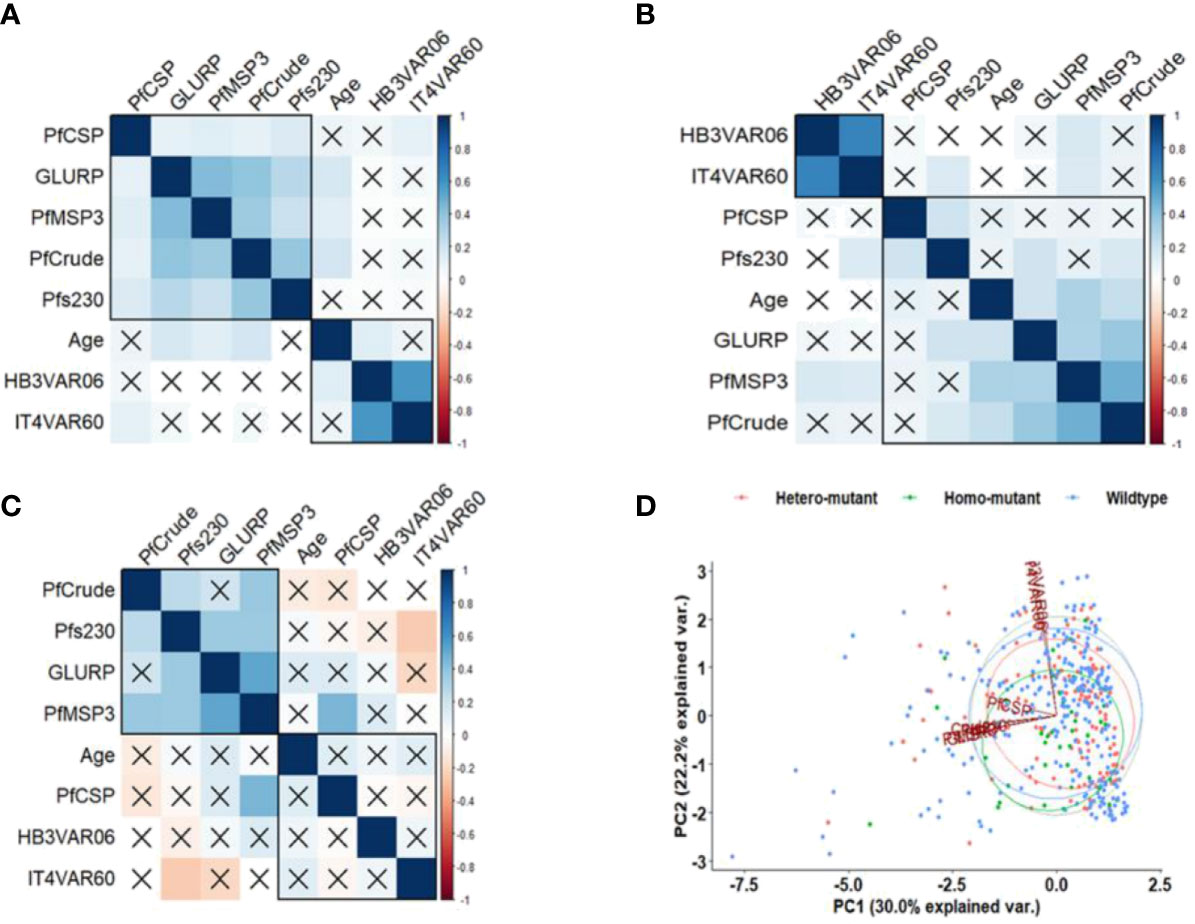
Figure 5 Correlation of IgG levels, age, and α-thalassaemia status. Pearson correlation matrixes of (A) wildtype, (B) heterozygous, and (C) homozygous mutant individuals. The values displayed are r values, deep blue colour shows strength of the variation among the variables. Insignificant correlations are crossed. (D) PCA based on the IgG levels to the antigens. The x-axis and y-axis on the PCA show the proportion of variance explained by Principal Component (PC) 1 and 2, respectively.
The PCA for α-thalassaemia status revealed that IgG levels to the PfEMP1 antigens differed from the non-PfEMP1 antigens, with the IgG levels against the PfCSP differentiated from the rest of the antigens (Figure 5D), as observed for Hb phenotype.
