- 1Faculty of Veterinary Medicine, International Excellence Campus for Higher Education and Research “Campus Mare Nostrum”, University of Murcia, Murcia, Spain
- 2Institute for Biomedical Research of Murcia (IMIB-Arrixaca), Campus de Ciencias de la Salud, Murcia, Spain
- 3Department of Clinical and Experimental Medicine (IKE), Linköping University, Linköping, Sweden
- 4Department of Morphology and Function, University of Lisbon, Lisbon, Portugal
Background: Seminal plasma (SP) promotes sperm survival and fertilizing capacity, and potentially affects embryo development, presumably via specific signaling pathways to the internal female genital tract.
Objectives: This study evaluated how heterologous SP, infused immediately before postcervical artificial insemination (AI) affected embryo development and the transcriptional pattern of the pig endometria containing embryos.
Materials and Methods: Postweaning estrus sows (n = 34) received 40-mL intrauterine infusions of either heterologous pooled SP or Beltsville Thawing Solution (BTS; control) 30 min before AI of semen extended to 10% of homologous SP. Embryos (all sows) and endometrium samples (3 sows/group) were removed during laparotomy 6 days after the infusion of SP or BTS to morphologically evaluate the embryos to determine their developmental stage and to analyze the endometrial transcriptome using microarrays (PORGENE 1.0 ST GeneChip array, Affymetrix) followed by qPCR for further validation.
Results: Embryo viability was equal between the groups (~93%), but embryo development was significantly (P < 0.05) more advanced in the SP-treated group compared to control. A total of 1,604 endometrium transcripts were differentially expressed in the SP group compared to the control group. An enrichment analysis showed an overrepresentation of genes and pathways associated with the immune response, cytokine signaling, cell cycle, cell adhesion, and hormone response, among others.
Conclusions: SP infusions prior to AI positively impacted the preimplantation embryo development and altered the expression of the endometrial genes and pathways potentially involved in embryo development.
Introduction
The relevance of keeping a proportion of seminal plasma (SP) in insemination doses to achieve acceptable fertility in the artificial insemination (AI) of pigs was a basic early conclusion of Russian pioneers (1). Since then, studies focused on the role of SP in transporting and protecting spermatozoa have included the design of chemically defined artificial SP [OLEP (2), Predil MR-A (3), etc.]. However, SP actions are wider, influencing the transient inflammation that semen deposition elicits in swine (4–6). Studies in rodents and pigs have suggested SP also impacts fertilization, implantation and pregnancy, modulating the local immunity of the female reproductive tract (7–9). Regulatory components in SP have the potential to alter the expression of active molecules in the female genital tract causing molecular, biochemical and cellular changes in the uterus through particular pathways (10). SP infusion has been recently shown to induce changes in the expression of immune-related genes in the reproductive tract of peri-ovulating pigs, indicating its very early signaling during mating/AI (11). However, we do not know if this signaling affects later events, particularly during the development of embryos preimplantation.
The most substantial evidence regarding the effects of SP on embryonic developmental competence comes from findings in rodents, in which SP infusion supports embryonic development and implantation (12, 13). In the absence of SP, fertilization and embryo development rates decrease, and fetal postimplantation losses increase (14, 15). Moreover, in mice and rats, embryo transfer (ET) protocols generally use recipients exposed to SP during estrus since it is fundamental for proper implantation and embryonic development (16). Despite such evidence, we still lack studies on endometrial molecular changes in response to SP infusions prior to AI and knowledge of how these alterations would influence the progression of porcine preimplantation embryos, a matter of utmost relevance not only for AI but also for other reproductive biotechnologies such as ET.
Therefore, the aims of this study were to examine the effects of heterologous SP infusions prior to AI on (i) changes in the global transcriptome of preimplantation endometrium and (ii) the development and viability of porcine preimplantation embryos.
Materials and Methods
Animals
All experimental procedures were assessed according to the 2010/63/EU EEC Directive for animal experiments and were revised and approved by the Ethical Committee for Experimentation with Animals of the University of Murcia, Spain (research code: 638/2012).
Crossbred sows (Landrace × Large-White), parity 2–7, with a lactation period of 21–24 days, were randomly selected at weaning and used for this experiment. The females were allocated into individual crates in a mechanically ventilated confinement facility at a commercial farm (Agropor SA, Murcia, Spain). The semen donors were sexually mature boars (2–3 years of age) of proven fertility, housed in climate-controlled individual pens (20–25°C) at a commercial breeding boar station for AI-dose semen production in Murcia (AIM Iberica, Spain). The animals had access to water ad libitum and were fed commercial diets according to their nutritional requirements.
Experimental Design
To evaluate the effects of additional heterologous SP-infusions on the endometrial transcriptome and embryo development, a total of 34 postweaning estrus sows received 40-mL intrauterine infusions of either heterologous, pooled SP (N = 16) or Beltsville Thawing Solution [BTS; (17)] (control group; N = 18) 30 min before each AI. At day 6 of the cycle (Day 0 = onset of estrus), sows were subjected to laparotomies to immediately evaluate the effect of SP infusions on embryonic development stage and embryo quality. Additionally, uterine samples from 3 randomly selected embryo-bearing sows were collected for histological and transcriptomic analysis.
Samples were obtained from two different locations of a uterine horn, distal (ad-cervical, i.e., next to the uterine body) and proximal (ad-ovarian, i.e., next to the utero-tubal junction) and processed as described below. Endometrial histology was screened in each of the two localizations of each sow, examining a total of four slides per localization and sow. For transcriptomic analysis, a total of 12 microarrays were performed (six microarrays for SP-treated sows and six for control sows; three for each region, distal and proximal). To validate the microarray results, 15 transcripts were selected, and gene expression was analyzed by qPCR. For this validation, three biological replicates (three sows) per region of the endometrium and three technical replicates per sample were performed.
Superovulation, Estrus Detection, and Insemination
The superovulation of donors was induced by the intramuscular administration of 1,000 IU equine chorionic gonadotropin (eCG; Folligon, Intervet, Boxmeer, The Netherlands) 24 h after weaning. Detection of estrus was performed by snout-to-snout contact of females with vasectomized mature boars while applying back-pressure to test for standing estrus reflex twice daily, beginning 1 day after weaning. Only sows with clear signs of estrus at 72–96 h posteCG administration were further intramuscularly administered 750 IU of human chorionic gonadotropin (Veterin Corion, Divasa, Farmavic S.A., Barcelona, Spain) at the first signs of estrus. Sows were postcervically inseminated 6 and 24 h after the onset of estrus with 1.5 × 109 spermatozoa in 40-mL doses prepared with semen from adult boars extended with BTS to 10% (v/v) of homologous SP (18) (i.e., around 5 mL of semen extended with 35 mL of BTS). A total of three boars was used and within each replicate a similar number of sows (n=6) from the two groups was inseminated with sperm doses from the same boar.
Ejaculate Collection and Seminal Plasma Preparation
Heterologous SP was obtained from full ejaculates without the gel fraction, which were collected from 16 healthy boars with known fertility using the gloved-hand method. These boars were different from those used for AI. Ejaculates were transported to the laboratory within 2 h after collection and centrifuged three times at 1,500 × g at 17°C for 10 min. The last SP-supernatant recovered was microscopically verified as sperm-free. SP from at least eight boars was then pooled, separated into aliquots of 40 mL and stored at −20°C until use. Immediately before their use, the SP doses were thawed at 37°C for 20 min and introduced into the uterine body of each sow by using a postcervical catheter.
Embryo and Tissue Collection
Sows were sedated with azaperone i.m. (Stresnil®, Landegger Strasse, Austria; 2 mg/kg body weight, intramuscular) and anesthetized with sodium thiopental (B.Braun VetCare SA, Barcelona, Spain; 7 mg/kg body weight, intravenous) administered in the marginal vein of an ear. Isofluorane (IsoFlo®, Madrid, Spain; 3–5% in air) was used to maintain anesthesia, and buprenorphine (Buprex®, Esteve, Barcelona, Spain; 0.3 mg/sow, intramuscular) was employed as analgesic.
For embryo recovery, the reproductive tract was exposed via mid-line incision, corpora lutea were counted on the ovaries, and the embryos were collected as previously reported (19). Briefly, a small incision in the uterine wall 30–40 cm below the uterotubal junction was performed with a blunt Adson forcep, and then, a glass cannula was inserted through the incision. A volume of 30 mL of Tyrode's lactate-HEPES-polyvinyl alcohol medium (20) at 37°C was introduced into the uterine horn from the tip of the uterine horn using a 60-mL syringe connected to a blunt needle. The washing medium was forced through the glass cannula into a 50-mL sterile tube by manual massage of the uterus. The incision in the uterus was closed with continuous 2–0 polyglactin 910 sutures. Then, the uterine horns were placed back inside the abdominal cavity, and the mid-ventral incision was closed with continuous one polyglactin 910 sutures in three layers. Finally, the incision area was treated with chlorhexidine, and a single intramuscular injection of a long-acting amoxicillin suspension at a dose of 15 mg/kg was administered.
For tissue collection, three pregnant sows from each group were subjected to hysterectomies immediately after embryo recovery and the uterine tracts were transported to the laboratory on ice within 2 h of collection. The uterine horns were then opened longitudinally at the anti-mesometrial side, and samples from the ad-cervical (distal) and ad-ovarian (proximal) areas of a uterine horn were surgically collected from each sow. For histological evaluation, immediately after collection, tissue samples were fixed for 24 h in 10% neutral-buffered formalin, embedded in paraffin wax and sectioned at 4 μm. For transcriptomic analysis, endometrial tissue samples were immediately transferred to 500 μL of RNAlater (Ambion, Thermo Fisher Scientific Baltics UAB, Vilnius, Lithuania), incubated at 4°C overnight and then stored at −80°C until further use.
