- 1Research Group for Marine Geochemistry (ICBM-MPI Bridging Group), School of Mathematics and Science, Institute for Chemistry and Biology of the Marine Environment (ICBM), Carl von Ossietzky Universität Oldenburg, Oldenburg, Germany
- 2Marine Isotope Geochemistry Group, Institute for Chemistry and Biology of the Marine Environment (ICBM), Carl von Ossietzky Universität Oldenburg, Oldenburg, Germany
- 3Hydrogeology and Landscape Hydrology Group, Institute of Biology and Environmental Science (IBU), Carl von Ossietzky Universität Oldenburg, Oldenburg, Germany
- 4Benthic Microbiology Group, Institute for Chemistry and Biology of the Marine Environment (ICBM), Carl von Ossietzky Universität Oldenburg, Oldenburg, Germany
Permeable sandy sediments beneath high-energy beaches act as potent biogeochemical reactors with high organic matter turnover rates. Despite their significant role in nutrient and Fe cycling in coastal environments, their capacity to trap and mobilize dissolved organic matter (DOM) remains poorly understood. This study analyzed the molecular composition of water- and acid-leachable sedimentary organic matter down to 24 meters depth in an exemplary subterranean estuary (STE) site of a high-energy beach on Spiekeroog Island, German North Sea. We also investigated DOM coprecipitation with Fe3+ (oxy)hydroxides in the aqueous phase in STE porewaters containing reduced Fe2+, exposed to atmospheric oxygen. We employed fluorescence spectroscopy and ultrahigh-resolution mass spectrometry for our molecular analysis. Seawater and STE porewater DOM were more complex and oxidized than DOM leached from the sediments. Sediment leaching with ultrapure water predominantly yielded aliphatic, oxygen-rich, and presumably labile compounds of marine and possibly autochthonous origin. In contrast, DOM in subsequent 0.5 M hydrochloric acid (HCl) leachates was more molecularly diverse, enriched with oxidized aromatic compounds likely terrigenous and refractory in nature. Notably, acid leachates also contained a substantial fraction of labile compounds. Approximately 10% of total organic carbon (TOC) was leachable as dissolved organic carbon (DOC) in both water and acid treatments. Leachable DOC concentrations were up to two orders of magnitude higher than porewater DOC, while TOC was up to three orders of magnitude higher. These findings indicate that deep sediments are important organic matter reservoirs. Elevated leachable DOC and Fe concentrations in acid treatments were associated with finer, likely clay-rich sediments, while lower levels coincided with coarser sediments. This suggests that Fe oxides/hydroxides act as adsorption sites for terrigenous DOM within the mineral matrix of STE sediments. While Fe-DOM coprecipitation of aerated porewater samples induced distinct molecular alterations, DOC removal was within analytical uncertainties, and molecular fractionation varied across sampling campaigns. Thus, Fe-DOM coprecipitation in the aqueous phases at redox interfaces in the deep (>5 m) STEs appears limited and influenced by transport-driven geochemical changes. We propose that Fe-rich minerals and clay fractions in deep STE sediments facilitate the preservation of refractory, terrigenous DOM, whereas loosely bound, labile DOM fractions—susceptible to rapid microbial respiration—may be readily mobilized by advective water flow in high-energy STEs.
1 Introduction
Dissolved organic matter (DOM) plays a pivotal role in the biogeochemical cycling of carbon, nutrients, and trace metals such as iron (Fe) between terrestrial and marine ecosystems (Dittmar and Stubbins, 2014). Continents contribute terrigenous DOM and associated Fe to the oceans via riverine discharge, atmospheric deposition, and submarine groundwater discharge (SGD; Moore, 1999; Seidel et al., 2015; Waska et al., 2021; Adyasari et al., 2023; Carvalho da Silva et al., 2023). SGD, an integral component of the coastal hydrological cycle, facilitates the exchange of water and dissolved constituents across the land-ocean interface (Cho et al., 2018).
Subterranean estuaries (STEs) are coastal aquifers in which meteoric groundwater mixes with recirculating seawater. They function as dynamic biogeochemical reactors that modulate the composition and fluxes of dissolved and particulate matter prior to their discharge into the ocean (Slomp and Van Cappellen, 2004; Anschutz et al., 2009; McAllister et al., 2015). In contrast to surface estuarine systems, STEs are characterized by steep redox and salinity gradients, prolonged porewater residence times, and intimate water-sediment interactions that drive a myriad of biogeochemical transformations, including the cycling of redox-sensitive elements such as iron (Brumsack and Gieskes, 1983; Beck et al., 2017; Reckhardt et al., 2017; O'Connor et al., 2018).
The redox conditions within STEs significantly influence the speciation, mobility, and fate of various groundwater constituents, including ammonium, nitrate, dissolved manganese, iron, and hydrogen sulfide (McAllister et al., 2015; Reckhardt et al., 2015; Beck et al., 2017; Ahrens et al., 2020). Fe, for instance, undergoes redox cycling between its oxidized (Fe3+) and reduced (Fe2+) forms, governed by microbial electron acceptor utilization and supply of oxygen and reduced species like hydrogen sulfide (Reckhardt et al., 2017). DOM exhibits a high affinity for freshly precipitated Fe3+(oxy) hydroxides at oxic-anoxic interfaces in subtidal sands, leading to the formation of organic matter (OM)-Fe3+ associations that can temporarily stabilize OM against microbial remineralization (Zhou et al., 2023). In STEs, these organo-mineral complexes could influence the bioavailability and transport of nutrients and Fe, thereby affecting their cycling and eventual delivery to coastal waters (Linkhorst et al., 2017; Sirois et al., 2018).
The extent of Fe-OM coprecipitation or coagulation is controlled by factors such as the dissolved organic carbon (DOC)/Fe molar ratio, OM composition, Fe speciation, and solution physicochemistry (e.g., pH and ionic strength; Nierop et al., 2002; Riedel et al., 2013; Lv et al., 2016; Linkhorst et al., 2017; Bao et al., 2023). Higher-molecular weight aromatic, oxygen-rich terrigenous DOM preferentially interacts with Fe3+ compared to aliphatic, marine DOM (Riedel et al., 2013; Gomez-Saez et al., 2015; Linkhorst et al., 2017), and amorphous ferrihydrites scavenge more DOM than other iron oxyhydroxides (Lv et al., 2016). Additionally, slightly acidic conditions (pH 4–5) enhance the DOM-Fe3+ coagulation potential (Nierop et al., 2002).
Beyond redox-driven coagulation, DOM from both terrestrial and marine sources can adsorb onto mineral surfaces within STEs, particularly those with high specific surface areas like clays, iron oxides, aluminum silicates, and phyllosilicates (Christensen, 2001; Kögel-Knabner et al., 2008; Groeneveld et al., 2020). The chemical composition of DOM governs its affinity for mineral surfaces. Aromatic, oxygenated, and nitrogen-enriched compounds often adsorb and form stable complexes (Deng and Dixon, 2002; Cyle et al., 2016; Groeneveld et al., 2020; Islam et al., 2022; Ahmad and Martsinovich, 2023). In addition to inner-sphere complexation with Al and Fe functionalities, organic matter is also loosely bound to sediment grain surfaces and other attached organic matter though hydrogen bonds, van der Waals forces, π-π interactions, and electrostatic attraction. Some of the loosely bound OM can be leached off the sediment matrix with ultrapure water (Zhou et al., 2024). This DOM fraction is partially labile and could be readily accessible to sediment-attached microbes (Zhou et al., 2024; Abarike et al., 2024).
Recent studies have shown that freshly precipitated iron oxides retain terrigenous DOM at redox boundaries in STEs (Linkhorst et al., 2017; Sirois et al., 2018), but the spatio-temporal dynamics of these DOM-Fe3+ interactions in high-energy beach environments remain understudied (Charette and Sholkovitz, 2002; Roy et al., 2011). These high-energy systems are characterized by redox fluctuations and high throughputs of seawater (Greskowiak et al., 2023). A recent SGD groundwater flow model for a high-energy beach STE at Spiekeroog Island, North Germany (Figure 1) predicts that the upper saline plume (Figure 1) alone can reach depths of ~20 m below sediment surface (Grünenbaum et al., 2020). Consequently, probing groundwater layers down to 20–30 m depth may be necessary to capture all sources of DOM to the beach STE system and their association with freshly precipitating iron at redox interfaces. Given that sandy beaches constitute ~30% of the world's ice-free coastline (Luijendijk et al., 2020), elucidating the processes governing organo-mineral associations in STEs is essential for evaluating their global significance as biogeochemical reactors and their role in the cycling of organic carbon between terrestrial and marine endmembers.
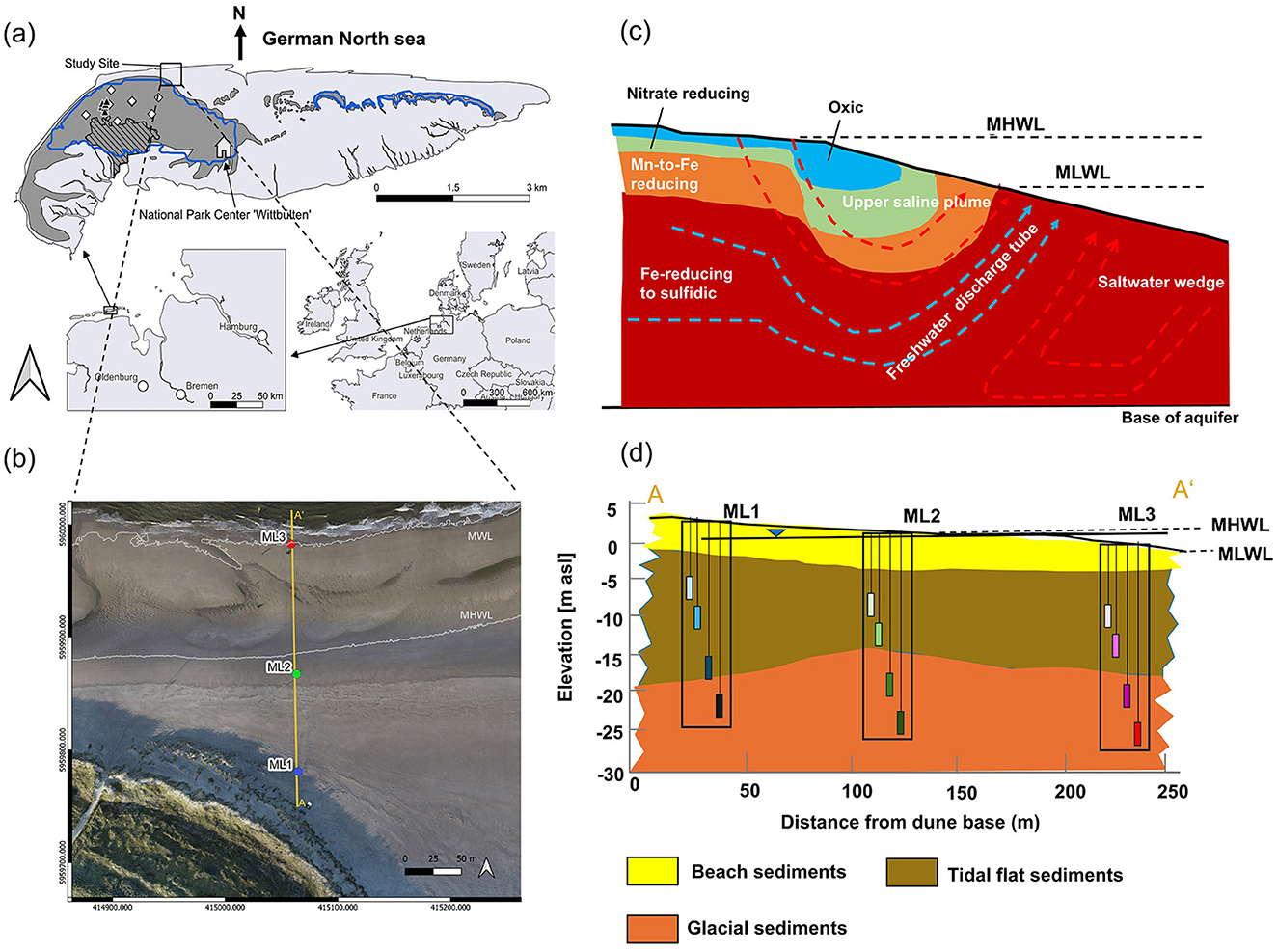
Figure 1. (a) Location of Spiekeroog Island at the North German coastline. The sandy dune areas are shaded in dark gray (based on Röper et al., 2013), while the urban areas are represented by black hatched regions. (b) The DynaDeep study site, situated on the northern beach of Spiekeroog Island, features the locations of multi-level groundwater monitoring wells ML1, ML2, and ML3 (blue, green, and red circles, respectively). The mean high-water line (MHWL), and mean water line (MWL; Massmann et al., 2023) are marked by white lines. (c) A schematic illustration of the principal flow system and redox zoning in the subterranean estuary (STE). Red arrows depict the flow paths of recirculated seawater, while blue arrows represent the flow paths of meteoric fresh groundwater. The colored areas delineate the different redox zones (modified from Greskowiak et al., 2023). (d) A cross-sectional view extending from the dune base to the sea, illustrating the main lithological units, well locations, and filter screen depths. The mean high-water line (MHWL) and mean low-water line (MLWL) are marked, with the solid line between the MHWL and MLWL representing the groundwater level (Massmann et al., 2023).
To further our understanding of DOM dynamics in deep sediments and porewaters of a high-energy beach environment, this study aimed to answer the following key questions:
1) What is the quantity and characteristic of organic matter that is loosely bound, as well as complexed to minerals, in deep sediments of a high-energy beach?
2) What is the potential role of these sediments in retaining terrigenous DOM?
3) What are the characteristics of DOM associated with precipitating Fe at redox interfaces in porewaters of the deep STE under high-energy beach conditions?
4) Are there discernible spatial-temporal trends in Fe-DOM coprecipitation in porewaters of deep STE under high-energy beach conditions?
To address these questions, we examined the composition of OM and Fe in sediment cores and compared them with pore- and seawater samples from a high-energy STE on the northern beach of Spiekeroog Island, facing the North Sea. Experimental Fe-DOM coprecipitation in shallow (up to 2 m) STE porewaters at this site induced DOM molecular fractionation and lead to the suggestion that at redox transition zones, DOM could be trapped in the sedimentary phase for unknown periods (Linkhorst et al., 2017). Recently, an observatory for the deep (6–24 m) STE was constructed on Spiekeroog beach (Massmann et al., 2023). Therefore, this location is ideal for exploring how Fe3+(oxy) hydroxides are involved in the retention of organic matter in the deeper (>5 m) porewaters and sediments of this high-energy beach STE. We isolated DOM from sediments using ultrapure water to extract weakly bound fractions (i.e., OM loosely attached by hydrogen bonds and van der Waals forces) and hydrochloric acid (0.5 M) to extract strongly bound fractions (i.e., OM complexed with iron oxides). Additionally, we investigated the coagulation of iron with DOM from porewater samples collected from permanent beach wells at depths where reduced iron in μM concentrations had been detected previously (Massmann et al., 2023). Changes in beach morphology are common in high-energy beaches and may induce variations in STE groundwater flow and redox zonation (Greskowiak et al., 2023). Therefore, we used porewaters from three different sampling campaigns between September and December 2022 to account for potential temporal dynamics in porewater DOM, redox regime, and Fe composition, and assess their influence on Fe-DOM coagulation patterns. These months were selected to capture temporal transitions, taking a time lag between surface and deep porewater conditions into account: September marks the shift from summer to autumn, characterized by high primary production in the prior months; October represents an intermediary period with declining primary production and temperatures; and December reflects the onset of winter, with low primary production and reduced biological activity (Reckhardt et al., 2024; Auer et al., 2025).
2 Materials and methods
2.1 Study site
The study site is located on Spiekeroog, a barrier island in the southern North Sea, adjacent to the German coast of Lower Saxony (Figure 1a). As one of Germany's least developed East Frisian Islands with a low number of permanent residents (≈800 inhabitants) and negligible agricultural or industrial activity, this pristine environment offers exceptionally favorable conditions for studying natural biogeochemical processes with little anthropogenic interference [CWSS (Common Wadden Sea Secretariat, Wilhelmshaven, Germany) and WHNPG (World Heritage Nomination Project Group), 2008]. Spanning ~10 km east to west and 2 km north to south, the island covers an area of 21.3 km2 (Zielinski et al., 2022). It experiences high-energy, meso-tidal conditions with a mean tidal range of 2.7 m and a mean significant wave height of 1.4 m (Herrling and Winter, 2015). The island's geology comprises medium to fine sands from the Pliocene, Pleistocene, and Holocene epochs, occasionally containing gravel, clay, peat, and shales, with a model-based estimation of the hydraulic conductivity of 11 m d−1 (Beck et al., 2017; Grünenbaum et al., 2020). A clay layer, ranging from 1.5 to 15 m in thickness, lies beneath the main dune area, and constrains the islands' freshwater lens at ~40 m below sea level, although its extent beneath the beach aquifer is unknown (Röper et al., 2012). The average annual precipitation is ~800 mm, with groundwater recharge rates ranging from 300 to 400 mm annually (Röper et al., 2012; Seibert et al., 2018). Groundwater temperatures in the freshwater lens remain around 10°C (Röper et al., 2014; Seibert et al., 2018), while seawater temperatures vary seasonally from 0 to 4°C in winter 16 to 20°C in summer (Reuter et al., 2009). Along the northern shoreline of Spiekeroog, three multilevel (ML) observation wells were installed in 2022 with filter depths at 6, 12, 18, and 24 m below the beach surface (Massmann et al., 2023). The beach stretch measures ~200–300 m, with well ML1 located near the dune base, well ML2 near the high-water line, and well ML3 near the low-water line (Figure 1). Previous studies of the shallow (upper 2 m) STE in the same location revealed distinct redox conditions, ranging from oxic near the dune base to sub- to anoxic toward the low-water line, with additional anoxic freshwater discharge from deeper layers of the freshwater lens into the North Sea (Reckhardt et al., 2017). Numerical models further demonstrated dynamic variations in redox conditions under high-energy beaches like Spiekeroog, extending to depths of 20 m due to constantly changing beach morphodynamics (Greskowiak et al., 2023; Figure 1c). A first survey of the newly installed wells by Massmann et al. (2023) reported distinct redox and DOC distribution patterns. ML1 and ML2 exhibited mostly oxic conditions above 18 m, transitioning to anoxic conditions from this depth. In contrast, ML3, near the low water line, was anoxic at all investigated depths. DOC concentrations increased from the 6 m well depth toward mid-depths (12–18 m) in ML1 and in the deepest part (18 and 24 m) of ML3, while ML2 showed less variability in DOC concentrations across depths (Reckhardt et al., 2024).
