- 1NASA Goddard Space Flight Center, Greenbelt, MD, United States
- 2Jet Propulsion Laboratory, California Institute of Technology, Pasadena, CA, United States
- 3NASA Ames Research Center, Moffett Field, CA, United States
- 4KapScience, LLP, Tewksbury, MA, United States
- 5Danell Consulting, Inc., Winterville, NC, United States
- 6Laboratoire Atmosphères, Milieux, Observations Spatiales (LATMOS), Guyancourt, France
- 7Honeybee Robotics Spacecraft Mechanisms Corp., Altadena, CA, United States
- 8Mini-Mass Consulting, Hyattsville, MD, United States
- 9University of Maryland, Baltimore County, Baltimore, MD, United States
- 10ATA Aerospace, Greenbelt, MD, United States
- 11Laboratoire Interuniversitaire des Systèmes Atmosphériques (LISA), Université Paris-Est, Créteil, France
- 12CentraleSupélec, Université Paris-Saclay, Paris, France
The Europan Molecular Indicators of Life Investigation (EMILI) is an instrument concept being developed for the Europa Lander mission currently under study. EMILI will meet and exceed the scientific and technical/resource requirements of the organic composition analyzer identified as a core instrument on the Lander. EMILI tightly couples two complementary analytical techniques, based on 1) liquid extraction and processing with capillary electrophoresis and 2) thermal and chemical extraction with gas chromatography, to robustly detect, structurally characterize, and quantify the broadest range of organics and other Europan chemicals over widely-varying concentrations. Dual processing and analysis paths enable EMILI to perform a thorough characterization of potential molecular biosignatures and contextual compounds in collected surface samples. Here we present a summary of the requirements, design, and development status of EMILI with projected scientific opportunities on the Europa Lander as well as on other potential life detection missions seeking potential molecular biosignatures in situ.
Introduction
Jupiter’s moon Europa holds promise as a potential abode of extraterrestrial life within its global subsurface ocean. The presence of abundant liquid water (greater volume even than on Earth), available energy, and chemical reservoirs together at Europa meet hypothesized criteria for habitability and for the possibility of biology of an origin utterly independent of Earth’s (National Research Council, 1999; Chyba and Phillips, 2002). Following the pathfinding discoveries of Europa’s unique features during the Galileo mission (Pappalardo et al., 2009) and from telescopic observations (Roth et al., 2014; Sparks et al., 2016), NASA is now preparing to launch the Europa Clipper flagship mission (planned for 2024), that will thoroughly analyze Europa’s habitability and provide surface maps of topography and composition from a multiple-flyby spacecraft (Howell and Pappalardo 2020; Jackson et al., 2020). Such data would support the final planning of a proposed surface mission directly focused on astrobiology. The Europa Lander under study (Hand et al., 2017) would perform detailed analysis of solid (ice-rich) samples from a carefully selected site hosting near-surface materials potentially in recent (e.g., Ma timescales) contact with the subsurface ocean. Such samples would be investigated for any potential biosignatures, as direct evidence of organisms or indirectly indicating possible pre-biotic or biogenic processes in the ocean, that have survived transport to the surface and its associated damaging radiation conditions. While pre-defining what is or is not a Europan biosignature may appear as a daunting task, recent directions in astrobiology have begun to put potential evaluation criteria on a rigorous footing (National Academies of Sciences, Engineering, and Medicine (NASEM), 2019; Neveu et al., 2018; Pohorille and Sokolowska 2020).
Detection of molecular biosignatures is the most powerful and universal analytical approach to the search for extraterrestrial life (National Academies of Sciences, Engineering, and Medicine (NASEM), 2017; National Academies of Sciences, Engineering, and Medicine (NASEM), 2019; Summons et al., 2008; Hand et al., 2017). Potential molecular biosignatures (PMBs) can be sought directly from a sample in situ using a set of complementary techniques to acquire an extensive, ideally complete, chemical inventory. This is possible not only by searching sensitively for the presence of established biomarkers (e.g., biomolecular units and informational polymers), but also by surveying a broadly representative profile of all organic molecular species and structures to reveal abundance and structural patterns that may comprise a collective PMB. By casting a sufficiently wide net of chemical analysis, even fundamentally “alien” PMBs may be discovered. Assessing the populations of molecules and their properties enables a thorough and potentially robust means for PMB identification and interpretation.
The Europan Molecular Indicators of Life Investigation (EMILI) is an instrument concept for detection and characterization of potential molecular biosignatures in solid samples acquired on the Europa Lander mission. EMILI seeks to achieve both analytical breadth, as motivated by the need for a comprehensive molecular analysis of unknown samples, and very high sensitivities for astrobiologically important targeted species such as amino acids, fatty acids, and key light gases. EMILI is designed to make these measurements with a low-risk, flight-compatible design matching the available Europa Lander resource envelope and concept of mission operations.
Scientific Measurement Requirements and Approach
The top-level Europa Lander Objective 1A: “Detect and characterize any organic indicators of past or present life” leads directly to two core Investigations (1A-1 and 1A-2) and associated Measurement Requirements (1A-1.1 through 1A-2.2), fully addressed by EMILI, as follows from Hand et al. (2017):
“1A-1: Determine the abundances and patterns (i.e., population distributions) of organic compounds in the sampled material, with an emphasis on identifying potentially biogenic characteristics.
1A-1.1 Determine the presence, identities, and relative abundances of amino acids, carboxylic acids, lipids, and other molecules of potential biological origin (biomolecules and metabolic products) at compound concentrations as low as 1 pmol in a 1 g sample of Europan surface material.
1A-1.2 Determine the broad molecular weight distribution to at least 500 Da (Threshold) and bulk structural characteristics of any organics at compound concentrations as low as 1 pmol in a 1 g sample of Europan surface material.
1A-2: Determine the types, relative abundances, and enantiomeric ratios of any amino acids in the sampled material.
1A-2.1 Identify and detect (at 1 nM LOD*) at least eight of the following amino acids: Ala, Asp, Glu, His, Leu, Ser, Val, Iva, Gly, β-Ala, GABA, and AIB, with at least one from each representative class (abiotic, biotic, proteinogenic). Note that for chiral amino acids, limit of detection is 1 nM for each of the two different chiral forms.
1A-2.2 Quantify abundances of all amino acids detected relative to glycine (Gly) at an accuracy of better than or equal to 2%.
1A-2.3 Quantify enantiomeric excess (ee) of at least three proteinogenic amino acids, one abiotic amino acid, and histidine, with an accuracy of 5% or better.”
These requirements call for the combination of organic molecule detection and quantification, as well as structural characterization of both targeted (e.g., amino acid) and untargeted (and composite) PMBs. Investigation 1A-1 covers the range of possibilities that exist for biogenic chemicals or patterns thereof to be expressed in the Europan environment and distinguished from abiotic chemistry. These could comprise known patterns, such as an even-odd carbon number bias in the hydrocarbon chains of fatty acids, or general identifiers such as bioselection of a very narrow sub-range of all possible molecular weights or isomers in a structurally related series of PMBs. Investigation 1A-2 highlights the central position that amino acids–their types, abundance ratios, and enantioselectivity–play in considerations of extraterrestrial biochemistry. On Earth, the measurements of requirement 1A-2 together are sufficient to establish biogenicity. While it is not necessarily expected that Europan life should utilize precisely the amino acid set of terrestrial biology, in precisely the same proportions, etc. it is acknowledged that a targeted investigation of these compounds and characteristics represents a plausible and potentially highly robust hypothesis-driven search for building blocks of extant Europan life. Amino acids are plentiful established precursors available from the protosolar nebula and/or primitive parent body chemistry, and are manifestly effective in the role of monomer units in the aperiodic crystal (Schrödinger 1944) (e.g., informational polymer) hypothesis of universal life, in particular forming peptide bonds which, in conjunction with chiral preference, form the basis of protein biochemistry.
In the Europa Lander report (Hand et al., 2017), Objective 1A additionally calls for a third Investigation 1A-3: “Determine whether the carbon stable isotope distribution among organic and inorganic carbon is consistent with biological activity,” with one associated Measurement Requirement 1A-3.1: “Measure the carbon stable isotope composition of multiple compounds, compound classes, or pools of carbon with a relative standard deviation of no greater than 5‰ (5 per mille) in each measurement. Note that to achieve such measurements for organic compound concentrations as low as 1 pmol per gram, the LOD would need to be at most 10 fmol per gram to measure 13C/12C in a C1 compound.” This investigation is nominally categorized as a Baseline but not a Threshold component of 1A; that is, the mission is conceived to include 1A-3 in its design, however it is still considered scientifically complete and viable without it, should simplifications be necessary for successful implementation within cost and schedule limits. Briefly, the reasoning is that while isotopic data are extremely powerful, with identification of isotopically light carbon (δ13C < 0) in some organics potentially associated with biology (as it is on Earth), the result is subject to ambiguity. Lacking assured isotope systematics across the Europan environment to establish clear fractionation statistics of multiple pools of carbon, the a priori false-positive rate may be too high, when compared to results from 1A-1 and 1A-2, which in principle can be assessed locally. As such, in the interest of simplicity, the EMILI design does not presently carry requirements to address Investigation 1A-3. The instrument is theoretically capable of making these measurements; however, doing so robustly with performance margin may lead to resource levels (mass, power, time) that are less likely to fit within allocated mission constraints. This pragmatic limitation in no way implies that the value of isotopic studies in understanding Europa should be discounted. Stable isotope ratios for H, C, O, S, and the noble gases are of particular value to understanding the evolution and habitability of Europa, when compared with Jovian, solar, and terrestrial planet reservoirs. Future work will assess the resource-performance trade for 1A-3 as well as other isotope systems of interest.
EMILI also supports a separate Europa Lander Objective 1D: “Determine the provenance of sampled material” via its chemistry-associated Investigation 1D-2: “Characterize the chemistry of the near-subsurface to determine the endogenous versus exogenous origin of the sample, and any surface processing of potential biosignatures.” While not seeking to detect potential molecular biosignatures directly, this investigation does provide critical data to help understand the likelihood that any molecular species found derive from the subsurface ocean vs. from external, circum-Jovian sources, as well as the degree of radiolytic processing/alteration these compounds may have endured. Such information can be partially inferred from analysis of inorganic ions (such as salts) and gases (such as H2O2, CO2, H2S, etc.), as well as the distribution of potentially degraded organic compounds themselves, thus providing environmental context for assessment of potential habitability and biosignatures. It was broadly required by the Europa Lander Science Definition Team (SDT) report that salts and light gases should be analyzed with a limit of detection of one part per thousand (ppth) by weight, assuming as in 1A that this measurement is taken from a collected bulk sample of approximately 1 g.
Following these Lander requirements, analytical targets of EMILI may be considered as a nested set, represented graphically in Figure 1. The broadest inventory of chemicals - All Species - include those providing contextual information that directly supports 1D-2. Within this realm is a population of organic compounds that exhibit the variety of structurally related Organic Classes that may include PMBs identifiable by their abundance distributions, complexity, or telltale functionality, directly supporting 1A-1. Furthermore, among these are a subset of key Targeted Organics, particularly amino acids and fatty acids, that merit focused attention for their value in constituting a PMB. Their analysis directly supports 1A-2 (which is exclusive to amino acids) as well as 1A-1. Moving from the outer to inner circle in Figure 1, as the breadth of analysis required decreases, the analytical figures of merit become more stringent. For example, standard error requirements for quantitative analysis of abundance and enantiomer ratios of amino acids at concentrations as low as nanomolar (nM) levels would need to be quite tight (relative standard deviations of a few %), necessitating high signal-to-noise ratios and reproducibility (Hand et al., 2017).
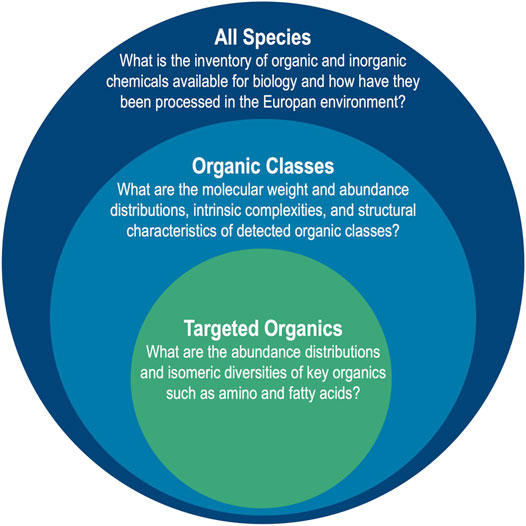
FIGURE 1. EMILI addresses molecular analysis requirements of the Europa Lander with a nested approach including broad characterization of All Species for context, and more focused and stringent analyses of organic compounds that could comprise a PMB. The need to answer questions associated with each target drives the design of EMILI instrumentation and the necessary processing of its data.
Extraction and clear detection of molecular species from extraterrestrial materials, unknown in advance, is a major challenge in designing in situ planetary instruments due to the enormous variety of potential species and sample properties. However, it is possible to avoid significant detection “blind spots” by considering two useful chemical parameters that describe chemical phase space in a robust and systematic way: solubility and volatility (Figure 2). The equilibrium solubility of organics in liquid water (brine) at Europa varies widely over a range of important molecular structures in correlation to their polarities: nonpolar hydrocarbons have very low water solubilities (below 10−2 mol L−1) while polar organics such as many carboxylic acids as well as salts expected at Europa, such as MgSO4, are highly water soluble (∼1 mol L−1). Some example priority species that may be found on Europa over this range are depicted in Figure 2. Independently, species mixed in Europa’s ice-rich surface may range over a wide range of volatility, expressed inversely in Figure 2 as the molar heat (or enthalpy) of vaporization, ΔHvap. Compounds with the lowest ΔHvap may be trapped as gas pockets within ice or minerals (e.g., CO2, CH4) or may enter the gas phase under modest heating (e.g., short aliphatic hydrocarbons, alcohols, benzene) while species with ΔHvap in the hundreds of kJ mol−1 range are nonvolatile (e.g., most polycyclic aromatic hydrocarbons (PAHs), peptides) and may or may not thermodesorb intact, depending on their chemical nature and structure.
