- 1Laboratorio de Biología Celular y Tisular, Departamento de Morfología, Centro de Ciencias Básicas, Universidad Autónoma de Aguascalientes, Aguascalientes, Mexico
- 2Laboratorio de Estudios Ambientales, Departamento de Fisiología y Farmacología, Centro de Ciencias Básicas, Universidad Autónoma de Aguascalientes, Aguascalientes, Mexico
- 3Departamento de Biología Celular, Centro de Investigación y de Estudios Avanzados del IPN, Ciudad de México, Mexico
- 4Groupe de Recherche sur les Maladies Infectieuses en Production Animale, Faculté de Médecine Vétérinaire, Université de Montréal, Saint-Hyacinthe, QC, Canada
Actinobacillus pleuropneumonia is a swine (host) specific respiratory pathogen and the etiological agent of swine pleuropneumonia which affects pigs of all ages, many being asymptomatic carriers. This pathogen has high morbidity and mortality rates which generates large economic losses for the pig industry. Actinobacillus pleuropneumoniae is a widely studied bacterium, however its pathogenesis is not yet fully understood. The prevalence of the 18 serotypes of A. pleuropneumoniae varies by geographic region, in North American area, more specifically in Mexico, serotypes 1, 3, 5b, and 7 show higher prevalence. Actinobacillus pleuropneumoniae is described as a strict extracellular pathogen with tropism for lower respiratory tract. However, this study depicts the ability of these serotypes to adhere to non-phagocytic cells, using an endothelial cell model, as well as their ability to internalize them, proposing it could be considered as an intracellular pathogen.
Introduction
Actinobacillus pleuropneumoniae (App) is a respiratory pathogen member of the family Pasteurellaceae and etiological agent of swine pleuropneumonia (1, 2). Depending on its nicotinamide adenine dinucleotide (NAD) requirements, it is classified into two biotypes, biotype 1 groups NAD-dependent strains, biotype 2 groups NAD-independent strains. Currently 18 different serotypes are recognized. The biotypes and serotypes of A. pleuropneumoniae have genetic, structural, and virulence differences. Serotyping is mainly based on capsular antigens. The presence and prevalence of serotypes varies between countries. In North America serotypes 1 and 5 historically are the most commonly isolated. In recent findings most prevalent App serotypes in Canada were 5 and 7 with a notable reduction of serotype 1 incidence due to serological control of breeders. While in Mexico serotype 1 still has a high prevalence alongside serotypes 3, 5, and 7 (3–8).
Swine pleuropneumonia caused by App and is a major problem for swine production. It has a significant impact on animal welfare and economic production, due to high morbidity and mortality rates, the reduction of average daily weight gain and feed conversion rates, loss due to slaughter and intervention costs (2, 9, 10). Actinobacillus pleuropneumoniae can be transmitted between herds through various vectors, including direct contact with infected animals, contaminated aerosols and fomites (10, 11).
Antibiotic and vaccination-based therapies help reduce the severity of disease signs and decrease mortality rates. However, they are not effective in eliminating bacteria; even pigs that survive the acute phase can reach subclinical infection and be persistent carriers, harboring App in the tonsils and suffering from chronic lung lesions (12–15).
App virulence is mainly determined by the production of pore forming RTX toxins: ApxI, ApxII, and Apx III. Which have hemolytic and cytotoxic properties and are secreted by the different serotypes in various combinations. ApxI is expressed by serotypes 1, 5, 9, 10, 11, and 14. ApxII is produced by all serotypes except 10 and 14; and ApXIII is present in serotypes 2, 4, 6, 8, and 15 (16). ApxIV is found in all serotypes, is specific to App therefore highly used in diagnostics for species identification (16, 17).
Many other App virulence factors have been described. Such as siderophores, transferrin binding proteins for iron acquisition and binding of porcine hemoglobin by lipopolysaccharide and outer membrane proteins involved in maltose uptake. The lesion induction by Apx toxins, that provokes cellular lysis. Presence of fimbriae, lipopolysaccharides, and adhesins involved in adhesion to name a few (18). Additionally, A. pleuropneumoniae can form biofilms that decreases its susceptibility to bactericides and the host's immune system. Likewise, other resistance genes and plasmids have been identified (12–14). Although the pathobiology of App infection has been extensively studied (17, 19), some aspects of its pathogenesis, mainly its relationship with host cells, are still to be known.
To establish infection, pathogenic bacteria must evade the immune response and host defense mechanisms. This is achieved thanks to the interaction established with non-phagocytic cells, such as endothelial and epithelial cells, managing to adhere, internalize, and in some cases survive and replicate (20).
The Gram-negative bacterium Haemophilus influenzae, member of Pasteurellaceae, colonizes the upper respiratory tract and causes middle ear infection, sinusitis, conjunctivitis, chronic bronchitis, bronchiectasis, cystic fibrosis, and pneumonia. This bacterium adheres to respiratory epithelial mucus. Provokes host cell cytoskeleton rearranges, form lamellipodia which surrounds adherent bacteria and produces a membrane-bound vacuole. Its invasion is inhibited by cytochalasin D (actin polymerization disruptor). Nevertheless, micropinocytosis may be the predominant invasion pathway for this pathogen, in which case, actin cytoskeleton has not this important role (21).