2.2 Sampling
2.2.1 Core collection and sediment sampling
Prior to well installation, in May 2022, single 24-meter-long undisturbed STE sediment cores were collected from each of the two sites: ML1, near the dune base, and ML2, close to the mean high-water line (Figure 1b), following DIN EN ISO 22475-1 dry drilling (Massmann et al., 2023). At ML3 (Figure 1b), located within the intertidal zone, 24 mixed sediment samples from 1 m intervals were taken during the drilling of a 24 m deep multilevel well by a drilling ship in August 2022 under favorable weather conditions. After core retrieval, the following procedures were undertaken for ML1 and ML2: One-meter-long core segments, encased in a 10 cm diameter PVC liner, were transported to the National Park Center Wittbülten on Spiekeroog (Figure 1a) using a handcart. On the same day, the 1-m core samples were divided into 0.5-m sections, and sediments from depths of 5.5, 11.5, 17.5, and 23.5 m (Figure 1d) were extracted from the undisturbed central part of the core using sterile cut-off syringes. Thus, the sediment collection depths corresponded to the depths of the filter screens in the subsequently installed sampling wells (5–6, 11–12, 17–18, and 23–24 m). For ML3, intact core retrieval from the water-saturated sediments was not possible during drilling. Therefore, sediment subsamples were collected with acid-washed polypropylene spoons from polyethylene jars containing mixed sediments from the respective depths, which may have caused artifacts due to sediment disturbance and short exposure to atmospheric oxygen.
The sediments intended for molecular and iron analysis were then transferred to acid-washed falcon tubes, stored at −20°C, and transported to the ICBM laboratories at the University of Oldenburg for subsequent processing. There, the frozen samples were freeze-dried, and triplicate samples weighing ~10 grams each were prepared for leaching experiments.
2.2.2 Grain size analysis
Grain size fractions of the sediments were measured with a laser scattering particle size distribution analyzer LA-950V2 by Horiba (Hanover, Germany). For preparation homogenized samples were thoroughly mixed with sodium metaphosphate (2%) and sieved through a 2 mm mesh. Measurements were performed in triplicates.
2.2.3 Sampling of STE porewater for bulk DOM and Fe-DOM coprecipitation experiments
Porewater samples were collected from the three multilevel wells (ML1, ML2, and ML3; Figure 1d) during campaigns conducted in September, October, and December 2022. These months were specifically chosen to capture temporal dynamics in porewater DOM, redox regime, and Fe composition, as well as to assess their influence on Fe-DOM coprecipitation patterns. September marks the transition from summer to autumn, characterized by elevated temperatures and high primary production in the coastal water column; October serves as an intermediary period of decreasing primary production; and December represents the onset of winter, a time of low temperatures, low oceanic primary production and reduced (micro)biological activity in the STE (Reckhardt et al., 2024; Auer et al., 2025). The porewater samples were obtained from the 1 m long filters at depths of 6, 12, 18, and 24 m using submersible battery-driven 12V pumps (Eijkelkamp “Gigant”). Prior to porewater sampling, environmental parameters (salinity, dissolved O2, pH) were measured in a flow-through cell using a WTW 3630 IDS multi-sensor. In addition, the well porewaters were tested in situ for detectable Fe2+ by adding small (1 mL) filtered aliquots to a ferrozine solution and visually comparing the color against a reference table (Viollier et al., 2000).
Thereafter, bulk DOM samples were collected from all wells and depths, yielding a total of 36 (12 per campaign). A second set of porewater samples was collected from those wells with detectable Fe2+ levels for Fe-DOM coprecipitation experiments (Figure 2a). They included the 18 and 24 m wells at ML1 and ML2, and all wells at ML3, totaling 24 samples (8 per campaign). Finally, seawater was sampled from the surf zone (Figure 3) at the low water line during each sampling campaign (3 total). Porewater and seawater samples for bulk DOC and DOM analyses were filtered through pre-combusted GMF-GFF filters (2 and 0.7 μm pore sizes, respectively) into acid-washed high-density polyethylene (HDPE) bottles and immediately acidified to pH = 2 (HCl, ultrapure grade). Porewater samples for iron analysis were filtered through acid-cleaned and ultrapure water-rinsed 0.2 μm pore size polyethersulfone (PES) filters into 30 mL acid-cleaned HDPE bottles and 300 μL concentrated distilled HNO3 was added to obtain a pH <2. The 24 replicate porewater samples for Fe-DOM coprecipitation analyses were filtered through pre-combusted GMF-GFF filters but afterwards allowed to oxygenate under atmospheric O2 for 24 h at 4°C. Thereafter, a second filtration was conducted to remove precipitated Fe-DOM, and the filtrate was acidified and stored for further analysis. Fluorescent dissolved organic matter (FDOM) was quantified using an AquaFluor fluorometer (Turner Instruments). This fluorometer has a sensitivity range 0.03–1,000 ppb QSE (quinine sulfate equivalents) and an excitation wavelength of 375 nm and an emission wavelength of >420 nm, which corresponds to terrestrial and aquatic humic-like organic matter (Waska et al., 2021). FDOM was determined in 4 mL filtered unacidified aliquots of bulk and Fe-DOM samples, both before and after oxygenation, to assess the impact of coagulation on the humic-like DOM fractions. After acidification, all DOM samples were stored at 4°C in the dark.
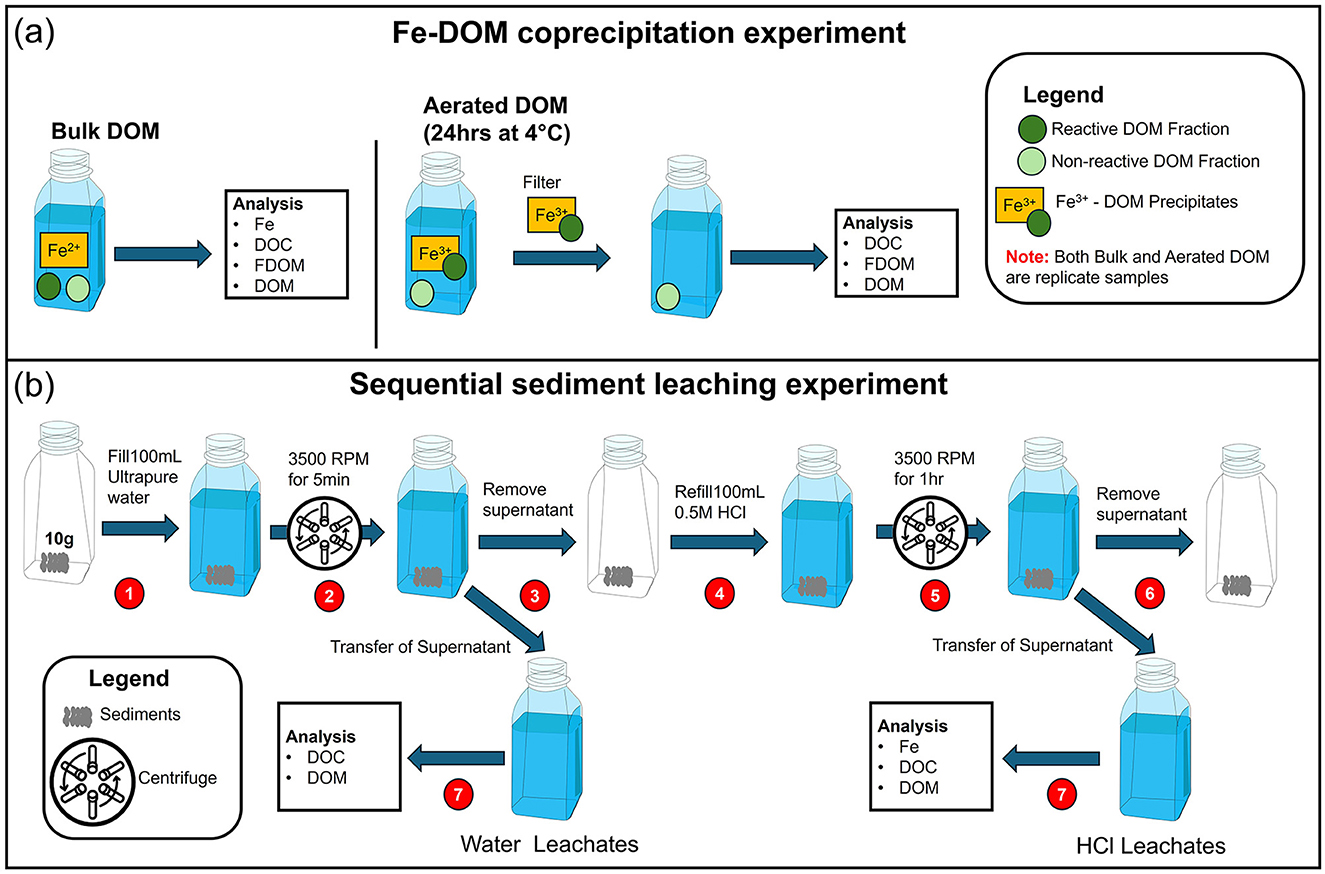
Figure 2. Schematic representation of the experimental setups: (a) Fe-DOM coprecipitation, and (b) sequential sediment leaching.
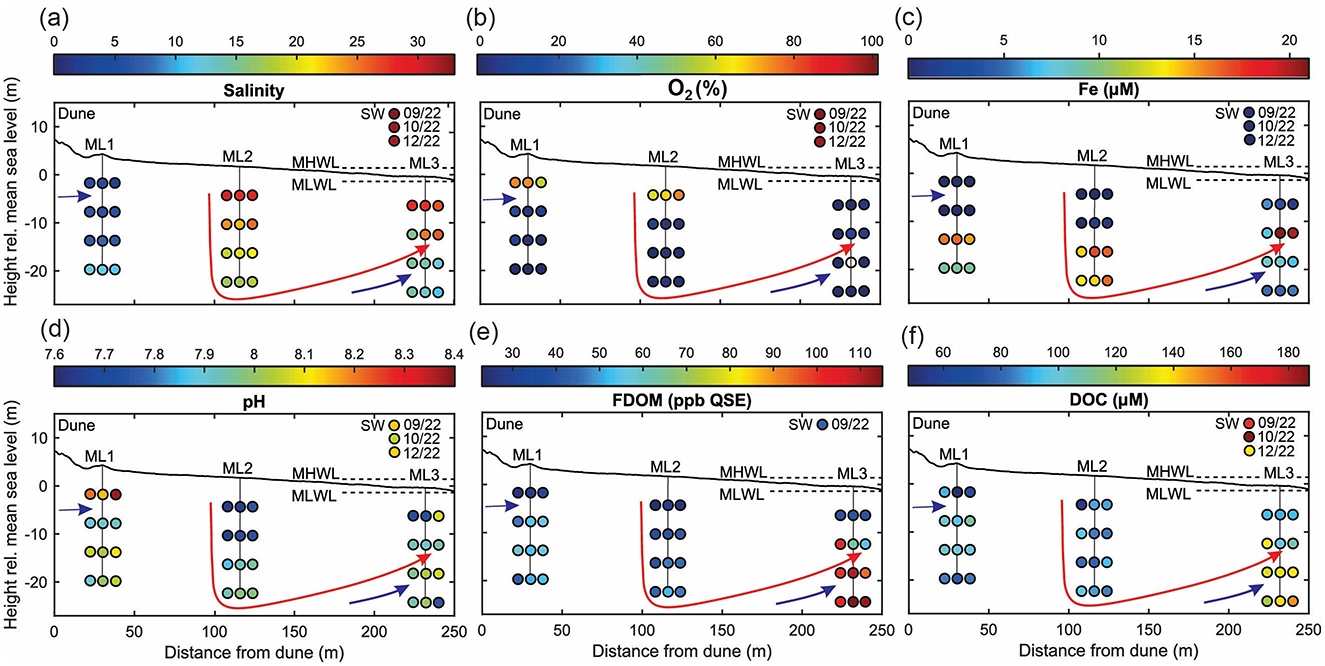
Figure 3. Spatio-temporal dynamics of porewater chemistry in the deep subterranean estuary (STE) across multiple locations (ML1, ML2, and ML3), from 6 to 24 m depth, during three sampling campaigns (September, October, and December 2022). Panels show: (a) salinity distribution, (b) oxygen saturation, (c) Fe concentrations, (d) pH, (e) FDOM distribution, and (f) DOC concentrations. Blue and red lines represent fresh/brackish and saline groundwater flow, respectively. The presented topography reflects the beach state in September 2022 and was adapted from Massmann et al. (2023).
2.3 Sample processing and analyses
2.3.1 Extraction of weakly and strongly bound DOM and trace metals from deep core sediments
To investigate organic matter and iron fractions associated with deep core sediments from the STE, sequential leaching experiments were conducted using different solvents (Figure 2b). First, ultrapure water was used to extract the loosely adsorbed OM. Afterwards, 0.5 M hydrochloric acid (HCl) was utilized to dissolve poorly crystalline Fe oxyhydroxides and monosulfides, and leach the excess, Fe-bound DOM (Zhou et al., 2024). Triplicate sediment samples of ~10 g each were used for both leaching steps. For the water leachates, the 10 g sediments were mixed with 100 mL of ultra-pure water in acid-washed HDPE bottles, shaken for 5 min, and centrifuged at 3,500 rpm to collect the supernatant. After centrifugation, water leachate subsamples were carefully pipetted from the supernatant and filled into acid-cleaned HDPE bottles for DOC, DOM, and iron analyses. To the residual sediments, we then added 100 mL of 0.5 M HCl, homogenized it for 60 min, and centrifuged it at 3,500 rpm to obtain the HCl leachate. After leaching, the remaining sediment was washed with ultra-pure water and freeze-dried for storage. Process blanks for water, 0.5 M HCl, and filters were collected at the beginning and end of the experiment. Overall, DOC concentrations in these blanks were <5% of sample DOC concentrations. The cold HCl method has practical advantages compared to other established approaches, such as those employing citrate-dithionite-bicarbonate as leaching solvents (e.g., Hyacinthe et al., 2006; Lalonde et al., 2012; Sirois et al., 2018). It offers a more reliable, rapid, and cost-effective alternative while reducing the potential for organic contamination from citrate and other reagents used in citrate-dithionite-bicarbonate protocols. Additionally, the use of ultrapure water and cold 0.5 M HCl ensures the effective sequential separation of loosely adsorbed OM and the dissolution of poorly crystalline Fe oxyhydroxides and monosulfides in sediments and has been applied increasingly in environmental studies (Sutherland, 2002; Seibert et al., 2019; Zhou et al., 2024).
2.3.2 Total organic carbon, trace metals, and DOC analysis
For total organic carbon (TOC) analysis, freeze-dried sediment samples were first homogenized using an agate mill. TOC was determined by subtracting the inorganic carbon (TIC) from the total carbon (TC). Both TC and TIC were measured with the Eltra CS 580 Carbon Sulfur Determinator. The trueness and precision of TC and TIC measurements, based on repeated Loess 2.3 tests, were within 3%. The precision of the calculated TOC was within 16%.
Trace metals in sediment HCl leachates were measured with ICP-OES (Thermo Fisher Scientific). Trueness and precision were assessed using the SLRS-6 international reference solution (National Research Council of Canada) and self-prepared reference materials. In the porewater samples, Fe was measured using triple quadrupole ICP-MS (Thermo Fisher Scientific) for low concentrations and ICP-OES (Thermo Fisher Scientific) for high concentrations. Trueness and precision were determined using international standards (CASS-6 and SLEW-4, National Research Council of Canada) and self-prepared solutions. Trueness and precision for both ICP-MS and ICP-OES measurements were better than 10%.