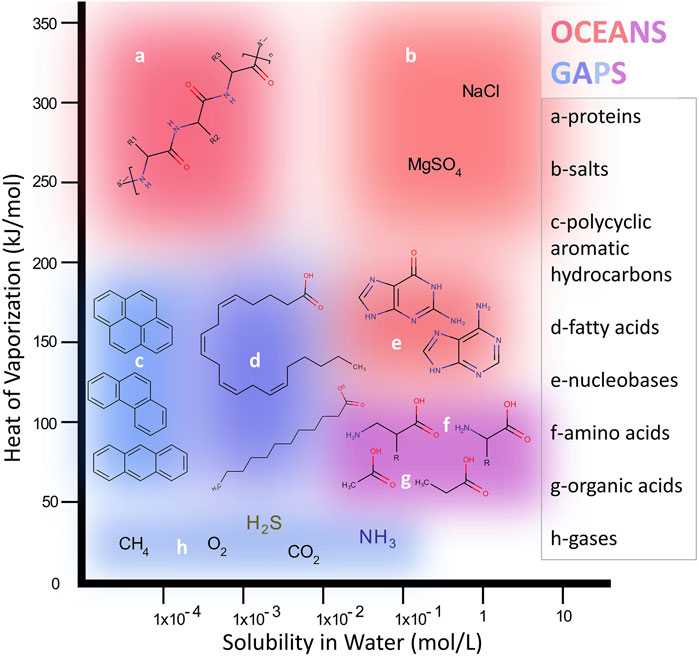
FIGURE 2. The two-dimensional molecular phase space spanned by water solubility and heat of vaporazation illustrates the wide range of compounds targeted for analysis on Europa. Different example classes (a–h) fall within zones that are more or less amenable to analysis by the two subsystem modes of EMILI: OCEANS primarily covering the upper/upper-right region and GAPS primarily covering the lower/lower-left region. Some compounds such as amino acids are analyzed by both approaches, which provides more robust identification as well as some science redundancy.
The strategy for EMILI is thus to provide maximal coverage of the phase space in Figure 2, through a complementary combination of front-end and analytical instrument capabilities based on optimized chemical extraction, separation, and detection steps in both liquid and gas phase. This is achieved by combining analyses by chemical derivatization gas chromatography (GC) and subcritical water extraction capillary electrophoresis (CE) protocols, interfaced to a common high-sensitivity mass spectrometer (MS). The GC protocol, realized in the EMILI Gas Analysis Processing System (GAPS), is most amenable to analysis of lower polarity, volatile and semi-volatile molecules, primarily in the lower and lower-left regions of Figure 2, notably including the fatty acids (d) accessible via thermochemolysis. GC-MS has been applied successfully on a number of planetary missions to Mars and Titan. The CE protocol, realized in the EMILI Organic Capillary Electrophoresis ANalysis System (OCEANS), is most amenable to detection of polar/water-soluble and less-volatile organics, primarily in the upper and upper-right regions of Figure 2. While new to spaceflight, CE robustly enables sensitive multi-detector analysis of the water-soluble fraction via liquid-based separation, most compatible with Ocean Worlds astrobiology. As such, merged gas/GC and liquid/CE protocols provide an optimal strategy for EMILI and a unique approach for future potential astrobiology missions.
EMILI Design Overview
EMILI comprises three analytical subsystems: OCEANS, GAPS, and an ion-trap mass spectrometer (ITMS), along with a main electronics box (MEB). A simplified block diagram is shown in Figure 3. Ice-rich (cryogenic) particulate sample obtained by a cutting and scooping tool on the lander’s arm is delivered to EMILI in cups, via the Collaborative Acceptance and Distribution for Measuring Europan Samples (CADMES) system (Malespin et al., 2020). In the baseline design, OCEANS and GAPS feature distinct cup types, each nominally accepting up to 1 g of sample. A several-cup cache of each type is thus intended to be stored within CADMES. The GAPS cup is loaded into a Pyrolysis Oven for both heating and chemical derivatization preparation for GC-MS. The OCEANS cup is loaded into a Liquid Extraction Module (LEM) for subcritical water extraction preparation for CE. Details are provided in the respective subsystem sections below. Concepts for a single common cup design serving both GAPS and OCEANS are in development and will be considered following planned EMILI performance vs. complexity trade studies. At a high level, GAPS provides the sample processing and analyte separation required for GC-MS (and evolved gas analysis, EGA), with the ITMS providing the sole GAPS molecular detection method. OCEANS provides liquid-based extraction via the LEM, preparation and delivery of the sample via the Sample Processing Unit (SPU), and electrophoretic separation to enable three independent detection methods for each sample: Capacitively-Coupled Contactless Conductivity Detection (C4D), Laser-Induced Fluorescence (LIF), and Mass Spectrometry via Electrospray Ionization (ESI) (also termed CE-MS). These multiple detection modes, detailed below, provide the complete and complementary analytical set enabling the EMILI strategy to meet mission objectives. The MEB includes modules to provide overall power and functional DC voltages, command and data handling (CDH) including data storage and autonomous initial analysis, valve and heater control, and micro-control of GAPS, OCEANS, and ITMS electrode voltage sequences which are programmed through parameterized CDH-driven scripts. AC voltage control for ion trapping and manipulation is provided separately and in close proximity to its application in the ITMS.
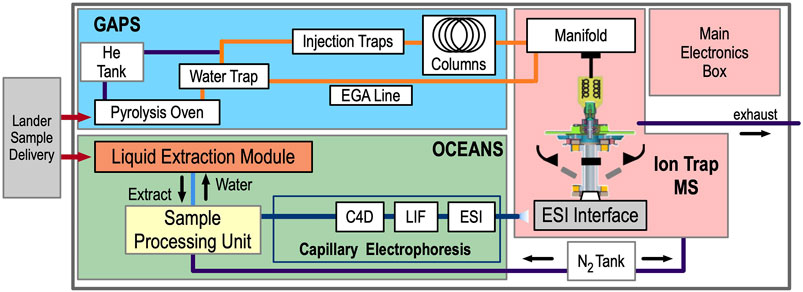
FIGURE 3. The EMILI block diagram illustrates the organization of the instrument into GAPS, OCEANS, ITMS, and MEB. Sample delivery and gas exhaust interfaces are shown only notionally, and electrical and thermal interfaces are not shown, for simplicity.
EMILI is designed to comply with all Lander-specified resource constraints, including the shape and volume of payload accommodations. A preliminary concept for the mechanical packaging configuration of EMILI is depicted in Figure 4. The bounding pink volume indicates the approximate “large instrument” envelope available in the Lander’s radiation-protected vault, wherein various instruments would be positioned to interface with CADMES to accept and analyze delivered sample. As shown in Figure 4, the EMILI instrumentation fits in the available volume with margin. In addition to the shown sample cup interface (with a notional delivery axis), EMILI additionally requires access for vent ports to low external pressure (ultimately the hard space vacuum at the Europan surface) and electrical connections to the Lander power and data subsystems (not shown). Assemblies of the main subsystems of EMILI: OCEANS, GAPS, ITMS, and MEB are indicated with color coded labels.
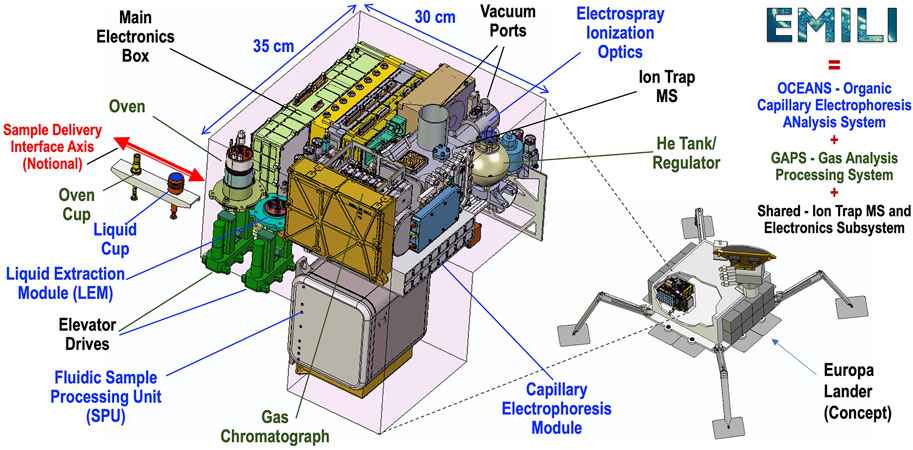
FIGURE 4. Mechanical packaging concept for EMILI, providing example configuration that accommodates volume envelope (pink box) provided by the Europa Lander vault (relative position depicted roughly in lower right lander image). EMILI comprises OCEANS, GAPS, and the (shared) ITMS and MEB subsystems indicated by blue, green, and black labeling, respectively. The notional positioning and interface axis of the Sample Delivery elements–Oven Cup for GAPS and Liquid Cup for OCEANS–illustrate a “deep port” concept where both cups are installed through the same vault wall penetration to individual receiving stations (with cup elevators as shown). Wire harnesses and narrow-gauge plumbing lines are not shown in this image.
While a complete description of other Lander mission-imposed requirements on EMILI, including electrical, thermal range, mechanical vibration and shock, and planetary protection aspects, is beyond the scope of this paper, the EMILI investigation is on track to meet all within system margins. Many of these are derived from prior flight instrument development heritage, such as for Mars and Moon missions. One particular challenge worth mention here is the extremely high-dose-rate ionizing radiation environment in the Jovian system, including at the surface of Europa. Any unshielded hardware on the Europa Lander will receive on the order of several megarad (Mrad) of total ionizing dose (TID) of charged-particle flux that has been dramatically accelerated by the Jovian magnetosphere, over a far greater span of kinetic energies than achieved simultaneously in any Earth particle accelerator. As such, all critical instrumentation and electronics that would otherwise be ravaged by such TID are housed within a protective multilayer metallic shield called a “vault.” The much-reduced TID level that must be tolerated by instruments in the vault is 150 krad (normally doubled to 300 krad for testing with 100% engineering margin). EMILI subsystems have been developed with this requirement in mind. The vault notwithstanding, one aspect of initial concern, based on prior work, was the stability of the various chemical reagents within EMILI against radiolytic degradation over all mission phases, including multi-year interplanetary cruise and pre-landing Jupiter orbits, as well as during surface operations. Through a series of published experimental tests over the past several years coordinated by EMILI subteams at GSFC, JPL, and ARC, we have demonstrated that the critical molecular reagents, including mass spectrometer calibrants, GC and CE derivatization agents, fluorescent tags, and other components in the OCEANS subsystem are not significantly degraded by the 300 krad TID (Creamer et al., 2018; Freissinet et al., 2019).
OCEANS–Organic Capillary Electrophoresis ANalysis System
The EMILI OCEANS subsystem is a liquid-based chemical analyzer for low-limit-of-detection measurements of water-soluble organics. As indicated (Figure 3), OCEANS comprises the LEM, the SPU, and the complete CE system including both LIF and C4D detection subsystems as well as the electrospray interface to the ITMS. OCEANS realizes within EMILI the integrated product of many years of scientific and technical development of CE-based capabilities for planetary missions led by the Jet Propulsion Laboratory and partners at NASA Ames Research Center and at Honeybee Robotics, now achieving a high technology readiness level (TRL) for mission implementation (e.g., Willis et al., 2015; Creamer et al., 2017; Mora et al., 2021).
CE is a high-resolution analytical separation technique for molecules in solution. CE separations occur within small fused slica capillaries, requiring miniscule amounts of sample and reagents (nl and μl respectively). CE is the simplest of all liquid separation techniques to implement for planetary science because the hardware does not require packed resin columns, functionalized coatings that can degrade with time, or high-pressure pumps (Willis et al., 2015). CE basically requires a glass capillary and a low-power high-voltage (HV) source. The HV drives the separation by generating electro-osmotic flow (EOF) – a bulk flow of the solution inside the capillary. This flow ensures that all molecules (negative, positive or neutral) travel from the entrance to the exit of the capillary. Within this flow, molecules separate according to differences in electrophoretic mobility (a function of charge/shape).
OCEANS Methods
Each of the three detection modes has specific CE method(s) developed for complementary classes of molecular targets to cover the widest possible range of biosignatures and chemical context markers. Figure 5 provides examples of OCEANS data in each of the modes on mixtures of amino acids, carboxylic acids, and inorganic salts in Europa analog samples. Each mode is described below.