Also in Pasteurellaceae, Actinobacillus suis, an opportunistic pig pathogen, colonize tonsils in asymptomatically way. This bacteria has similarities with App, shares several virulence factors and causes similar diseases such as hemorrhagic fibrinopleuropneumonia and septicemia. Even though its pathogenesis is poorly understood, it is known that the OmpA protein, also present in App, plays a key role in adhesion to the tonsils and is crucial for achieving initial colonization and invasion of the central nervous system (22). Actinobacillus pleuropneumoniae has been described as an extracellular pathogen capable of adhering to host cell surface as tracheal or alveolar cells, but unable to invade them (6, 23).
The mammalian lung's structure is design for its main function, the gas exchange, which takes place in the alveolar region where air and blood are brought in close proximity over a large surface. Blood flows in a capillary network embedded in inter-alveolar septa. The barrier between air and blood consists of a continuous alveolar epithelium (a mosaic of type I and type II alveolar epithelial cells), a continuous capillary endothelium and the connective tissue layer in-between (24). Endothelial cells lining the pulmonary microvessels are in proximity to the alveolar epithelial cells and their individual basement membranes fused together in order to facilitate gas diffusion. There is evidence that respiratory pathogen as influenzae virus may sometimes spread beyond respiratory tract to cause disseminated infection taken advantage of the proximity between the alveolar epithelium and the endothelia (25, 26). Lubkin and Torres (26) mention that pathogenic bacteria use the bloodstream as a means of transport through the body, so that at some point they come in contact with the endothelium (27). This can exacerbate the lung pathologies (28–32).
Bacterial pathogens that causes systemic infections disseminate through vascular or lymphatic vessels to different target organs. To achieve this, they must adhere to the endothelial tissue, causing the rearrangement of the endothelial membrane through various stimuli, modifying the permeability of the endothelium or using endothelial vasculature or caveolins to reach the bloodstream (33–35).
App is considered a pathogen exclusive of the porcine respiratory system. However, it has been found in tonsils of carrier pigs. Sometimes produces arthritis, osteomyelitis, hepatitis, meningitis, and nephritis, in which the only detectable pathogen is A. pleuropneumoniae, it is not known if this represent the possibility of App spread in cases with extensive damage (26, 36). In this sense, the purpose of the present study was to confirm App serotypes 1, 3, 5b, and 7 (4074, JL03, L20, and AP76 strains) adhesion capacity as well to determine A. pleuropneumoniae invasion ability into a non-phagocytic cell culture model of endothelial cells as a novel infection mechanism.
Materials and Methods
Endothelial Cell Culture
Pig aortic endothelial cell culture previously established by Burciaga-Nava et al. (37) was maintained using Dulbecco's Modified Eagle Medium (DMEM) (Gibco, BRL, Grand Island, NY) supplemented with 10% fetal bovine serum (FBS) (Gibco, BRL, Grand Island, NY), 100 μg/ml penicillin/streptomycin and 2.5 μg/ml amphotericin at 37°C in a humid environment with 5% CO2 and 95% air.
Bacterial Culture Growth
Actinobacillus pleuropneumoniae 4074, JL03, L20, and AP76 strains (serotypes 1, 3, 5b, and 7, respectively) were grown in Brain Heart Infusion (BHI) medium (BD BIOXON, Madrid, Spain) supplemented with 15 μg/ml nicotinamide adenine dinucleotide (Sigma Chemical Co., St. Louis, Mo, USA) at 37°C. Overnight bacterial cultures with optical density of 0.6 at 600 nm were harvested by centrifugation (10,000 g × 5 min) and resuspended in DMEM medium until reaching an optical density of 0.6 at 600 nm providing the final concentration required for the assays (6, 38) based on previous growth curve models performed for each strain (data not included).
Adhesion Assay
Endothelial cells (104) were seeded into 48-well-tissue culture plates. After overnight incubation confluent cells were infected with the different App strains at a multiplicity of infection (MOI) of 10:1. Plates were incubated for 0.5, 1, and 2 h, and subsequently washed several times with Dulbecco's Phosphate-Buffered Saline (DPBS) to remove non-adherent bacteria. Cells with adhered bacteria were released from the wells using Tryple™ (Gibco, BRL, Grand Island, NY) and resuspended in BHI medium. Serial dilutions were performed and plated on agar to determine the number of bacteria adhered to the endothelial cells by colony forming unit (CFU) per well counting (6, 38, 39).
Internalization Assay
Endothelial cells were seeded into 48-well-culture plates and infected as previously described allowing the interaction for 3 h. After incubation non-adhered bacteria was removed with DPBS washes. Gentamicin protection assay was performed by incubating cultures in DMEM supplemented with 100 μg/ml gentamicin for 1 h at 37°C. Cultures were washed three times with DPBS and cells were lysed with 100 μl pre-chilled sterile distilled water. The lysate underwent several dilutions and was plated on agar plates for subsequent CFU count (6, 40, 41).