Discussion
HbAS, HbAC, and α-thalassaemia have been reported to protect against severe P. falciparum malaria (1, 48); however, the effect on these highly frequent haemoglobinopathies on the risk of P. falciparum parasite carriage is less clear. Here, we show lower but not statistically significant parasite carriage (19%) in individuals carrying the HbAS phenotype compared to HbAA (23%) and HbAC (24%) as evidenced in literature, that this could be an indication of enhanced parasite clearance in those carrying the sickle cell trait (16). In contrast, individuals carrying homozygous α-thalassaemia had higher parasite carriage (25%) compared to the heterozygous (21%) and wild type (23%). We also report that co-inheritance of homozygous α-thalassaemia with HbAC and heterozygous α-thalassaemia with HbAS increased the risk of P. falciparum asymptomatic parasitaemia about threefold compared to wild-type. Our results agree with previous reports of protection against severe malaria but not asymptomatic infections conferred by HbAS and HbAC phenotypes (1, 8, 16, 39, 49–51), and protection against severe malaria anaemia but not against parasite carriage in homozygous α-thalassaemia (52, 53). Taken together, our findings and previous studies reinforce the hypothesis that reduced cytoadhesion of infected RBCs (54) rather than a parasitaemia reduction in vivo is the most likely explanation for the protection afforded by these haemoglobinopathies.
The increased risk of parasite carriage among individuals that co-inherited α‐thalassaemia, with HbAC or HbAS suggests a negative epistasis between α-thalassaemia and HbAC/HbAS in Ghana, as reported previously in a study in Kenya on negative epistasis associated with co-inheritance of HbAS and α-thalassaemia (55). It is also consistent with reports of chronic parasite carriage in HbAS and HbAC individuals (56, 57). Previously, reduction in PfEMP1 expression and cytoadhesion of HbAS erythrocytes has been reported (27); however, co-inheritance of HbAS with α-thalassemia might reverse this effect (58), which might explain the loss of protection observed in vivo (55). We observed no significant risk of parasite carriage among HbAS individuals co-inherited with the homozygous α-thalassaemia phenotype, this could be due to sample size in our cohort. The conflicting effects of the HbAS heterozygous and HbAS homozygous on parasite carriage could be that the AS-heterozygous α-thalassaemia co-inheritance supports preferential persistence of parasites, but AS-homozygous α-thalassaemia co-inheritance may retard parasite growth (58). However, this hypothesis was not tested in this work, we hope to test this in future studies.
Naturally acquired immunity to malaria is sustained by premunition (59), and the clinical and epidemiological consequences of reduced parasite exposure on protective immunity are reported, with rebounds of malaria in previously eliminated areas (60, 61). On the other hand, haemoglobinopathies might act like a ‘double-edged’ sword in malaria-endemic areas, operating against severe malaria on the one hand and promoting asymptomatic parasitaemia on the other. Hence, such chronic parasite carriage could facilitate parasite transmission (57) but also sustain naturally acquired protective immunity to malaria.
To assess the impact of these haemoglobinopathies on naturally acquired immunity, we measured antibody levels to several recombinant P. falciparum antigens spanning the various life cycle stages. The selected antigens have been associated with protection against malaria and are potential vaccine candidates (62–64). Here, we found higher IgG levels against PfCSP and lower to IT4VAR60 in HbAS individuals than in HbAA and HbAC. Similarly, children carrying the homozygous α-thalassaemia mutation had significantly higher IgG levels to PfCSP and GLURP but lower to HB3VAR06 and IT4VAR60 than the heterozygous α-thalassaemia and wildtype individuals.
The higher antibody responses observed to some malaria antigens measured (non-PfEMP1 antigens) in our study among the HbAS, HbAC compared to the wild type, and the homozygous α-thalassaemia agrees with previous studies, which found higher antibody responses among individuals with various Hb variants and these have been associated with clinical protection from malaria (31, 33, 65). This finding could also be associated with the higher asymptomatic infection rate in haemoglobinopathy individuals, as chronic parasitaemia could boost antibody responses (46, 63, 66, 67). Studies have proposed that HbAS and HbAC have similar mechanisms of conferring protection through impaired cytoadhesion, which enhances the acquisition of protective IgG responses to clear parasites (31, 68). However, studies found no association with acquisition of protective IgG responses (69) or lower IgG levels (70) in HbAS compared to HbAA individuals, perhaps due to limited exposure to malaria. Since acquiring IgG antibodies only to merozoite antigens does not reduce the risk of malaria in HbAS individuals (69–71), other factors concerning transmission intensity and different repertoire of antigens should also be considered.