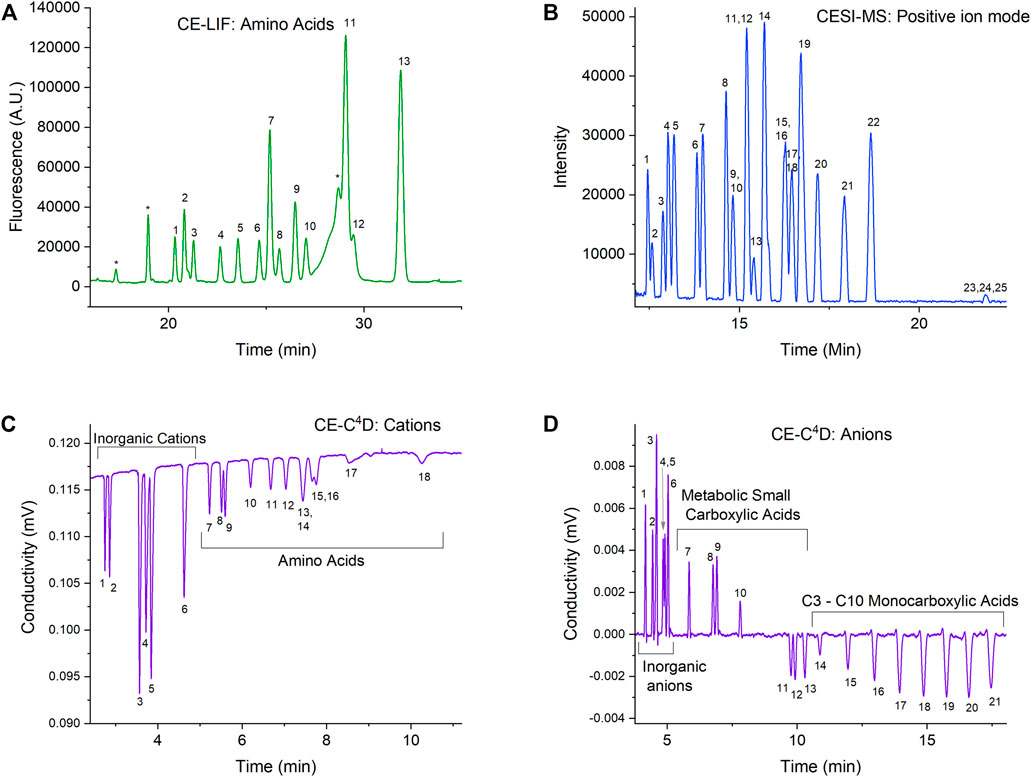
FIGURE 5. OCEANS example data from analysis of mixtures of amino acids, carboxylic acids, and inorganic salts in Europa analog samples using EMILI-proxy commercial CE instrumentation and ground-support mass spectrometer. Numbered peaks in fluorescence at 50 nM (A), mass spectrometry at 50 μM (B), cation conductivity at 100 μM (C), and anion conductivity at 100 μM (D) indicate separated compounds in grouped categories (individual labels not shown for simplicity). Astrobiologically-important amino acids indicated (1–13) in CE-LIF spectrum (A) follow those of Creamer et al. (2017).
CE-LIF is used for separating enantiomeric forms of amino acids and detecting them at very low concentrations (nM), taking advantage of high-sensitivity LIF detection. We have previously demonstrated chiral separation of the 13 most astrobiologically relevant amino acids and validated this method by analyzing amino acids (5–25 nM) in hypersaline samples without desalting or preconcentration (Creamer et al., 2017). We have also shown that the performance of the reagents needed for both the CE separations and the fluorescent labeling of amino acid analytes are not affected by the radiation levels expected during the lifetime of a mission to Europa (Creamer et al., 2018). In addition, we have shown that the reagents and fluorescent dye are also stable for years when stored dry at moderate temperatures, making them suitable for use after the long transit times required by planetary missions (Creamer et al., 2019).
The CE method for ESI-MS allows detection of a wide range of polar organics that are not optimally analyzed by GC-MS (Figure 2). CE is an ideal separation method to couple with ESI, since the performance of ESI significantly improves with a reduction of flow to the “nanospray” regime (Schultz and Moini 2003). We have recently demonstrated CE-MS analysis of a mixture of 25 compounds including amino acids, peptides, nucleosides, and nucleobases as an example of what a broad-based positive ion mode can achieve during a mission (Mora et al., 2021). This method is tolerant to high concentrations of salts, as we demonstrated separation performance of solutions at half saturation for both sodium chloride and magnesium sulfate. Like the reagents needed for CE-LIF, we have also shown that the simple one-component background electrolyte solution needed for this method, 5 M acetic acid, is stable under Europa Lander mission TID levels and for years of storage without special handling (Ferreira Santos et al., 2021).
Conductivity detection using C4D allows a broad capability for the label-free detection of charged compounds. Taking advantage of this, we have developed two CE-C4D methods targeting either positively charged or negatively charged analytes. The cation method allows simultaneous separation of inorganic cations such as sodium, magnesium, calcium etc. and positively charged organic ions such as amino acids and amines at suitably low pH (Ferreira Santos et al., 2018). The anion method enables simultaneous separation of inorganic ions such a chloride, phosphate, nitrate etc., carboxylate anions such as formate, oxalate, malate, and short-chain fatty acids from C3 to C10 (Jaramillo et al., 2021). Both methods are broadly salt tolerant to allow direct analysis of natural samples. The cations method uses a background electrolyte that is identical to that of the CE-MS method, and therefore is stable under required radiation and storage conditions. In addition, this allows for the two detection modes to operate simultaneously in one capillary at the same time as the C4D detector is noncontact and nondestructive and can be placed just upstream of the electrospray tip.
OCEANS Hardware
The core of the OCEANS hardware is a capillary-based CE system compatible with all three detection modes, described in detail previously (Zamuruyev et al., 2021). Briefly, the system is based on rotor-stator style valves to allow robust sample injection with mechanical decoupling of the high voltage (HV) electrode reservoir from the rest of the fluidic system. In addition, a pneumatic gas supply can dry out the appropriate fluidic pathways to provide reliable HV isolation during CE separations. This system implements all CE methods needed for the different detection modes.
The LIF detection system is a fiber-coupled confocal epifluorescence approach, similar to our microchip electrophoresis system (Mora et al., 2020). The differences are that a 488-nm laser and micro-photomultiplier tube (PMT), along with the appropriate wavelength dichroic and clean up filters, have been selected based on the fluorescent dye used for CE-LIF method described above. We have also shown that the laser and PMT combination survives the TID of the Europa Lander Mission concept, as well as maintains the necessary signal-to-noise-ratio (SNR) for single-digit nM detection of amino acids when powered on under ionizing radiation dose rates that would be experienced on Europa’s surface (Oborny et al., 2021).
For CE-MS, the ITMS system is described in detail below. The separation capillaries have 100/30 µm O.D./I.D. with an etched porous electrospray tip at the end of the separation capillary (SCIEX, Brea, CA, United States). A second conductive buffer capillary is used to complete the electrical circuit for CE, making the electrical connection through the porous glass just upstream of the electrospray tip. An interface volume allows control of the gas composition, temperature, pressure, and humidity in the electrospray region in front of the entrance to the ITMS.
The C4D detector is based on the design originally published by da Silva and do Lago (Fracassi da Silva and do Lago 1998). The design has since been adapted to allow simultaneous or serial use of up to three capillaries if multiplexing. The circuit has also been redesigned keeping in mind the much more limited selection of parts available for spaceflight missions with 300 krad TID survival requirements. With careful parts selection we have demonstrated that a high-performance C4D detector can be designed to be compatible with challenging Europa Lander environmental conditions (Hand et al., 2017).
The overall layout of EMILI uses a multi-capillary approach so that one can be dedicated to CE-LIF and the chiral amino acid method, and one can be used for both C4D and CE-MS measurements by taking advantage of their identical background electrolyte for the cation/positive ion electrospray methods. A high-fidelity CAD of the OCEANS system including the rotor stator valves, integrated CE HV power supply (HVPS) and electrode reservoirs, LIF laser, detector, and electronics, and C4D detector along with how they package for coupling to the SPU is shown in Figure 6.
OCEANS operation begins at the LEM, which allows high-temperature extraction (200°C) to release adsorbed organics and lyse any cells. These extraction methods have been described in detail previously (Amashukeli et al., 2007; Noell et al., 2018; Kehl et al., 2019), and more details on the design and performance of the LEM are provided below. Extracted sample is then drawn into the SPU using an internal piston pump. The SPU is capable of all the reagent storage and fluidic processing needed to enable OCEANS analysis, including determining overall sample matrix composition (conductivity, pH, ORP etc.) to evaluate if the sample can be concentrated (or needs to be diluted) ahead of organic analysis. And while it uses a different fluidic platform, the SPU takes advantage of the methods for automated fluorescent labeling and CE analysis of amino acids demonstrated previously (Mora et al., 2020). First, both the sample and a blank are simultaneously reacted with the fluorescent dye. Then three consecutive injections and subsequent CE-LIF analysis are performed on each sample, followed by one injection of the blank to verify that low-level contaminants have not accumulated in the fluidic microchannels during transit. After CE-LIF analysis, another aliquot of sample is mixed with CE-MS buffer and the capillary system is prepared for CE-MS analysis, similarly operated with triplicate injections of sample and one of blank. C4D analysis occurs at the same time as CE-MS, and can help identify the nature of specific cations in the sample and hence any salt clusters that may appear in the MS data. At the conclusion of CE analyses, the SPU will rinse the pathways with water and then dry gas to remove liquid from the system to prepared it for safe below-freezing storage until the next sample is ready for analysis.
SPU–Sample Processing Unit
The SPU, which provides all fluidic manipulations required to retrieve thermally-treated and extracted samples from the LEM and to prepare and provide samples for analysis by OCEANS, leverages the integrated (micro)fluidics technologies of the Sample Processor for Life on Icy worlds (SPLIce). This technology includes development and/or qualification, including relevant-environment testing (radiation: 300 krad; shock and vibration: General Environmental Verification Standard levels; temperature: various ranges for survival and operation) of microfluidic components comprising active valves, check valves, pumps, concentrators, bubble traps, mixers, high-surface-area dry-reagent storage-and-reconstitution polymer monoliths, aqueous-phase pressure sensors, and fluidic connectors, along with monolithic multilayer fusion-bonded fluidic manifolds that provide microchannel connections and robust integration of components in a compact form factor (Chinn et al., 2017; Chin et al., 2018; Willis et al., 2018; Radosevich et al., 2019). SPLIce technologies, in turn, are built based on Ames Research Center’s cubesat/small-payload microfluidics heritage, including five successful Earth-orbiting astro- and space-biology missions (Ricco et al., 2007; Nicholson et al., 2011; Ricco et al., 2011; Ehrenfreund et al., 2014; McCutcheon et al., 2016; Padgen et al., 2020), the pending BioSentinel mission—destined for year-long operation in interplanetary space (Ricco et al., 2020; Padgen et al., 2021)—and three small-payload technology-development projects (Fleming et al., 2014; Tan et al., 2011; Park et al., 2017). Together, this work comprises 15 years of development, qualification, and successful flight operation of microfluidic systems for the support of biological experimentation and analysis in space.
A block diagram representation of the SPU is shown in Figure 7. Due to the long-duration cruise of an outer-planets mission, the SPU stores liquids in metal-enclosed flight-proven fluoropolymer bags connected to high-heritage, ultra-low-leak-rate solenoid-operated valves, now being qualified for use with the particular liquids required by OCEANS: water (including freeze/thaw cycles), acetic acid, and an acetonitrile/water mixture. Buffers and conditioning reagents are stored as freeze-dried powders in fluoropolymer bags; SPLIce experiments show that this approach yields complete redissolution on shortened timescales. Labeling dyes for LIF are stored in lyophilized form on several independently accessible porous poly (ethylene) monoliths built into the manifold; the SPU is housed inside a hermetic container (not shown), filled during integration for flight with ultra-high purity N2, preventing degradation of the dyes by oxygen or water over the long storage period. This approach provides a high surface-area:volume environment with short diffusion lengths that enable rapid labeling; the acceleration factor is currently being characterized.
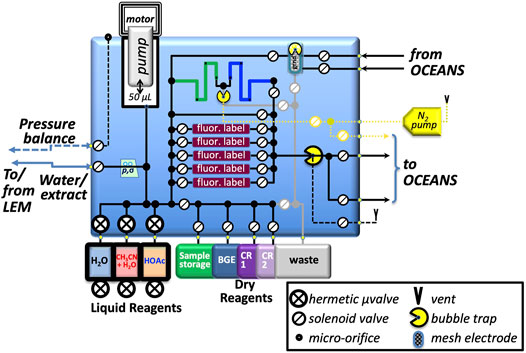
FIGURE 7. Sample-Processing Unit utilizes a multilayer, precision-machined, thermally-fused polycarbonate manifold with 1-mm diameter internal channels and vias to integrate and interconnect the fluidic components, including: LEM, from which the SPU retrieves thermally-extracted sample; metering pump; hermetic liquid and dry reagent storage; fluorescent reagent sample-labeling compartments for CE-LIF; interfaces to HV and ground electrodes to drive CE separations; N2 pump for pneumatic pressure-driven injections and to provide gas-phase gaps to isolate the manifold when HV is energized. BGE, background electrolyte; CR1 and CR2 are conditioning reagents for the CE capillaries; pressure and ionic conductivity sensors denoted p, σ, respectively.
The SPU provides a pressure-driven injection capability (Figure 7, N2 pump and bubble trap at upper center of manifold) for accurate, pulse-free low-volume injections for CE separation: control of valve open time with this approach provides sub-nl resolution of injection plugs. The system uses a gas pump that operated throughout a 6-month spaceflight mission that implemented a very similar pressure-based fluid-delivery system (Ehrenfreund et al., 2014). The serpentine sample-storage channel (dark blue) in the manifold and connected bubble trap couples the pressurized N2 to the (labeled) sample for delivery to the CE system.