Embryo Quality Evaluation
Immediately after collection, the washing medium was placed into Petri dishes and the embryos were located and assessed for morphological quality and developmental stage under a stereomicroscope. One-cell eggs and poorly developed embryos were classified as oocytes and degenerate embryos, respectively. The remaining embryos that exhibited appropriate morphology according to the criteria determined by the International Embryo Transfer Society (21) were considered viable (Figure 1). Embryos with completely compacted blastomeres, an undistinguishable cell boundary and a large perivitelline space were classified as morulae. Embryos with a differentiated blastocoel, inner cell mass and trophoblast and reduced thickness of the zona pellucida (ZP) were classified as full blastocysts. Blastocysts with increased outer diameter and very thinned ZP or with fractured or lost ZP were classified as peri-hatching blastocysts. The total cell number, as an indicator of embryo quality, was evaluated. Embryos were fixed in 4% paraformaldehyde in 0.1 M phosphate-buffered saline (PBS) for 30 min at room temperature. The embryos were then washed twice with PBS supplemented with 3 mg/mL BSA and slide-mounted in 4 μL of Vectashield (Vector Laboratories, Burlingame, CA, USA) containing 10 μg/mL Hoechst-33342 (Sigma–Aldrich Co., Madrid, Spain). Then, a small drop of vaseline was added to each corner of a coverslip, which was placed on the top of medium containing the embryos and depressed on the vaseline drops until the embryos were compressed. Embryos were analyzed by fluorescence microscopy using an excitation filter of 330–380 nm. The total cell number per blastocyst was determined by counting the number of nuclei with blue fluorescence.
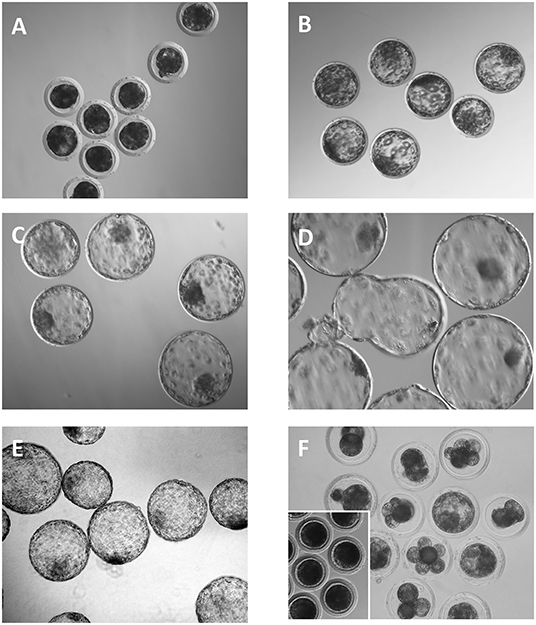
Figure 1. Embryos collected 6 days after the onset of estrus. (A) Compacted morulae. (B) Full blastocysts. (C–E) Expanded, hatching and hatched blastocysts, respectively. (F) Degenerated embryos. Note that most of the embryos have a developmental stage that is inappropriate for the day of the collection. Inset shows some unfertilized oocytes.
Histological Evaluation
Paraffin sections were cut and mounted on slides. The sections were deparaffinized, rehydrated, subjected to routine hematoxylin-eosin staining and examined using light microscopy (× 400) for general evaluation (22). The presence of congestion and margination of immune cells in blood vessels, edema, hemorrhage and inflammatory infiltrate in mucosal connective tissue, mitosis in cellular glands, and inflammatory cells and cellular debris in glands were evaluated and ranked as indicated in Table 1.
Total RNA Extraction
Total RNA was isolated from endometrium samples using Trizol reagent (Invitrogen, Carlsbad, CA, USA) according to the manufacturer's instructions. The quantity of the obtained total RNA was measured with a NanoDrop ND-1000 (Thermo Fisher Scientific, Fremont, CA, USA). The quality of the samples was assessed using an Agilent 2100 Bioanalyzer (Agilent Technologies, Santa Clara, CA, USA). The RNA integrity number (RIN) values obtained were in the range of 8–10.
Affymetrix GeneChip Microarray
Affymetrix Porcine Genome gene expression microarrays (Affymetrix, Santa Clara, CA, USA) were used. Microarray target sample processing, target hybridization, washing, staining and scanning steps were completed according to the manufacturer's instructions (Affymetrix, ThermoFisher Scientific, USA). Briefly, equal amounts of total RNA (250 ng) from each sample were converted to sense-strand cDNA using the one-cycle cDNA Synthesis Kit (Affymetrix) and an oligo-dT primer containing the T7 RNA polymerase promoter. In vitro transcription (IVT) of cRNA from cDNA was conducted following the RT-IVT method (23) using T7 RNA Polymerase. The cDNA and cRNA were purified using the Sample Cleanup Module (Affymetrix). The GeneChip IVT Labeling Kit (Affymetrix) was used for synthesis of biotin-labeled cRNA. Fragmented and labeled cDNA was loaded on the array chip (Porcine gene 1.0 ST GeneChip® Cartridge Array, Affymetrix), and hybridized during incubation at 45°C under rotation at 60 revolutions per minute, for 16 h. The hybridized array chip was then unloaded, subjected to washing and staining using a GeneChip® Fluidics Station 450 (Affymetrix), and finally scanned using the Affymetrix GeneChip® scanner GCS3000.
Microarray Data Analysis
The array data were examined using Partek Genomics Suite 7.0 (Partek). Data were first normalized using the Robust Multichip Average RMA method (24). Differentially expressed genes (DEGs) between endometrial samples of animals treated with SP and controls were established by performing a one way-ANOVA setting parameters as a fold change (FC) >1 or < −1 with p < 0.05. To obtain a biologically meaningful overview of the significantly modified transcripts of the SP group relative to the control group, an enrichment analysis was performed. Analysis of overrepresented Gene Ontology (GO) terms and pathways was performed with the DAVID (database for annotation, visualization and integrated discover) and KEGG (Kyoto Encyclopedia of Genes and Genomes) databases. The Spearman's rank-correlation test was used to assess the relationship between the fold changes obtained by microarray platform and q-PCR from a total of 15 genes.
Quantitative PCR Assay
For microarray data validation, 15 differentially expressed genes were tested by qPCR. First, total RNA from the same samples used for microarrays analysis was transcribed into cDNA using the High Capacity cDNA Reverse Transcription Kit (Applied Biosystems, Foster City, CA) with 25 mM dNTPs Mix, RT random primers, 20 U of RNase inhibitor and MultiScribe Reverse Transcriptase according to the manufacturer's protocol. All primers were designed using Primer Express™ software v3.0.1 (Applied Biosystems) and were commercially synthesized. Primer sequences are shown in Table 2. qPCR was performed with iTaq™ Universal SYBR® Green Supermix in 10-μL reactions with 50 or 150 nM for each set of primers. All reactions were carried out in a QuantStudio™ 5 Real-Time PCR System (Applied Biosystems). The thermal cycling profile was 50°C for 2 min, 95°C for 10 min, 40 cycles at 95°C for 15 s, and 60°C for 1 min. Melt curve analysis was carried out to verify the absence of primer-dimer.
The qPCRs were run in triplicate per gene and per sample, and data were analyzed with the Pfaffl method to identify eventual differences in PCR efficiency. Briefly, the relative expression ratio of the target genes was calculated based on target gene efficiency (E) and the mean Ct value of the target gene in an experimental sample condition vs. the control sample one and expressed via normalization to a housekeeping reference gene (GAPDH). The corresponding gene efficiency of one cycle in the exponential phase was calculated according to the equation: E = 10(−1/slope). Relative mRNA expression obtained by qPCR and microarray fold change data were compared using the Spearman's rank correlation coefficient.
Embryonic Data Analysis
The data were analyzed with the IBM SPSS 24.0 statistics package (IBM, Chicago, IL, USA). Differences were considered significant at P < 0.05. The results (six replicates) are presented as the mean ± standard deviation (SD) and as percentages. Data were analyzed for normality of residuals using the Kolgomorov-Smirnov test. Data expressed as the mean ± SD (number of corpora lutea, number of embryos and oocytes collected and total cell number per embryo) were compared by an unpaired Student's t-test corrected for inequality of variances (Levene test). The means of the percentage data (recovery and fertilization rates) were analyzed using the Mann-Whitney test for two independent samples. Percentage data were compared using Fisher's exact test.
Results
Endometrium Morphology Is Affected by the Infusion of SP
Endometrial samples collected 6 days after heterologous SP or BTS (control) infusions prior to AI showed that inflammatory changes were accentuated in the SP group relative to endometria from the control group. These changes included congestion, leukocyte margination, edema, hemorrhages and infiltrates of immune cells in the mucosal connective tissue and the uterine glands (Table 3; Figure 2).
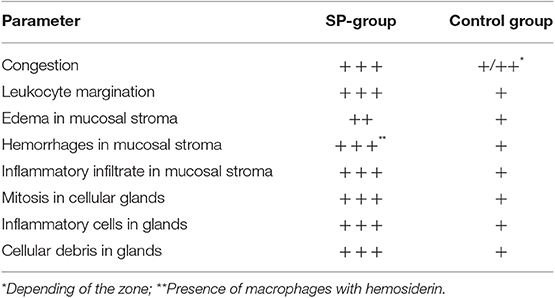
Table 3. Description of histological changes in the uterus 6 days after heterologous seminal plasma (SP) or BTS (control) infusions prior to insemination.
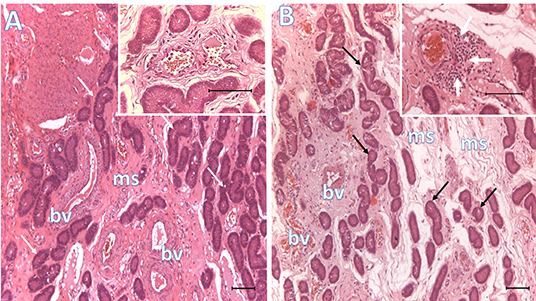
Figure 2. Histological representative images of the endometrium. Microphotographs of endometria 6 days after BTS (control, A) or heterologous seminal plasma (SP, B) infusions prior to AI, depicting conspicuous differences in mucosal edema, vascular congestion and peri-vascular infiltration of immune cells (see inset in B, white arrows; inset in A shows lack of peri-vascular infiltration of immune cells). Arrows, uterine glands; bv, blood vessels; ms, mucosal stroma. Bar: 100 μm.
Additional Infusion of Heterologous SP Modifies the Endometrial Transcriptome Profile
Following statistical analysis of the data yield by the Affymetrix microarray, we found in the SP-group that 1,052 genes were differentially expressed in the endometrium of the distal uterine horn (514 upregulated and 537 downregulated), and 552 in the proximal uterus (245 upregulated and 307 downregulated), relative to controls. After an overall enrichment analysis, there was a conspicuous overrepresentation of biological processes associated with biological regulation, cellular processes, or immune system-related genes (Figure 3). A selection of the most significant differentially expressed biological pathways (Tables 4, 5) included pathways for cytokine-cytokine receptor interaction, MAPK-ERK signaling, TGF-beta signaling, focal adhesion, cell cycle, and cell adhesion molecules (CAMs).