In contrast with our findings using two recombinant proteins, studies conducted in Gabon and Burkina Faso found higher antibody levels in HbAS children compared to HbAA to variant surface antigens expressed by clinical isolates (31, 68). However, no differences were found in Mali using a few PfEMP1 recombinant proteins (72). A recent study among HbAS and HbAC Beninese pregnant women showed no differences in the IgG levels to selected PfEMP1 variants (46, 73). Hence analysis with a broader range of PfEMP1 variants would be more informative. Less is known in α-thalassaemia, but our data indicate a lower exposure to those specific proteins used here. The differences in IgG levels to Pf-EMP1 could be due to transmission intensity.
We acknowledge that the nature of our study may bias the results with respect to protection in certain Hb variants, since a longitudinal study may provide a better estimate of the exposure status. However, this study confirmed the lack of protection for asymptomatic parasitaemia conferred by Hb variants and the increased risk of parasite carriage among children carrying on α-thalassaemia and HbAS or HbAC. Therefore, screening for these haemoglobinopathies in malaria-endemic communities is important to help reduce the risk it might pose to chronic parasite carriage. Differences in IgG levels to the antigens tested here also indicate changes in the exposure and potentially reduced exposure of PfEMP1 on the infected erythrocytes of HbAS and α-thalassaemia individuals. Identifying P. falciparum antigens that would induce protective antibody responses in future vaccine development must consider these Hb variants in endemic populations since they influence naturally acquired immunity in these individuals.
Data availability statement
The raw data supporting the conclusions of this article will be made available by the authors, without undue reservation.
Ethics statement
This study was approved by the Ethics Review Committee of the Ghana Health Service (GHS; GHS-ERC 008/07/19) and by the Noguchi Memorial Institute for Medical Research (NMIMR) Institutional Review Board (Federal-wide Assurance FWA 00001824, NMIMR-IRB CPN 006/19). Written informed consent to participate in this study was provided by the participants’ legal guardian/next of kin.
Author contributions
HL, ZS, ML-P, LH, GA and MO conceived and designed the study. HL, ZS and EK-B collected data. HL, ZS and EK-B performed laboratory experiments. HL and ZS analysed the data and wrote the manuscript with inputs from all authors. All authors contributed to the article and approved the submitted version.
Funding
This work was funded by DANIDA (grant 17 02 KU) and by the Independent Research Fund Denmark (grant 0134-00123B). HL and ZS were supported by a Postdoctoral Fellowship and a PhD scholarship from the DANIDA-sponsored Building Stronger Universities initiative grant (BSUIII-UG), respectively. The funders had no role in study design, data collection and analysis, decision to publish, or preparation of the manuscript.
Acknowledgments
We thank the parents of the participants for consenting to join this study, and the leaders and household heads for allowing us to enter their communities and homes to conduct this study. We are grateful for the support from GHS staff, our field research team, Alex Danso-Coffie, Nicholas Atsu, Naporo Mathias, and Bernard Tornyigah for technical support. We thank Michael Theisen (University of Copenhagen, Denmark), Asamoah Kusi, and Linda Eva Amoah (University of Ghana) for providing the GLURP, PfCSP, PfMSP3, and Pfs230 recombinant proteins, respectively.
Conflict of interest
The authors declare that the research was conducted in the absence of any commercial or financial relationships that could be construed as a potential conflict of interest.
Publisher’s note
All claims expressed in this article are solely those of the authors and do not necessarily represent those of their affiliated organizations, or those of the publisher, the editors and the reviewers. Any product that may be evaluated in this article, or claim that may be made by its manufacturer, is not guaranteed or endorsed by the publisher.
Supplementary material
The Supplementary Material for this article can be found online at: https://www.frontiersin.org/articles/10.3389/frhem.2023.1150134/full#supplementary-material
Supplementary Figure 1 | IgG levels against crude antigens. (A) crude-specific IgG levels among HbAA, HbAC, and HbAS individuals. (B) crude-specific IgG levels among wildtype, heterozygous, and homozygous α-thalassaemia individuals. IgG responses are expressed in log arbitrary units (AU) on the y-axis. P-values were determined using Kruskal-Wallis test followed by Dunnett’s test for Multiple Comparisons.