DOC concentrations in the sediment leachates, bulk DOM and Fe-DOM samples, and solid-phase extracted samples were quantified using the high-temperature catalytic oxidation method implemented on a DOC Analyzer (Shimadzu TOC-VCPH). The analytical precision and trueness were confirmed to be within 10%, validated against deep-sea reference material (provided by Dr. Hansell at the University of Miami, FL, USA), and low-carbon ultrapure water.
2.4 Molecular characterization of DOM using solid-phase extraction and ultrahigh-resolution mass spectrometry
Solid-phase extraction and ultrahigh-resolution mass spectrometry were employed to investigate the molecular composition of sediment leachates, porewater before and after Fe-DOM coagulation, and seawater DOM. For the sediment leachates, ~45 mL of water and HCl leachates were adjusted to pH = 2 with HCl and sodium hydroxide and desalted and concentrated using 100 mg Agilent Bond Elut PPL cartridges, following Dittmar et al. (2008). Prior to extraction, the cartridges were soaked and repeatedly rinsed with methanol and acidified ultrapure water (pH = 2). After passing the samples through, the resins were washed with acidified ultrapure water (pH = 2), dried under N2 gas and DOM was sequentially eluted with 5 mL Optima-grade methanol and 3 mL dichloromethane. The elutes were mixed, dried under argon, reconstituted in 2 mL methanol, and stored at −20°C. It should be noted that the leachates were not filtered prior to the extraction step. Although the PPL resin retains only DOM (i.e., dissolved molecules and small colloids) in its pore space, it is still possible that some suspended solids were captured on the 20 μm frit or between the resin grains. Therefore, some DOM may have been leached from these particles during elution (Waska et al., 2015). In contrast to porewater and seawater extractions, a dichloromethane elution step was included for the sediment leachates. Previous sediment leaching experiments yielded lower SPE-PPL recoveries compared to those from water samples (Dittmar et al., 2008; Zhou et al., 2024) which we attributed to a higher affinity of more aromatic, hydrophobic mineral-associated compounds to the PPL resin that could not be mobilized by methanol. However, extraction efficiencies including the additional dichloromethane elution were still rather low compared to those for porewater and seawater DOM samples and ranged from 4% to 63% (average: 17% ± 20%) for water leachates and from 3% to 43% (average: 12% ± 9%) for HCl leachates, based on DOC. For bulk porewater DOM, porewater Fe-DOM, and seawater, ~200 mL of filtered and acidified (pH = 2) samples (n = 75) were desalted and concentrated using 200 mg Agilent Bond Elut PPL cartridges, following Dittmar et al. (2008). Samples were eluted with Optima-grade methanol and stored at −20°C, with average extraction efficiencies ranging between 48% and 66% (Average of 53% ± 11% based on DOC).
Extracts were analyzed using a 15 Tesla Bruker solariX XR Fourier transform ion cyclotron resonance mass spectrometer with electrospray ionization (ESI-FT-ICR-MS) in negative mode. Samples were adjusted to 2.5 ppm DOC in a 1:1 v/v methanol: ultrapure water matrix, filtered (0.2 μm PTFE), and directly infused with a flow rate of 20 μL min−1. Ion accumulation time was 0.2 s, and 200 broadband mass scans were accumulated per sample. An in-house deep-sea reference (Green et al., 2014) was analyzed daily to monitor instrument drift. Mass spectra were internally calibrated (<0.1 ppm error) and processed using the open-source program ICBM-OCEAN (Merder et al., 2020) for noise removal, peak alignment, and molecular formula assignment (<0.5 ppm error, C1 − 100H1 − 200O1 − 70N0 − 6S0 − 2P0 − 1.). To ensure unique formula assignments, the ICBM-OCEAN program applies a hierarchy of knock-out criteria, prioritizing isotopolog verification, homologous series length, and assignment error. All assigned unique molecular formulae were categorized into distinct molecular groups based on their elemental ratios. The resulting cross tables containing molecular formulae, chemical characteristics, and sample-specific signal intensities were then exported from ICBM-OCEAN for further processing.
2.5 Data processing
Two main datasets were assembled for DOM molecular composition and environmental parameters (salinity, oxygen concentration, pH): (1) sediment leachates and (2) Fe-DOM coprecipitation data (bulk and removed), including porewater and seawater samples. Data processing was conducted individually for each dataset. Data crosstables were then filtered to retain only monoisotopic masses for assigned molecular formulae, enhancing the prominence of less prevalent compounds by removing 13C-containing formulae before statistical analysis. Samples were normalized based on their intensity sums, and cumulative sums of molecular properties (elemental composition, compound group classification) were calculated from the relative signal intensity distributions. Five distinct DOM molecular indices from existing literature were retrieved and applied:
(1) A terrestrial index (ITerr) of 80 prevalent formulae correlated with the salinity gradient in the Amazon estuary (Medeiros et al., 2016).
(2) A broad DOM lability index (MLBL), partitioning formulae based on the H/C ≥ 1.5 molecular lability boundary (D'Andrilli et al., 2015).
(3) The molecular degradation index (IDEG), comprising 10 commonly occurring formulae from open ocean DOM which are significantly positively and negatively correlated with 14C radiocarbon ages (Flerus et al., 2012).
(4) The modified aromaticity index (AImod), first defined by Koch and Dittmar (2006), provides a measure of the aromaticity of a molecule based on its elemental stoichiometry.
(5) The double bond equivalence (DBE), as reported by Stenson et al. (2003) represents the number of rings plus double bonds to carbon in a molecule and is calculated for each molecular formula.
3 Results
3.1 Hydrochemistry of the deep STE
In line with Reckhardt et al. (2024), the porewaters of the investigated STE wells contained varying proportions of fresh groundwater and recirculating seawater under oxic to anoxic conditions (Figure 3). Spatially, ML1 showed salinities ranging from ~2 to 12 (average = 7) and remained oxic to a depth of 18 m (Figures 3a, b). ML2 exhibited salinities between ~17 and 30 (average = 23) and was oxic down to 12 m. ML3 displayed higher salinities from 6 to 12 m (15–28) but lower salinities below 18 m (11–15), with anoxic conditions observed throughout. Dissolved Fe was detectable at depths ≥18 m in ML1 and ML2, while it was measurable at all depths in ML3 (Figure 3c). Overall, porewater pH was slightly alkaline to mildly basic (7.6–8.4) in the investigated wells and was highest at ML1 at 6 m (Figure 3d). FDOM values varied across stations, with the highest average observed at ML3 (80 ± 3 ppb QSE), approximately double that of ML1 (45 ± 12 ppb QSE) and ML2 (39 ± 9 ppb QSE; Figure 3e). At ML3, FDOM was lower in the upper 12 m and higher in deeper, low-salinity waters. DOC concentrations followed a similar trend, with the highest average recorded at ML3 (119 ± 25 μM), exceeding ML1 (92 ± 27 μM) and ML2 (86 ± 5 μM). At ML3, DOC was stratified, showing lower concentrations at 6–12 m and higher concentrations at 18–24 m. All porewater DOC concentrations were lower than seawater levels (162 ± 24 μM) from the same campaign (Figure 3f). Groundwater salinity and redox gradients at ML1, and to a lesser extent at ML2, were consistent across the investigated months and depths compared to ML3 (Figure 3). DOC concentrations at ML1 and ML2 were highest in September, lowest in October, and intermediate in December. In contrast, FDOM exhibited a different trend, with the highest values recorded in December at ML1 and in October at ML2. At ML3, Fe and DOC concentrations, as well as FDOM values, particularly at 12 m across the investigated months, covaried with the porewater salinity gradient above and below 12 m (Figure 3a).
3.2 Sediment stratigraphy, grain size, and geochemical profile
Grain sizes at depths of 6, 12, 18, and 24 m below the beach surface varied across ML1, ML2, and ML3 (Figures 4a–c), with coarser grain sizes observed at greater depths. At ML1, fine sand predominated, with median sizes ranging from 145 to 302 μm. ML2 showed a slight trend toward coarser grain sizes, from fine-medium sand in the upper layers (198–208 μm) to medium sand at greater depths (380–381 μm). At ML3, grain size gradually increased from fine sand in the upper layers (183–209 μm) to medium sand at deeper levels (329–353 μm). Gravel was present at 18 and 24 m depths in ML2 and ML3 but was removed prior to measurement, preventing quantification of the gravel fraction.
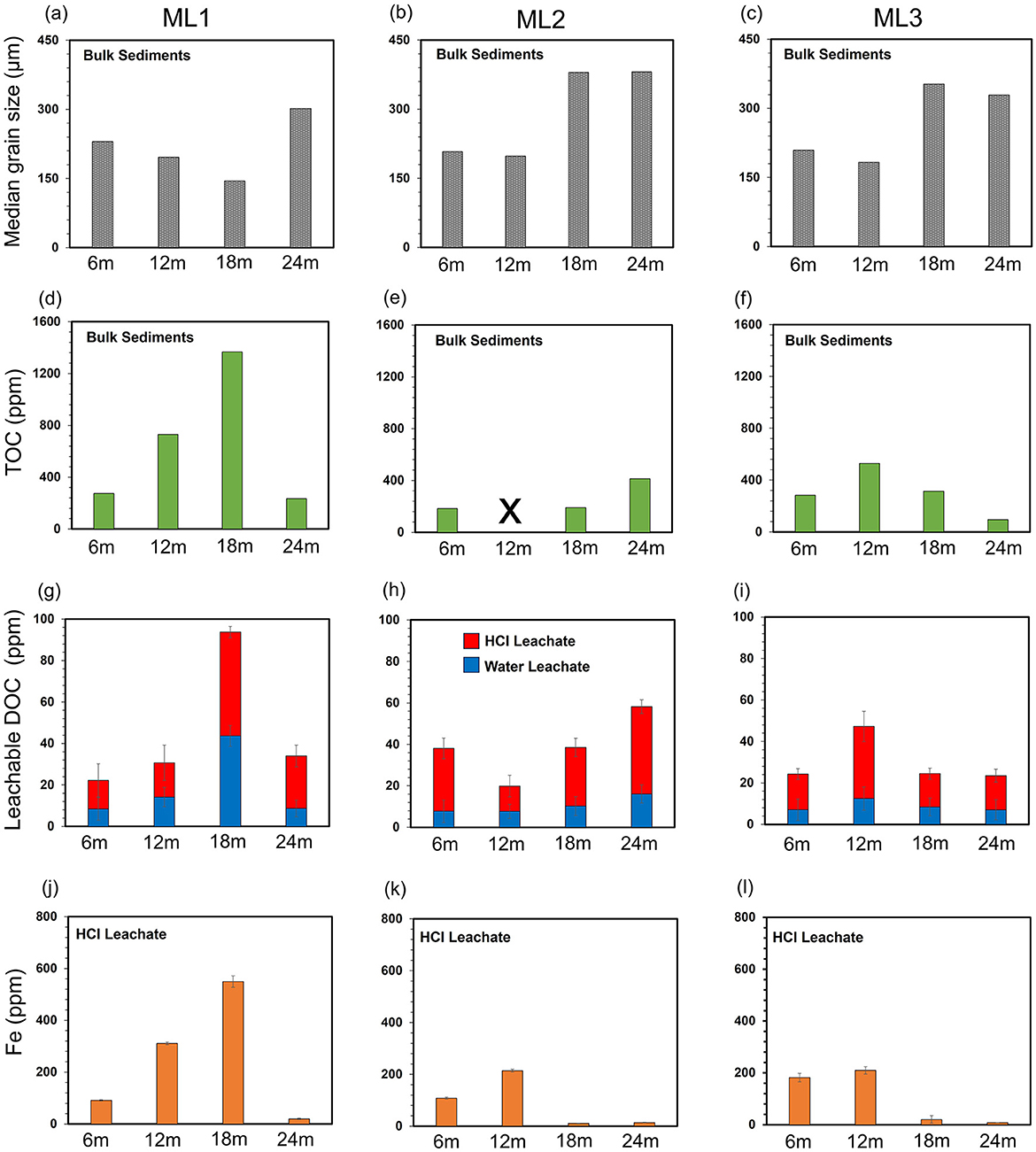
Figure 4. Sediment stratigraphy, median grain size distribution, and geochemical profiles of bulk sediments, porewater, and 0.5 M HCl leachates from deep subterranean estuary (STE) core sediments (6–24 m depth) at stations ML1, ML2, and ML3. Panels (a–c) median grain size distributions in STE sediments; (d–f) TOC distribution in STE sediments; (g–i) DOC concentrations in water and HCl leachates; and (j–l) Fe concentrations in HCl leachates. Triplicate samples were analyzed for leachable DOC and Fe. Bars represent average values, and error bars indicate standard deviations.
Total organic carbon (TOC) concentrations ranged from below the detection limit to 1,370 ppm and varied across stations and depths (Figures 4d–f). The highest levels were observed at ML1, followed by ML3 and ML2. With the exception of ML2, where TOC was highest in the coarse 24 m fraction, TOC concentrations were generally higher in fine-grained sediments and lower in the coarse 24 m fraction. In comparison, TOC was about 200–4,200 times higher than DOC in the ambient porewater over the 3 months studied (September, October, and December 2022; Supplementary Table 1).
The concentrations of leachable dissolved organic carbon (LOC) were slightly higher in HCl leachates (13 ± 5 to 50 ± 2 ppm) than in water leachates (7 ± 4 to 44 ± 4 ppm) and decreased progressively from ML1 to ML3 in both cases (Figures 4g–i). Although no consistent depth-related trends were observed for LOC concentrations, their general patterns mirrored those of TOC. Normalized to bulk sediment concentrations, leachable LOC from both HCl and water leachates accounted for ~4%−14% of the TOC in the bulk sediments (Supplementary Table 1). Furthermore, loosely bound DOC and DOC associated with the poorly crystalline Fe phases were up to ~130 and 160 times higher than porewater DOC, respectively (Supplementary Table 1).
Fe concentrations in the HCl leachates ranged from 8 to 550 ppm (Figures 4j–l). No consistent depth-related trends were observed for Fe; however, its concentration generally decreased from ML1 to ML3. With the exception of ML2, Fe profiles at ML1 and ML3 mirrored those of TOC and LOC, with higher Fe concentrations found in finer sediments. Additionally, Fe/LOC ratios for HCl leachates at ML1 and ML3 generally mirrored the depth profiles of Fe and LOC concentrations, while discrepancies were observed at ML2 (Supplementary Table 1). At ML1 and ML2, the highest Fe/LOC ratios occurred at 18–24 m, and the lowest at 6 m. At ML3, the highest ratio was observed at 12 m, and the lowest at 18 m. No consistent depth trend was observed across the stations (Supplementary Table 1).
3.3 Molecular composition of DOM in sediment leachates, porewater and seawater
Overall, sea- and porewaters in the deep STE of Spiekeroog had higher SPEDOC/SPETDN ratios compared to both water and HCl leachates (Table 1). Porewater also exhibited the highest molecular diversity indicated by numbers of assigned molecular formulae, surpassing both seawater and leachates. Water leachates had the lowest molecular diversity, except at ML1, where it was slightly higher than in HCl leachates. Porewater DOM had the highest molecular mass, exceeding seawater and leachates. Molecular diversity and mass increased with depth at ML1 and ML2 but decreased at ML3. Depth trends for water leachates were inconsistent, although molecular diversity and mass decreased from ML1 to ML3. In HCl leachates, depth and spatial trends were inconsistent at ML2 and ML1; however, at ML1, both molecular diversity and mass increased with depth.
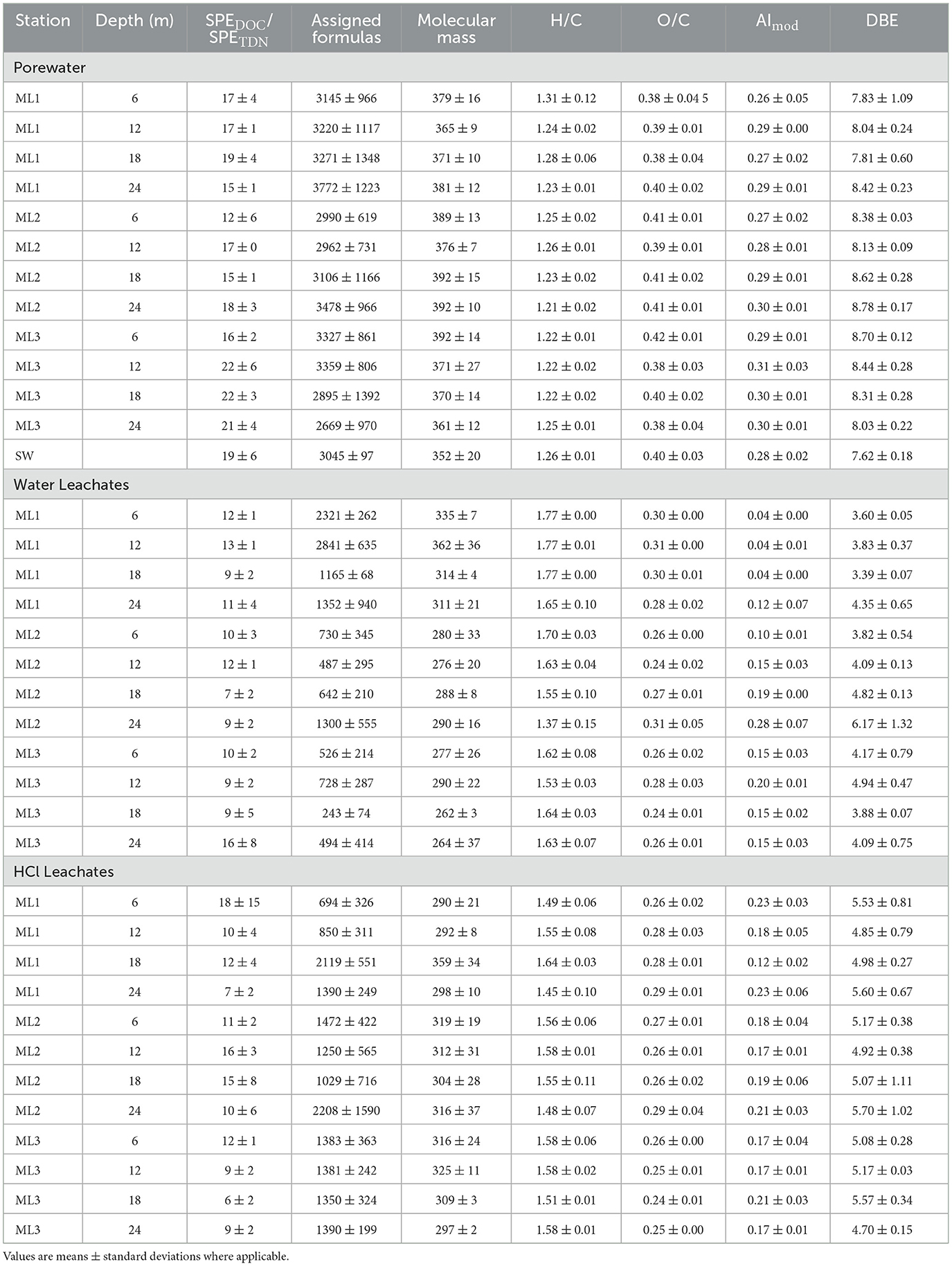
Table 1. Molecular characteristics of DOM in porewater, sediment leachates (water and HCl) across three multilevel wells (ML1, ML2, ML3), and seawater.