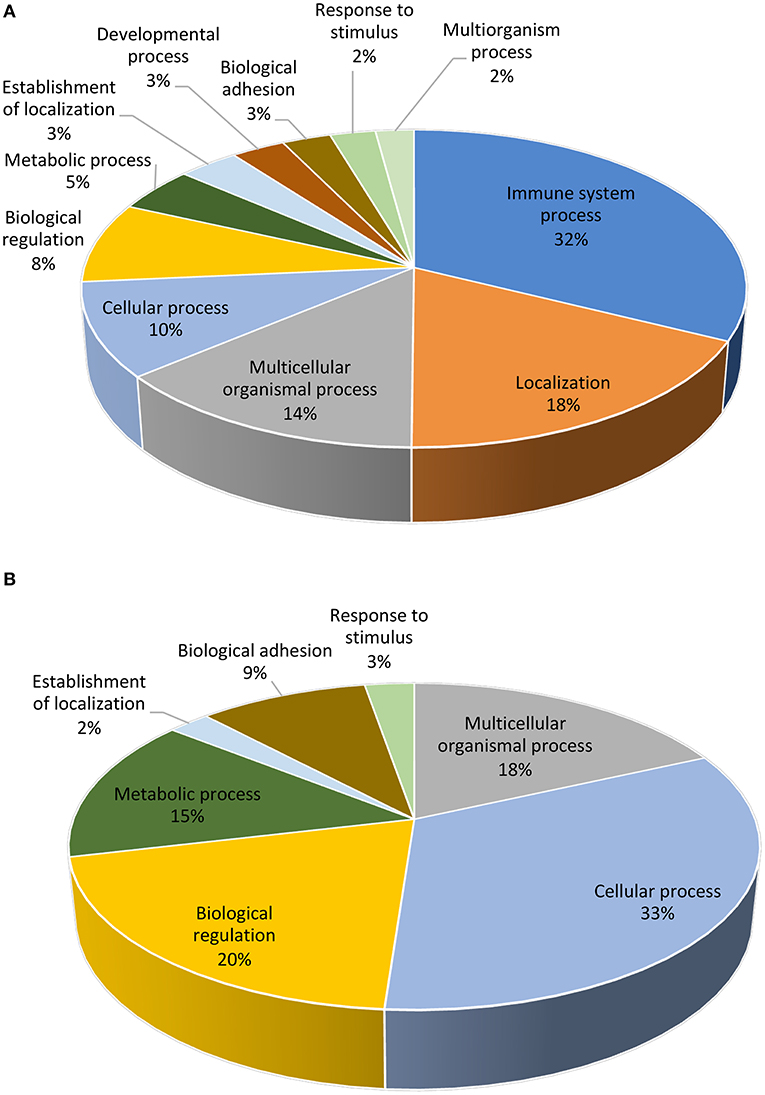
Figure 3. Functional distribution (KEGG database) of transcripts. The differentially expressed (P < 0.05) genes represented correspond to the distal (A) or proximal (B) regions of the uterine horn endometrium 6 days after heterologous seminal plasma (SP) or BTS (control) infusions prior to insemination.
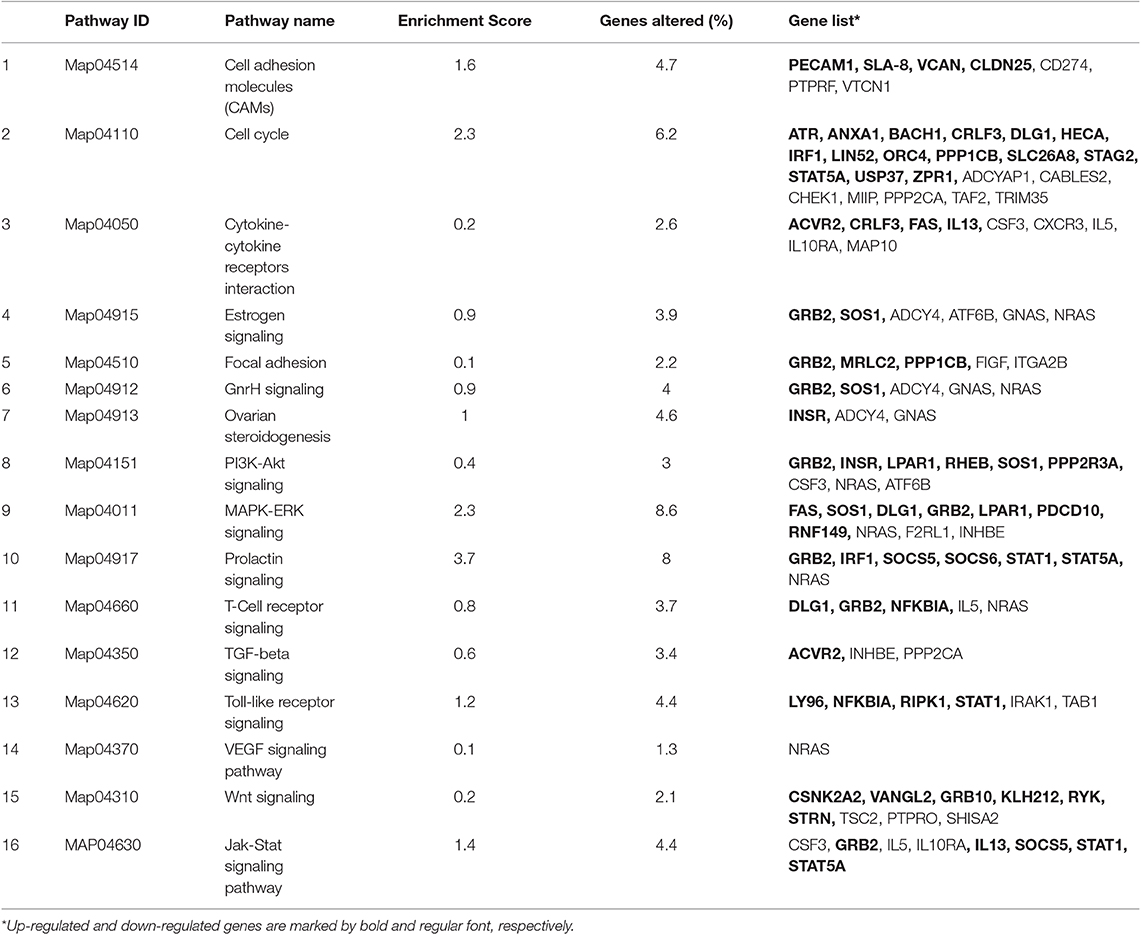
Table 4. The most significant differently expressed biological pathways examined with KEGG database (P < 0.05) in the distal region of the uterine horn endometrium 6 days after heterologous seminal plasma (SP) or Beltsville Thawing Solution (BTS; control) infusions prior to insemination.
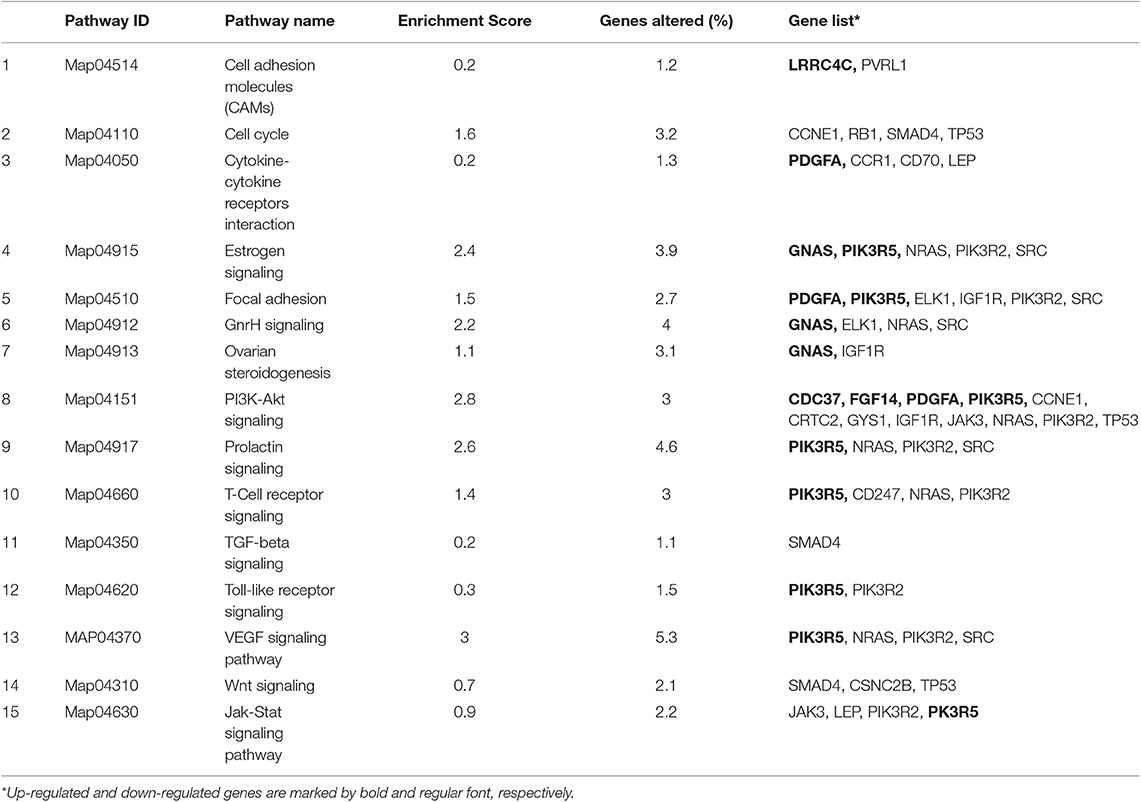
Table 5. The most significant differently expressed biological pathways examined with KEGG database (P < 0.05) in the proximal region of the uterine horn endometrium collected 6 days after heterologous seminal plasma (SP) or Beltsville Thawing Solution (BTS; control) infusions prior to insemination.