References
1. Taylor SM, Parobek CM, Fairhurst RM. Haemoglobinopathies and the clinical epidemiology of malaria: a systematic review and meta-analysis. Lancet Infect Dis (2012) 12(6):457–68. doi: 10.1016/S1473-3099(12)70055-5
2. Luzzatto L. Genes expressed in red cells could shape a malaria attack. Lancet Haematol (2018) 5(8):e322–3. doi: 10.1016/S2352-3026(18)30110-8
3. Modiano D, Bancone G, Ciminelli BM, Pompei F, Blot I, Simporé J, et al. haemoglobin S and haemoglobin c: ‘quick but costly’ versus ‘slow but gratis’ genetic adaptations to plasmodium falciparum malaria. Hum Mol Genet (2007) 17(6):789–99. doi: 10.1093/hmg/ddm350
4. Haldane JBS. The rate of mutation of human genes. Hereditas (1949) 35(S1):267–73. doi: 10.1111/j.1601-5223.1949.tb03339.x
5. Allison AC. Protection afforded by sickle-cell trait against subtertian malareal infection. Br Med J (1954) 1:290-4. doi: 10.1136/bmj.1.4857.290
6. Weatherall DJ, Provan AB. Red cells I: inherited anaemias. Lancet (2000) 355(9210):1169–75. doi: 10.1016/S0140-6736(00)02073-0
7. Modiano D, Luoni G, Sirima BS, Simporé J, Verra F, Konaté A, et al. Haemoglobin c protects against clinical plasmodium falciparum malaria. Nature (2001) 414(6861):305–8. doi: 10.1038/35104556
8. Agarwal A, Guindo A, Cissoko Y, Taylor JG, Coulibaly D, Koné A, et al. Hemoglobin c associated with protection from severe malaria in the dogon of Mali, a West African population with a low prevalence of hemoglobin S. Blood (2000) 96(7):2358–63. doi: 10.1182/blood.V96.7.2358
9. Williams TN, Mwangi TW, Wambua S, Alexander ND, Kortok M, Snow RW, et al. Sickle cell trait and the risk of plasmodium falciparum malaria and other childhood diseases. J Infect Dis (2005) 192(1):178–86. doi: 10.1086/430744
10. Chotivanich K, Udomsangpetch R, Pattanapanyasat K, Chierakul W, Simpson J, Looareesuwan S, et al. Hemoglobin e: a balanced polymorphism protective against high parasitemias and thus severe p falciparum malaria. Blood (2002) 100(4):1172–6. doi: 10.1182/blood.V100.4.1172.h81602001172_1172_1176
11. Willcox M, Björkman A, Brohult J, Pehrson PO, Rombo L, Bengtsson E. A case-control study in northern Liberia of Plasmodium falciparum malaria in haemoglobin S and beta-thalassaemia traits. Ann Trop Med Parasitol (1983) 77(3):239–46. doi: 10.1080/00034983.1983.11811704
12. Weatherall D. From genotype to phenotype: genetics and medical practice in the new millennium. Philos. Trans R Soc Lond B Biol Sci (1999) 354(1392):1995–2010. doi: 10.1098/rstb.1999.0539
13. Piel FB, Howes RE, Patil AP, Nyangiri OA, Gething PW, Bhatt S, et al. The distribution of haemoglobin c and its prevalence in newborns in Africa. Sci. Rep (2013) 3:1671. doi: 10.1038/srep01671
14. Williams TN, Weatherall DJ. World distribution, population genetics, and health burden of the hemoglobinopathies. Cold Spring Harbor Perspect Med (2012) 2(9):a011692. doi: 10.1101/cshperspect.a011692
15. Piel FB, Patil AP, Howes RE, Nyangiri OA, Gething PW, Williams TN, et al. Global distribution of the sickle cell gene and geographical confirmation of the malaria hypothesis. Nat Commun (2010) 1:104. doi: 10.1038/ncomms1104
16. Danquah I, Ziniel P, Eggelte TA, Ehrhardt S, Mockenhaupt FP. Influence of haemoglobins s and c on predominantly asymptomatic plasmodium infections in northern Ghana. Trans R Soc Trop Med Hygiene (2010) 104(11):713–9. doi: 10.1016/j.trstmh.2010.08.001
17. Mockenhaupt FP, Ehrhardt S, Otchwemah R, Eggelte TA, Anemana SD, Stark K, et al. Limited influence of haemoglobin variants on plasmodium falciparum msp1 and msp2 alleles in symptomatic malaria. Trans R Soc Trop Med Hygiene (2004) 98(5):302–10. doi: 10.1016/j.trstmh.2003.10.001
18. Lamptey H, Ofori MF, Adu B, Kusi KA, Dickson EK, Quakyi I, et al. Association between alpha-thalassaemia trait, plasmodium falciparum asexual parasites and gametocyte carriage in a malaria endemic area in southern Ghana. BMC Res Notes (2019) 12(1):134. doi: 10.1186/s13104-019-4181-8
19. Mockenhaupt FP, Ehrhardt S, Gellert S, Otchwemah RN, Dietz E, Anemana SD, et al. Alpha(+)-thalassemia protects African children from severe malaria. Blood (2004) 104(7):2003–6. doi: 10.1182/blood-2003-11-4090