Elemental stoichiometries of water leachates exhibited the highest H/C ratios and MLBL values, followed by HCl leachates, and then sea- and porewaters (Table 1; Figures 5a–c). In contrast, sea- and porewaters had the highest O/C ratios, AImod, and DBE values, followed by HCl and water leachates. Spatial variation in H/C and O/C ratios, AImod, and DBE values in porewater and leachates were not associated with specific depths or stations, although MLBL in porewater was higher at ML1 compared to ML2 and ML3. Among the molecular indices, the terrestrial index (ITerr) was highest in HCl leachates and showed the strongest variation between depths and stations (Figures 5d–f). In contrast, ITerr values in porewater and seawater were relatively low and remained fairly constant across the spatial scale. The degradation index (IDEG) was lowest in sediment leachates and highest in STE porewaters, with intermediate values for seawater (Figures 5g–i). Consistent with the lower H/C ratios, sea- and porewaters had higher proportions of aromatic compounds and were dominated by highly unsaturated compounds compared to water and HCl leachates (Supplementary Figure 1). In contrast, the sediment leachates were primarily composed of unsaturated compounds (Supplementary Figure 1). No clear depth or beach cross-sectional trend was observed, except for water leachates at ML2, where aromatic and unsaturated compound classes increased with sampling depth.
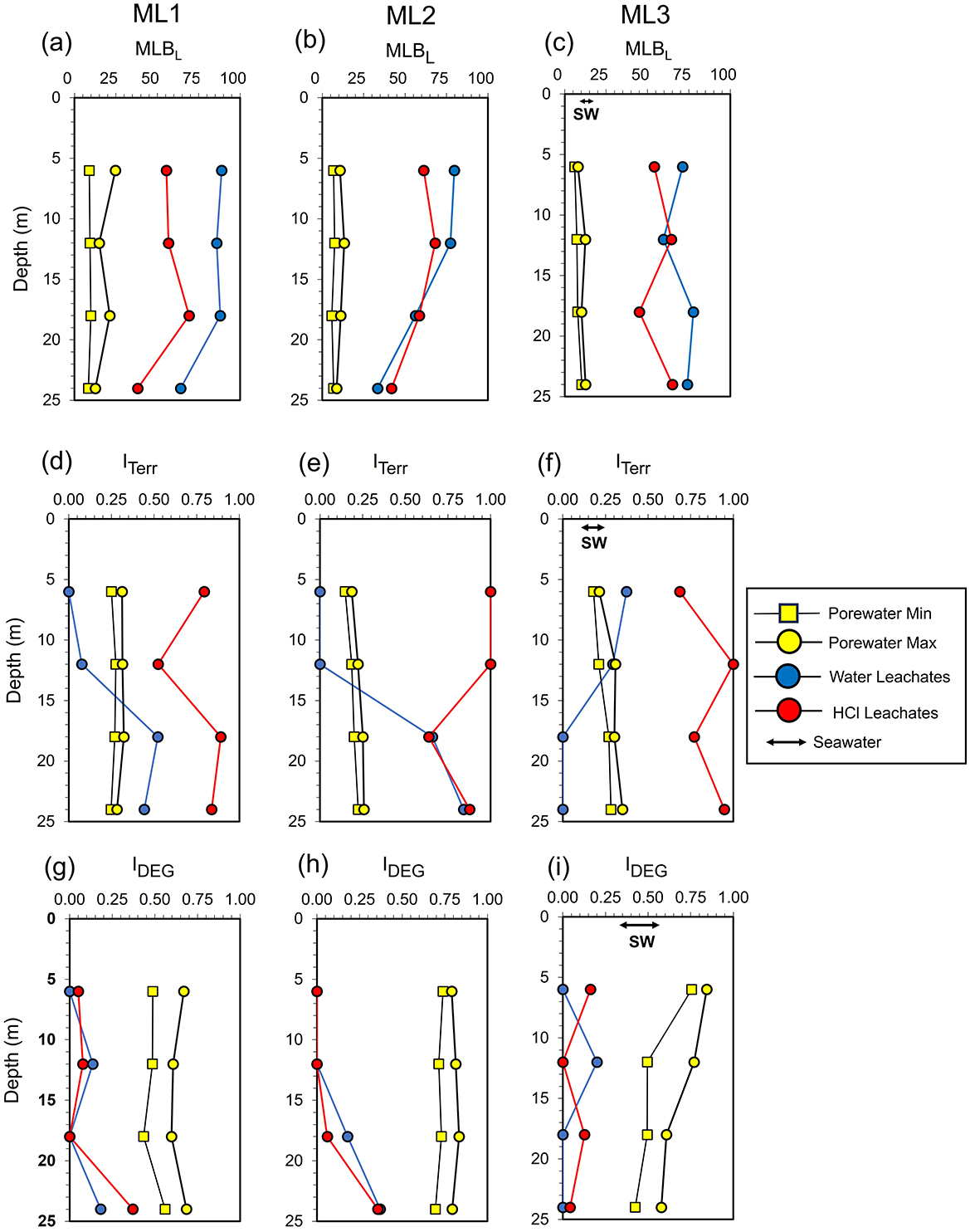
Figure 5. Depth profiles of molecular indices for dissolved organic matter (DOM) in porewater, seawater (SW), water leachates, and 0.5 M HCl leachates at stations ML1, ML2, and ML3. The indices include (a–c) the molecular lability boundary index (MLBL) from D'Andrilli et al. (2015); (d–f) the terrestrial index (ITerr) from Medeiros et al. (2016); (g–i) the degradation index (IDEG) from Flerus et al. (2012). The porewater min/max represent ranges from September, October, and December 2022 samples, while leachate values are based on averages from experimental triplicates.
Van Krevelen plots (Figure 6) of unique molecular formulas for each leachate type revealed distinct compositional differences. Water leachates were characterized by a higher proportion of oxygen- and hydrogen-rich labile compounds (upper right quadrant), with decreasing abundance from ML1 to ML3. HCl leachates exhibited a more diverse composition, with a higher proportion of oxygen-poor aromatic compounds (lower left quadrant) which decreased from ML1 to ML3 and increased with depth, along with a substantial amount of oxygen-poor, hydrogen-rich formulas (upper left quadrant).
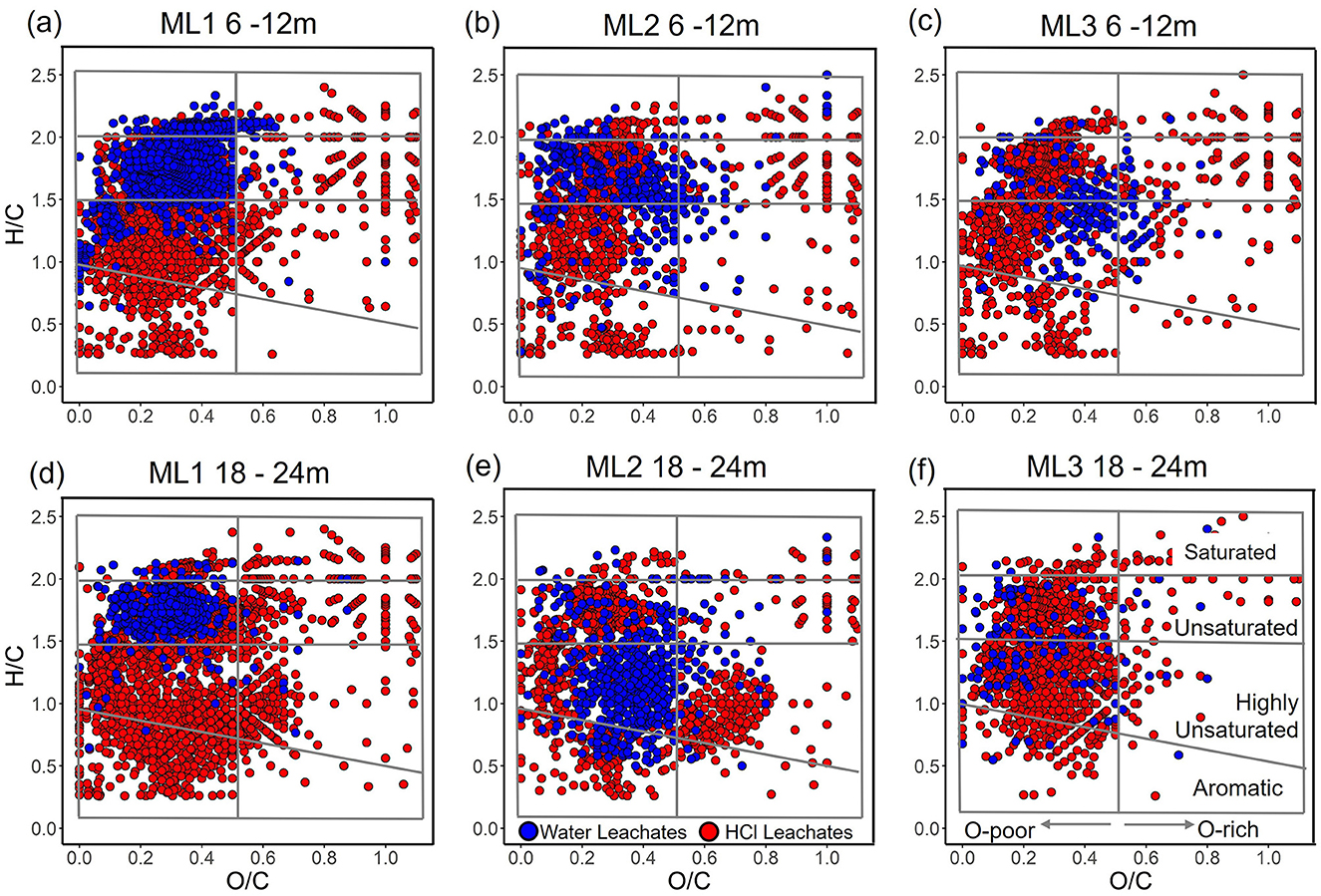
Figure 6. van Krevelen plots of molecular formulas unique to ultrapure water (blue) and 0.5 M HCl (red) leachates from deep core sediments (6–24 m) at stations ML1, ML2, and ML3. Panels (a–c) H/C and O/C ratios at 6 and 12 m depth at ML1, ML2, and ML3, respectively, while panels (d–f) H/C and O/C ratios at 18 and 24 m depth at ML1, ML2, and ML3, respectively. Compound classes are defined by H/C and O/C ratios according to Merder et al. (2020).
3.4 Molecular changes after aerating anoxic porewaters of the deep STE
The Fe-DOM coprecipitation experiment revealed complex spatiotemporal variations in DOM removal efficacy across anoxic porewater samples (i.e., samples with detectable concentrations of Fe2+; Reckhardt et al., 2024). This was particularly evident at depths of 18–24 m at ML1 and ML2, and 6–24 m at ML3 (Figures 3b, c). In these anoxic porewaters, pH levels ranged from 7.6 to 8.2 (Figure 3d). Initial DOC concentrations prior to aeration ranged from ~75 to 153 μM, while Fe concentrations ranged from 9 to 21 μM, resulting in molar Fe/DOC ratios of 0.01 to 0.22. FDOM values before aeration ranged from 24 ppb QSE to 114 ppb QSE, with maximum values observed at 18–24 m at ML3 (Supplementary Table 2).
Following aeration, FDOM removal exhibited a spatiotemporal gradient: highest in September (~50%), moderate in October (~11%), and minimal in December (~7%; Figures 7a–c). Spatially, the greatest FDOM reductions occurred at ML1 and ML3 (≥12 m), with intermediate reductions at ML2. DOC concentrations showed minimal changes after aeration, with notable decreases in September (~12% at ML1; ~5% at ML3). In October and December, slight increases in DOC concentrations were observed. Overall, changes of <10% were considered negligible, as they were below the precision of the TOC analyzer used (Figures 7d–f). In line with FDOM trends, molecular DOM composition in porewater also changed following aeration, showing both spatial and temporal differences (Tables 2–4; Figure 8). In September, removal primarily affected highly unsaturated and saturated compounds, resulting in decreases of ~5% and 52%, respectively (Figures 8a–d; Table 2). This selective removal led to slight reductions in molecular mass, O/C ratio, DBE, and AImod values of up to 5%. The highest removal of highly unsaturated compounds occurred at ML1 and ML2, with intermediate values at ML3. This differential removal caused a ~65% increase in unsaturated compounds and a ~49% increase in aromatic compounds in the remaining DOM fraction (Table 2). In October, the removal shifted toward aromatic compounds (~27%) and highly unsaturated compounds (~14%), lowering AImod and DBE values by up to 14% (Figures 8e–h; Table 3). The greatest removal was observed at ML1, while the lowest was at ML3, resulting in a ~119% enrichment of unsaturated compounds in the remaining DOM fraction. In December, the removal was less pronounced compared to October and September, primarily affecting aromatic and unsaturated compounds, which decreased by up to 39% and 42%, respectively (Figures 8i–l; Table 4). This resulted in a decrease in AImod by up to 14%. The highest removal occurred at ML2 and ML3 (18 and 24 m), leading to an enrichment of saturated compounds at these depths. Despite a higher percentage of saturated compounds being removed, their relative contribution remained low (<0.5%) in the bulk samples compared to other compound classes (Supplementary Figure 1).
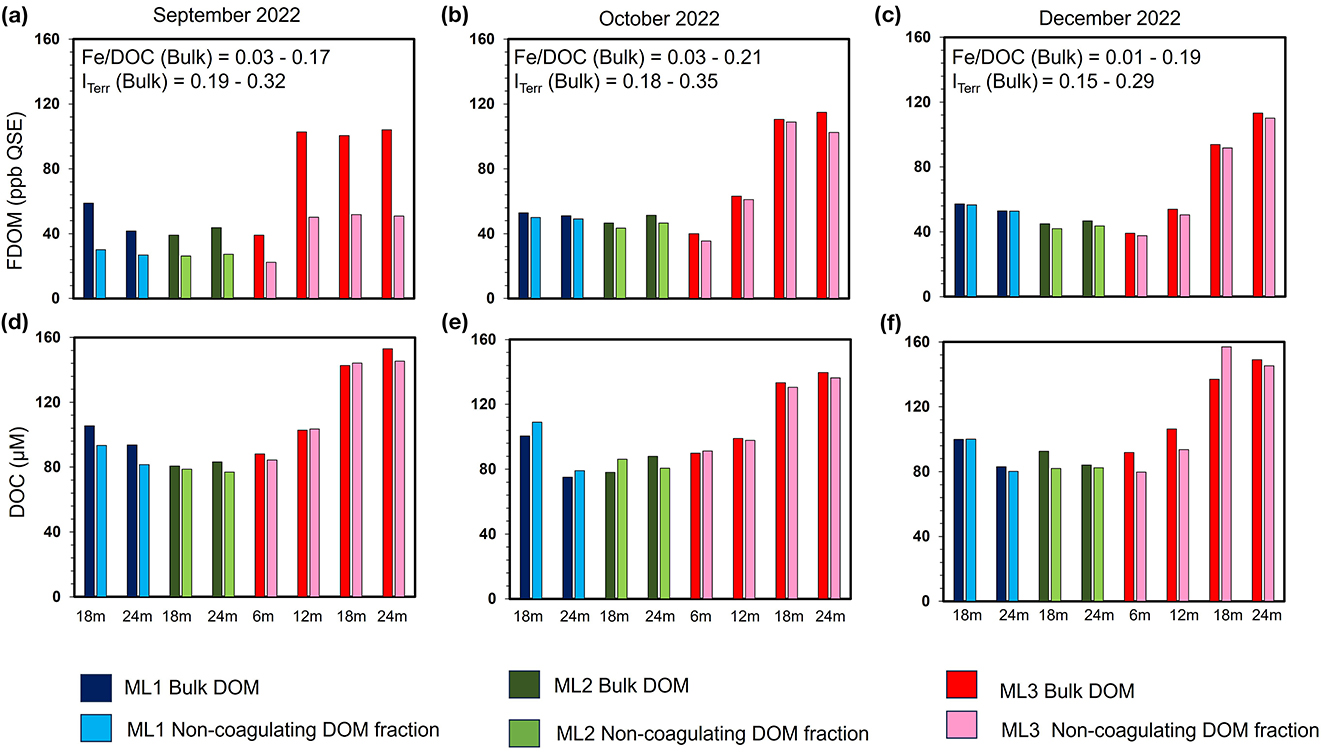
Figure 7. Spatio-temporal dynamics of humic-like fluorescent dissolved organic matter (FDOM) and dissolved organic carbon (DOC) in anoxic porewaters before and after Fe-DOM coprecipitation. (a–c) Show FDOM, and (d–f) show DOC results at stations ML1, ML2, and ML3 during the September, October, and December 2022 sampling campaigns. FDOM reduction followed a spatio-temporal gradient, with highest removal in September (~50%), moderate in October (~11%), and minimal in December (~7%). Removal was generally greatest at ML1 and ML3, with intermediate values at ML2. % loss in DOC concentrations post coagulation in most stations were within the analytical error range ( ≤ 10 μM; i.e., negative values) of the TOC Analyzer.