From that general gene set, we selected 126 significantly altered genes involved in different biological processes and pathways, with potential roles in embryonic development, implantation, or progression of pregnancy; 84 of them were in the distal uteri and 42 in the proximal uterine regions. The most significant findings in this gene set were embryonic skeletal system development, reproductive system development, interleukin-2 production, regulation of T cell activation, and steroid metabolic process (Figure 4).
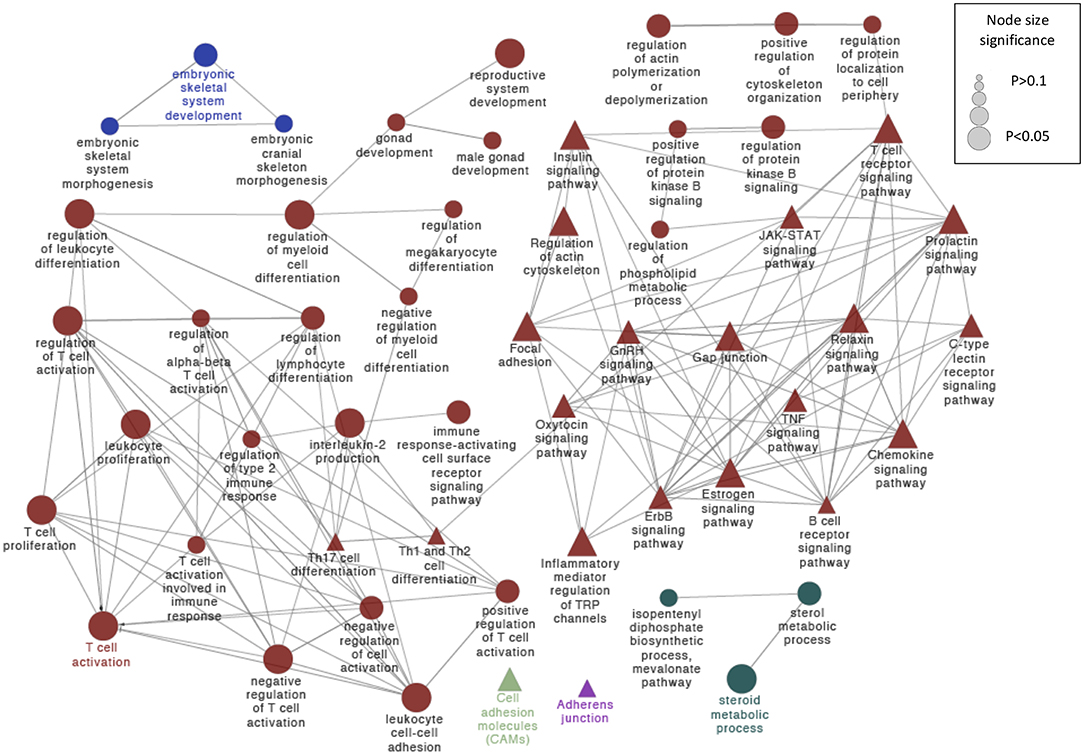
Figure 4. Schematic representation of selected altered transcripts potentially involved in embryo kinetics in distal and proximal regions of the SP treated endometrium relative to the BTS (control) group. The analysis of overrepresented functional categories was performed using the Cytoscape v3.0.0 application ClueGo v2.0.3. The following databases were used: GO subgroup biological process shown as circles and KEGG pathways shown as triangles. Terms are functionally grouped based on shared genes (kappa score) and are shown in different colors. The sizes of the nodes indicate the degree of significance, where the biggest nodes correspond to the highest significance. The most significant term defines the name of the group. The following ClueGo parameters were used: GO tree levels, 2–5 (first level = 0); minimum number of genes, 3; minimum percentage of genes, 5; P-value correction, Benjamini-Hochberg, terms with P < 0.05, GO term fusion; GO term connection restriction (kappa score), 0.4; GO term grouping, initial group size of 2 and 50% for group merge. The resulting network was modified; that is, some redundant and non-informative terms were deleted, and the network was manually rearranged.
Moreover, to gain insight into similarities among replicates, this particular set of genes was subjected to a hierarchical clustering procedure and presented as heatmaps (Figure 5; find gene id in this figure). The heatmap indicates that the selected differential gene set associates the biological samples correctly into two groups each representing one of the two conditions (SP vs. control).
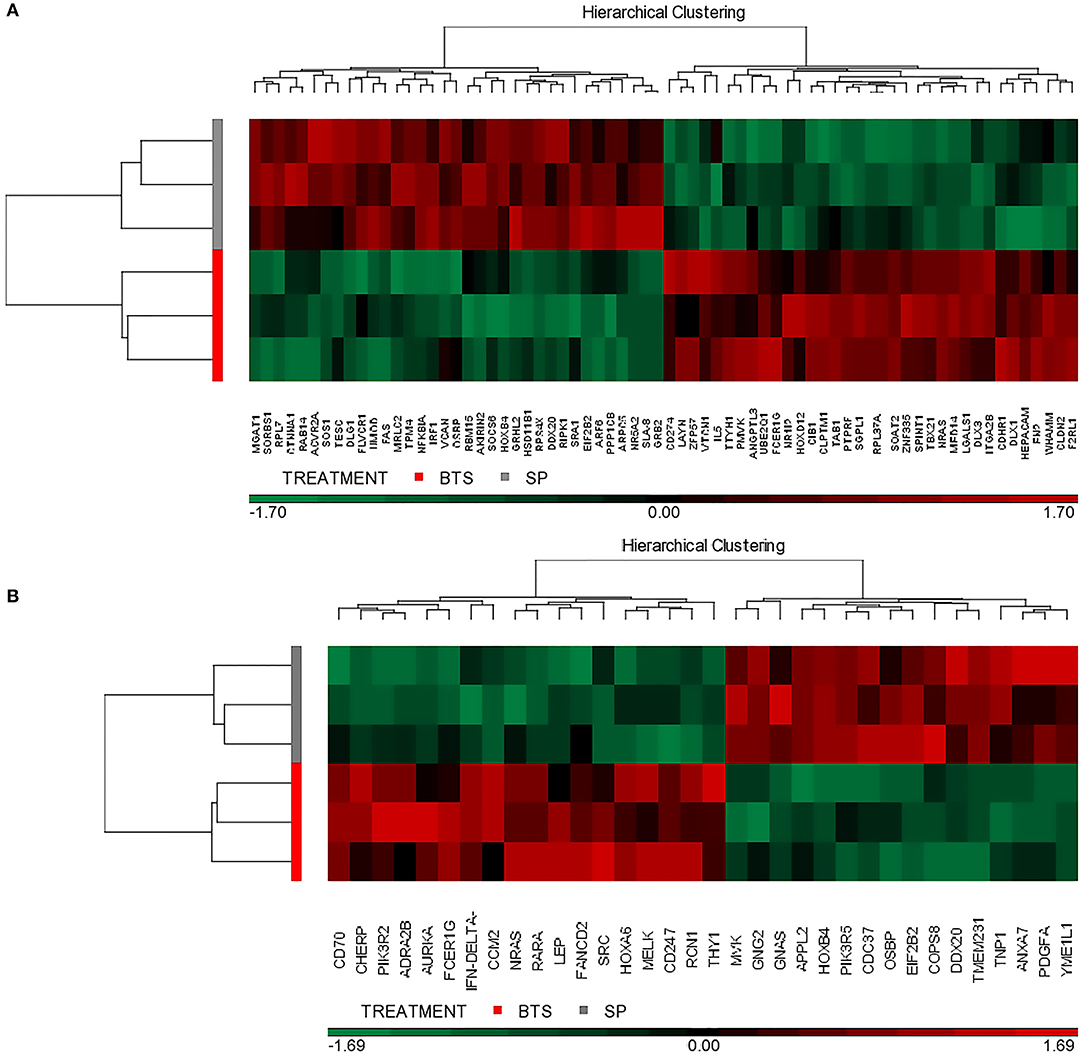
Figure 5. Hierarchical cluster analysis of gene expression patterns. The selected transcriptional profiles (DAVID database) were obtained from the distal (A) or proximal (B) regions of the uterine horn endometrium collected 6 days after heterologous seminal plasma (SP) or BTS (control) infusions prior to insemination. Colors correspond to relative RNA abundance for the detected genes, each of which is represented by one vertical bar. Red color indicates upregulated expression and green downregulated expression while black indicates the mean value.
Validation of the Microarray Data
To validate the microarray data, 15 genes were selected for qPCR, using the same RNA samples that were used for the microarray. The analyzed genes presented uniform patterns of expression when studied by both methods. There was a high correlation (Rh0 = 0.77; P < 0.001) between the fold change of genes analyzed by microarray and the fold change of genes analyzed by qPCR (Figure 6). The genes selected for real-time PCR are involved in the regulation of initial pregnancy, including embryonic development (GNAS, SGFL1, HOXB4), cell proliferation (VCAN, STAT5A, PDGFA, EIF2B2), signal transduction (DIRAS3, DDX20), and protein binding (TOR1A, RPL27A, TMEM200A, KCNIP2).
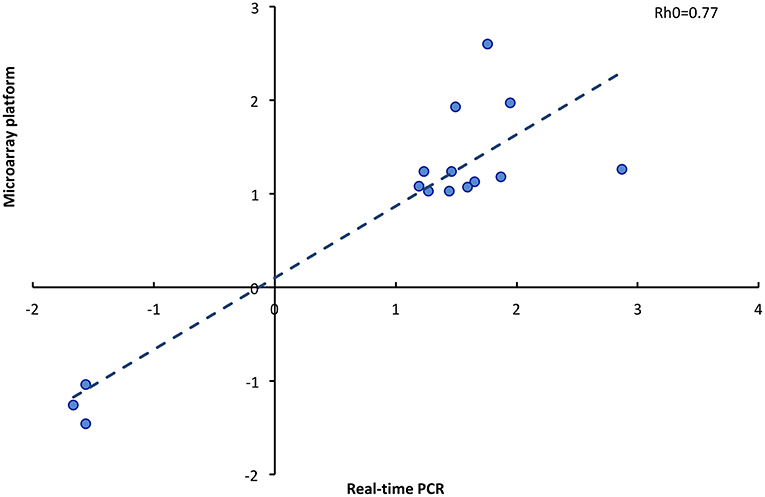
Figure 6. Correlation of fold change determined by microarray platform and real time PCR of 15 genes (Rho, Spearman coefficient).