20. Fucharoen S, Winichagoon P. Haemoglobinopathies in southeast Asia. Indian J Med Res (2011) 134(4):498–506.
21. Fairhurst RM, Fujioka H, Hayton K, Collins KF, Wellems TE. Aberrant development of plasmodium falciparum in hemoglobin CC red cells: implications for the malaria protective effect of the homozygous state. Blood (2003) 101(8):3309–15. doi: 10.1182/blood-2002-10-3105
22. Friedman MJ, Roth EF, Nagel RL, Trager W. The role of hemoglobins c, s, and nbalt in the inhibition of malaria parasite development in vitro. Am J Trop Med Hygiene (1979) 28(5):777–80. doi: 10.4269/ajtmh.1979.28.777
23. LaMonte G, Philip N, Reardon J, Lacsina JR, Majoros W, Chapman L, et al. Translocation of sickle cell erythrocyte microRNAs into Plasmodium falciparum inhibits parasite translation and contributes to malaria resistance. Cell Host Microbe (2012) 12(2):187–99. doi: 10.1016/j.chom.2012.06.007
24. Brockelman CR, Wongsattayanont B, Tan-ariya P, Fucharoen S. Thalassemic erythrocytes inhibit in vitro growth of plasmodium falciparum. J. Clin. Microbiol (1987) 25(1):56–60. doi: 10.1128/jcm.25.1.56-60.1987
25. Cyrklaff M, Sanchez CP, Kilian N, Bisseye C, Simpore J, Frischknecht F, et al. Hemoglobins s and c interfere with actin remodeling in plasmodium falciparum-infected erythrocytes. Science (2011) 334(6060):1283–6. doi: 10.1126/science.1213775
26. Krause MA, Diakite SA, Lopera-Mesa TM, Amaratunga C, Arie T, Traore K, et al. α-thalassemia impairs the cytoadherence of plasmodium falciparum-infected erythrocytes. PloS One (2012) 7(5):e37214. doi: 10.1371/journal.pone.0037214
27. Cholera R, Brittain NJ, Gillrie MR, Lopera-Mesa TM, Diakité SA, Arie T, et al. Impaired cytoadherence of Plasmodium falciparum-infected erythrocytes containing sickle hemoglobin. Proc. Natl. Acad. Sci (2008) 105(3):991–6. doi: 10.1073/pnas.0711401105
28. Hviid L, Jensen AT. PfEMP1 - a parasite protein family of key importance in Plasmodium falciparum malaria immunity and pathogenesis. Adv. Parasitol (2015) 88:51–84. doi: 10.1016/bs.apar.2015.02.004
29. Taylor SM, Cerami C, Fairhurst RM. Hemoglobinopathies: slicing the Gordian knot of plasmodium falciparum malaria pathogenesis. PloS Pathog (2013) 9(5):e1003327. doi: 10.1371/journal.ppat.1003327
30. Goheen M, Wegmüller R, Bah A, Darboe B, Danso E, Affara M, et al. Anemia offers stronger protection than sickle cell trait against the erythrocytic stage of falciparum malaria and this protection is reversed by iron supplementation. EBioMedicine (2016) 14:123–30. doi: 10.1016/j.ebiom.2016.11.011
31. Verra F, Simpore J, Warimwe GM, Tetteh KK, Howard T, Osier FH, et al. Haemoglobin c and s role in acquired immunity against Plasmodium falciparum malaria. PloS One (2007) 2(10):e978. doi: 10.1371/journal.pone.0000978
32. Bwire GM, Majigo M, Makalla R, Nkinda L, Mawazo A, Mizinduko M, et al. Immunoglobulin G responses against falciparum malaria specific antigens are higher in children with homozygous sickle cell trait than those with normal hemoglobin. BMC Immunol (2019) 20(1):12. doi: 10.1186/s12865-019-0294-z
33. Nafady H, Eida A, Eida O. Immunological characterization in malaria patients with and without the sickle-cell trait. Adv Infect Dis (2014) 04:152–64. doi: 10.4236/aid.2014.43022
34. Ghana Statistical Service. 2010 population and housing census:. district analytical report: tamale metropolis (2010). Available at: https://www2.statsghana.gov.gh/docfiles/2010_District_Report/Northern/TamaleMetropolitan.pdf2014.