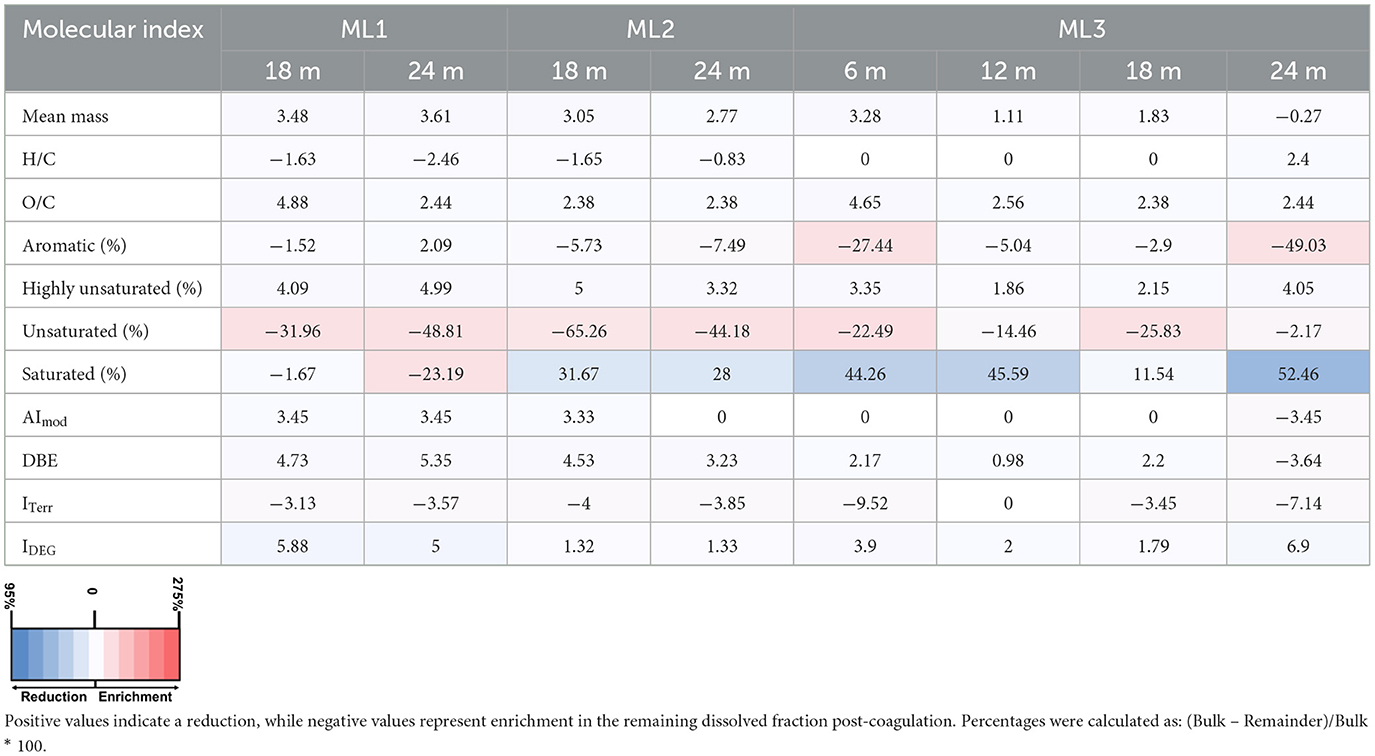
Table 2. Percentage changes in molecular indices of DOM after Fe-DOM coprecipitation experiments in September 2022 across three multilevel wells (ML1, ML2, ML3) at various depths.
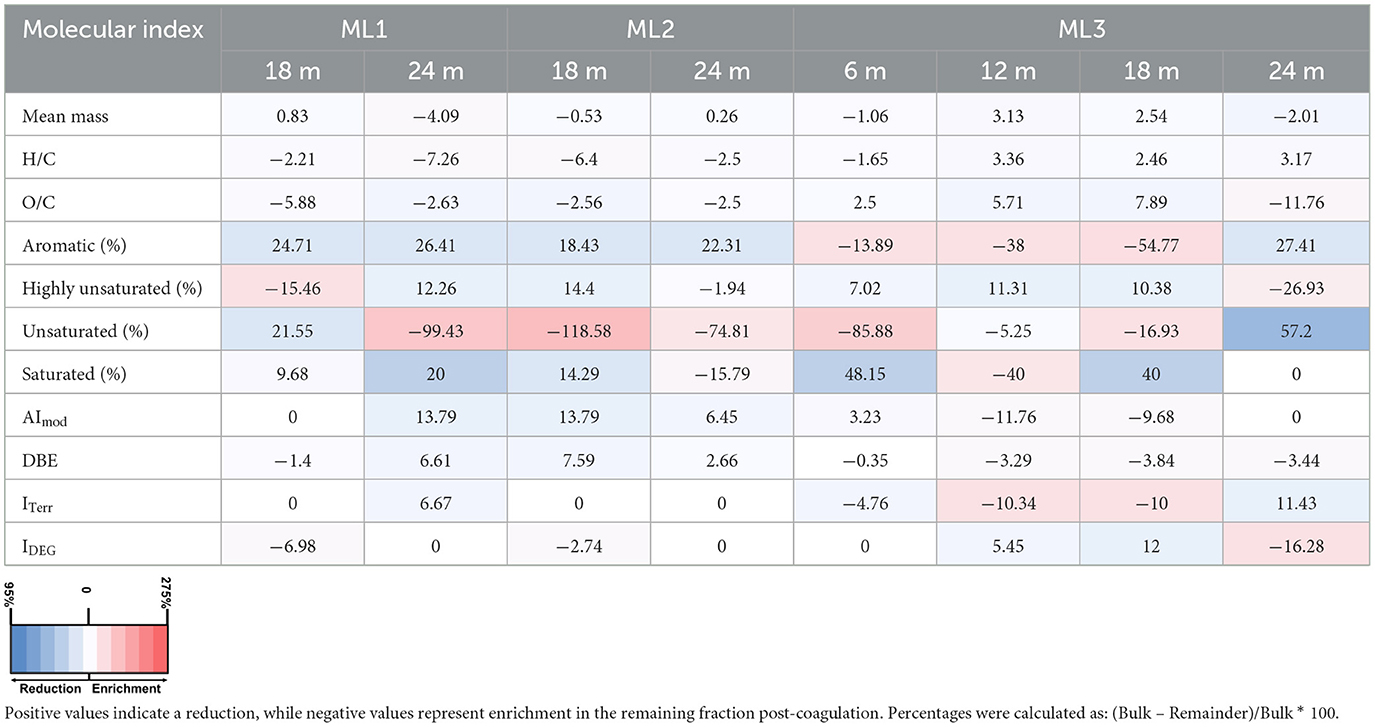
Table 3. Percentage changes in molecular indices of dissolved organic matter after Fe-DOM coprecipitation experiments in October 2022 across three multilevel wells (ML1, ML2, ML3) at various depths.
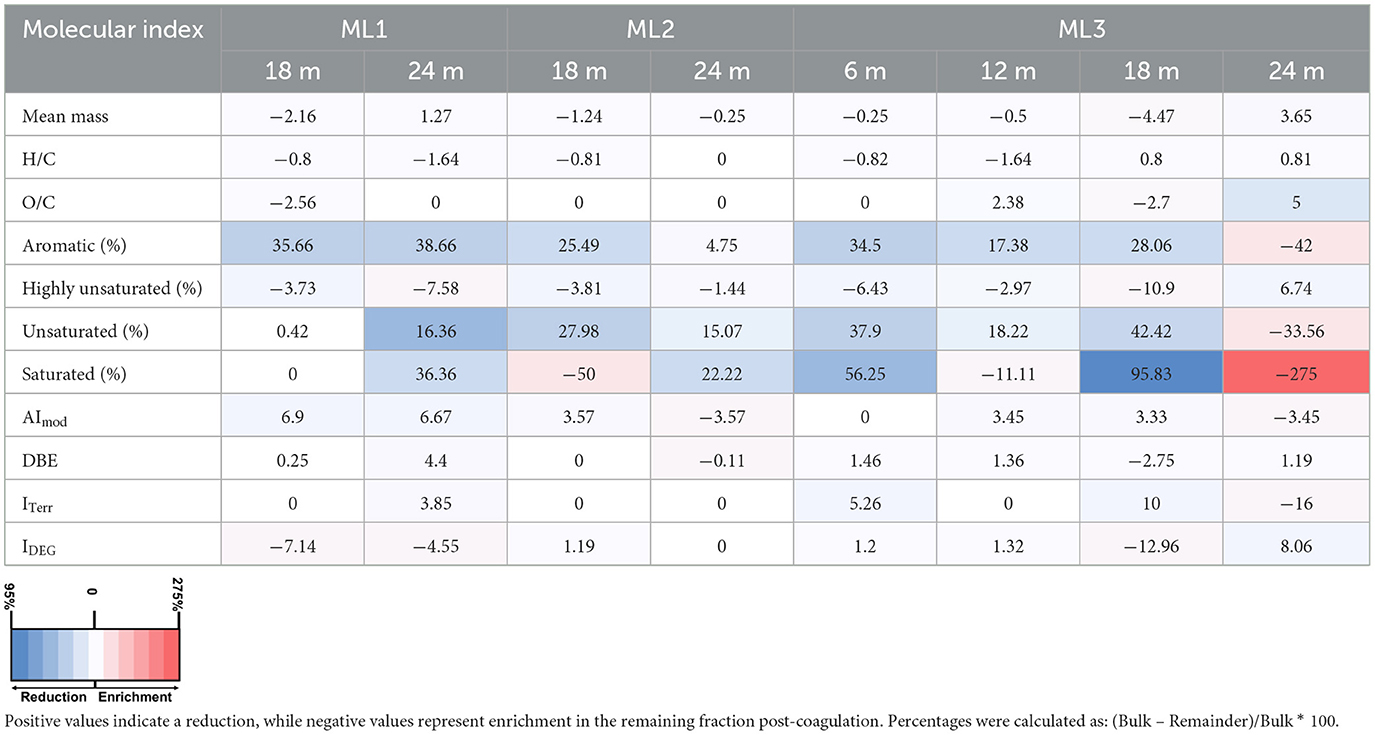
Table 4. Percentage changes in molecular indices of dissolved organic matter after Fe-DOM coprecipitation experiments in December 2022 across three multilevel wells (ML1, ML2, ML3) at various depths.
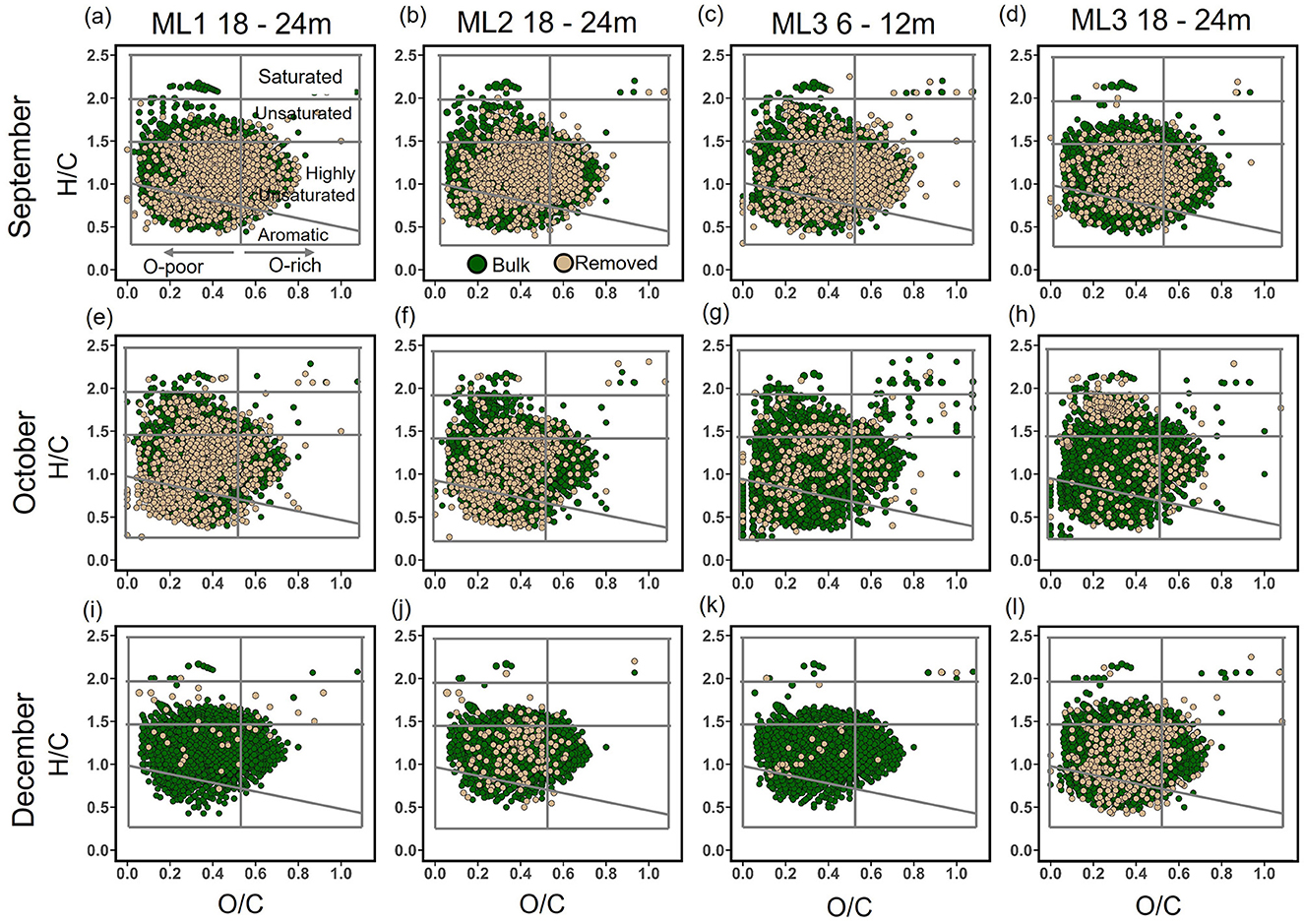
Figure 8. van Krevelen plots illustrating bulk dissolved organic matter (DOM) in anoxic porewater (dark green) and their removed fractions (light brown) before and after Fe-DOM coprecipitation across three time points: September, October, and December 2022, at stations ML1, ML2, and ML3. Panels (a–d) H/C and O/C ratios in Bulk DOM and Fe-DOM coprecipitated fractions for the September 2022 campaign; (e–h) H/C and O/C in Bulk DOM and Fe-DOM coprecipitated fractions for the October 2022 campaign; and (i–l) show H/C and O/C ratios in Bulk DOM and Fe-DOM coprecipitated fractions for December 2022 campaign. Regions correspond to compound classes defined by their H/C and O/C ratios (Merder et al., 2020).