LEM–Liquid Extraction Module
Solid samples for OCEANS will be delivered by CADMES in a metallic OCEANS cup that interfaces with the LEM to permit extraction followed by preparation for CE by the SPU (Figure 8). A hermetic seal is made by compression of a compliant gasket at the cup lip to the LEM port by means of an elevator mechanism. After the cup is sealed to the LEM, the enclosed sample is brought to the SPU operating temperature and pressure (25°C and 1 bar) and ∼3 ml of ultra-high purity, sterile water, providing a 3:1 water:sample ratio, is transferred from the SPU to accommodate mineral/salt-rich samples and hasten extraction. The LEM is heated to the sub-critical water extraction temperature and pressure (∼200°C/15.5 bar) to release adsorbed organics and lyse any cells. After extraction, the LEM is cooled to 25°C and the pressure matched to the 1 bar operating pressure of the SPU, which then draws in the sample with its metering pump. The SPU measures the total ionic conductivity of the sample to identify if further dilution is required, then prepares the sample for analysis as described above.
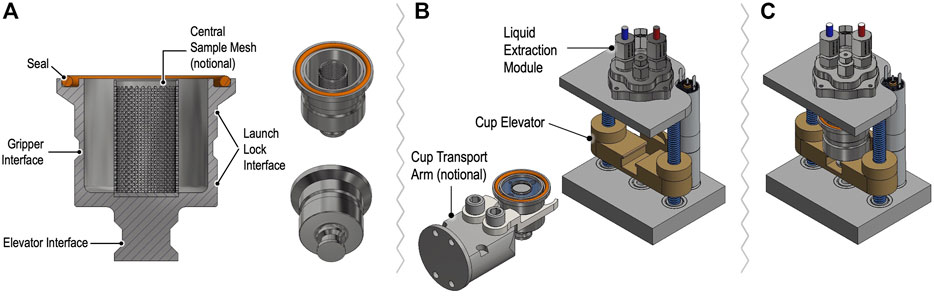
FIGURE 8. Concept for OCEANS sample cups and Liquid Extraction Module assembly showing cup cross section (A) with features designed for manipulation by the CADMES sample-handling system, with notional cup gripping transport arm (B). Notional internal mesh sleeve shown is under consideration for testing optimal mixing and extraction processing in the presence of potential mineral particulates. The elevator assembly in (B,C) accepts and seals the cup vertically. Sealing gasket is depicted as an elastomer o-ring for trade study of possible re-use of demountable liquid cups.
GAPS–Gas Analysis and Processing System
The EMILI Gas Analysis Processing System (GAPS) is a gas-based chemical analysis subsystem that enables low-limit-of-detection measurements of lower-polarity volatile and semivolatile organics via GC-MS and selected mineral phases via EGA-MS. As indicated (Figure 3), GAPS comprises the Pyrolysis Oven and He Tank, a Water Trap, and the complete GC system including Injection Trap and GC Columns with (nominally) splitless injection to the source of the ITMS. GAPS additionally includes a dedicated leak manifold between the oven subsystem and the ITMS for direct EGA. GAPS realizes within EMILI the integrated product of many years of scientific and technical development of GC/GC-MS capabilities for planetary missions through a partnership between NASA Goddard Space Flight Center and French laboratories LISA and LATMOS, with recent and upcoming flight experiments (e.g., Mahaffy et al., 2012; Goesmann et al., 2017; Barnes et al., 2021).
GAPS Methods
GAPS coupled to MS offers a state-of-the-art method for identifying PMBs complementary to those analyzed by CE, including biomolecular patterns and structural selectivity. Through chemical derivatization, less-volatile species such as fatty acids and amino acids are also amenable to GC-MS, providing an independent analysis for science confidence and robustness.
The oven cups utilised by GAPS are designed to heat samples delivered from CADMES up to 600°C. This temperature is lower than maxima of 1100°C for the Sample Analysis at Mars (SAM) investigation (Mahaffy et al., 2012) or nominally 800°C for Mars Organic Molecule Analyzer (MOMA) (Goesmann et al., 2017), which are designed to enable pyrolysis of martian rock samples. EMILI does not require high-temperature mineral breakdown to achieve its GAPS-related analytical objectives, and with the lower temperature operation, EMILI/GC-MS consumes significantly less power than the Mars protocols. Two GC stationary phases provide broad detection sensitivity for enantiomeric separation of both derivatized and non-derivatized molecules (GC-1, Chirasil Dex) and for light-to-heavier volatile and semi-volatile organics and inorganics, of low to medium polarities (GC-2, MXT-5-type). The latter column in particular is versatile enough to separate medium to high molecular weight (m.w.) organics including aromatic hydrocarbons, thermochemolyzed fatty acids, as well as providing general organic characterization that enables resolution of common isobaric interferences. Moreover, the use of two columns maximizes breadth of selectivity and provides redundancy.
The gas chromatograph separates gaseous compounds transferred from the Pyrolysis Oven prior to their analysis with the mass spectrometer. Eluted analytes (ideally single species) enter the MS over time and their individual spectra are recorded sequentially. The retention time of different compound classes separated with a given GC column (over a nominal duration of 10s of minutes) is characteristic of the species and can be calibrated precisely with peak widths on the order of fractions of a second to a few seconds. This combination of a pre-separation with mass spectrometry detection provides two-dimensional data allowing confident chemical identification over a wide effective dynamic range. The GAPS GC design seeks to maximize this performance with careful materials selection, column conditioning, minimizing excess reagent and water, and thermal control, which when combined with the advanced EMILI ITMS (below), allows the system to achieve LOD and dynamic range at least an order of magnitude improved over MOMA.
Wet Chemistry: The EMILI baseline employs two liquid reagent-based methylation reactions for derivatization of the compounds of interest. Examples of EMILI derivatization GC-MS protocol chromatograms are shown in Figure 9. The first approach uses N,N-dimethylformamide dimethylacetal (DMF-DMA), which reacts at the site of labile protons, such as on amino or carboxylic acids. This one-step reaction (proceeding rapidly at 140°C) leads to products easily detected by GC-MS. Futhermore, this derivatization reaction preserves any asymmetric center(s) of the molecules, facilitating chiral separations on GC-1. Enantiomeric excess (ee), or the degree of imbalance between left- and right-handed chiral forms, can be measured over a wide range with uncertainties (relative standard deviations) as low as a few % for most compounds, sufficient to distinguish potentially biogenic patterns even among degraded (partially-racemized) compounds or in mixtures. Figure 9 (lower panel) shows separation (in retention time on GC-1 following DMF-DMA treatment) of three example amino acids–proline, aspartic acid, and cysteine–as detected with the MS (total ion count shown). When combined with the high-precision quantification of total abundance and ee of amino acids to nM LODs by OCEANS (LIF), the GAPS/GC-1 analysis can provide an independent, cross-checked measurement that will be an important aspect leanding confidence to conclusions about biogenicity. DMF-DMA derivatization is included on the MOMA instrument that will operate on Mars in 2023 (Goesmann et al., 2017).
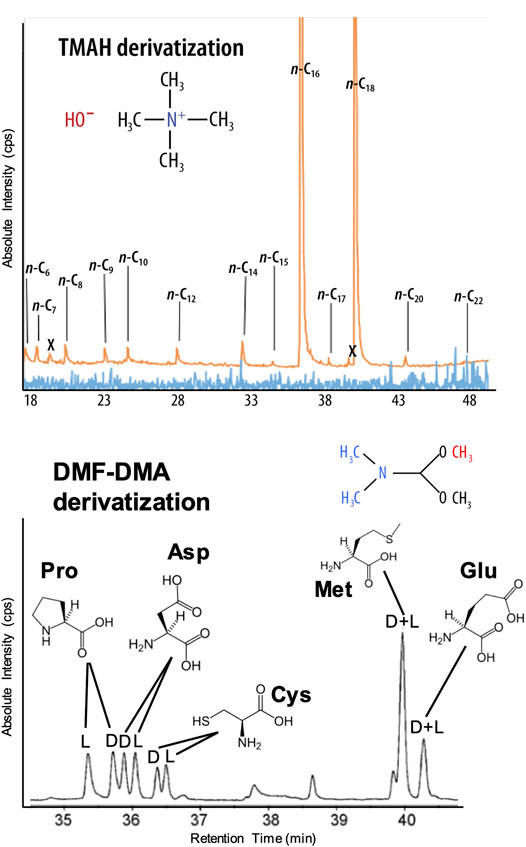
FIGURE 9. EMILI’s GC-MS protocol enabled by GAPS and ITMS provides broad TMAH thermochemolysis detection of fatty acids (top) and DMF-DMA derivatization analysis of amino acid enantiomers (bottom) in an ocean hydrothermal vent water sample. For comparison the blue trace is from GC-MS without TMAH thermochemolysis. These protocols can be automatically run sequentially on a split individual sample or following a science-dependent decision tree based on real-time data.
The second method is a one-step thermochemolysis (proceeding over 300–600°C) employing tetramethyl-ammonium hydroxide (TMAH) methylation, effective for analysis of long-chain carboxylic acids, derived from the lipid building blocks of cell membranes, as well as other, more refractory moieties (such as kerogen-like compounds) that could help identify organics originating from abiotic synthesis mechanisms (and found in meteorites and interplanetary/cometary dust). As a thermally-assisted hydrolysis/methylation technique, TMAH thermochemolysis provides a less-destructive (lower-temperature) cleavage of ester and ether bonds in these compounds, compared with direct high-temperature pyrolysis, yielding sensitive detection of higher molecular weights (up to hundreds of g/mol (u)). Figure 9 (upper panel) shows TMAH thermochemolysis derivatization-enabled analysis of fatty acids in a dilute terrestrial ocean water analog sample, exhibiting clear even-over-odd chain-length abundance bias that can be a hallmark of cellular wall molecular structure. TMAH thermochemolysis is incorporated on both SAM and MOMA.
Evolved Gas Analysis (EGA): Gases evolved from samples, from immediate vacuum sublimation and as the sample is heated to 600°C, are analyzed via both GC columns, as well as directly in the MS via EGA, which detects low-m.w. gases–both hypervolatiles such as CO2, CH4, and CO trapped in ice, and possible mineral-breakdown products such as O2 and SO2. EGA is also being used during development to continue investigation of ITMS carbon isotope performance. EGA mode has proven extremely valuable on SAM for minerals, molecular abundances, and isotopes (Glavin et al., 2013; Leshin et al., 2013; Ming et al., 2014; McAdam et al., 2020). The low conductance leak (split ratio) of EGA gas into the ITMS would also allow an independent check of the quantity of water ice remaining in the oven cup, which may be used autonomously to determine the degree of water exposure and trapping for optimization of the GC-MS protocol.
GAPS Hardware
Samples for GAPS are delivered in dedicated metallic oven cups to the Pyrolysis Oven. Figure 10 depicts a simplified model of a GAPS cup compatible with delivery by the CADMES system to an EMILI station that supports motorized elevator insertion into the oven, engaging a knife edge to seal hermetically to a Cu gasket at the rim of the cup. The oven consists of an insulated sleeve with embedded wrapped heater wire and fixtures for admitting regulated He gas and transporting entrained evolved gas into the GAPS manifold (not shown for simplicity). Maintaining the single oven assembly as part of GAPS, rather than designing each cup as an integrated oven sleeve (as done in MOMA), dramatically simplifies the cup design, contamination control management, and interface with the Lander. The trade-off of this choice is a modest lag in the sample temperature when heated via the external sleeve, which for EMILI is not a serious performance driver. In the baseline design, similar to MOMA, GAPS cups contain small internal metallic capsules welded to the bottom of the cup. The capsules are pre-filled with either of the derivatization agents, the baseline for prototype development. Concepts for dual derivatization and post-sample capsule selection, also under development, could lead to more flexible, science-dependent protocols.
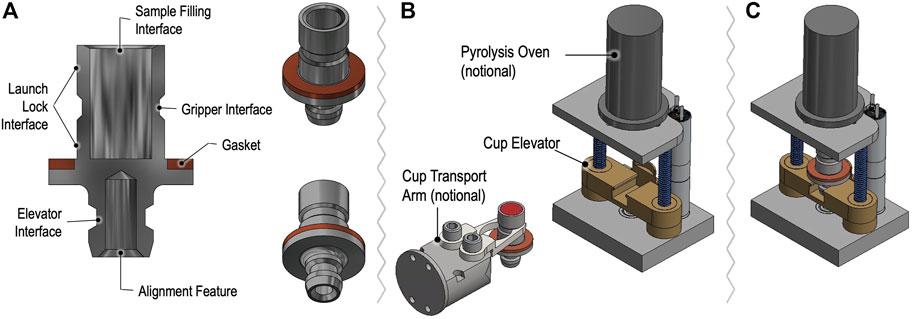
FIGURE 10. Concept for GAPS sample cups and oven assembly showing cup cross section (A) with features designed for manipulation by the CADMES sample handling system, with (B) notional cup gripping transport arm. The elevator assembly in (B,C) accepts and seals the cup vertically.
To achieve the best separation of the analytes, the gas chromatograph is composed of separate trap/injection and column/separation stages, along with a manifold allowing dynamic routing of gaseous flow into the instrument depending on the analytical step. Figure 11 shows a 3D drawing of a prototype GC that serves as a pathfinder for the more flight-like GC engineering test unit (ETU) under staggered development for the integrated EMILI ETU. The prototype is designed to operate on the bench; as such, selected components such as multi-port valves and manifolds require a sealed housing to enable efficient heating for analyte transport in He carrier gas (not required in the flight instrument that operates in a low pressure environment), and utilize non-flight motor drives and mounting layouts, following lessons learned from MOMA development. He gas tanks are not shown. A pressure control microvalve is used to actively adjust the flow of helium (typically from 1 to a few ml min−1) through the pathway using pressures measured at key points in the GAPS manifold. The GAPS ETU architecture will also be similar to the engineering model GC of the DraMS instrument on the Dragonfly mission, permitting efficient exchange of testing experience between the two projects.