35. Ghana Statistical Service. 2010 population and housing census: northern regional analytical report (20102013). Available at: https://www2.statsghana.gov.gh/docfiles/2010phc/2010_PHC_Regional_Analytical_Reports_Northern_Region.pdf.
36. Baidjoe A, Stone W, Ploemen I, Shagari S, Grignard L, Osoti V, et al. Combined DNA extraction and antibody elution from filter papers for the assessment of malaria transmission intensity in epidemiological studies. Malaria J (2013) 12(1):272. doi: 10.1186/1475-2875-12-272
37. Alvarez OA, Hustace T, Voltaire M, Mantero A, Liberus U, Saint Fleur R. Newborn screening for sickle cell disease using point-of-Care testing in low-income setting. Pediatrics (2019) 144(4). doi: 10.1542/peds.2018-4105
38. Piety NZ, Yang X, Kanter J, Vignes SM, George A, Shevkoplyas SS. Validation of a low-cost paper-based screening test for sickle cell anemia. PloS One (2016) 11(1):e0144901. doi: 10.1371/journal.pone.0144901
39. Oppong M, Lamptey H, Kyei-Baafour E, Aculley B, Ofori EA, Tornyigah B, et al. Prevalence of sickle cell disorders and malaria infection in children aged 1-12 years in the Volta region, Ghana: a community-based study. Malaria J (2020) 19(1):426. doi: 10.1186/s12936-020-03500-5
40. Liu Y, Old J, Miles K, Fisher C, Weatherall D, Clegg J. Rapid detection of alpha-thalassaemia deletions and alpha-globin gene triplication by multiplex polymerase chain reactions. Br. J. Haematol (2000) 108(2):295–9. doi: 10.1046/j.1365-2141.2000.01870.x
41. Theisen M, Soe S, Oeuvray C, Thomas AW, Vuust J, Danielsen S, et al. The glutamate-rich protein (GLURP) of Plasmodium falciparum is a target for antibody-dependent monocyte-mediated inhibition of parasite growth in vitro. Infection Immun (1998) 66(1):11–7. doi: 10.1128/IAI.66.1.11-17.1998
42. Amoah LE, Acquah FK, Ayanful-Torgby R, Oppong A, Abankwa J, Obboh EK, et al. Dynamics of anti-MSP3 and Pfs230 antibody responses and multiplicity of infection in asymptomatic children from southern Ghana. Parasites Vectors (2018) 11(1):13. doi: 10.1186/s13071-017-2607-5
43. Acquah FK, Obboh EK, Asare K, Boampong JN, Nuvor SV, Singh SK, et al. Antibody responses to two new lactococcus lactis-produced recombinant Pfs48/45 and Pfs230 proteins increase with age in malaria patients living in the central region of Ghana. Malaria J (2017) 16(1):306. doi: 10.1186/s12936-017-1955-0
44. Stevenson L, Huda P, Jeppesen A, Laursen E, Rowe JA, Craig A, et al. Investigating the function of fc-specific binding of IgM to Plasmodium falciparum erythrocyte membrane protein 1 mediating erythrocyte rosetting. Cell Microbiol (2015) 17(6):819–31. doi: 10.1111/cmi.12403
45. Ampomah P, Stevenson L, Ofori MF, Barfod L, Hviid L. Kinetics of b cell responses to plasmodium falciparum erythrocyte membrane protein 1 in ghanaian women naturally exposed to malaria parasites. J Immunol (2014) 192(11):5236–44. doi: 10.4049/jimmunol.1400325
46. Lopez-Perez M, Viwami F, Seidu Z, Jensen ATR, Doritchamou J, Ndam NT, et al. PfEMP1-specific immunoglobulin G reactivity among beninese pregnant women with sickle cell trait. Open Forum Infect Dis (2021) 8(12). doi: 10.1093/ofid/ofab527
47. Thomas AG, Torp-pedersen C, Holst KK, & Ozenne B. Publish: format output of various routines in a suitable way for reports and publication. R package version 2020. (2021).