Seminal Plasma Indirectly Affects Embryo Development
To evaluate the indirect influence of heterologous SP on embryo development, a total of 800 structures (embryos and unfertilized oocytes) recovered from 34 superovulated sows were assessed at Day 6 post-AI. The parameters evaluated are shown in Table 6.

Table 6. Reproductive parameters obtained 6 days after heterologous seminal plasma (SP) or Beltsville Thawing Solution (BTS; control) infusions 30 min prior to insemination.
All sows were embryo-bearing (“pregnant”) at the time of embryo collection. There were no differences in recovery rates between SP and control groups. The mean number of corpora lutea (CL) was also similar between groups, ranging from 16 to 49 CL and from 18 to 39 CL in the SP- and control groups, respectively. Of the recovered structures, more than 90% were classified as morphologically viable embryos, and the rest were oocytes or degenerated embryos, with no differences between groups. In total, the number of viable embryos recovered was 741, of which 17.3, 47.5, and 35.4% were morulae, full blastocysts and peri-hatching blastocysts, respectively. This embryo developmental distribution at collection varied according to the treatment used. Thus, while morulae were obtained only in 18.7% of the SP sows, in control sows the percentage was almost double (33.3%). Consequently, the sows infused with SP had fewer (P < 0.05) morulae and significantly (P < 0.05) more embryos at the full and peri-hatching blastocyst stages relative to controls (Figure 7). There were no differences in the number of total cells per embryo between the groups, within each embryonic stage (Figure 8).
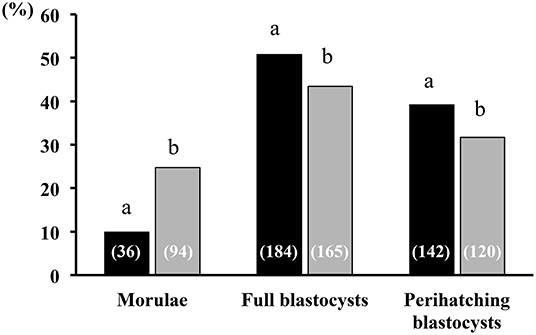
Figure 7. Embryonic developmental stage at collection. Developmental stages of embryo recovery 6 days after heterologous seminal plasma (SP; black bars; N = 16 sows) or BTS (Control; gray bars; N = 18 sows) infusions prior to insemination. Thirty minutes before each postcervical insemination (1.5 × 109 sperm/dose in 40 mL of BTS diluent), sows were infused with 40 mL of heterologous SP or BTS (control). At day 6 after the first insemination, the sows were subjected to laparotomy to collect the embryos and to evaluate their quality and developmental stage. A total of 362 and 379 viable embryos were collected in sows from the SP and BTS groups, respectively. Numbers in parentheses are the number of embryos for each stage. a,bDifferent letters within each embryonic developmental stage represent significant differences (P < 0.05; Fisher's exact test).
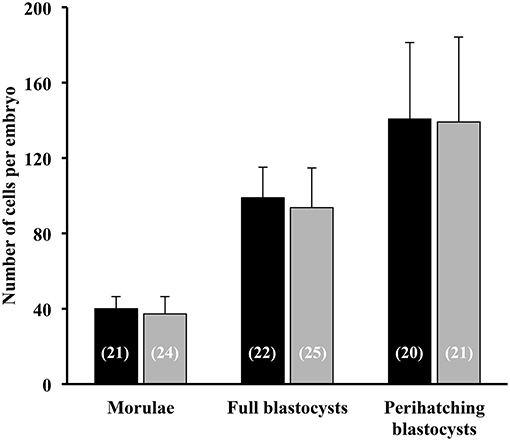
Figure 8. Total cell number of morulae, full blastocysts and peri-hatching blastocysts recovered 6 days after the postcervical infusion of heterologous seminal plasma (SP; black bars; N = 16 sows) or BTS (Control; gray bars; N = 18 sows) prior to insemination. Numbers in parentheses are the number of embryos counted for each stage.
Discussion
The present study demonstrated that cervical infusion of heterologous, pooled SP prior to AI indirectly influences preimplantation embryo development in pigs. SP treatment resulted in a higher percentage of advanced stage embryos as early as 6 days post-AI relative to the control group (that solely received BTS infusions before AI).
The observed differences in embryo development could be directly related to changes in the time of ovulation in response to SP exposure. Previous studies have reported that SP modifies the endocrine-immune-cytokine network in preovulatory follicles (25, 26) leading to an acceleration of ovulation through a decrease in the interval LH peak-ovulation time-point (27). Consequently, embryos derived from heterologous SP-treated sows should be in a more advanced stage of development. However, in addition to evidence of the potential biological effects that SP induces in the ovary, it is well-known that the actions of seminal fluid can extend beyond the immediate time of exposure and become a key point for pregnancy success (28–30). Unfortunately, the number of studies exploring this subject is low. To the best of our knowledge, only one study investigated the effects of SP infusions before AI on embryo viability and developmental competence (31). These authors concluded that SP altered the dynamics of pig embryos, increasing their number and viability, and suggested that SP influenced the embryo kinetics, delaying embryo development. Our findings, in which SP did not affect embryo viability but advanced the developmental stage of the embryos strongly disagree with that report. The discrepancies may be related to the fact that those authors evaluated developmental stage by measuring embryo diameter on day 9 of pregnancy, when embryos had already hatched. It is known that a variable percentage of hatched embryos collapses or presents an irregular morphology (32), making it a non-reliable result of measurement. Moreover, as in previous studies on ET (20, 33, 34) we obtained a high embryo viability rate in the control group (>90%), which makes it difficult to detect significant differences between groups.
Multiple studies of the endometrial transcriptome during the peri-implantation period, including comparisons of transcriptomic profiles in pregnant and non-pregnant animals have been carried out. In all of these studies, alterations in the expression of genes related to the immune response have been identified (35–38). However, endometrial receptivity is also crucial for the adequate progression of pregnancy during preimplantation period and is highly influenced by processes involving changes in cytokines and other molecules secreted into the luminal fluid (39, 40). Our study focused on investigating whether differences in embryo development could be associated with changes in the uterine environment motivated by infusions of heterologous, pooled SP prior to AI. For that purpose, we performed a transcriptional analysis of the uterine endometrium collected 6 days after heterologous SP or BTS (control) infusions prior to AI. We identified the presence of ~27,000 expressed transcripts in each region (distal and proximal) of the uterine horn endometrium examined, and following statistical analysis, we identified 1,052 transcripts with differential abundance in the distal region and 552 in the proximal region when the SP treatment group was compared to the control group. We used this gene list to identify pathways and gene ontology terms modified between experimental groups.
The enrichment analysis revealed an overrepresentation of genes and pathways associated with immune processes. It is known that components in SP initiate the inflammatory cascade in the female reproductive tract during the peri-ovulatory period and induce the widely studied maternal status of immune tolerance (11), which is maintained during the course of pregnancy to allow for successful attachment to the endometrium and proper development of the fetus thereafter (41–43). Some of the most notable immune-related pathways found to be altered in our study, mostly by downregulation, were T-cell receptor signaling, TNF signaling, NK cell-mediated cytotoxicity, JAK-STAT signaling and Toll-like receptor, among others. Interestingly, a particular overrepresentation of genes associated with regulatory T cells was found. It is known that a unique subpopulation of regulatory T cells (known as Treg cells) plays an essential role in attaining the status of maternal immune tolerance by acting as effective suppressors of inflammation and cell-mediated immunity (44). Therefore, these cells are fundamentally important for preventing immune rejection and thus tolerating the fetal allograft. They inhibit activation and proliferation of T cells (45, 46), prevents natural killer cell cytotoxicity (47), suppress B-cell proliferation (48), and inhibit dendritic cell and macrophage maturation and activation (49, 50) to facilitate pregnancy progression. In the absence of signals potent enough to drive Treg cells at the early time of gestation, the result is faulty implantation or posterior rejection of the fetuses (51). To ensure that sufficient Treg cells are present in the implantation area, their activation and proliferation during the preimplantation period are crucial. In the present study, some Treg-associated genes were found to be upregulated in SP-treated endometrium, including DLG1, FAS, LGALS1, STAT5A, and IRF1 in the uterine horn distal region (52–56). None of these genes or others associated with Treg cells were altered in the proximal uterine region. We also found an overrepresentation of the transforming growth factor-ß (TGF-ß) signaling pathway, which is especially important for inducing Treg cell development, in the distal region (45). The TGF-ß superfamily plays a critical role in driving the proliferation of Treg cells by modulating the function of dendritic cells (47). The present results suggest that exposure of the female genital tract to heterologous SP before AI enhance the proliferation of Treg cells and impact the maternal immune response to hemiallogeneic embryos at the early gestation phase (6 days post-AI). This is of particular importance for pig ET programs to decrease the immune response of the recipient females against the fully allogeneic embryos (i.e., different parents) that are transferred.
Furthermore, several studies have reported that preimplantation embryo development and growth are profoundly impacted by uterine cytokines and growth factors secreted into the luminal fluid (57–61). At this early time of pregnancy, the embryo is free-floating in the uterus, is not in direct contact with the maternal matrix, lacks a blood supply and is extremely dependent on signals generated by these cytokines and growth factors present in the maternal environment, which either support or restrict its development as they pass through the female reproductive tract (57). Embryos express cytokine receptors from fertilization until implantation, where they play an important role in embryo metabolic function and gene expression, cell number, and viability and developmental competence (61). Some of these embryotrophic cytokines and growth factors are regulated by semen exposure (41).
Interestingly, we found many genes related to cytokine-cytokine receptor-signaling pathways that were either over- or underexpressed (repressed) in uterine samples exposed to heterologous SP. For instance, we found CD27 and CD70 to be downregulated in distal and proximal uterine segments, respectively. These cytokines are costimulator of TNF (tumor necrosis factor), which is an embryotoxic cytokine that induces apoptosis and hinders embryonic development and implantation (8, 62). Our results demonstrate that heterologous SP infusions downregulate the expression of these genes, which may favor embryonic developmental ability.