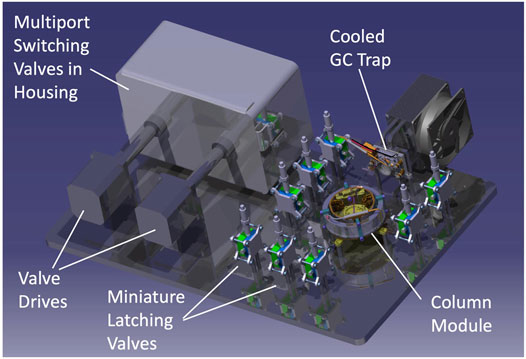
FIGURE 11. Model of the prototype GC with flight-like functionality but designed for ease of testing in a laboratory environment with various GAPS and ITMS interfaces under development. Most inter-component plumbing lines and all electrical harnesses not shown for simplicity. Housing permits bulk gases to be processed at high temperatures without excessive heater power losses to atmosphere. The single-column module is interchangeable, permitting testing of different column types and conditions.
Desorbed and derivatized gas-phase analytes are transferred to the injection system composed of two thermal-desorption traps. These traps are fabricated using a tube filled with an adsorbent powder such as Tenax© TA and/or Tenax© GR, which, when initially cooled to ∼0°C, trap a wide range of organic molecules, with Tenax© GR biased to lighter molecules compared to Tenax© TA. Nominal trap dimensions of 5 cm in length and 1 mm internal diameter, following SAM (Mahaffy et al., 2012), contain few tens of mg of adsorbent. Analytes are trapped at low temperature with unidirectional flow-through. Once sampling is done, the carrier gas flow is reversed to desorb the molecules and the trap is flash heated to ∼300°C in a few seconds. This heated back-flush protocol yields rapid release of compounds and subsequent sharp injection pulse, resulting in optimal GC peak separation.
Once the injection trap desorption is complete, the analytes are carried through a chromatographic column for separation, with performance dependent on the dimensions of the columns, the stationary phase bonded to the inner wall of the column, the types of analytes, and the operating conditions (primarily temperature and flow rate). The general-purpose column (GC-2, MXT-5; Restek) and chiral column (GC-1, CP-Chirasil Dex; Agilent) are coiled stainless steel capillaries, for robustness and ease of integration, in the 20–30 m length range (to be finalized) and 0.25 mm internal diameter. Each column is assembled in a cylindrical mechanical module incorporating a heater and a temperature sensor to control column temperature actively throughout an experiment. Column temperatures typically follow a programmed ramp from GAPS baseline (∼30–40°C, pre-heated from the instrument chassis in the -20°C–0°C range) up to ∼300°C at approximately 10°C min−1. Analytes elute from the end of the column to the mass spectrometer inlet manifold, which includes a fixed conductance split into the electron ionization source. The transfer line and manifold volume are minimized and maintained at high temperature to prevent pulse broadening or loss of analytes prior to analysis.
In the flight design, all GC components are interconnected in a dense arrangement of uniformly heated metallic lines and bistable microvalves used to select the flow pathway. Such technology is already used in MOMA and is currently being adapted to DraMS. GC manifolds are integrated on a metallic plate and heated to prevent analyte condensation and loss. The operating temperature for analyte flow lines is targeted at 180°C. Through valve programming, it is possible to operate the GC in single-flow mode (one injection trap into one column for MS analysis) or in a variety of multiplex modes (such as directing different oven temperature cuts into separate injection traps or performing sequential injections from one trap into two columns). Testing with Europa analogs over a range of concentrations will allow optimization of protocols for the mission baseline.
ITMS–Ion Trap Mass Spectrometer
The EMILI ITMS detects positive and negative ions (via both electron ionization (EI) and ESI) with masses in the m/z 20 to 800 range and with a mass resolution better than 0.5 Da (i.e., maximum peak full width at half maximum, FWHM, of less than 0.5 Da over the m/z range). Having a single mass analyzer and associated electronics interfaced with separate front-end separation and ionization techniques is critical for EMILI, as this leads to the most compact and low-resource approach to analyze the widest breadth of samples (from GAPS and OCEANS), while permitting quantitative inter-comparison of GC-MS and CESI-MS spectra. The dual ionization techniques and mass analyzer capabilities enable the detection, identification, and analysis of small elemental species as well as complex, high-molecular-weight compounds at sub-ppbw concentrations. The EMILI mass range extends lower than MOMA (with a MOMA GC-MS low-mass cutoff of m/z 50) by using slightly higher frequency; however, the fundamental radio-frequency (RF) power supply design is the same as MOMA. The addition of negative polarity (anion) detection in EMILI further expands the range of detectable compounds, such as carboxylic acids and alcohols that may be more electronegative and/or stable to electron attachment. Importantly, the ITMS imports all the capabilities for tandem mass spectrometry, or MS/MS, developed for the MOMA investigation. During MS/MS, an ion of choice is isolated and subsequently fragmented, permitting a clear association of fragment ion masses with structural features of the original molecule. This technique is expected to play an important role in the full analysis of unknowns across both modes of EMILI for potential biogenic structural patterns. A full exposition of the application of MS/MS in EMILI analysis of Europa analog samples, currently in development, will be provided in a future report.
ITMS Hardware
The EMILI linear ion trap itself (breadboard assembly shown in Figure 12) is largely a copy of the MOMA ion trap. Its design and operation have been described in detail (Goesmann et al., 2017). The analyzer comprises four short hyperbolic rods in a quadrupolar arrangement, bounded by endcap electrodes, that permit external ions from different sources to be admitted from either end. The sensor includes two detectors positioned on either side of one opposing pair of rods incorporating narrow slits for ion ejection. Each detector comprises a high voltage dynode, a buffer electrode, and a channel electron multiplier, providing high sensitivity over the mass and charge range, dual polarity ion detection capability, as well as redundancy. The EI source is slightly modified from MOMA (which draws heavily from quadrupole-based instruments on Cassini, MAVEN, and MSL missions), allowing access to very short ionization times, which increases dynamic range, important for EMILI. In combination with additional EMILI-specific modifications such as full-sensor high-temperature bakeouts and high-precision gas control, the projected ITMS limit of detection is below 1 pmol g−1 equivalent of sample effluent introduced to the GC, or more than one order of magnitude lower than on MOMA (GC-MS mode), to meet derived Europa requirements.
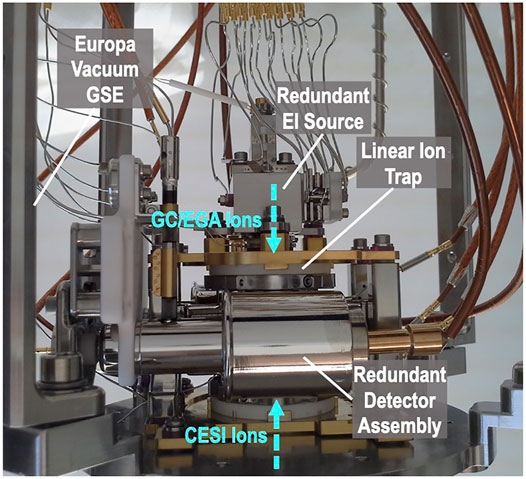
FIGURE 12. EMILI linear ion trap sensor breadboard, side view showing dual-polarity ion detector in front of trap electrode assembly, accessed above by a dual-filament EI source (for GCMS) and below by the CESI source, via multi-stage ion optics (shown in Figure 13).
Novel miniature ESI ion optics are required to interface the OCEANS electrospray output with the opposing end plate of the ITMS while transferring ions across several orders of magnitude in pressure into the analyzer all within a constrained length (Figure 13). These optics are somewhat more involved than the EI transfer optics: two intermediate pressure regions with distinct RF-based ion guides are utilized to maintain efficient ion transfer from the ∼1 bar spray chamber from OCEANS to the ∼0.004 mbar (3 mTorr) operating pressure of the linear trap. The ion optical configuration draws from terrestrial/commercial instrument developments combined with prior space instrument miniaturization efforts that inform mechanical scaling approaches while managing environmental and mission constraints.
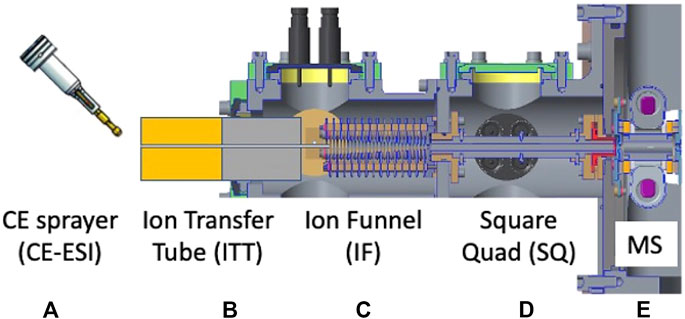
FIGURE 13. Cross-sectional diagram of the EMILI nano-ESI ion trap mass spectrometer. Instrument consists of (A) capillary electrophoresis sprayer to electrospray ionization (CE-ESI), (B) the ion transfer tube (ITT), (C) the ion funnel (IF), (D) the square quad (SQ), and (E) the ion trap mass spectrometer (MS).
The OCEANS nano-ESI sprayer (modeled as a generic sprayer in Figure 13) brings the CE module output into precise alignment with the interface optics. When electrospray voltage is applies at the end of the separation capillary, charged nebulized sample droplets exit the sprayer at few-nanoliter min−1 rates and are drawn toward the grounded mass spectrometer inlet through a heated ion-transfer tube (ITT). Ions, drawn from microscopic electrospray droplets under nominally ∼1 bar of N2, enter the ITT and eventually undergo a supersonic gas expansion when the pressure decreases nearly 2000-fold. Ions are then captured in an RF ion funnel (IF), which captures divergent ions and brings them close to the central axis of the instrument. Following the IF, ions are then more gently transferred into an RF quadrupole ion guide with square cross-section electrodes, referred to as the Square Quad (SQ). Ions are substantially cooled in this region and are thus amenable to efficient transfer into the ITMS analyzer at modest energy through a set of low-voltage ion lenses at the exit of the SQ. This stage can be flexibly designed and may prove particularly critical later in development if it is determined that the ion flight path needs to be steered through an angle to comply with instrument geometry requirements.
The main differences in the nano-ESI ion optical stages, compared with commercial designs, are reduced dimensions to meet volume and mass constraints of the EMILI instrument on the Lander. As such, lens assemblies are mechanically scaled moderately, limited somewhat by nonlinear size effects (e.g., the ion beam dispersion does not scale proportionally with the electrodes) and the need to maintain throughput performance. The ITT inner diameter is roughly 60% smaller than most commercial instruments. The resulting reduced gas load is important for limiting gas consumption, however potential sensitivity losses must then be managed carefully in the overall experiment. Additionally, the EMILI CE-MS design has only three separately-pumped pressure regions, also to save mass and volume, compared to commercial designs that generally include an additional vacuum region between the IF and the SQ. As such, the overall configuration of the EMILI instrument must be considered carefully as part of the ion optical design in order to maximize conductance of passive vent ports to the high-vacuum environment of the Europa Lander (or other airless body environment). In the lab, the payload environment is approximated with dedicated pumps for each pressure stage to match the pumping speed expected in the port arrangement of the mechanical package (shown partially in Figure 4).
ITMS Spectra
Preliminary test spectra for each ion source of the ITMS are presented here to illustrate data from miniature (flight-scale) prototype instrumentation in a laboratory setting using analysis protocols and constraints that are expected on a mission. The GC-MS and EGA-MS modes produce spectra via 70 eV electron ionization of ingested gas. Basic ITMS checkouts in this mode are typically performed using the volatile compound perfluoro-tributylamine (PFTBA), also known as FC-43, diluted in He gas (in the ppmv to sub-ppmv range). PFTBA has also been used as a flight (in situ) mass calibration gas for Mars mass spectrometers; for example, it is carried on MOMA. It is a candidate compound for an in situ calibrant on EMILI as well. Figure 14 shows an example early PFTBA spectrum from the EMILI ITMS, used to verify the basic instrument build meets functionality and performance requirements. Peak positions of “raw” data (m/z derived from initial calculation based on ejection voltage ramp) are generally accurate within the 10−2–10−1 u range, meeting specification; calibration in situ (such as with PFTBA itself) can be used to assure and maintain mass accuracy of all compounds under flight conditions (and to track any drift) through small corrections. Mass resolution of ∼0.2–0.4 u (FWHM) in this spectrum meets requirements. While not considered “high mass resolution” mass spectrometry, the accuracy across the mass range (e.g., including parent molecular ions and their fragment ions) is sufficient to confidently assign compounds from separated peaks (from GC or CE), as well as to associate peaks of organics arising from 13C which occurs at the ∼1% relative abundance level for C1 compounds, etc.