4 Discussion
4.1 Partitioning of organic matter in the deep beach STE
4.1.1 Sources and distribution of porewater DOM of the deep STE
DOC, FDOM, and molecular data revealed spatial, source-related heterogeneity across porewater samples in the deep STE (Table 1; Figure 5). At ML1, near the dune base, elevated DOC and FDOM concentrations, along with increasing molecular diversity and mass with depth in porewater, suggest a potential contribution from the island's freshwater lens (Waska et al., 2021). The high ITerr index (Figure 5d), coupled with low porewater salinity (Figure 3a), further supports the influence of terrestrial DOM at this site. At ML2, near the high tide line, porewater DOM was strongly influenced by infiltrating seawater, as indicated by the high salinity values (Figure 3a). While molecular characteristics (such as O/C and H/C ratios) at ML2 largely resembled those at ML1, suggesting a mixture of terrestrial and marine DOM, relatively lower ITerr values (Figure 5e) indicate a greater marine influence. At ML3, near the seawater exfiltration zone, DOC and FDOM patterns diverged, with lower concentrations in the upper 6 m and higher concentrations in deeper porewaters (Figures 3e–f). These variations reflect contributions from discharging deep fresh groundwater and recirculating seawater, as indicated by salinity stratifications (Figure 3a). This pattern was consistent with the ITerr profile, where porewaters at the upper 6 m of ML3 showed lower ITerr values compared to deeper zones (Figure 5f). Despite the spatial heterogeneity in DOC and FDOM concentrations, porewater molecular composition shared similarities with seawater, particularly in H/C and O/C ratios, suggesting contributions from both fresh groundwater and recirculating seawater. These findings are consistent with previous studies at shallow depths (<5 m; Waska et al., 2021). However, porewater showed slightly higher AImod, DBE and ITerr values (Table 1; Figures 5d–f), indicating a stronger influence from fresh groundwater than from seawater (Waska et al., 2021). Moreover, porewater contained fewer labile compounds (as indicated by MLBL) and exhibited lower molecular degradation (as indicated by IDEG) compared to seawater, likely due to the older and more degraded nature of fresh groundwater DOM relative to the more freshly produced DOM in seawater (Figures 5g–i; Beck et al., 2017; Waska et al., 2021). The decrease in DOC concentrations from seawater to porewater, along with the high degradation index and abundance of unsaturated and aromatic compounds in STE porewater, indicates that due to microbial utilization, DOM in porewater ages and becomes more recalcitrant as it moves through the system, consistent with previous findings (McDonough et al., 2022; Carvalho da Silva et al., 2023). Our dataset further reveals that these changes in DOM composition occur both horizontally and vertically, extending tens of meters into the subsurface.
4.1.2 Sources and distribution of sedimentary DOM of the deep STE
In stark contrast to STE porewaters and seawater, sediment leachates revealed a distinct pool of organic matter, both in quantity and quality. Leachable dissolved organic carbon (LOC) from the sediments was up to two orders of magnitude greater than porewater DOC (Supplementary Table 1). This suggests that a substantial portion of the organic matter in the deep STE is embedded within the sediments rather than dissolved in the porewater. Variations in TOC across the beach transect (Figures 4d–f) further highlighted the heterogeneous contributions of organic matter in the deep STE, likely driven by different depositional environments, including beach, glacial, and tidal flat sediments (Figure 1d). Each of these sediment types reflects distinct depositional processes and organic matter sources, influencing their TOC content and spatial distribution. For example, beach sediments at this study site, shaped by high-energy wave action and constant reworking, are likely to have lower TOC compared to glacial sediments, which often contain organic-rich deposits like peats from past ice-covered landscapes (Foster et al., 1991). Tidal flat sediments, in contrast, are subject to periodic inundation and sedimentation, fostering the accumulation of both marine and terrestrial organic matter (Volkman et al., 2000). The low TOC and finer grain fractions observed at 6 m depths (ML1 and ML3, Figure 4) contrast with the relatively higher TOC and finer sediment fractions found in the deeper sediment layers (ML1 and ML3, Figure 4), supporting the influence of depositional environments. Discrepancies between TOC and grain size at ML2 may reflect non-uniform sediment stratigraphy of the beach (Figure 1d) or post-depositional physical (Xu et al., 2021) and biogeochemical changes (Audry et al., 2011). Additionally, the relatively low leachable DOC concentrations (about 10%−14% of the TOC) compared to the TOC in bulk sediments suggest that only a small fraction of this organic matter is easily mobilized. This highlights the role of STE sediments as long-term reservoirs for organic matter, potentially persisting over geological time scales.
Water leachates, representing the loosely bound DOM fraction in the sediments, contained a higher proportion of aliphatic and nitrogen-rich compounds with lower molecular diversity and weight, compared to other OM pools investigated here. For example, the distinct composition of these leachates, characterized by high H/C ratios and a low degradation state (Table 1; Figures 5g–i), differed markedly from ambient porewater DOM (Figure 5). These compounds are likely of marine and possibly autochthonous origin (Table 1; Figure 6). Their presence suggests that sediments could serve as a readily accessible source of organic matter for STE microbes, particularly in the deeper STE where freshly produced labile DOM from the surface is scarce. Generally, particulate organic matter (POM) from the water column is more recently bio-synthesized than DOM and is therefore more nutritionally preferred by microorganisms due to its higher labile organic content (Wakeham and Lee, 1989). In contrast, sediment POM is often much older, having been deposited over long geological timescales, and has undergone various degradation processes. As a result, sediment POM tends to be less labile and less readily available to microorganisms compared to water column POM. Water-leachable sedimentary organic matter, however, is likely more reactive and preferentially utilized by microbes compared to organic matter found in acid-based leachates, as the most labile fraction is leached first. Consequently, this sediment-derived DOM is considered more bioavailable to microorganisms than the DOM originally present in the STE's porewater. One explanation for the occurrence of these reduced, saturated compounds in the sediments is that they originate from ancient organic matter deposited during island formation which since was reworked by microbial communities in the STE (McDonough et al., 2022). However, no evidence was found to suggest that solubilization of H/C-rich DOM from sediments significantly influences porewater composition in the deep STE, as DOC concentrations and DOM composition appeared decoupled from leachable and total organic carbon in the solid phase (Figures 3d–i). At the study site, oxygen and nitrate penetrate deep into the sediments (Massmann et al., 2023), and the sand grains contain sufficient reactive iron to sustain slow but steady iron reduction (Ahrens et al., 2020). This suggests that organic matter released from the solid phase—including labile DOM, which has been shown in a recent experimental study to rapidly stimulate microbial growth (Abarike et al., 2024)—can be efficiently utilized by sediment-associated microbes. As a result, porewater signatures of sedimentary OM are likely attenuated, preventing their accumulation. The sand grains, along with their associated organic matter and minerals, may thus function as micro-reactors, driving organic carbon turnover primarily through in situ processing of solid-phase substrates rather than relying on aqueous-phase inputs.
Compared to water leachates, HCl leachates, which represent the mineral-bound DOM fraction of the sediments, exhibited even higher DOC concentrations (Figure 4). These leachates also contained a larger proportion of terrestrial DOM and were more molecularly diverse and complex, as evidenced by the higher number of assigned molecular formulas, molecular weight, ITerr values, AImod, DBE values, and aromatic compound abundance (Table 1; Figures 5d–f; Supplementary Figure 1). This suggests that a broader variety of oxidized aromatic compounds, likely terrigenous and refractory in nature, is preferentially bound to minerals in the sediments, consistent with the association of terrigenous organic matter with poorly crystalline iron in subtidal permeable sediments (Zhou et al., 2024). Lalonde et al. (2012) demonstrated that labile organic matter tends to bind more readily to Fe oxides, which may appear to contrast with our findings. However, the differences between the two studies can be explained by variations in the environmental setting and the nature of the sedimentary organic matter. In coastal STEs like our study site, the organic matter pool is largely depleted in fresh, highly reactive compounds due to prolonged subsurface residence times and extensive microbial processing (Waska et al., 2021; McDonough et al., 2022; Abarike et al., 2024). As a result, the fraction of organic matter that remains available for interaction with Fe oxides is inherently more degraded and refractory. This suggests that the reactivity of organic matter with iron oxides is not solely determined by its intrinsic lability but is also strongly influenced by its diagenetic history and the prevailing biogeochemical conditions within the system.
4.2 Experimental Fe-DOM interactions in the solid phase
In high-energy subterranean estuaries (STEs), the dynamic redox conditions, influenced by advection, enable iron to play a crucial yet intricate role in the transformation of dissolved organic matter (DOM). Iron alternates between its Fe3+ and Fe2+ states, acting as an electron acceptor during organic matter decomposition (Reckhardt et al., 2017; Zhou et al., 2023). From our experimental leaching experiment (Figure 2), we observed that DOM linked to poorly crystalline iron (extracted using 0.5 M HCl) showed higher levels of aromatic, oxygen-rich, and highly unsaturated compounds compared to porewater, along with some saturated labile compounds (Figure 6). The enrichment of aromatic, oxygen-rich, and highly unsaturated compounds was most pronounced in deep anoxic zones (Figure 6), consistent with previous iron oxyhydroxide adsorption studies (Lv et al., 2016; Linkhorst et al., 2017; Zhou et al., 2024). Furthermore, high leachable DOC concentrations in HCl extracts at ML1 and ML3 corresponded with elevated leachable Fe concentrations, Fe/DOC ratios, and finer grain sizes. Conversely, coarser sediments with lower Fe concentrations exhibited reduced leachable DOC in HCl extracts (Figure 4 and Supplementary Table 1). This pattern might be attributed to finer, clay-rich sediments enhancing organic matter retention. Iron, often associated with clay-rich sediments (Ilgen et al., 2019), likely contributes to organic matter preservation through various mechanisms. For instance, clay minerals can restrict oxygen penetration, creating anoxic microenvironments that slow down organic matter decomposition (Hulthe et al., 1998). Additionally, clay minerals provide extensive surface areas for the adsorption of organic molecules, increasing the sediment's organic matter retention capacity (Mayer, 1994a,b; Hulthe et al., 1998; Kaiser and Zech, 2000). The layered structure of clay minerals can also physically protect organic matter from microbial degradation (Mayer, 1994b). The lower degradation states of HCl leachates compared to the ambient porewater DOM support the protective effects of fine sediments against microbial degradation or DOM oxidation (Figure 5).
Additionally, during reoxygenation events triggered by porewater advection from oxic zones, dissolved Fe2+ can be reoxidized and coprecipitate with DOM. The resulting Fe3+ oxyhydroxides, with their large surface area, can further adsorb DOM (Chen et al., 2014; Zhou et al., 2018). Under reducing conditions, DOM bound to Fe3+-oxyhydroxides may be remobilized through microbial Fe reduction and release (Hu et al., 2023). We observed higher DOC and Fe2+ concentrations in anoxic porewaters at ML1 and ML2 (18–24 m) and ML3 (6–24 m) compared to oxic zones (Figure 3). At our study site, oxygen and nitrate penetrate deep into the sediments (Massmann et al., 2023). We hypothesize that frequent O2 intrusion into deeper sediments, driven by porewater advection, may restrict the release of Fe2+ from sediments into porewater, thereby limiting the release of Fe3+-bound DOM.
In line with Zhou et al. (2024), we propose that poorly crystalline iron phases in deep beach sediments function as intermediate storage for terrestrial organic carbon in the deep STE. The quantity of DOM associated with poorly crystalline Fe phases was ~160 times greater than the ambient porewater DOM (Supplementary Table 1), indicating their significance as an intermediate reservoir for terrestrial DOM. Moreover, the loosely bound-labile DOM fraction of the sediments was about 130 times higher than the ambient porewater DOM, suggesting that advective flow in the deep STE may readily mobilize this DOM fraction. We also propose that Fe oxides/hydroxides in clay-rich minerals serve as key adsorption sites for terrigenous organic matter within deep STE sediments, facilitating long-term preservation through organo-mineral associations. In contrast, the labile DOM fraction—shown by Abarike et al. (2024) to rapidly stimulate microbial activity upon release—is readily mobilized by advective flow, creating a dynamic balance between carbon retention and transport. This dual mechanism establishes the STE as a biogeochemical reactor where: (1) mineral-bound DOM accumulates as a refractory reservoir, and (2) labile fractions either fuel subsurface microbial communities or are exported to coastal waters. The predominance of fine-grained, Fe-rich sediments enhances this selective preservation, while porewater flow governs the mobilization and fate of reactive DOM fractions.
4.3 Experimental Fe-DOM interactions in the aqueous phase
In contrast to the findings of Linkhorst et al. (2017), who reported locally distinct reductions in DOC following Fe-DOM coprecipitation in shallow STEs (<5 m), our experimental study—conducted at the same site but focusing on the deep subsurface (>5 m)—yielded less consistent results. Anoxic porewater samples (18–24 m at ML1/ML2, 6–24 m at ML3; Figures 3) collected during three sampling periods showed little DOC changes after aeration within the precision range of the TOC analyzer (≤ 10%; Figures 7a–c). This suggests that Fe-DOM coprecipitation in the aqueous phase has a much lower impact on organic carbon mobility in deeper STEs compared to shallower depths. Reported dissolved iron and DOC concentrations in the upper meters of the porewater exfiltration zone at Spiekeroog beach were much higher (Reckhardt et al., 2015, 2017; Beck et al., 2017) than what we found in the deep STE porewaters, enhancing the Fe-DOM coprecipitation potential in the upper STE. In our study, there was no clear relationship between abundance of iron, DOC or iron-DOC molar ratios and observed removal of DOC. However, a neutral to alkaline pH of the porewater, together with Fe/DOC ratios of <0.5, indicate that the Fe-DOM coprecipitation potential could be overall low in this environment (Supplementary Table 2, Nierop et al., 2002; Du et al., 2018).
Despite the low Fe/DOC ratios and low amounts of removed DOC, some molecular changes were observed after coprecipitation. FDOM values decreased up to nearly 50% after coagulation, especially at ML3 (Figures 7d–f). The FDOM measured here is indicative of humic-like terrestrial and aquatic DOM. In previous studies, its abundance was significantly positively correlated with porewater dissolved iron concentrations, leading to the suggestion that reductive dissolution of iron oxides may lead to FDOM release into STE porewaters (Waska et al., 2019; Sirois et al., 2018). Our study results imply that the reverse reaction, e.g., immobilization of Fe and FDOM, can take place at STE redox interfaces as well. Still, not all FDOM was removed during coagulation. Within the defined excitation/emission spectra, FDOM fractions still encompass a diverse range of compounds, which may not all bind to oxidized iron. In line with FDOM reduction, throughout the STE, oxygen-rich aromatic and highly unsaturated compounds were removed as well (Figure 8). Spatially, ML1 and ML2 exhibited substantial removal of aromatic and unsaturated DOM fractions, whereas ML3 showed a more variable response (Figure 8; Tables 2–4). Although the observed fractionations in part supported the findings of Riedel et al. (2013) and Linkhorst et al. (2017), they too varied substantially across different seasons and stations.
While research on the composition, persistence, and inventory of sediment-bound organic matter both from the marine and terrestrial realm gained considerable traction in recent years (e.g., Zhao et al., 2018; Zhou et al., 2024), Fe-DOM coprecipitation experiments to date are fewer and still mainly employ terrestrial natural organic matter (Du et al., 2018). One comparative study found that marine (estuarine) DOM precipitating with ferric hydroxide was more saturated (higher H/C) than precipitates from terrestrially derived SRNOM (Suwannee River Natural Organic Matter) reference material (Li et al., 2019). For a shallow brackish hydrothermal vent system with high Fe2+ fluxes, Gomez-Saez et al. (2015) conducted precipitation experiments similar to ours. They found that while DOC and DOM from the fluids and adjacent seawater did not reveal strong fractionation patterns, the organic matter re-released from Fe-DOM precipitates was distinctly different and had higher O/C ratios than compounds which remained in the fluid. Compared to terrestrial DOM, marine DOM has smaller molecular sizes and overall different moieties involved in Fe complexation, and Fe-DOM binding could lead to an increase in Fe solubility, instead of Fe-DOM coprecipitation. For example, Fe-binding ligands in marine DOM were likened to fulvic acids in their acid-base properties (Lodeiro et al., 2020), and fulvic acids are characterized by higher solubility and lower Fe-coagulation potential than humic acids, which are common in terrestrial aquatic systems (Du et al., 2018).
Recent experimental work revealed that the amount of carboxyl groups which occur ubiquitously in natural DOM, can increase the affinity of a molecule to precipitate with ferrihydrite (Curti et al., 2021). We cannot determine structural entities like carboxyl groups from FT-ICR-MS-derived molecular formulae, but O/C ratios, as well as the number of carboxyl groups, are predicted to increase in the DOM pool with increasing degradation (Lechtenfeld et al., 2014). Therefore, one could speculate that (i) increasing O/C ratios as a function of DOM aging might increase the intensity of Fe-DOM coprecipitation of natural organic matter, (ii) mineral-bound organic matter contains elevated O/C ratios compared to ambient DOM, and that (iii) O/C ratios should decrease after Fe-DOM coprecipitation due to the removal of carboxyl-rich compounds. However, in our study, pore- and seawaters, rather than HCl sediment leachate samples, had the highest O/C ratios amongst all investigated sample types (Table 1), and although O/C ratios decreased slightly in porewater after Fe-DOM coprecipitation, this trend was not persistent (Tables 2–4). All in all, our and previous research indicate that the factors found to govern Fe-DOM coprecipitation in the terrestrial environment may not be entirely transferrable to marine systems, both due to intrinsic DOM properties and environmental conditions.