In addition, the enrichment analysis revealed many functional categories comprised of hormone-related genes, such as relaxin and estrogen signaling, steroid metabolic process, oxytocin signaling and more. It is well-known that embryo development and its timing are regulated by many hormones that alter the physiology to support pregnancy (43). From fertilization to subsequent embryo implantation and placentation, each phase requires hormones. The present study detected the overrepresentation of steroid- and estrogen-related genes in particular, including upregulation of STAT5A, SRA1, OSBP, and many protein-coding genes such as DDX20 and INSR, which play important roles in steroid biosynthesis, or SOS1, GRB2, or TRIP4, which are implicated in estrogen signaling. This indicates that the uterine environment experiences modifications in hormone control due to the presence of heterologous SP.
Moreover, our study provided a number of genes involved in cellular changes in the uterus that are related to embryonic development and implantation. The PI3K/AKT and MAPK/ERK signaling pathways happened to be upregulated in both regions of the endometrium. It is believed that these signal transduction pathways are key regulators of a number of cellular functions, including cellular proliferation and migration, mitogenesis, differentiation, anti-apoptotic processes and cell survival (63). Moreover, they have been implicated in the differentiation, migration and cytoskeletal remodeling of embryo trophoblast cells during the preimplantation period (64, 65). In addition, studies in different species have agreed that deficiencies in MAPK/ERK proteins lead to early embryonic death due to the lack of signal transduction for proliferation and invasion of trophoblasts (66–68). Another interesting pathway found to be upregulated in our study was the Wnt signaling pathway. It has been speculated that the Wnt family plays an important role in regulating many biological functions, such as embryonic development, migration, tissue homeostasis and cell proliferation, among others. Previous studies have indicated that the Wnt signaling pathways are important during the early pregnancy period (69). However, its role in early embryo development is controversial.
Some other interesting genes appeared to be highly overexpressed. For instance, HOXB4 was upregulated in either the distal or proximal region of the endometrium in SP group. This gene is typically expressed in porcine endometrium during early embryo development due to the presence of the embryo (70), and it is crucial for embryo segmentation (71, 72). We also observed other embryo development-related genes highly overrepresented, such as GRHL2, a protein-coding gene that plays an important role in establishing distinct zones of primary neurulation, regulating epithelial morphogenesis by acting as a target gene-associated transcriptional activator of apical junctional complex components (73, 74). Other upregulated genes of interest found in our study include RAB14, MGAT1, and ACVR2A, which have previously been associated with early embryo development. The RAB14 complex is fundamental for the development of the germ layers of the fetuses (75), ACVR2A is involved in trophoblast proliferation, adhesion, migration and invasion (76), and lack of MGAT1 has been related to delayed embryo development (77).
Although the adhesion of embryos to endometrium occurs later in time (Day 12–16 of pregnancy), we found many altered genes specifically involved in the regulation of cell adhesion during the pig embryo preimplantation stage, including focal adhesion and cell adhesion molecule (CAM) pathways. Since implantation requires a reciprocal interaction between the embryo and the endometrium, we suggest that constituents of SP may be responsible for enhancing implantation success as early as Day 6 when the pig embryo starts to interact with the endometrial milieu making it competent for implantation. Interestingly, previous studies have reported higher pregnancy rates when embryos develop faster (78, 79), leading to increased embryo-endometrial synchrony and implantation rates (80). In this way, pregnancy rates were reduced when embryos blastulated on Day 6 compared to embryos that blastulated on day 5 (81).
Customary high-extension of semen and hence of its seminal plasma is applied for liquid pig AI and even more dramatic for frozen boar semen. Although SP is seemingly not needed for fertility, there are data available indicating that removal of SP is of no benefit (82) for sperm motility (83), sperm intactness and viability (84), capacitation and in vitro fertility (85) or even contraindicated since a total removal also removed cholesterol from the sperm membrane (86). It is praxis to maintain a 10–20% of SP in pig AI semen doses, although higher proportions of 20% do not improve farrowing rates (87). Differences are also present in the effect of homologous (own SP) or heterologous (unrelated boars) SP, probably for the compensating effect of boars with higher fertility or for the differential influence of the SP from selected ejaculate fractions vs. the entire ejaculate (18, 88, 89). Noteworthy, these influences seem related to the differential presence of specific proteins (82) and/or their variable quantity (90, 91). Therefore, it might be relevant to continue studying the effect of SP over fertility outcomes, including farrowing rates and litter size, for the sake of improving breeding efficiency using AI.
In conclusion, considering all of the information above, it seems that our findings are the first to demonstrate that heterologous SP infusions prior to conventional AI influence the molecular profile of the uterine endometrium during early pregnancy. Our findings further suggest that SP infusions positively impact preimplantation embryo development and modified the expression of certain genes involved in several biological processes and pathways associated with embryonic development and maternal immune system tolerance. More research is needed to determine the real potential of SP infusion or its active constituents to maximize reproductive outcomes after AI or ET, which is of great importance for the pig industry.
Data Availability Statement
The datasets generated for this study are available on request to the corresponding author.
Ethics Statement
The animal study was reviewed and approved by Ethical Committee for Experimentation with Animals of the University of Murcia, Spain (research code: 638/2012).
Author Contributions
CM, EM, MG, HR-M, CC, and MÁ-R conceived and designed the study. IP, EM, MG, and CC directed the experiments. CM, JC, IP, JR, JV, GF-D, XL, FP, EM, MG, CC, and MÁ-R performed the experiments. CM, EM, MG, HR-M, CC, and MÁ-R analyzed and interpreted the data. CM wrote the manuscript. IP, EM, MG, HR-M, CC, and MÁ-R revised and discussed the manuscript. All authors read and approved the manuscript for publication.
Funding
This study was supported by the Ministry of Economy and Competitiveness (Madrid, Spain)/the European Regional Development Fund (AGL2015-69735-R), the Seneca Foundation, Murcia, Spain (grant number 19892/GERM/15) and the Research Council FORMAS, Stockholm, Sweden (Project 2017-00946). EM, MG, HR-M, and MÁ-R obtained the funding to carry out the study.
Conflict of Interest
The authors declare that the research was conducted in the absence of any commercial or financial relationships that could be construed as a potential conflict of interest.
Acknowledgments
The authors are grateful to Alicia Nohalez and Moises Gonzalvez for their assistance throughout this work. We also thank the staff of Agropor SA (Murcia, Spain) and Porcisan (Murcia, Spain) piggeries for the excellent management of the animals. CM was supported by a postdoctoral grant from the Fundación Séneca (Murcia, Spain; 20780/PD/18), and JC was supported by a predoctoral grant from the Ministry of Economy and Competitiveness (Madrid, Spain; BES-2016-077869).
References
1. Rodin IM, Lipatov VI. Artificial insemination of sows. Problemy Zhivotnovodstva. (1935) 9:108–13.
2. Larsson K, Einarsson S. Fertility of deep frozen boar spermatozoa: influence of thawing diluents and of boars. Acta Vet Scand. (1976) 17:43–62.
3. Lyczynski A, Soczywko T, Rillo SM, de Alba Romero C. The effects of Predil-MRA synthetic seminal plasma used to inseminate sows and gilts on their reproductive efficiency. In: Johnson LA, Guthrie HD, editors. Boar Semen Preservation IV. Lawrence, KS: Allen Press, Inc. (2000). p. 250.
4. Matthijs A, Harkema W, Engel B, Woelders H. In vitro phagocytosis of boar spermatozoa by neutrophils from peripheral blood of sows. J Reprod Fertil. (2000) 120:265–73. doi: 10.1530/jrf.0.1200265
5. Rozeboom KJ, Troedsson MH, Crabo BG. Characterization of uterine leukocyte infiltration in gilts after artificial insemination. J Reprod Fertil. (1998) 114:195–9. doi: 10.1530/jrf.0.1140195
6. Rozeboom KJ, Troedsson MH, Rocha GR, Crabo BG. The chemotactic properties of porcine seminal components toward neutrophils in vitro. J Anim Sci. (2001) 79:996–1002. doi: 10.2527/2001.794996x
7. Robertson SA. Seminal plasma and male factor signalling in the female reproductive tract. Cell Tissue Res. (2005) 322:43–52. doi: 10.1007/s00441-005-1127-3
8. Robertson SA. Seminal fluid signaling in the female reproductive tract: lessons from rodents and pigs. J Anim Sci. (2007) 85:36–44. doi: 10.2527/jas.2006-578
9. Bromfield JJ. Seminal fluid and reproduction: much more than previously thought. J Assist Reprod Genet. (2014) 31:627–36. doi: 10.1007/s10815-014-0243-y
10. Rodriguez-Martinez H, Kvist U, Ernerudh J, Sanz L, Calvete JJ. Seminal plasma proteins: what role do they play? Am J Reprod Immunol. (2011) 66 (Suppl. 1):11–22. doi: 10.1111/j.1600-0897.2011.01033.x
11. Alvarez-Rodriguez M, Atikuzzaman M, Venhoranta H, Wright D, Rodriguez-Martinez H. Expression of immune regulatory genes in the porcine internal genital tract is differentially triggered by spermatozoa and seminal plasma. Int J Mol Sci. (2019) 20:1–20. doi: 10.3390/ijms20030513
12. Pang SF, Chow PH, Wong TM. The role of the seminal vesicle, coagulating glands and prostate glands on the fertility and fecundity of mice. J Reprod Fertil. (1979) 56:129–32. doi: 10.1530/jrf.0.0560129
13. Queen F, Dhabuwala CB, Pierrepoint CG. The effect of removal of the various accessory sex glands on the fertility of male rats. J Reprod Fertil. (1981) 62:423–36. doi: 10.1530/jrf.0.0620423
14. Peitz B, Olds Clarke P. Effects of seminal vesicle removal on fertility and uterine sperm motility in the house mouse. Biol Reprod. (1986) 35:608–17. doi: 10.1095/biolreprod35.3.608
15. Chen HQ, Chow PH. Effects of male accessory sex gland secretions on early embryonic development in the golden hamster. J Reprod Fertil. (1988) 84:341–4. doi: 10.1530/jrf.0.0840341
16. Carp HJ, Serr DM, Mashiach S, Nebel L. Influence of insemination on the implantation of transferred rat blastocysts. Gynecol Obstet Invest. (1984) 18:194–8. doi: 10.1159/000299080
17. Pursel VG, Johnson LA. Freezing of boar spermatozoa: fertilizing capacity with cncentrated semen and a new thawing procedure. J Anim Sci. (1975) 40:99–102. doi: 10.2527/jas1975.40199x
18. Caballero I, Vazquez JM, Centurion F, Rodriguez-Martinez H, Parrilla I, Roca J, et al. Comparative effects of autologous and homologous seminal plasma on the viability of largely extended boar spermatozoa. Reprod Domest Anim. (2004) 39:370–5. doi: 10.1111/j.1439-0531.2004.00530.x
19. Martinez CA, Nohalez A, Parrilla I, Vazquez JL, Roca J, Cuello C, et al. Surgical embryo collection but not non-surgical embryo transfer compromises postintervention prolificacy in sows. Theriogenology. (2017) 87:316–20. doi: 10.1016/j.theriogenology.2016.09.009
20. Martinez EA, Martinez CA, Nohalez A, Sanchez-Osorio J, Vazquez JM, Roca J, et al. Non-surgical deep uterine transfer of vitrified, in vivo-derived, porcine embryos is as effective as the default surgical approach. Sci Rep. (2015) 5:10587. doi: 10.1038/srep10587
21. Wright JM. Photographic illustrations of embryo developmental stage and quality codes. In: Stringfellow DA, Seidel SM, editors. Manual of the International Embryo Transfer Society. Savoy, IL: IETS (1998). p. 167–70.