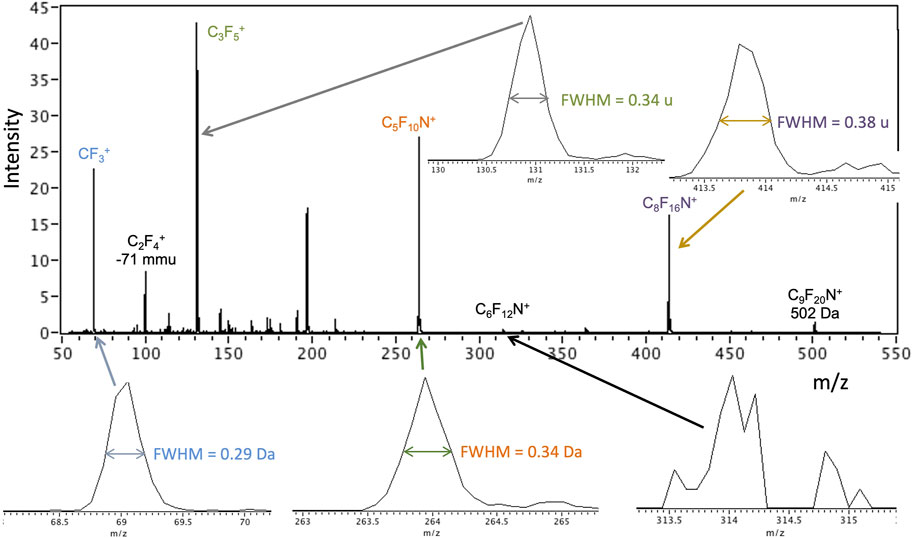
FIGURE 14. EMILI ITMS scan of mass calibration compound perfluoro-tributylamine (PFTBA) at ppm by volume level in He bath gas using the filament emission-based 70 eV EI mode that is applied in GAPS GC-MS and EGA-MS analysis. Insets show example peak shapes with widths indicated.
The nano-ESI mode of the ITMS has been checked using potential calibrants as well as example amino acid and peptide analog analytes through direct infusion from a spray source modeling the full OCEANS CE output. Full OCEANS-ITMS coupling results will be the focus of a subsequent publication. Figure 15 provides illustrative demonstration of both positive and negative ion mode EMILI nano-ESI-MS spectra of histidine (at a nominal 1 μM concentration; LoDs well below this level are being established through tuning). When the MS is appropriately tuned, single-species spectra are typically clean with minimal background and high SNR, suggesting detection can improve dynamically (potentially autonomously) via co-adding over an integration time (CE peak width), with further potential to supplement multi-amino acid detection capabilities of the OCEANS LIF mode. The histidine ion is detected as a protonated parent (M+H)+ and a protonated dimer (2M+H)+ for positive-ion mode, and corresponding deprotonated species in anion spectra, with the corresponding mass shifts that, through correlation of both polarities, can engender high confidence for assignment of such compounds in a potential mixture.
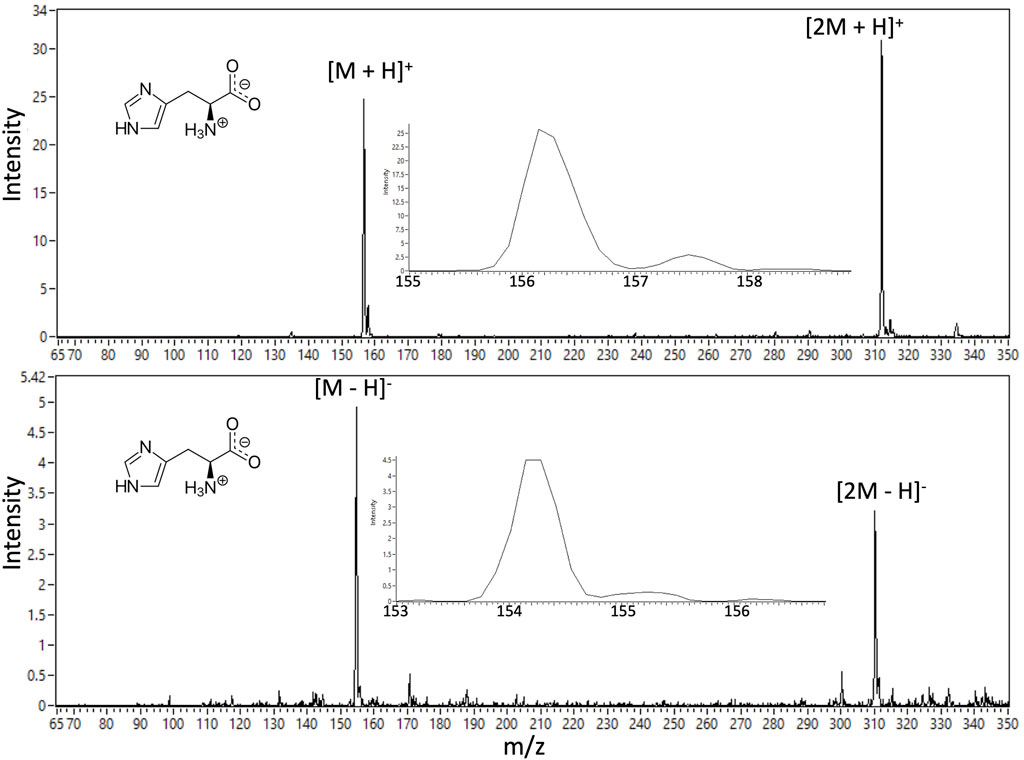
FIGURE 15. Example EMILI ESI-ITMS prototype spectra for amino acid histidine (m.w. 155.2 u) at 1 μM concentration. The parent compound is detectable in positive-ion mode (protonated) and in negative ion mode (deprotonated) by switching the bias voltage of the ESI sprayer tip relative to the ITMS ITT inlet (as well as other ion optics and detector potentials). Histidine dimers are also detected in each spectrum.
Among other analysis objectives, the potential detection of hetero-oligomers, generally, and oligopeptides as prime examples, could indicate prebiotic or biological synthesis when coupled with other supporting data. The EMILI ITMS has the m/z range, of at least 800, to detect some oligopeptides, readily separated by CE, directly. Larger species are also directly detectable by taking advantage of the tendency of ESI to produce multiply-charged ions. That is, the m/z value of compounds larger than 800 u with z > 1 may be less than 800 u/e. Such an example is shown in Figure 16 with the analysis of octapeptide angiotensin II (monoisotopic mass 1045.5 u), through detection of the 2+ and 3+ ions of the protonated parent. The analytical challenge identifying the structure of such higher m.w. species is addressed in part through fragment analysis. While ESI is considered a softer ionization method, compared with EI, spectra of readily-cleaved species such as these frequently exhibit multiple minor peaks corresponding to losses of one or more amino acid units, termed b-ions or y-ions depending on their position on the chain (amino vs. carboxyl terminus, respectively), or a-ions for those broken between C atoms. Preliminary analysis of such peaks can inform selection of peaks to be further isolated, in a series of spectra, and subjected to MS/MS analysis, potentially allowing de novo sequencing of unknown oligomeric species found on Europa.
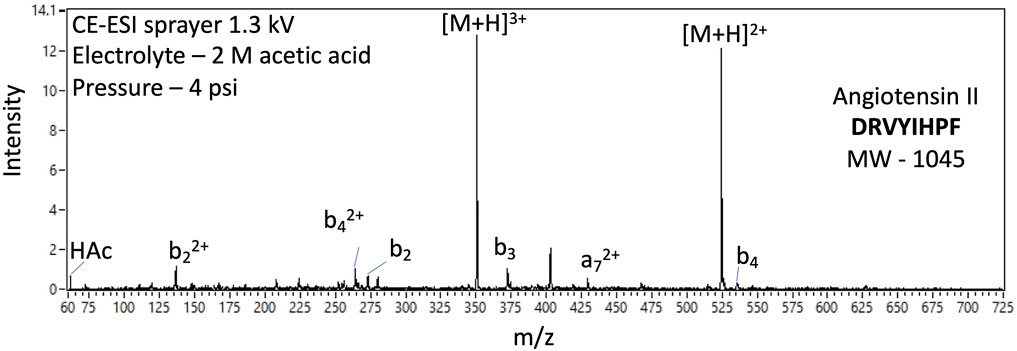
FIGURE 16. Example EMILI ESI-ITMS prototype spectra for 100 nM oligopeptide angiotensin II (m.w. 1045 u) with 2+ and 3+ protonated parent ions as well as several 2+ and 1+ fragment ions corresponding to neutral loss of amino acid monomer units.
EMILI Operational Concept
The overall EMILI instrument design offers a wide range of operational capabilities to meet the organic composition analysis objectives of the Europa Lander quite robustly, even with the uncertainty of what may ultimately be found in the surface to be investigated. Samples may vary in their overall composition, from nearly-pure water ice to a host of potential non-ice phases (e.g. MgSO4 salts), and organics therein may exhibit a range of distributions, characteristics, and levels of radiolytic degradation. These challenges are faced confidently by the breadth, sensitivity, and flexibility of the EMILI toolset. Nonetheless, the intrinsic uncertainty involved in the robotic application of such a broad suite of chemical analyses in an utterly unfamiliar environment necessitates rigorous testing of hardware, software, and data analysis methods under all modes and interfaces and at increasing fidelity to actual mission conditions.
The baseline mission approach for EMILI includes a full analytical survey, through both OCEANS and GAPS, for each collected sample, followed by subsequent activities, such as focused follow-up analyses, depending on results and mission status. A conceptual diagram of EMILI sample analytical flow is depicted in Figure 17. Collected solid samples are delivered to EMILI via CADMES. Nominally this delivery would be carried out with minimal delay to avoid sample loss or alteration (e.g. through vacuum sublimation). However, it may be scientifically valuable to consider accepting this risk and reserving some of the collected sample for the scientific benefit of performing replicate or optimized follow-up analyses. For simplicity, such options are not represented in the links of Figure 17, but may be considered as part of the iterative analytical cycles shown.
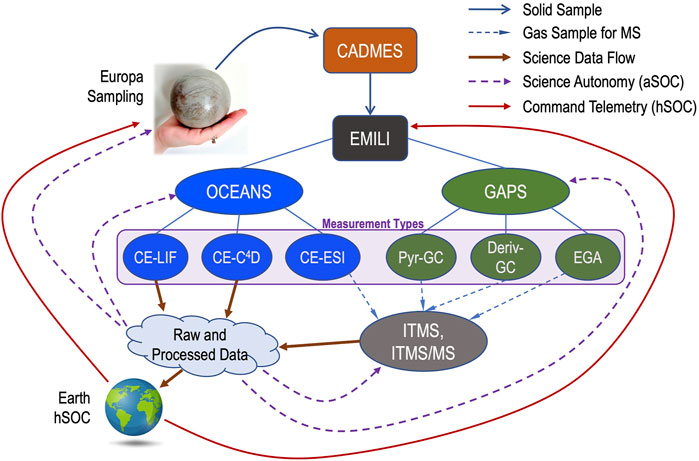
FIGURE 17. EMILI analytical flow diagram shows the interconnections of EMILI subsystem modes with produced data and feedback to follow-up analysis. EMILI combines analyses from the several OCEANS and GAPS protocols (six Measurement Types indicated), either sequentially or as a combined set. In addition to contributing to data returned to Earth and the normal science operations cycle involving humans (hSOC), EMILI may also perform subsequent actions and analyses autonomously (aSOC), enabling real-time data and science optimization as well as more effective overall decision-making for the mission.
The six basic measurement types of EMILI are shown from left to right in Figure 17. To recap, OCEANS provides: CE-LIF for sensitive detection and quantification of amino acids and their chirality; CE-C4D, primarily for inorganic/salt chemistry; and CE coupled through the ESI interface to the ITMS (CE-MS) including tandem MS capability for structural analysis (CE-MS/MS). GAPS provides: direct-pyrolysis GC-MS for chromatographic analysis of volatiles as a survey baseline and to compare with other GAPS-based data; derivatization GC-MS with DMF-DMA and/or TMAH reagents for broad organic and chiral analysis; and evolved gas analysis MS (EGA-MS) for light volatiles. EGA-MS data are collected during all GAPS operations as it automatically samples a small fraction of gas, when so configured, during its transport from oven to GC. All GAPS analyses as well as the CE-MS measurements produce mass spectra as indicated by their gas/sample interfaces to the ITMS and subsequent data output. The CE-LIF and CE-C4D analyses produce their own data in parallel to the ITMS.
As with any robotic planetary science mission, instrument data sets are the ultimate Europa Lander science products upon which mission and payload requirements are based. Raw EMILI data, representing the first detailed bulk analysis of potentially ocean-borne organics on Europa, would, in the absence of engineering constraints, unquestionably be returned to Earth in their entirety for careful processing and decision-making steps as part of a standard science operations cycle with humans in the loop (hSOC). However, exclusive reliance on such an approach for the extremely constrained Europa Lander mission, with limited time, data bandwidth, and energy, is impractical; some onboard processing of raw data will be required. In addition to standard lossless compression of EMILI data by ∼10× (based on prior mission experience), further compressed and/or down-selected data, with some fidelity loss, will likely be needed to provide a snapshot of some or all spectra as a priority telemetry product.
Such optimization of the hSOC cycle, well known from Mars and other missions, is insufficient on its own for EMILI, given that “real-time” (onboard) decisions may be required to maximize Lander science return. These could include both the mentioned down-selection (prioritization) of collected data, as well as choosing when and where to take a subsequent sample, selection of instrument protocols, and in the case of EMILI, even rapid, data-dependent adjustment of sensor settings or ranges during the analysis of a given sample. In other words, EMILI results would strongly benefit from the inclusion of science autonomy (Theiling et al., 2021) at multiple levels of operation. These are represented as the autonomous SOC (aSOC) feedback arrows in Figure 17, which notionally indicate processed EMILI data informing decisions at the Lander sampling and CADMES level, as well as sequence and/or parameter selection for the ongoing operation of EMILI subsystems. A brief general example of the latter is consideration of automatic selection of peaks in mass spectra for isolation and MS/MS, which could have decision turnaround times of well under one second (within an elution peak) or up to 10s of minutes (between instrument modes).
Assessment and testing of science autonomy requirements and opportunities are fundamental to EMILI development and will be increasingly applied to flight software and protocol design as the project moves forward toward potential mission opportunities.