4.4 Spatiotemporal dynamics of DOM retention in the aqueous phase
From our experimental study, we found a remarkable change in FDOM distribution patterns across different months (September, October and December 2022) and a strong decrease in FDOM values after Fe-DOM coprecipitation (Figures 7a–c). Since we only observed high FDOM values in one of the three campaigns, we cannot know whether it was a unique occurrence or may happen more often during the year. The September 2022 campaign was the first where all three wells were installed and ready to sample, and ML3 had to this point only been installed for a month, which may have caused a disruption of sediment stratigraphy and flow regime, and perhaps suspension of organic-rich particles. However, environmental parameters like salinity and oxygen, DOC, and dissolved iron concentrations were in the same range as in the October and December campaigns (Figure 3), indicating that the samples extracted from the wells were in equilibrium with the surrounding groundwater. Nevertheless, long-term observations will be necessary to confirm the extraordinary FDOM dynamics found here.
In addition to FDOM, spatio-temporal variations in the molecular changes of DOM following coprecipitation with Fe3+ (Figure 8) were found in all three campaigns. This pattern could be attributed to temporal shifts in DOM signatures across different zones of the deep STE, which may be driven by the intense mixing of fresh groundwater and seawater in high-energy STEs (Greskowiak et al., 2023). For example, in September, samples exhibited the highest removal of oxygenated and highly unsaturated compounds (Figures 8a–c). Conversely, substantial removal of aromatic compounds was observed in October (Figures 8d–f). In December, almost no removal of specific molecular formulae was found after coagulation (Figures 8d–i). These observed differences in DOM fractions removed through Fe-DOM coprecipitation were consistent with overall changes in the molecular composition of the whole DOM geometabolome across the three different campaigns (Figure 8). This suggests that the temporal, transport-driven dynamics of DOM composition influence the Fe-DOM coprecipitation process within the STE. Moreover, these temporal shifts in DOM regimes not only influence the types of organic compounds preferentially removed but also impact the overall quantity of DOM that undergoes Fe-DOM coprecipitation at any given time. The dynamic interplay between changing DOM inputs and iron-mediated coagulation suggests that the STE functions as a temporally variable filter for organic matter transport from land to sea. The efficiency and selectivity of this filtration process are likely to fluctuate in response to temporal and event-driven changes in DOM inputs, potentially leading to variations in both the quantity and quality of organic matter ultimately exported to coastal waters. Ultimately, while environmental parameters (Figure 3) did not vary significantly across the three campaigns, we observed molecular fractionation of DOM composition following aeration, which differed across the studied months (Figure 8). Therefore, we recommend that future studies prioritize investigating the spatio-temporal dynamics of DOM composition in the deep STE to better understand its reactivity with iron under changing redox conditions, typical of high-energy STEs (Greskowiak et al., 2023).
5 Summary and conclusions
Understanding the dynamics of dissolved organic matter (DOM) and its interactions with iron (Fe) in high-energy subterranean estuaries (STEs) is crucial for assessing the mobility and retention of organic carbon in coastal regions. In this study, we analyzed the molecular composition of sedimentary organic matter by sequentially extracting loosely bound organic compounds with ultra-pure water, and poorly crystalline Fe oxides/hydroxides, as well as mineral-bound organic compounds using hydrochloric acid (HCl) down to a depth of 24 meters from an exemplary high-energy beach on Spiekeroog Island in the German North Sea. We also investigated aqueous phase coprecipitation of DOM with Fe3+ (oxy)hydroxides in Fe2+-containing STE porewater along salinity and redox gradients over three sampling campaigns (September, October, and December 2022). We employed fluorescence spectroscopy and ultrahigh-resolution mass spectrometry for our molecular analysis of DOM.
Our experimental results showed that DOM from seawater and STE porewater is more complex and oxidized than sediment-leached DOM. Water-leachable (loosely bound DOM) fractions were enriched in aliphatic, oxygen-rich, and presumably labile compounds of marine or autochthonous origin. The reduced, saturated nature of this DOM compared to the ambient porewater DOM suggested that its distribution is driven by microbial transformation of in situ sedimentary organic matter rather than retention from porewater. In contrast, the 0.5 M HCl leachates (mineral-bound DOM) fraction was more molecularly diverse, included oxidized aromatic compounds, and had a stronger terrigenous signature. However, these leachates also contained a notable fraction of labile, saturated compounds. Sequential leaching highlighted that much of the DOM is mineral-bound, with a relatively smaller fraction loosely bound to the sediments. Higher leachable DOC in the mineral-bound fraction was associated with finer, likely clay-rich sediments and higher Fe concentrations, whereas lower levels coincided with coarser sediments. This suggests that Fe oxides/hydroxides act as key adsorption sites for the retention of terrestrial DOM, while the labile fraction can be easily mobilized by advective flow in the deep STEs. While leachable DOC for both water and acid treatments represented ~10% of total organic carbon (TOC), concentrations of the latter were up to three orders of magnitude higher than porewater DOC, indicating that deep sediments serve as significant organic matter reservoirs.
In the aqueous phase, Fe-DOM coprecipitation experiments showed that Fe3+ selectively binds aromatic, oxygen-rich, and unsaturated compounds. However, DOM removal was within the error margin of the analytical instruments and varied across sampling events. This contrasts with the stronger molecular changes observed at shallower depths or in environments with a stronger terrestrial influence (Linkhorst et al., 2017). Thus, Fe-DOM coprecipitation at redox interfaces in deeper STEs (>5 m) appears to be limited, likely driven by dynamic shifts in porewater geochemistry, low Fe/DOC ratios, and near-neutral pH conditions. Overall, our results suggest that the “iron curtain,” originally coined by Chambers and Odum (1990) to describe the scavenging of organic carbon by oxidized Fe at oxic-anoxic interfaces in coastal environments, could instead be considered an iron zone with extensions spanning tens to hundreds of meters in high-energy STEs. Furthermore, its impact on organic carbon and Fe mobility in the aqueous phase appears to decrease in deeper STEs (>5 m).
We propose that Fe-rich, clay-containing minerals within the sedimentary matrices of permeable sediments serve as long-term storage sites for refractory, terrigenous DOM, while loosely bound, labile DOM fractions—shown by Abarike et al. (2024) to rapidly fuel microbial growth upon release—are readily mobilized by advective flow in the deep STE. These findings highlight the critical role of organo-mineral interactions in regulating the mobility, retention, and fate of organic matter at the land-ocean boundary. In organic-poor, coastal sandy beach environments, this interplay creates a dual system: mineral-stabilized terrestrial DOM persists over timescales relevant to carbon sequestration, whereas labile DOM is either rapidly metabolized by sediment-associated microbes or advected into the aqueous phase. Together, these processes shape the STE's function as a biogeochemical reactor, modulating carbon fluxes across the terrestrial-marine continuum.
Data availability statement
The original contributions presented in the study are included in the article/Supplementary material, further inquiries can be directed to the corresponding author.
Author contributions
KA: Data curation, Formal analysis, Investigation, Methodology, Software, Visualization, Writing – original draft. AR: Methodology, Writing – review editing; Visualization, Investigation, Data curation. MR: Methodology, Writing – review & editing. RM: Methodology, Writing – review & editing. SB: Methodology, Writing – review & editing, Data curation, Formal analysis. TD: Conceptualization, Funding acquisition, Project administration, Supervision, Validation, Writing – review & editing. HW: Conceptualization, Funding acquisition, Resources, Supervision, Validation, Visualization, Writing – original draft, Writing – review & editing.
Funding
The author(s) declare that financial support was received for the research and/or publication of this article. This study was funded by the Deutsche Forschungsgemeinschaft (DFG) through the research unit Dynamic Deep Subsurface of High-Energy Beaches (DynaDeep, FOR5094).
Acknowledgments
We express our sincere gratitude to the National Park Authorities for granting permits to collect porewater and deep core sediments from Spiekeroog Beach. We are also thankful for the technical support provided by Kai Schwalfenberg and Dietmar Pommerin during sampling. Our appreciation goes to Grace Awinmalsim Abarike, Iris Valeria Medina Cordova, Pia Eckert, and Fenno Firnhaber for their invaluable assistance in field sampling. We acknowledge the contributions of Ina Ulber, Matthias Friebe, Katrin Klaproth, Martina Schulz, and Heike Simon for their technical support throughout the experiments. Finally, we are grateful to Prof. Timothy Shaw and Dr. Jutta Niggemann for their constructive feedback on the manuscript.
Conflict of interest
The authors declare that the research was conducted in the absence of any commercial or financial relationships that could be construed as a potential conflict of interest.
Generative AI statement
The author(s) declare that no Gen AI was used in the creation of this manuscript.
Publisher's note
All claims expressed in this article are solely those of the authors and do not necessarily represent those of their affiliated organizations, or those of the publisher, the editors and the reviewers. Any product that may be evaluated in this article, or claim that may be made by its manufacturer, is not guaranteed or endorsed by the publisher.
Supplementary material
The Supplementary Material for this article can be found online at: https://www.frontiersin.org/articles/10.3389/frwa.2025.1507564/full#supplementary-material
References
Abarike, G. A., Brick, S., Engelen, B., and Niggemann, J. (2024). Different dissolved organic matter sources sustain microbial life in a sandy beach subterranean estuary - an incubation study. Front. Mar. Sci. 11:1501781. doi: 10.3389/fmars.2024.1501781
Adyasari, D., Dimova, N., Waska, H., and Chadhain, S. N. (2023). Dissolved organic matter and nutrient processing in organic-rich subterranean estuaries: implications for future land use and climate scenarios. Geochim. Cosmochim. Acta 362, 65–76. doi: 10.1016/j.gca.2023.10.025
Ahmad, A., and Martsinovich, N. (2023). Atomic-scale modelling of organic matter in soil: adsorption of organic molecules and biopolymers on the hydroxylated α-Al2O3 (0001) surface. Philos. Trans. R. Soc. A 381:20220254. doi: 10.1098/rsta.2022.0254
Ahrens, J., Beck, M., Marchant, H. K., Ahmerkamp, S., Schnetger, B., and Brumsack, H. J. (2020). Seasonality of organic matter degradation regulates nutrient and metal net fluxes in a high energy sandy beach. J. Geophys. Res. Biogeosci. 125:e2019JG005399. doi: 10.1029/2019JG005399
Anschutz, P., Smith, T., Mouret, A., Deborde, J., Bujan, S., Poirier, D., et al. (2009). Tidal sands as biogeochemical reactors. Estuarine Coastal Shelf Sci. 84, 84–90. doi: 10.1016/j.ecss.2009.06.015
Audry, S., Pokrovsky, O. S., Shirokova, L. S., Kirpotin, S. N., and Dupré, B. (2011). Organic matter mineralization and trace element post-depositional redistribution in Western Siberia thermokarst lake sediments. Biogeosciences. 8, 3341–3358. doi: 10.5194/bg-8-3341-2011
Auer, F., Ahmerkamp, S., Cueto, J., Winter, C., and Holtappels, M. (2025). Extensive oxygen consumption in the intertidal infiltration zone of beach aquifers—the impact of seasonal input, filtration efficiency, and morphodynamics. J. Geophys. Res. Biogeosci. 130:e2024JG008291. doi: 10.1029/2024JG008291
Bao, T., Wang, P., Hu, B., Wang, X., and Qian, J. (2023). Mobilization of colloids during sediment resuspension and its effect on the release of heavy metals and dissolved organic matter. Sci. Total Environ. 861:160678. doi: 10.1016/j.scitotenv.2022.160678
Beck, M., Reckhardt, A., Amelsberg, J., Bartholomä, A., Brumsack, H. J., Cypionka, H., et al. (2017). The drivers of biogeochemistry in beach ecosystems: a cross-shore transect from the dunes to the low-water line. Marine Chem. 190, 35–50. doi: 10.1016/j.marchem.2017.01.001
Brumsack, H. J., and Gieskes, J. M. (1983). Interstitial water trace-metal chemistry of laminated sediments from the Gulf of California, Mexico. Marine Chem. 14, 89–106. doi: 10.1016/0304-4203(83)90072-5
Carvalho da Silva, R., Seidel, M., Dittmar, T., and Waska, H. (2023). Groundwater springs in the German Wadden Sea tidal flat: a fast-track terrestrial transfer route for nutrients and dissolved organic matter. Front. Marine Sci. 10:1128855. doi: 10.3389/fmars.2023.1128855
Chambers, R. M., and Odum, W. E. (1990). Porewater oxidation, dissolved phosphate, and the iron curtain: iron-phosphorus relations in tidal freshwater marshes. Biogeochemistry. 10, 37–52. doi: 10.1007/BF00000891
Charette, M. A., and Sholkovitz, E. R. (2002). Oxidative precipitation of groundwater-derived ferrous iron in the subterranean estuary of a coastal bay. Geophys. Res. Lett. 29, 1–4. doi: 10.1029/2001GL014512
Chen, C. M., Dynes, J. J., Wang, J., and Sparks, D. L. (2014). Properties of Fe-organic matter associations via coprecipitation versus adsorption. Environ. Sci. Technol. 48, 13751–13759. doi: 10.1021/es503669u
Cho, H. M., Kim, G. Y., Kwon, E. Y., Moosdorf, N., Garcia-Orellana, J., and Santos, I. R. (2018). Radium tracing nutrient inputs through submarine groundwater discharge in the global ocean. Sci. Rep. 8:2439. doi: 10.1038/s41598-018-20806-2
Christensen, B. T. (2001). Physical fractionation of soil and structural and functional complexity in organic matter turnover. Euro. J. Soil Sci. 52, 345–353. doi: 10.1046/j.1365-2389.2001.00417.x
Curti, L., Moore, O. W., Babakhani, P., Xiao, K. Q., Woulds, C., Bray, A. W., et al. (2021). Carboxyl-richness controls organic carbon preservation during coprecipitation with iron (oxyhydr)oxides in the natural environment. Commun. Earth Environ. 2:229. doi: 10.1038/s43247-021-00301-9
CWSS (Common Wadden Sea Secretariat Wilhelmshaven, Germany) and WHNPG (World Heritage Nomination Project Group).. (2008). Nomination of the DutchGerman Wadden Sea as World Heritage Site. Kirchhatten: Plakativ Grafische Medien GmbH.