22. Feldman AT, Wolfe D. Tissue processing and hematoxylin and eosin staining. Methods Mol Biol. (2014) 1180:31–43. doi: 10.1007/978-1-4939-1050-2_3
23. Van Gelder RN, Von Zastrow ME, Yool A, Dement WC, Barchas JD, Eberwine JH. Amplified RNA synthesized from limited quantities of heterogeneous cDNA. Proc Natl Acad Sci USA. (1990) 87:1663–7. doi: 10.1073/pnas.87.5.1663
24. Bolstad BM, Irizarry RA, Astrand M, Speed TP. A comparison of normalization methods for high density oligonucleotide array data based on variance and bias. Bioinformatics. (2003) 19:185–93. doi: 10.1093/bioinformatics/19.2.185
25. Einer-Jensen N, Hunter RHF. Counter-current transfer in reproductive biology. Reproduction. (2005) 129:9–18. doi: 10.1530/rep.1.00278
26. O'Leary S, Jasper MJ, Robertson SA, Armstrong DT. Seminal plasma regulates ovarian progesterone production, leukocyte recruitment and follicular cell responses in the pig. Reproduction. (2006) 132:147–58. doi: 10.1530/rep.1.01119
27. Schuberth HJ, Taylor U, Zerbe H, Waberski D, Hunter R, Rath D. Immunological responses to semen in the female genital tract. Theriogenology. (2008) 70:1174–81. doi: 10.1016/j.theriogenology.2008.07.020
28. Mann GV. The concept of predisposition. Arch Environ Health. (1964) 8:840–5. doi: 10.1080/00039896.1964.10663765
29. Aumüller G1, Riva A. Morphology and functions of the human seminal vesicle. Andrologia. (1992) 24:183–96. doi: 10.1111/j.1439-0272.1992.tb02636.x
30. Maegawa M, Kamada M, Irahara M, Yamamoto S, Yoshikawa S, Kasai Y, et al. A repertoire of cytokines in human seminal plasma. J Reprod Immunol. (2002) 54:33–42. doi: 10.1016/S0165-0378(01)00063-8
31. O'Leary S, Jasper MJ, Warnes GM, Armstrong DT, Robertson SA. Seminal plasma regulates endometrial cytokine expression, leukocyte recruitment and embryo development in the pig. Reproduction. (2004) 128:237–47. doi: 10.1530/rep.1.00160
32. Sun R, Lei L, Liu S, Xue B, Wang J, Shen J, et al. Morphological changes and germ layer formation in the porcine embryos from days 7-13 of development. Zygote. (2005) 23:266–76. doi: 10.1017/S0967199413000531
33. Angel MA, Gil MA, Cuello C, Sanchez-Osorio J, Gomis J, Parrilla I, et al. The effects of superovulation of donor sows on ovarian response and embryo development after non-surgical deep-uterine embryo transfer. Theriogenology. (2014) 81:832–9. doi: 10.1016/j.theriogenology.2013.12.017
34. Martinez EA, Angel MA, Cuello C, Sanchez-Osorio J, Gomis J, Parrilla I, et al. Successful non-surgical deep uterine transfer of porcine morulae after 24 hour culture in a chemically defined medium. PLoS ONE. (2014) 9:e104696. doi: 10.1371/journal.pone.0104696
35. Østrup E, Bauersachs S, Blum H, Wolf E, Hyttel P. Differential endometrial gene expression in pregnant and non-pregnant sows. Biol Reprod. (2010) 83:277–85. doi: 10.1095/biolreprod.109.082321
36. Samborski A, Graf A, Krebs S, Kessler B, Bauersachs S. Deep sequencing of the porcine endometrial transcriptome on day 14 of pregnancy. Biol Reprod. (2013) 88:1–13. doi: 10.1095/biolreprod.113.107870
37. Kiewisz J, Krawczynski K, Lisowski P, Blitek A, Zwierzchowski L, Ziecik AJ, et al. Global gene expression profiling of porcine endometria on days 12 and 16 of the estrous cycle and pregnancy. Theriogenology. (2014) 82:897–909. doi: 10.1016/j.theriogenology.2014.07.009
38. Lin H, Wang H, Wang Y, Liu C, Wang C, Guo J. Transcriptomic analysis of the porcine endometrium during embryo implantation. Genes. (2015) 6:1330–46. doi: 10.3390/genes6041330
39. Morris D, Diskin M. Effect of progesterone on embryo survival. Animal. (2008) 2:1112–9. doi: 10.1017/S1751731108002474
40. Bazer FW, Johnson GA. Pig blastocyst-uterine interactions. Differentiation. (2014) 87:52–65. doi: 10.1016/j.diff.2013.11.005
41. Robertson SA, Sharkey DJ. The role of semen in induction of maternal immune tolerance to pregnancy. Semin Immunol. (2001) 13:243–54. doi: 10.1006/smim.2000.0320
42. Ziecik A, Waclawik A, Kaczmarek M, Blitek A, Jalali BM, Andronowska A. Mechanisms for the establishment of pregnancy in the pig. Reprod Domest Anim. (2011) 46:31–41. doi: 10.1111/j.1439-0531.2011.01843.x
43. Waclawik A, Kaczmarek M, Blitek A, Kaczynski P, Ziecik AJ. Embryo-maternal dialogue during pregnancy establishment and implantation in the pig. Mol Reprod Dev. (2017) 84:842–55. doi: 10.1002/mrd.22835
44. Rudensky AY. Regulatory T cells and Foxp3. Immunol Rev. (2011) 241:260–8. doi: 10.1111/j.1600-065X.2011.01018.x
45. Sakaguchi S, Sakaguchi N. Role of genetic factors in organ-specific autoimmune diseases induced by manipulating the thymus or T cells, and not self-antigens. Rev Immunogenet. (2000) 2:147–53.
46. Shevach EM. CD4+CD25+ suppressor T cells: more questions than answers. Nat Rev Immunol. (2018) 2:389–400. doi: 10.1038/nri821
47. Ghiringhelli F, Ménard C, Terme M, Flament C, Taieb J, Chaput N, et al. CD4 + CD25 + regulatory T cells inhibit natural killer cell functions in a transforming growth factor–β-dependent manner. J Exp Med. (2005) 202:1075–85. doi: 10.1084/jem.20051511
48. Lim B, Sutherland RM, Zhan Y, Deliyannis G, Brown LE, Lew AM. Targeting CD45RB alters T cell migration and delays viral clearance. Int Immunol. (2006) 18:291–300. doi: 10.1093/intimm/dxh367
49. Misra N, Bayry J, Lacroix-Desmazes S, Kazatchkine MD, Kaveri SV. Cutting edge: human CD4+CD25+ T cells restrain the maturation and antigen-presenting function of dendritic cells. J Immunol. (2004) 172:4676–80. doi: 10.4049/jimmunol.172.8.4676
50. Taams LS, Akbar AN. Peripheral generation and function of CD4+CD25+ regulatory T cells. Curr Top Microbiol Immunol. (2005) 293:115–31. doi: 10.1007/3-540-27702-1_6
51. Aluvihare VR, Kallikourdis M, Betz AG. Regulatory T cells mediate maternal tolerance to the fetus. Nat Immunol. (2004) 5:266–71. doi: 10.1038/ni1037
52. Zanin-Zhorov A, Lin J, Scher J, Kumari S, Blair D, Hippen KL, et al. Scaffold protein Disc large homolog 1 is required for T-cell receptor-induced activation of regulatory T-cell function. Proc Natl Acad Sci USA. (2012) 109:1625–30. doi: 10.1073/pnas.1110120109
53. Liu H, Wang Y, Zeng Q, Zeng YQ, Liang CL, Qiu F, et al. Suppression of allograft rejection by CD8+CD122+PD-1+ Tregs is dictated by their Fasligand-initiated killing of effector T cells versus Fas-mediated own apoptosis. Oncotarget. (2017) 8:24187–95. doi: 10.18632/oncotarget.15551
54. Rodríguez-Muñoz A, Vitales-Noyola M, Ramos-Levi A, Serrano-Somavilla A, González-Amaro R, Marazuela M. Levels of regulatory T cells CD69(+) NKG2D(+) IL-10(+) are increased in patients with autoimmune thyroid disorders. Endocrine. (2016) 51:478–89. doi: 10.1007/s12020-015-0662-2
55. Cibrián D, Sánchez-Madrid F. CD69: from activation marker to metabolic gatekeeper. Eur J Immunol. (2017) 47:946–53. doi: 10.1002/eji.201646837
56. Chowdhury BP, Das S, Majumder S, Halder K, Ghosh S, Biswas S, et al. Immunomodulation of host-protective immune response by regulating Foxp3 expression and Treg function in Leishmania-infected BALB/c mice: critical role of IRF1. Pathog Dis. (2015) 73:ftv063. doi: 10.1093/femspd/ftv063
57. Hardy K, Spanos S. Growth factor expression and function in the human and mouse preimplantation embryo. J Endocrinol. (2002) 172:221–36. doi: 10.1677/joe.0.1720221
58. Kaye PL. Preimplantation growth factor physiology. Rev Reprod. (1997) 2:121–7. doi: 10.1530/ror.0.0020121
59. Sjöblom C, Roberts CT, Wikland M, Robertson SA. Granulocyte-macrophage colony-stimulating factor alleviates adverse consequences of embryo culture on fetal growth trajectory and placental morphogenesis. Endocrinology. (2005)146:2142–53. doi: 10.1210/en.2004-1260
60. O'Neill C. The potential roles for embryotrophic ligands in preimplantation embryo development. Hum Reprod Update. (2008) 14:275–88. doi: 10.1093/humupd/dmn002
61. Sharkey AM, Dellow K, Blayney M, Macnamee M, Charnock-Jones S, Smith SK. Stage-specific expression of cytokine and receptor messenger ribonucleic acids in human preimplantation embryos. Biol Reprod. (1995) 53:974–81. doi: 10.1095/biolreprod53.4.974