Discussion and Path Forward
The EMILI investigation addresses the central molecular analysis objectives of the Europa Lander mission concept, from both perspectives of individual measurement specifications (performance requirements) and of science deliverables (derived from complementary experimental protocols within an iterative operations concept). The power of the combined analyses of OCEANS and GAPS with the ITMS is the thorough inventory and cross-correlated relative abundances they provide for molecular/organic species in a bulk sample extracted from the Europan surface–planetary materials that may contain evidence of an oceanic biosphere.
As reviewed in Hand et al. (2017), potential molecular biosignatures may appear as distinct abundance distributions and structural biases of key species such as amino acids, fatty acids, and even nucleotides or other building blocks of biomolecules analogous to those known from terrestrial biology. Hetero-oligomers or other complex (and information-rich) structures, that may more directly represent the molecular machinery of alien life, are also potential targets within relatively “fresh” materials (young or well-protected from degradation). As described, these are measured to the appropriate limits of detection by the CE- and GC-based separation and detection modes in EMILI, with overlap of molecular coverage in key areas (Figure 2).
The investigation and the mission must be prepared for, and must expect, the “unknown”: both PMBs that are entirely unfamiliar except for their general properties or statistics consistent with biology, as well as other interesting compounds or patterns that, out of context, could be misinterpreted as biogenic. In the former category we may encounter, for example, a series of organics well out of the abundance distribution expected abiotically, due to clear bias to particular isomers or a subset of molecular weights (Summons et al., 2008). These could possibly be combined with anomalously-high overall concentrations. Among such compounds, we may find some that could only be synthesized through a large number of steps, and are therefore intrinsically complex suggesting biochemical processing (Marshall et al., 2017; Marshall et al., 2021). In the latter “false positive” category we may detect numerous molecular species that are individually consistent with biology, but taken collectively are not best-explained as the expression of a Europan biosphere. We may even speculate, conservatively, that radiolysis of organics, derived from abiotic sources within or outside of Europa, could produce abundance patterns (e.g., molecular weight or bonding biases) that partially mimic biochemical structure. For both of these types of reasons, it is important to analyze Europan organic chemistry in its full context, with as broad an understanding of the full inventory of molecules and their host environment as possible; and further, approach data interpretation from an “agnostic” standpoint (Johnson et al., 2018), commensurate with our lack of knowledge of Europa.
In planning to obtain and interpret results from EMILI, it is critical to acknowledge that even if we have a robust set of PMBs to seek, and an appreciation of potential false positives to avoid, we may in the absence of any compelling signal be unable to distinguish true from false negatives with a single investigation, or even with a single mission. Within the scope of EMILI, a tractable scenario relevant to such ambiguity is that of a mixed sample. Ocean-borne pre-biotic or biogenic organics, in a pristine or modified state, may comprise a fraction of the total organic content of a sample, which could derive from a number of possible endogenous or exogenous abiotic processes and sources. Analytically biogenic organic signals could be unidentifiable in a bulk assay, on first examination. For example, a sample may contain a variety of organics with aliphatic chains of different lengths, such that any strong bias in carbon number associated with just a few compounds could appear only as a subtle modulation of the overall abundances, or could be entirely buried by noise. Treatment of such mixed-sample scenarios requires careful development of both hardware capability and experimental approach. The EMILI instrumentation through its dual-source, dual-separation protocols and sensitive detection modes is designed to have both the required effective dynamic range (e.g., separation via CE and GC with MS) and focusing power (e.g., separation via evolution temperature or MS/MS) to isolate specific organic compounds from different sources. Such capability must be engaged with the most effective experimental approach, particularly on a resource-constrained mission. This approach is only learned through systematic testing, with a suite of analog sample types and compositions, under increasingly flight-realistic conditions–the focus of EMILI development work going forward.
At present each analytical subsystem has been developed to the flight-like prototype stage and has been tested primarily with laboratory analog instrumentation for interfaces to other subsystems. Systematic testing at this level has begun with shared common standard and analog samples–both synthetic and natural mixtures. The overall system architecture and interfaces to potential Lander elements, such as CADMES, have also been designed and developed to some degree. In the near term, the integrated subsystems will be brought together for “end-to-end” testing, specifically OCEANS + ITMS and GAPS + ITMS, including support for Europa-like thermal and vacuum conditions of sample delivery to interface with the LEM and Pyrolysis Oven, respectively. This testing will enable the full combined analysis of common analog samples and development of key science protocols discussed above. This stage additionally represents a “gate” for integration of the EMILI system including more flight-like electronics and facilities for validating the approach to flight qualification: mission electrical, thermal, mechanical, vacuum, radiation, planetary protection, and contamination control requirements must be met with a single minimal mass and power design.
As resources permit, expanded capabilities that may be within the design and operational flexibility of EMILI will be explored. These would certainly include a quantitative investigation of isotope systematics among selected elements, particularly if these can be compared between distinct sources within the sampling reach of the lander. In addition to carbon (via δ13C), the fractionation of sulfur (via δ34S) would be of high interest. Sulfur will likely be found in Europan samples in multiple forms, such as hydrated sulfate salts (e.g., McCord et al., 1999), sulfuric acid, and possibly organosulfur compounds. In analogy with the early Earth (e.g., Kaplan 1975), it is conceivable that isotopically light S could reflect a potential Europan biosignature derived from sulfate-reducing organisms. Other examples may include direct detection of trapped gases in ice phases, that could be released for evolved gas analysis prior to any heating for GCMS, and the characterization of enantiomeric or diastereomeric excess in compounds beyond the amino acids.
When taken together the EMILI requirements are challenging. The performance necessary to analyze Europa analog samples; the assurance of full instrument functionality through development, launch, cruise, Jupiter arrival, and Europa surface science phases; and support of necessary science operations, including autonomy, within the energy and time available, all represent science-driven development objectives that require continuous attention as flight hardware options are realized. However, EMILI is fundamentally based on established analytical technique and flight-compatible engineering; no totally new technologies are required. While the timeframe for a Europa Lander mission is presently uncertain, EMILI is on track to be ready for consideration as a valuable central element of its payload.
Data Availability Statement
The raw data supporting the conclusions of this article will be made available by the authors, without undue reservation.
Author Contributions
WB produced the draft manuscript, integrated sections, and performed overall editing. Selected co-authors including AN, AR, CS, DK, JE, CF, RD, MM, JC, and PW provided substantial written contributions and/or figures. All co-authors contributed significantly to the manuscript through generation of data, consulting on content, and editing of text.
Funding
Support for development of the EMILI instrument has been provided by the NASA Instrument Concepts for Europa Exploration 2 (ICEE-2) program, Solicitation NNH18ZDA001N-ICEE2, Program Executive Dr. Mitch Schulte, and by internal research and development seed funding available at GSFC, JPL, and ARC. Support of the development of the EMILI GC in France has been provided by the Centre Nationale d’Etudes Spatiales (CNES).
Conflict of Interest
Author DK was employed by the company KapScience, LLP. Author RD was employed by the company Danell Consulting, Inc. Authors CC, JS, and KZ were employed by the Honeybee Robotics Spacecraft Mechanisms Corp.
The remaining authors declare that the research was conducted in the absence of any commercial or financial relationships that could be construed as a potential conflict of interest.
Publisher’s Note
All claims expressed in this article are solely those of the authors and do not necessarily represent those of their affiliated organizations, or those of the publisher, the editors and the reviewers. Any product that may be evaluated in this article, or claim that may be made by its manufacturer, is not guaranteed or endorsed by the publisher.
Acknowledgments
The authors wish to acknowledge the significant support of their respective institutions that have provided the work facility, environment, and larger group of collaborations necessary to carry out this work. This support has been particularly appreciated during the many challenging months of the ongoing COVID-19 global pandemic. Co-authors at NASA/GSFC wish to acknowledge the support of key technical and engineering personnel who have enabled the EMILI concept to mature into a flight-viable concept, including R. Wilkinson, M. Noreiga, D. Carrigan, M. Bendt, F. Noreiga, C. Gundersen, C. Budinoff, and S. Meyer. WBB wishes to acknowledge the fruitful discussions and collaboration as part of the Center for Life Detection, a Research Coordination Network team led by Tori Hoehler, NASA Ames; particularly for the inspiration of the search for extraterrestrial life being a scientific effort of the full community.
Supplementary Material
The Supplementary Material for this article can be found online at: https://www.frontiersin.org/articles/10.3389/frspt.2021.760927/full#supplementary-material
Footnotes
*LOD = limit of detection
References
Amashukeli, X., Pelletier, C. C., Kirby, J. P., and Grunthaner, F. J. (2007). Subcritical Water Extraction of Amino Acids from Atacama Desert Soils. J. Geophys. Res. 112, 1–10. doi:10.1029/2006JG000308
Barnes, J. W., Turtle, E. P., Trainer, M. G., Lorenz, R. D., MacKenzie, S. M., Brinckerhoff, W. B., et al. (2021). Science Goals and Objectives for the Dragonfly Titan Rotorcraft Relocatable Lander. Planet. Sci. J. 2, 130. doi:10.3847/PSJ/abfdcf
Chin, M., Ricco, A., Quinn, R., Lee, A., Boone, T., Chinn, T., et al. (2018). SPLIce: A Microfluidic Sample Processor to Enable the Search for Life on Icy Worlds. July 14–20, 2018. Pasadena, CA: 42nd COSPAR Scientific Assembly. Available at: http://cospar2018.org/wp-content/uploads/2018/07/COSPAR-2018-Abstract-Book_July21-2018-UPDATE.pdf.
Chinn, T. N., Lee, A., Boone, T., Tan, M. X., Chin, M., McCutcheon, G., et al. (2017). “Sample Processor for Life on Icy Worlds (SPLIce): Design and Test Results,” in Proceedings µTAS 2017 (21st International Conference on Miniaturized Systems for Chemistry and Life Sciences), Savannah, GA, October 22-26, 2017 (San Diego, CA: Chemical and Biological Microsystems Society), 1479–1480.
Chyba, C. F., and Phillips, C. B. (2002). Europa as an Abode of Life. Origins Life Evol. Biosph. 32, 47–67. doi:10.1023/A:1013958519734
Creamer, J. S., Mora, M. F., Noell, A. C., and Willis, P. A. (2019). Long‐term Thermal Stability of Fluorescent Dye Used for Chiral Amino Acid Analysis on Future Spaceflight Missions. Electrophoresis 40, 3117–3122. doi:10.1002/elps.201900268
Creamer, J. S., Mora, M. F., and Willis, P. A. (2017). Enhanced Resolution of Chiral Amino Acids with Capillary Electrophoresis for Biosignature Detection in Extraterrestrial Samples. Anal. Chem. 89, 1329–1337. doi:10.1021/acs.analchem.6b04338
Creamer, J. S., Mora, M. F., and Willis, P. A. (2018). Stability of Reagents Used for Chiral Amino Acid Analysis During Spaceflight Missions in High‐radiation Environments. Electrophoresis 39, 2864–2871. doi:10.1002/elps.201800274
Ehrenfreund, P., Ricco, A. J., Squires, D., Kitts, C., Agasid, E., Bramall, N., et al. (2014). The O/OREOS mission-Astrobiology in Low Earth Orbit. Acta Astronautica 93, 501–508. doi:10.1016/j.actaastro.2012.09.009
Ferreira Santos, M. S., Metz, B. C., da Costa Et, do Lago, C. L., Willis, P. A., Mora, M. F., and Noell, A. C. (2021). Towards a Radiation Tolerant Contactless Conductivity Detector for Spaceflight Applications. Acta Astronautica. Life Sci. Space Res. 3, 63–75.
Ferreira Santos, M. S., Cordeiro, T. G., Noell, A. C., Garcia, C. D., and Mora, M. F. (2018). Analysis of Inorganic Cations and Amino Acids in High Salinity Samples by Capillary Electrophoresis and Conductivity Detection: Implications for In‐situ Exploration of Ocean Worlds. Electrophoresis 39, 2890–2897. doi:10.1002/elps.201800266
Fleming, M. S., Bebout, B. M., Tan, M. X., Selch, F., and Ricco, A. J. (2014). Biological system development for GraviSat: A new platform for studying photosynthesis and microalgae in space. Life Sci. Space Res. 3, 63–75. doi:10.1016/j.lssr.2014.09.004
Fracassi da Silva, J. A., and do Lago, C. L. (1998). An Oscillometric Detector for Capillary Electrophoresis. Anal. Chem. 70, 4339–4343. doi:10.1021/ac980185g
Freissinet, C., Millan, M., Glavin, D. P., Li, X., Grubisic, A., Eigenbrode, J. E., et al. (2019). Investigating the Effects of Gamma Radiation on Selected Chemicals for Use in Biosignature Detection Instruments on the Surface of Jupiter's Moon Europa. Planet. Space Sci. 175, 1–12. doi:10.1016/j.pss.2019.05.009
Glavin, D. P., Freissinet, C., Millan, K. E., Eigenbrode, J. L., Brunner, A., Buch, A., et al. (2013). Evidence for perchlorates and the origin of chlorinated hydrocarbons detected by SAM at the Rocknest aeolian deposit in Gale Crater. J. Geophys. Res. Planets 118, 1955–1973. doi:10.1002/jgre.20144
Goesmann, F., Brinckerhoff, W. B., Raulin, F., Goetz, W., Danell, R. M., Getty, S. A., et al. (2017). The Mars Organic Molecule Analyzer (MOMA) Instrument: Characterization of Organic Material in Martian Sediments. Astrobiology 17, 655–685. doi:10.1089/ast.2016.1551
Hand, K. P., Murray, A. E., Garvin, J. N., Brinckerhoff, W. B., Christner, B. C., Edgett, K. S., et al. (2017). Report of the Europa Lander Science Definition Team. Available at: https://europa.nasa.gov/resources/58/europa-landerstudy-2016-report/.