Cyle, K. T., Hill, N., Young, K., Jenkins, T., Hancock, D., Schroeder, P. A., et al. (2016). Substrate quality influences organic matter accumulation in the soil silt and clay fraction. Soil Biol. Biochem. 103, 138–148. doi: 10.1016/j.soilbio.2016.08.014
D'Andrilli, J., Cooper, W. T., Foreman, C. M., and Marshall, A. G. (2015). An ultrahigh-resolution mass spectrometry index to estimate natural organic matter lability. Rapid Commun. Mass Spectrom. 29, 2385–2401. doi: 10.1002/rcm.7400
Deng, Y., and Dixon, J. B. (2002). “Soil organic matter and organic-mineral interactions,” in Soil Mineralogy with Environmental Applications, eds. J. B. Dixon and D. G. Schulze (Madison, WI: Soil Science Society of America), 69–107. doi: 10.2136/sssabookser7.c3
Dittmar, T., Koch, B., Hertkorn, N., and Kattner, G. (2008). A simple and efficient method for the solid-phase extraction of dissolved organic matter (SPE-DOM) from seawater. Limnol. Oceanogr. Methods 6, 230–235. doi: 10.4319/lom.2008.6.230
Dittmar, T., and Stubbins, A. (2014). “Dissolved organic matter in aquatic systems,” in Treatise on Geochemistry, 2nd Edn., eds. K. K. Turekian and H. D. Holland (Oxford: Elsevier), 125–156. doi: 10.1016/B978-0-08-095975-7.01010-X
Du, Y., Ramirez, C. E., and Jaffé, R. (2018). Fractionation of dissolved organic matter by co-precipitation with iron: effects of composition. Environ. Process 5, 5–21. doi: 10.1007/s40710-017-0281-4
Flerus, R., Lechtenfeld, O. J., Koch, B. P., McCallister, S. L., Schmitt-Kopplin, P., Benner, R., et al. (2012). A molecular perspective on the ageing of marine dissolved organic matter. Biogeosciences 9, 1935–1955. doi: 10.5194/bg-9-1935-2012
Foster, I. D. L., Albon, A. J., Bardell, K. M., Fletcher, J. L., Jardine, T. C., Pritchard, M. A., et al. (1991). High energy coastal sedimentary deposits; an evaluation of depositional processes in southwest England. Earth Surface Processes Landforms 16, 341–356. doi: 10.1002/esp.3290160407
Gomez-Saez, G. V., Riedel, T., Niggemann, J., Pichler, T., Dittmar, T., and Bühring, S. I. (2015). Interaction between iron and dissolved organic matter in a marine shallow hydrothermal system off Dominica Island (Lesser Antilles). Marine Chem. 177, 677–686. doi: 10.1016/j.marchem.2015.10.003
Green, N. W., Perdue, E. M., Aiken, G. R., Butler, K. D., Chen, H., Dittmar, T., et al. (2014). An intercomparison of three methods for the large-scale isolation of oceanic dissolved organic matter. Marine Chem. 161, 14–19. doi: 10.1016/j.marchem.2014.01.012
Greskowiak, J., Seibert, S. L., Post, V. E., and Massmann, G. (2023). Redox-zoning in high-energy subterranean estuaries as a function of storm floods, temperatures, seasonal groundwater recharge and morphodynamics. Estuarine Coastal Shelf Sci. 290:108418. doi: 10.1016/j.ecss.2023.108418
Groeneveld, M., Catalán, N., Attermeyer, K., Hawkes, J., Einarsdóttir, K., Kothawala, D., et al. (2020). Selective adsorption of terrestrial dissolved organic matter to inorganic surfaces along a boreal inland water continuum. J. Geophys. Res. Biogeosci. 125:e2019JG005236. doi: 10.1029/2019JG005236
Grünenbaum, N., Greskowiak, J., Sültenfuß, J., and Massmann, G. (2020). Groundwater flow and residence times below a meso-tidal high-energy beach: a model-based analysis of salinity patterns and 3H-3He groundwater ages. J. Hydrol. 587:124948. doi: 10.1016/j.jhydrol.2020.124948
Herrling, G., and Winter, C. (2015). Tidally- and wind-driven residual circulation at the multiple-inlet system East Frisian Wadden Sea. Continent. Shelf Res. 106, 45–59. doi: 10.1016/j.csr.2015.06.001
Hu, L., Ji, Y., Zhao, B., Liu, X., Du, J., Liang, Y., et al. (2023). The effect of iron on the preservation of organic carbon in marine sediments and its implications for carbon sequestration. Sci. China Earth Sci. 66, 1946–1959. doi: 10.1007/s11430-023-1139-9
Hulthe, G., Hulth, S., and Hall, P. O. (1998). Effect of oxygen on degradation rate of refractory and labile organic matter in continental margin sediments. Geochim. Cosmochim. Acta 62, 1319–1328. doi: 10.1016/S0016-7037(98)00044-1
Hyacinthe, C., Bonneville, S., and Van Cappellen, P. (2006). Reactive iron (III) in sediments: chemical versus microbial extractions. Geochim. Cosmochim. Acta 70, 4166–4180. doi: 10.1016/j.gca.2006.05.018
Ilgen, A. G., Kukkadapu, R. K., Leung, K., and Washington, R. E. (2019). “Switching on” iron in clay minerals. Environ. Sci. Nano. 6, 1704–1715. doi: 10.1039/C9EN00228F
Islam, M. R., Singh, B., and Dijkstra, F. A. (2022). Stabilisation of soil organic matter: interactions between clay and microbes. Biogeochemistry 160, 145–158. doi: 10.1007/s10533-022-00956-2
Kaiser, K., and Zech, W. (2000). Dissolved organic matter sorption by mineral constituents of subsoil clay fractions. J. Plant Nutr. Soil Sci. 163, 531–535. doi: 10.1002/1522-2624(200010)163:5<531::AID-JPLN531>3.0.CO;2-N
Koch, B. P., and Dittmar, T. (2006). From mass to structure: an aromaticity index for high-resolution mass data of natural organic matter. Rapid Commun. Mass Spectrom. 20, 926–932. doi: 10.1002/rcm.2386
Kögel-Knabner, I., Guggenberger, G., Kleber, M., Kandeler, E., Kalbitz, K., Scheu, S., et al. (2008). Organo-mineral associations in temperate soils: integrating biology, mineralogy, and organic matter chemistry. J. Plant Nutr. Soil Sci. 171, 61–82. doi: 10.1002/jpln.200700048
Lalonde, K., Mucci, A., Ouellet, A., and Gélinas, Y. (2012). Preservation of organic matter in sediments promoted by iron. Nature 483, 198–200. doi: 10.1038/nature10855
Lechtenfeld, O. J., Kattner, G., Flerus, R., McCallister, S. L., Schmitt-Kopplin, P., and Koch, B. P. (2014). Molecular transformation and degradation of refractory dissolved organic matter in the Atlantic and Southern Ocean. Geochim. Cosmochim. Acta 126, 321–337. doi: 10.1016/j.gca.2013.11.009
Li, L., Fang, Z., He, C., and Shi, Q. (2019). Separation and characterization of marine dissolved organic matter (DOM) by combination of Fe(OH)3 co-precipitation and solid-phase extraction followed by ESI FT-ICR MS. Anal. Bioanal. Chem. 411, 2201–2208. doi: 10.1007/s00216-019-01663-y
Linkhorst, A., Dittmar, T., and Waska, H. (2017). Molecular fractionation of dissolved organic matter in a shallow subterranean estuary: the role of the iron curtain. Environ. Sci. Technol. 51, 1312–1320. doi: 10.1021/acs.est.6b03608
Lodeiro, P., Rey-Castro, C., David, C., Achterberg, E. P., Puy, J., and Gledhill, M. (2020). Acid-base properties of dissolved organic matter extracted from the marine environment. Sci. Total Environ. 729:138437. doi: 10.1016/j.scitotenv.2020.138437
Luijendijk, E., Gleeson, T., and Moosdorf, N. (2020). Fresh groundwater discharge insignificant for the world's oceans but important for coastal ecosystems. Nat. Commun. 11:1260. doi: 10.1038/s41467-020-15064-8
Lv, J., Zhang, S., Wang, S., Luo, L., Cao, D., and Christie, P. (2016). Molecular-scale investigation with ESI-FT-ICR-MS on fractionation of dissolved organic matter induced by adsorption on iron oxyhydroxides. Environ. Sci. Technol. 50, 2328–2336. doi: 10.1021/acs.est.5b04996
Massmann, G., Abarike, G., Amoako, K., Auer, F., Badewien, T. H., Berkenbrink, C., et al. (2023). The DynaDeep observatory–a unique approach to study high-energy subterranean estuaries. Front. Marine Sci. 10:1189281. doi: 10.3389/fmars.2023.1189281
Mayer, L. M. (1994a). Relationships between mineral surfaces and organic carbon concentrations in soils and sediments. Chem. Geol. 114, 347–363. doi: 10.1016/0009-2541(94)90063-9
Mayer, L. M. (1994b). Surface area control of organic carbon accumulation in continental shelf sediments. Geochim. Cosmochim. Acta 58, 1271–1284. doi: 10.1016/0016-7037(94)90381-6
McAllister, S. M., Barnett, J. M., Heiss, J. W., Findlay, A. J., MacDonald, D. J., Dow, C. L., et al. (2015). Dynamic hydrologic and biogeochemical processes drive microbially enhanced iron and sulfur cycling within the intertidal mixing zone of a beach aquifer. Limnol. Oceanogr. 60, 329–345. doi: 10.1002/lno.10029
McDonough, L. K., Andersen, M. S., Behnke, M. I., Rutlidge, H., Oudone, P., Meredith, K., et al. (2022). A new conceptual framework for the transformation of groundwater dissolved organic matter. Nat. Commun. 13:2153. doi: 10.1038/s41467-022-29711-9
Medeiros, P. M., Seidel, M., Niggemann, J., Spencer, R. G., Hernes, P. J., Yager, P. L., et al. (2016). A novel molecular approach for tracing terrigenous dissolved organic matter into the deep ocean. Glob. Biogeochem. Cycl. 30, 689–699. doi: 10.1002/2015GB005320
Merder, J., Freund, J. A., Feudel, U., Hansen, C. T., Hawkes, J. A., Jacob, B., et al. (2020). ICBM-OCEAN: processing ultrahigh-resolution mass spectrometry data of complex molecular mixtures. Anal. Chem. 92, 6832–6838. doi: 10.1021/acs.analchem.9b05659
Moore, W. S. (1999). The subterranean estuary: a reaction zone of ground water and sea water. Marine Chem. 65, 111–125. doi: 10.1016/S0304-4203(99)00014-6
Nierop, K. G., Jansen, B., and Verstraten, J. M. (2002). Dissolved organic matter, aluminum and iron interactions: precipitation induced by metal/carbon ratio, pH and competition. Sci. Total Environ. 300, 201–211. doi: 10.1016/S0048-9697(02)00254-1
O'Connor, A. E., Krask, J. L., Canuel, E. A., and Beck, A. J. (2018). Seasonality of major redox constituents in a shallow subterranean estuary. Geochim. Cosmochim. Acta 224, 344–361. doi: 10.1016/j.gca.2017.10.013
Reckhardt, A., Beck, M., Greskowiak, J., Schnetger, B., Böttcher, M. E., Gehre, M., et al. (2017). Cycling of redox-sensitive elements in a sandy subterranean estuary of the southern North Sea. Marine Chem. 188, 6–17. doi: 10.1016/j.marchem.2016.11.003
Reckhardt, A., Beck, M., Seidel, M., Riedel, T., Wehrmann, A., Bartholomä, A., et al. (2015). Carbon, nutrient and trace metal cycling in sandy sediments: a comparison of high-energy beaches and backbarrier tidal flats. Estuarine Coastal Shelf Sci. 159, 1–14. doi: 10.1016/j.ecss.2015.03.025
Reckhardt, A., Meyer, R., Seibert, S. L., Greskowiak, J., Roberts, M., Brick, S., et al. (2024). Spatial and temporal dynamics of groundwater biogeochemistry in the deep subsurface of a high-energy beach. Marine Chem. 267:104461. doi: 10.1016/j.marchem.2024.104461
Reuter, R., Badewien, T. H., Bartholomä, A., Braun, A., Lübben, A., and Rullkötter, J. (2009). A hydrographic time series station in the Wadden Sea (southern North Sea). Ocean Dyn. 59, 195–211. doi: 10.1007/s10236-009-0196-3
Riedel, T., Zak, D., Biester, H., and Dittmar, T. (2013). Iron traps terrestrially derived dissolved organic matter at redox interfaces. Proc. Natl. Acad. Sci. U.S.A. 110, 10101–10105. doi: 10.1073/pnas.1221487110
Röper, T., Greskowiak, J., Freund, H., and Massmann, G. (2013). Freshwater lens formation below juvenile dunes on a barrier island (Spiekeroog, Northwest Germany). Estuar. Coast. Shelf Sci. 121, 40–50. doi: 10.1016/j.ecss.2013.02.004
Röper, T., Greskowiak, J., and Massmann, G. (2014). Detecting small groundwater discharge springs using handheld thermal infrared imagery. Groundwater 52, 936–942. doi: 10.1111/gwat.12145
Röper, T., Kröger, K. F., Meyer, H., Sültenfuss, J., Greskowiak, J., and Massmann, G. (2012). Groundwater ages, recharge conditions and hydrochemical evolution of a barrier island freshwater lens (Spiekeroog, Northern Germany). J. Hydrol. 454–455, 173–186. doi: 10.1016/j.jhydrol.2012.06.011
Roy, M., Martin, J. B., Smith, C. G., and Cable, J. E. (2011). Reactive-transport modeling of iron diagenesis and associated organic carbon remineralization in a Florida (USA) subterranean estuary. Earth Planet. Sci. Lett. 304, 191–201. doi: 10.1016/j.epsl.2011.02.002
Seibert, S. L., Greskowiak, J., Prommer, H., Böttcher, M. E., and Massmann, G. (2019). Modeling of biogeochemical processes in a barrier island freshwater lens (Spiekeroog, Germany). J. Hydrol. 575, 1133–1144. doi: 10.1016/j.jhydrol.2019.05.094
Seibert, S. L., Holt, T., Reckhardt, A., Ahrens, J., Beck, M., Pollmann, T., et al. (2018). Hydrochemical evolution of a freshwater lens below a barrier island (Spiekeroog, Germany): the role of carbonate mineral reactions, cation exchange and redox processes. Appl. Geochem. 92, 196–208. doi: 10.1016/j.apgeochem.2018.03.001
Seidel, M., Beck, M., Greskowiak, J., Riedel, T., Waska, H., Suryaputra, I. N., et al. (2015). Benthic-pelagic coupling of nutrients and dissolved organic matter composition in an intertidal sandy beach. Marine Chem. 176, 150–163. doi: 10.1016/j.marchem.2015.08.011
Sirois, M., Couturier, M., Barber, A., Gélinas, Y., and Chaillou, G. (2018). Interactions between iron and organic carbon in a sandy beach subterranean estuary. Marine Chem. 202, 86–96. doi: 10.1016/j.marchem.2018.02.004
Slomp, C. P., and Van Cappellen, P. (2004). Nutrient inputs to the coastal ocean through submarine groundwater discharge: controls and potential impact. J. Hydrol. 295, 64–86. doi: 10.1016/j.jhydrol.2004.02.018
Stenson, A. C., Marshall, A. G., and Cooper, W. T. (2003). Exact masses and chemical formulas of individual Suwannee River fulvic acids from ultrahigh resolution electrospray ionization Fourier transform ion cyclotron resonance mass spectra. Anal. Chem. 75, 1275–1284. doi: 10.1021/ac026106p
Sutherland, R. A. (2002). Comparison between non-residual Al, Co, Cu, Fe, Mn, Ni, Pb, and Zn released by a three-step sequential extraction procedure and a dilute hydrochloric acid leach for soil and road deposited sediment. Appl. Geochem. 17, 353–365. doi: 10.1016/S0883-2927(01)00095-6
Viollier, E., Inglett, P. W., Hunter, K., Roychoudhury, A. N., and Van Cappellen, P. (2000). The ferrozine method revisited: Fe (II)/Fe (III) determination in natural waters. Appl. Geochem. 15, 785–790. doi: 10.1016/S0883-2927(99)00097-9
Volkman, J. K., Rohjans, D., Rullkötter, J., Scholz-Böttcher, B. M., and Liebezeit, G. (2000). Sources and diagenesis of organic matter in tidal flat sediments from the German Wadden Sea. Cont. Shelf Res. 20, 1139–1158. doi: 10.1016/S0278-4343(00)00016-9
Wakeham, S. G., and Lee, C. (1989). Organic geochemistry of particulate matter in the ocean: the role of particles in oceanic sedimentary cycles. Org. Geochem. 14, 83–96. doi: 10.1016/0146-6380(89)90022-3
Waska, H., Greskowiak, J., Ahrens, J., Beck, M., Ahmerkamp, S., Böning, P., et al. (2019). Spatial and temporal patterns of pore water chemistry in the intertidal zone of a high-energy beach. Front. Mar. Sci. 6:154. doi: 10.3389/fmars.2019.00154
Waska, H., Koschinsky, A., Chancho, M. J. R., and Dittmar, T. (2015). Investigating the potential of solid-phase extraction and Fourier-transform ion cyclotron resonance mass spectrometry (FT-ICR-MS) for the isolation and identification of dissolved metal–organic complexes from natural waters. Marine Chem. 173, 78–92. doi: 10.1016/j.marchem.2014.10.001
Waska, H., Simon, H., Ahmerkamp, S., Greskowiak, J., Ahrens, J., Seibert, S. L., et al. (2021). Molecular traits of dissolved organic matter in the subterranean estuary of a high-energy beach: indications of sources and sinks. Front. Marine Sci. 8:607083. doi: 10.3389/fmars.2021.607083
Xu, X., Shi, R., Lv, C., Liu, H., Yang, W., Qian, S., et al. (2021). Hydrodynamic-driven changes in the source and composition of sedimentary organic matter via grain size distribution in shallow lakes. J. Geophys. Res. Biogeosci. 126:e2021JG006502. doi: 10.1029/2021JG006502
Zhao, B., Yao, P., Bianchi, T. S., Shields, M. R., Cui, X. Q., Zhang, X. W., et al. (2018). The role of reactive iron in the preservation of terrestrial organic carbon in estuarine sediments. J. Geophys. Res. Biogeosci. 123, 3556–3569. doi: 10.1029/2018JG004649
Zhou, Z., Henkel, S., Kasten, S., and Holtappels, M. (2023). The iron “redox battery” in sandy sediments: its impact on organic matter remineralization and phosphorus cycling. Sci. Total Environ. 865:161168. doi: 10.1016/j.scitotenv.2022.161168
Zhou, Z., Latta, D. E., Noor, N., Thompson, A., Borch, T., and Scherer, M. M. (2018). Fe(II)-catalyzed transformation of organic matter–ferrihydrite coprecipitates: a closer look using Fe isotopes. Environ. Sci. Technol. 52, 11142–11150. doi: 10.1021/acs.est.8b03407
Zhou, Z., Waska, H., Henkel, S., Dittmar, T., Kasten, S., and Holtappels, M. (2024). Iron promotes the retention of terrigenous dissolved organic matter in subtidal permeable sediments. Environ. Sci. Technol. 58, 6204–6214. doi: 10.1021/acs.est.3c09531
Keywords: permeable sediments, reactive iron, redox cycling, land-ocean fluxes, dissolved organic matter, subterranean estuary
Citation: Amoako K, Reckhardt A, Roberts M, Meyer R, Brick S, Dittmar T and Waska H (2025) Organo-mineral interactions modulate organic carbon retention and mobility in a deep subterranean estuary of a high-energy beach. Front. Water 7:1507564. doi: 10.3389/frwa.2025.1507564
Received: 08 October 2024; Accepted: 14 April 2025;
Published: 12 May 2025.
Edited by:
Luca Zaggia, CNR-IGG Institute of Geosciences and Earth Resources, ItalyReviewed by:
Gwénaëlle Chaillou, Université du Québec à Rimouski, CanadaJeonghyun Kim, Jeju National University, Republic of Korea
Giuseppe Francesco Cesare Lama, University of Naples Federico II, Italy
Copyright © 2025 Amoako, Reckhardt, Roberts, Meyer, Brick, Dittmar and Waska. This is an open-access article distributed under the terms of the Creative Commons Attribution License (CC BY). The use, distribution or reproduction in other forums is permitted, provided the original author(s) and the copyright owner(s) are credited and that the original publication in this journal is cited, in accordance with accepted academic practice. No use, distribution or reproduction is permitted which does not comply with these terms.
*Correspondence: Kojo Amoako, a29qby5hbW9ha29AdW5pLW9sZGVuYnVyZy5kZQ==