48. Williams TN, Wambua S, Uyoga S, Macharia A, Mwacharo JK, Newton CR, et al. Both heterozygous and homozygous α+ thalassemias protect against severe and fatal Plasmodium falciparum malaria on the coast of Kenya. Blood (2005) 106(1):368–71. doi: 10.1182/blood-2005-01-0313
49. Tetteh M, Addai-Mensah O, Siedu Z, Kyei-Baafour E, Lamptey H, Williams J, et al. Acute phase responses vary between children of HbAS and HbAA genotypes during Plasmodium falciparum infection. J Inflamm Res (2021) 14:1415–26. doi: 10.2147/JIR.S301465
50. Aidoo M, Terlouw DJ, Kolczak MS, McElroy PD, ter Kuile FO, Kariuki S, et al. Protective effects of the sickle cell gene against malaria morbidity and mortality. Lancet (2002) 359(9314):1311–2. doi: 10.1016/S0140-6736(02)08273-9
51. Kreuels B, Kreuzberg C, Kobbe R, Ayim-Akonor M, Apiah-Thompson P, Thompson B, et al. Differing effects of HbS and HbC traits on uncomplicated falciparum malaria, anemia, and child growth. Blood (2010) 115(22):4551–8. doi: 10.1182/blood-2009-09-241844
52. Fowkes FJI, Allen SJ, Allen A, Alpers MP, Weatherall DJ, Day KP. Increased microerythrocyte count in homozygous α+-thalassaemia contributes to protection against severe malarial anaemia. PloS Med (2008) 5(3):e56. doi: 10.1371/journal.pmed.0050056
53. Mockenhaupt FP, Bienzle U, May J, Falusi AG, Ademowo OG, Olumese PE, et al. Plasmodium falciparum Infection: influence on hemoglobin levels in α-thalassemia and microcytosis. J Infect Dis (1999) 180(3):925–8. doi: 10.1086/314959
54. Fairhurst RM, Bess CD, Krause MA. Abnormal PfEMP1/knob display on plasmodium falciparum-infected erythrocytes containing hemoglobin variants: fresh insights into malaria pathogenesis and protection. Microbes Infect (2012) 14(10):851–62. doi: 10.1016/j.micinf.2012.05.006
55. Williams TN, Mwangi TW, Wambua S, Peto TEA, Weatherall DJ, Gupta S, et al. Negative epistasis between the malaria-protective effects of α+-thalassemia and the sickle cell trait. Nat. Genet (2005) 37(11):1253–7. doi: 10.1038/ng1660
56. Gouagna LC, Bancone G, Yao F, Yameogo B, Dabire KR, Costantini C, et al. Genetic variation in human HBB is associated with Plasmodium falciparum transmission. Nat. Genet (2010) 42(4):328–31. doi: 10.1038/ng.554
57. Gonçalves BP, Sagara I, Coulibaly M, Wu Y, Assadou MH, Guindo A, et al. Hemoglobin variants shape the distribution of malaria parasites in human populations and their transmission potential. Sci. Rep (2017) 7(1):14267. doi: 10.1038/s41598-017-14627-y
58. Opi DH, Ochola LB, Tendwa M, Siddondo BR, Ocholla H, Fanjo H, et al. Mechanistic studies of the negative epistatic malaria-protective interaction between sickle cell trait and α+ thalassemia. EBioMedicine (2014) 1(1):29–36. doi: 10.1016/j.ebiom.2014.10.006
59. Obi R, Okangba C, Nwanebu F, Ndubuisi U, Orji N. Premunition in plasmodium falciparum malaria. Afr. J. Biotechnol (2010) 9(10):1397–401. doi: 10.5897/AJBx09.034
60. Brasseur P, Badiane M, Cisse M, Agnamey P, Vaillant MT, Olliaro PL. Changing patterns of malaria during 1996-2010 in an area of moderate transmission in southern Senegal. Malaria J (2011) 10(1):203. doi: 10.1186/1475-2875-10-203
61. Ceesay SJ, Casals-Pascual C, Erskine J, Anya SE, Duah NO, Fulford AJC, et al. Changes in malaria indices between 1999 and 2007 in the Gambia: a retrospective analysis. Lancet (Lond Engl) (2008) 372(9649):1545–54. doi: 10.1016/S0140-6736(08)61654-2
62. Fowkes FJ, Boeuf P, Beeson JG. Immunity to malaria in an era of declining malaria transmission. Parasitology (2016) 143(2):139–53. doi: 10.1017/S0031182015001249
63. Dodoo D, Aikins A, Kusi KA, Lamptey H, Remarque E, Milligan P, et al. Cohort study of the association of antibody levels to AMA1, MSP1 19, MSP3 and GLURP with protection from clinical malaria in ghanaian children. Malaria J (2008) 7(1):1. doi: 10.1186/1475-2875-7-142