Howell, S. M., and Pappalardo, R. T. (2020). NASA's Europa Clipper-A Mission to a Potentially Habitable Ocean World. Nat. Commun. 11, 1311. doi:10.1038/s41467-020-15160-9
Jackson, M., Bayer, T., Sheldon, C., Buffington, B., Harris, I., Jones-Wilson, L., et al. (2020). “Europa Clipper Mission: Onward to Implementation!,” in 2020 IEEE Aerospace Conference, Big Sky, MT, March 7-14, 2020, 1–20. doi:10.1109/AERO47225.2020.9172447
Jaramillo, E. A., Ferreira Santos, M. S., Noell, A. C., and Mora, M. F. (2021). Capillary Electrophoresis Method for Analysis of Inorganic and Organic Anions Related to Habitability and the Search for Life. Electrophoresis 42, 1956–1964. doi:10.1002/elps.202100134
Johnson, S. S., Graham, H., Anslyn, E., Conrad, P., Cronin, L., Ellington, A., et al. (2018). Agnostic Biosignatures: Towards a More Inclusive Life Detection Strategy. White paper submitted to the. Committee on an Astrobiology Science Strategy for the Search for Life in the Universe. National Academies Press.
Kaplan, I. R. (1975). Stable Isotopes as a Guide to Biogeochemical Processes. Proc. R. Soc. Lond. B. 189, 183–211. doi:10.1098/rspb.1975.0052
Kehl, F., Kovarik, N. A., Creamer, J. S., Costa, E. T., and Willis, P. A. (2019). A Subcritical Water Extractor Prototype for Potential Astrobiology Spaceflight Missions. Earth Space Sci. 6, 2443–2460. doi:10.1029/2019ea000803
Leshin, L. A., Mahaffy, P. R., Webster, C. R., Cabane, M., Coll, P., Conrad, P., et al. (2013). Volatile, Isotope, and Organic Analysis of Martian Fines with the Mars Curiosity Rover. Science 341, 1238937. doi:10.1126/SCIENCE.1238937
Mahaffy, P. R., Webster, C. R., Cabanem, M., Conrad, P. G., Coll, P., Atreya, S. K., et al. (2012). The Sample Analysis at Mars Investigation and Instrument Suite. Space Sci. Rev. 170, 401–478. doi:10.1007/s11214-012-9879-z
Malespin, C. A., Chu, P., Pinnick, V., Grossman, A., Barfknecht, P., Francom, M., et al. (2020). “The Collaborative Acceptance and Distribution for Measuring Europan Samples (CADMES) Sample Delivery System for a Future Europa Lander,” in 51st Lunar and Planetary Science Conference, The Woodlands, TX, March 16–20, 2020.
McAdam, A. C., Sutter, B., Archer, P. D., Franz, H. B., Wong, G. M., Lewis, J. M. T., et al. (2020). Constraints on the Mineralogy and Geochemistry of Vera Rubin Ridge, Gale Crater, Mars, from Mars Science Laboratory Sample Analysis at Mars Evolved Gas Analyses. J. Geophys. Res. Planets 125, 1–26. doi:10.1029/2019JE006309
McCord, T. B., Hansen, G. B., Matson, D. L., Johnson, T. V., Crowley, J. K., Fanale, F. P., et al. (1999). Hydrated Salt Minerals on Europa's Surface from the Galileo Near-Infrared Mapping Spectrometer (NIMS) Investigation. J. Geophys. Res. 104, 11827–11851. doi:10.1029/1999JE900005
McCutcheon, G., Ricco, A., Hu, S., White, B., Hoang, D., Hyde, E., et al. (2016). “PowerCell Payload on Eu:CROPIS—Measuring Synthetic Biology in Space,” in Proceeding of the 30th Annual AIAA/USU Conference on Small Satellites, Logan, UT, August 6-11, 2016 (Reston, VA: The American Institute of Aeronautics and Astronautics).
Ming, D. W., Archer, P. D., Glavin, D. P., Eigenbrode, J. L., Franz, H. B., Sutter, B., et al. (2014). Volatile and organic compositions of sedimentary rocks in Yellowknife Bay, Gale crater, Mars. Science 343, 1245267. doi:10.1126/SCIENCE.1245267
Mora, M. F., Kehl, F., Tavares da Costa, E., Bramall, N., and Willis, P. A. (2020). Fully Automated Microchip Electrophoresis Analyzer for Potential Life Detection Missions. Anal. Chem. 92, 12959–12966. doi:10.1021/acs.analchem.0c01628
Mora, M. F., Kok, M. G. M., Noell, A. C., and Willis, P. A. (2021). Detection of Biosignatures by Capillary Electrophoresis and Mass Spectrometry in the Presence of Salts Relevant to Missions to Ocean Worlds. Astrobiology. Submitted.
National Academies of Sciences, Engineering, and Medicine (NASEM) (2019). An Astrobiology Strategy for the Search for Life in the Universe. Washington, DC: The National Academies Press. doi:10.17226/25252
National Academies of Sciences, Engineering, and Medicine (NASEM) (2017). Searching for Life Across Space and Time: Proceedings of a Workshop. Washington, DC: The National Academies Press. doi:10.17226/24860
National Research Council (1999). A Science Strategy for the Exploration of Europa. Washington, DC: National Academy Press.
Neveu, M., Hays, L. E., Voytek, M. A., New, M. H., and Schulte, M. D. (2018). The Ladder of Life Detection. Astrobiology 18, 1375–1402. doi:10.1089/ast.2017.1773
Nicholson, W. L., Ricco, A. J., Agasid, E., Beasley, C., Diaz-Aguado, M., Ehrenfreund, P., et al. (2011). The O/OREOS Mission: First Science Data from the Space Environment Survivability of Living Organisms (SESLO) Payload. Astrobiology 11, 951–958. doi:10.1089/ast.2011.0714
Noell, A. C., Fisher, A. M., Fors‐Francis, K., and Sherrit, S. (2018). Subcritical Water Extraction of Amino Acids from Mars Analog Soils. Electrophoresis 39, 2854–2863. doi:10.1002/elps.201700459
Oborny, N. J., Kehl, F., Cretu, V., Noell, A. C., and Willis, P. A. (2021). A Radiation Tolerant Laser-Induced Fluorescence Detection System for a Potential Europa Lander mission. Acta Astronautica 186, 465–472. doi:10.1016/j.actaastro.2021.06.012
Padgen, M. R., Liddell, L. C., Bhardwaj, S. R., Gentry, D., Marina, D., Parra, M., et al. (2021). BioSentinel: A Biofluidic Nanosatellite Monitoring Microbial Growth and Activity in Deep Space. Astrobiology 21, 1–11. doi:10.1089/ast.2020.2305
Padgen, M. R., Lera, M. P., Parra, M. P., Ricco, A. J., Chin, M., Chinn, T. N., et al. (2020). EcAMSat Spaceflight Measurements of the Role of σs in Antibiotic Resistance of Stationary Phase Escherichia coli in Microgravity. Life Sci. Space Res. 24, 18–24. doi:10.1016/j.lssr.2019.10.007
Park, J., Salmi, M. L., Wan Salim, W. W. A., Rademacher, A., Wickizer, B., Schooley, A., et al. (2017). An Autonomous Lab on a Chip for Space Flight Calibration of Gravity-Induced Transcellular Calcium Polarization in Single-Cell Fern Spores. Lab. Chip 17, 1095–1103. doi:10.1039/c6lc01370h
Pohorille, A., and Sokolowska, J. (2020). Evaluating Biosignatures for Life Detection. Astrobiology 20, 1236–1250. doi:10.1089/ast.2019.2151
Radosevich, L. A., Ricco, A. J., Quinn, R. C., Boone, T., Chin, M., Bywaters, K. F., et al. (2019). “SPLIce: A Microfluidic Sample Processor to Enable the Search for Life on Icy Worlds,” in Astrobiology Science Conference (AbSciCon) 2019, Seattle, WA, June 24–28, 2019.
Ricco, A. J., Parra, M., Niesel, D., Piccini, M., Ly, D., McGinnis, M., et al. (2011). “PharmaSat: Drug Dose Response in Microgravity from A Free-Flying Integrated Biofluidic/Optical Culture-And-Analysis Satellite,” in Proceeding of the SPIE 7929: Microfluidics, BioMEMS, and Medical Microsystems IX, 79290T, San Francisco, CA, January 22-27, 2011 (Bellingham, WA: SPIE), 9. doi:10.1117/12.881082
Ricco, A. J., Parra, M., Hines, J. W., Piccini, M., Barker, V., Storment, C., et al. (2007). “Autonomous Genetic Analysis System to Study Space Effects on Microorganisms: Results from Orbit,” in Proceeding of the 14th Int’l. Conf. on Solid-State Sensors, Actuators, & Microsystems (Transducers ‘07/Eurosensors XXI), Lyon, France, June 10-14, 2007 (New York, NY: IEEE), 33–37. doi:10.1109/sensor.2007.4300065
Ricco, A. J., Maria, S. R. S., Hanel, R. P., and Bhattacharya, S. (2020). BioSentinel: A 6U Nanosatellite for Deep-Space Biological Science. IEEE Aerosp. Electron. Syst. Mag. 35, 6–18. doi:10.1109/maes.2019.2953760
Roth, L., Saur, J., Retherford, K. D., Strobel, D. F., Feldman, P. D., McGrath, M. A., et al. (2014). Transient Water Vapor at Europa's South Pole. Science 343, 171–174. doi:10.1126/science.1247051
Pappalardo, R. T., McKinnon, W. B., and Kurana, K. (2009). Europa. 2nd Edn (Tucson, AZ: University of Arizona Press).
Schrödinger, E. (1944). What Is Life? the Physical Aspect of the Living Cell. Cambridge, UK: Cambridge University Press.
Schultz, C. L., and Moini, M. (2003). Analysis of Underivatized Amino Acids and Their D/l-Enantiomers by Sheathless Capillary Electrophoresis/Electrospray Ionization-Mass Spectrometry. Anal. Chem. 75, 1508–1513. doi:10.1021/ac0263925
Sparks, W. B., Hand, K. P., McGrath, M. A., Bergeron, E., Cracraft, M., and Deustua, S. E. (2016). Probing for Evidence of Plumes on Europa with HST/STIS. ApJ 829, 121. doi:10.3847/0004-637X/829/2/121
Tan, M. X., Piccini, M., and Ricco, A. (2011). “Integrated Fluidic System for Growth and Fluorescence Imaging of Multicellular Organisms in Nanosatellite Applications,” in Proceeding µTAS 2011 (15th International Conference on Miniaturized Systems for Chemistry and Life Sciences), Seattle, WA, October 2-6, 2011 (San Diego, CA: Chemical and Biological Microsystems Society), 1719–1721.
Theiling, B., Brinckerhoff, W. B., Castillo-Rogez, J., Chou, L., Da Poian, V., Graham, H., et al. (2021). Non-Robotic Science Autonomy Development. Bull. AAS 53. doi:10.3847/25c2cfeb.ee4e6b64
Willis, P., Brinckerhoff, W. B., Ricco, A., Creamer, J., Mora, F., Noell, A., et al. (2018). A Universal Approach in the Search for Life at the Molecular Level. Pasadena, CA: 42nd COSPAR Scientific Assembly.
Willis, P. A., Creamer, J. S., and Mora, M. F. (2015). Implementation of Microchip Electrophoresis Instrumentation for Future Spaceflight Missions. Anal. Bioanal. Chem. 407, 6939–6963. doi:10.1007/s00216-015-8903-z
Keywords: Europa, mass spectrometry, capillary electrophoresis, gas chromatography, life detection, Europa Lander, molecular biosignature
Citation: Brinckerhoff WB, Willis PA, Ricco AJ, Kaplan DA, Danell RM, Grubisic A, Mora MF, Creamer JS, Noell A, Stern J, Szopa C, Freissinet C, Kehl F, Zamuruyev K, Castle C, Spring J, Drevinskas T, Badescu M, Ferreira Santos MS, Jaramillo EA, van Amerom F, Li X, Castillo M, Eigenbrode J, Theiling B, Quinn RC, Stalport F, Buch A and Zacny K (2022) Europan Molecular Indicators of Life Investigation (EMILI) for a Future Europa Lander Mission. Front. Space Technol. 2:760927. doi: 10.3389/frspt.2021.760927
Received: 19 August 2021; Accepted: 18 November 2021;
Published: 13 January 2022.
Edited by:
Andreas Riedo, University of Bern, SwitzerlandReviewed by:
Julian Chela-Flores, The Abdus Salam International Centre for Theoretical Physics (ICTP), ItalyPo Bian, Hefei Institutes of Physical Science (CAS), China
Copyright © 2022 Brinckerhoff, Willis, Ricco, Kaplan, Danell, Grubisic, Mora, Creamer, Noell, Stern, Szopa, Freissinet, Kehl, Zamuruyev, Castle, Spring, Drevinskas, Badescu, Ferreira Santos, Jaramillo, van Amerom, Li, Castillo, Eigenbrode, Theiling, Quinn, Stalport, Buch and Zacny. This is an open-access article distributed under the terms of the Creative Commons Attribution License (CC BY). The use, distribution or reproduction in other forums is permitted, provided the original author(s) and the copyright owner(s) are credited and that the original publication in this journal is cited, in accordance with accepted academic practice. No use, distribution or reproduction is permitted which does not comply with these terms.
*Correspondence: William B. Brinckerhoff, d2lsbGlhbS5iLmJyaW5ja2VyaG9mZkBuYXNhLmdvdg==