62. Chaouat G, Menu E, Clark DA, Dy M, Minkowski M, Wegmann TG. Control of fetal survival in CBA x DBA/2 mice by lymphokine therapy. J Reprod Fertil. (1990) 89:447–58. doi: 10.1530/jrf.0.0890447
63. Songyang Z, Cantley LC, Kaplan DR, Baltimore D, Franke TF. Interleukin 3-dependent survival by the Akt protein kinase. Proc Natl Acad Sci USA. (2002) 94:11345–50. doi: 10.1073/pnas.94.21.11345
64. Qiu Q, Yang M, Tsang BK, Gruslin A. EGF-induced trophoblast secretion of MMP-9 and TIMP-1 involves activation of both PI3K and MAPK signalling pathways. Reproduction. (2004) 128:355–63. doi: 10.1530/rep.1.00234
65. Bazer FW, Wu G, Spencer TE, Johnson GA, Burghardt RC, Bayless K. Novel pathways for implantation and establishment and maintenance of pregnancy in mammals. Mol Hum Reprod. (2009) 16:135–52. doi: 10.1093/molehr/gap095
66. Saba-El-Leil MK, Vella FD, Vernay B, Voisin L, Chen L, Labrecque N, et al. An essential function of the mitogen-activated protein kinase Erk2 in mouse trophoblast development. EMBO Rep. (2003) 4:964–8. doi: 10.1038/sj.embor.embor939
67. Jeong W, Kim J, Bazer FW, Song G. Epidermal growth factor stimulates proliferation and migration of porcine trophectoderm cells through protooncogenic protein kinase 1 and extracellular-signal-regulated kinases 1/2 mitogen-activated protein kinase signal transduction cascades during early pregnancy. Mol Cell Endocrinol. (2013) 381:302–11. doi: 10.1016/j.mce.2013.08.024
68. Sugiura R, Kita A, Shimizu Y, Shuntoh H, Sio SO, Kuno T. Feedback regulation of MAPK signalling by an RNA-binding protein. Nature. (2003) 424:961–5. doi: 10.1038/nature01907
69. Nayeem SB, Arfuso F, Dharmarajan A, Keelan JA. Role of Wnt signalling in early pregnancy. Reprod Fertil Dev. (2016) 28:525–44. doi: 10.1071/RD14079
70. Blitek A, Morawska E, Kiewisz J, Ziecik AJ. Effect of conceptus secretions on HOXA10 and PTGS2 gene expression, and PGE2 release in co-cultured luminal epithelial and stromal cells of the porcine endometrium at the time of early implantation. Theriogenology. (2011) 76:954–66. doi: 10.1016/j.theriogenology.2011.05.002
71. Gehring WJ. Homeotic genes, the homeobox, and the spatial organization of the embryo. Harvey Lect. (1985–1986) 81:153–72.
72. Scott MP, Carroll SB. The segmentation and homeotic gene network in early Drosophila development. Cell. (1987) 51:689–98. doi: 10.1016/0092-8674(87)90092-4
73. Petrof G, Nanda A, Howden J, Takeichi T, McMillan JR, Aristodemou S, et al. Mutations in GRHL2 result in an autosomal-recessive ectodermal dysplasia syndrome. Am J Hum Genet. (2014) 95:308–14. doi: 10.1016/j.ajhg.2014.08.001
74. Ming Q, Roske Y, Schuetz A, Walentin K, Ibraimi I, Schmidt-Ott KM, et al. Structural basis of gene regulation by the Grainyhead/CP2 transcription factor family. Nucleic Acids Res. (2018) 46:2082–95. doi: 10.1093/nar/gkx1299
75. Ueno H, Huang X, Tanaka Y, Hirokawa N. KIF16B/Rab14 molecular motor complex is critical for early embryonic development by transporting FGF receptor. Dev Cell. (2011) 20:60–71. doi: 10.1016/j.devcel.2010.11.008
76. Brennecke SP, Murthi P, Keogh RJ, Yong HJ, Kalionis B. Decidual ACVR2A regulates extravillous trophoblast functions of adhesion, proliferation, migration and invasion in vitro. Pregnancy Hypertens. (2017) 12:189–93. doi: 10.1016/j.preghy.2017.11.002
77. Grasa P, Kaune H, Williams SA. Embryos generated from oocytes lacking complex N- and O-glycans have compromised development and implantation. Reproduction. (2012) 144:455–65. doi: 10.1530/REP-12-0084
78. Lewin A, Schenker JG, Safran A, Zigelman N, Avrech O, Abramov Y, et al. Embryo growth rate In vitro as an indicator of embryo quality in IVF cycles. J Assist Reprod Genet. (1994) 11:500–3. doi: 10.1007/BF02216029
79. Shapiro BS, Richter KS, Harris DC, Daneshmand ST. A comparison of day 5 and day 6 blastocyst transfers. Fertil Steril. (2001) 75:1126–30. doi: 10.1016/S0015-0282(01)01771-X
80. Van Voorhis BJ, Dokras A. Delayed blastocyst transfer: is the window shutting? Fertil Steril. (2008) 89:31–2. doi: 10.1016/j.fertnstert.2007.01.172
81. Shapiro BS, Daneshmand ST, Garner FC, Aguirre M, Thomas S. Large blastocyst diameter, early blastulation, and low preovulatory serum progesterone are dominant predictors of clinical pregnancy in fresh autologous cycles. Fertil Steril. (2008) 90:302–9. doi: 10.1016/j.fertnstert.2007.06.062
82. Bromfield JJ. A role for seminal plasma in modulating pregnancy outcomes in domestic species. Reproduction. (2016) 152:R223–32. doi: 10.1530/REP-16-0313
83. Rodríguez-Martinez H, Saravia F, Wallgren M, Roca J, Peña FJ. Influence of seminal plasma on the kinematics of boar spermatozoa during freezing. Theriogenology. (2008) 70:1242–50. doi: 10.1016/j.theriogenology.2008.06.007
84. Saravia F, Wallgren M, Johannisson A, Calvete JJ, Sanz L, Peña FJ, et al. Exposure to the seminal plasma of different portions of the boar ejaculate modulates the survival of spermatozoa cryopreserved in MiniFlatPacks. Theriogenology. (2009) 71:662–75. doi: 10.1016/j.theriogenology.2008.09.037
85. Suzuki K, Asano A, Eriksson B, Niwa K, Shimizu H, Nagai T, et al. Capacitation status and In vitro fertility of boar spermatozoa: effects of seminal plasma, cumulus-oocytes-complexes-conditioned medium and hyaluronan. Int J Androl. (2002) 25:84–93. doi: 10.1046/j.1365-2605.2002.00330.x
86. Kruse R, Dutta PC, Morrell JM. Colloid centrifugation removes seminal plasma and cholesterol from boar spermatozoa. Reprod Fertil Develop. (2011) 23:858–65. doi: 10.1071/RD10260
87. Kirkwood RN, Vadnais ML, Abad M. Practical application of seminal plasma. Theriogenology. (2008) 70:1364–7. doi: 10.1016/j.theriogenology.2008.07.018
88. Caballero I, Vazquez JM, Rodriguez-Martinez H, Gil MA, Calvete JJ, Sanz L, et al. Influence of seminal plasma PSP-I/PSP-II spermadhesin on pig gamete interaction. Zygote. (2005) 13:11–6. doi: 10.1017/S0967199405003072
89. Alkmin DV, Perez-Patiño C, Barranco I, Parrilla I, Vazquez JM, Martinez EA, et al. Boar sperm cryosurvival is better after exposure to seminal plasma from selected fractions than to those from entire ejaculate. Cryobiology. (2014) 69:203–10. doi: 10.1016/j.cryobiol.2014.07.004
90. Barranco I, Rubér M, Perez-Patiño C, Atikuzzaman M, Martinez EA, Roca J, et al. The seminal plasma of the boar is rich in cytokines, with significant individual and intra-ejaculate variation. Am J Reprod Immunol. (2015) 74:523–32. doi: 10.1111/aji.12432
Keywords: seminal plasma, endometrium, transcriptome, embryo, preimplantation, pig
Citation: Martinez CA, Cambra JM, Parrilla I, Roca J, Ferreira-Dias G, Pallares FJ, Lucas X, Vazquez JM, Martinez EA, Gil MA, Rodriguez-Martinez H, Cuello C and Álvarez-Rodriguez M (2019) Seminal Plasma Modifies the Transcriptional Pattern of the Endometrium and Advances Embryo Development in Pigs. Front. Vet. Sci. 6:465. doi: 10.3389/fvets.2019.00465
Received: 31 October 2019; Accepted: 02 December 2019;
Published: 18 December 2019.
Edited by:
Arumugam Kumaresan, National Dairy Research Institute (ICAR), IndiaReviewed by:
M. Karunakaran, National Dairy Research Institute (ICAR), IndiaDivakar Justus Ambrose, University of Alberta, Canada
Copyright © 2019 Martinez, Cambra, Parrilla, Roca, Ferreira-Dias, Pallares, Lucas, Vazquez, Martinez, Gil, Rodriguez-Martinez, Cuello and Álvarez-Rodriguez. This is an open-access article distributed under the terms of the Creative Commons Attribution License (CC BY). The use, distribution or reproduction in other forums is permitted, provided the original author(s) and the copyright owner(s) are credited and that the original publication in this journal is cited, in accordance with accepted academic practice. No use, distribution or reproduction is permitted which does not comply with these terms.
*Correspondence: Cristina Cuello, ccuello@um.es