64. Jones S, Grignard L, Nebie I, Chilongola J, Dodoo D, Sauerwein R, et al. Naturally acquired antibody responses to recombinant Pfs230 and Pfs48/45 transmission blocking vaccine candidates. J. Infection (2015) 71(1):117–27. doi: 10.1016/j.jinf.2015.03.007
65. Bejon P, Warimwe G, Mackintosh CL, Mackinnon MJ, Kinyanjui SM, Musyoki JN, et al. Analysis of immunity to febrile malaria in children that distinguishes immunity from lack of exposure. Infect Immun (2009) 77(5):1917–23. doi: 10.1128/IAI.01358-08
66. Dodoo D, Atuguba F, Bosomprah S, Ansah NA, Ansah P, Lamptey H, et al. Antibody levels to multiple malaria vaccine candidate antigens in relation to clinical malaria episodes in children in the kasena-nankana district of northern Ghana. Malaria J (2011) 10(1):108. doi: 10.1186/1475-2875-10-108
67. Kusi KA, Aguiar J, Kumordjie S, Aggor F, Bolton J, Renner A, et al. Antigenicity and immune correlate assessment of seven plasmodium falciparum antigens in a longitudinal infant cohort from northern Ghana. Sci. Rep (2019) 9(1):8621. doi: 10.1038/s41598-019-45092-4
68. Cabrera G, Cot M, Migot-Nabias F, Kremsner PG, Deloron P, Luty AJF. The sickle cell trait is associated with enhanced immunoglobulin G antibody responses to plasmodium falciparum variant surface antigens. J Infect Dis (2005) 191(10):1631–8. doi: 10.1086/429832
69. Miura K, Diakite M, Diouf A, Doumbia S, Konate D, Keita AS, et al. Relationship between malaria incidence and IgG levels to plasmodium falciparum merozoite antigens in malian children: impact of hemoglobins s and c. PloS One (2013) 8(3):e60182–e. doi: 10.1371/journal.pone.0060182
70. Sarr JB, Pelleau S, Toly C, Guitard J, Konaté L, Deloron P, et al. Impact of red blood cell polymorphisms on the antibody response to plasmodium falciparum in Senegal. Microbes Infect (2006) 8(5):1260–8. doi: 10.1016/j.micinf.2005.12.005
71. Le Hesran JY, Personne I, Personne P, Fievet N, Dubois B, Beyemé M, et al. Longitudinal study of plasmodium falciparum infection and immune responses in infants with or without the sickle cell trait. Int. J. Epidemiol (1999) 28(4):793–8. doi: 10.1093/ije/28.4.793
72. Tan X, Traore B, Kayentao K, Ongoiba A, Doumbo S, Waisberg M, et al. Hemoglobin s and c heterozygosity enhances neither the magnitude nor breadth of antibody responses to a diverse array of plasmodium falciparum antigens. J Infect Dis (2011) 204(11):1750–61. doi: 10.1093/infdis/jir638
Keywords: antibodies, asymptomatic infection, parasite carriage, Plasmodium falciparum, α-thalassaemia, haemoglobinopathies, Ghana
Citation: Lamptey H, Seidu Z, Lopez-Perez M, Kyei-Baafour E, Hviid L, Adjei GO and Ofori MF (2023) Impact of haemoglobinopathies on asymptomatic Plasmodium falciparum infection and naturally acquired immunity among children in Northern Ghana. Front. Hematol. 2:1150134. doi: 10.3389/frhem.2023.1150134
Received: 23 January 2023; Accepted: 10 April 2023;
Published: 25 April 2023.
Edited by:
Anna Rita Migliaccio, Campus Bio-Medico University, ItalyReviewed by:
Camille Roussel, Assistance Publique Hopitaux De Paris, FranceMahamadou Diakite, des Techniques et des Technologies de Bamako, Mali
Copyright © 2023 Lamptey, Seidu, Lopez-Perez, Kyei-Baafour, Hviid, Adjei and Ofori. This is an open-access article distributed under the terms of the Creative Commons Attribution License (CC BY). The use, distribution or reproduction in other forums is permitted, provided the original author(s) and the copyright owner(s) are credited and that the original publication in this journal is cited, in accordance with accepted academic practice. No use, distribution or reproduction is permitted which does not comply with these terms.
*Correspondence: Helena Lamptey, aGxhbXB0ZXlAbm9ndWNoaS51Zy5lZHUuZ2g=
†These authors have contributed equally to this work