Internalization percentage was calculated dividing the organisms inoculated (CFU/ml) by organisms recovered (CFU/ml) and multiplying by 100. Results are presented as mean ± SD (42).
Immunofluorescence Labeling for Cytoskeleton, Endoplasmic Reticulum, and Golgi Apparatus
To test App invasion, endothelial cells were seeded (104) (37) into permanox Lab-Tek chamber slides (Thermo Scientific) and incubated overnight. Cells were infected with the different App strains with a MOI of 3:1. After a 3-h incubation, non-adherent bacteria were removed by a DPBS wash and subsequently fixed 30 min using formaldehyde 3.7% at room temperature. Then cells were gently wasehed three times with DPBS.
After fixation, endothelial cells' membrane was permeabilized with 0.1% Triton X-100 (US Biological, Salem, Ma, USA) for 5 min at room temperature (43, 44), followed by 2 washes with DPBS. Once the membrane was permeabilized to observe the implication of different cellular organelles in App invasion by immunofluorescent labeling different labels were used according to the organelle to be observed, which are described below. Several repetitions were performed for each organelle.
Actin Labeling
After cell fixation and permeabilization, cells were washed twice with DPBS. Then cells were blocked with 1% BSA-DPBS. Cells were washed twice with DPBS and then actin cytoskeleton was labeled with Alexa Fluor 488 Phalloidin [1:100] (Molecular Probes, Eugene, Oregon, USA) for 30 min at 37°C, after labeling cells were gently washed three times with DPBS. Then cells were blocked with 1% BSA-DPBS, washed twice with DPBS and then cells were incubated with primary antibody against App. After that, cells were washed twice with DPBS, cells were incubated with Anti Rabbit IgG Alexa Fluor 594. The cells were washed twice with DPBS, then nuclei were labeling with Hoetch (2 μM) during 15 min at room temperature, then were washed twice, and finally cells were mounted with Prolong Gold (Molecular Probes). Cell control only were labeled with phalloidin as described above.
Tubulin Labeling
After cell fixation and permeabilization, cells were washed twice with DPBS. Then cells were blocked with 1% BSA-DPBS. Cells were washed twice with DPBS and then tubulin cytoskeleton was labeled by 2 h incubation at 37°C using a primary monoclonal antibody mouse anti α-tubulin [2 μM] (Sigma Chemical Co., St. Louis, Mo, USA), after this, cells were gently washed twice with DPBS. Then cells were incubated 1 h at 37°C with the secondary monoclonal antibody Alexa Fluor 488 Goat anti-mouse IgG [2 μM] (Sigma Chemical Co., St. Louis, Mo, USA). Then cells were blocked with 1% BSA-DPBS, washed twice with DPBS and then cells were incubated with primary antibody against App. After that cells were washed twice with DPBS, cells were incubated with Anti Rabbit IgG Alexa Fluor 594. The cells were washed twice with DPBS, then nuclei were labeling with Hoechst 33258 [2 μM] (Molecular Probes, Eugene, Oregon, USA) during 15 min at room temperature, then were washed twice, and finally cells were mounted with Prolong Gold (Molecular Probes, Eugene, Oregon, USA). Cell control only were labeled for tubulin as described above.
Actin and Tubulin Influence on App Internalization Test
In order to stablish the actin or tubulin cytoskeleton role in App entry to the endothelial cell, cultures underwent a pre-incubation for 5 min with 1 μM actin disruptor Cytochalasin D (CD) (Sigma Chemical Co., St. Louis, Mo, USA) or for tubulin, 1 μM tubulin disruptor Colchicine (Sigma Chemical Co., St. Louis, Mo, USA) (45–47). After the pretreatment with cytoskeleton disruptors, the cells were gently washed twice with DPBS. Then they were labeled with phalloidin or with the anti α-tubulin antibody, as is described above.
Golgi and Endoplasmic Reticulum Labeling
Golgi Apparatus was labeled using CellLight Golgi RFP BacMam 2.0 (Molecular Probes, Eugene, Oregon, USA) as for endoplasmic reticulum (ER) SelectFX Alexa Fluor 488 Endoplasmic Reticulum Labeling Kit was used (Molecular Probes, Eugene, Oregon, USA). Labeling was performed following manufacturers' protocols.
Actinobacillus pleuropneumoniae was labeled for 2 h at 37°C with a primary polyclonal antibody Rabbit anti-App obtained by Guerrero-Barrera et al. (48) and a secondary polyclonal antibody Alexa Fluor 594 Goat anti-rabbit IgG [2 μM] (Molecular Probes, Eugene, Oregon, USA) for 1 h at 37°C. Samples were observed with a Carl Zeiss LSM700 confocal scanning microscope at 40X.
The length of 40 randomly selected nuclei from samples of the different interactions performed were measured using the blue ZEN software (Carl Zeiss, Germany) to compare the difference between the control and the cells with the internalized App. Results are presented as mean ± SD.
Vacuole Presence Evaluation by Acidic Organelles Labeling
Using Lab Tek chambers internalization assay was performed as mentioned, with positive control E. coli Bl21 (pUCmT::Vat) as Díaz et al. (49) propose. After interaction cultures were washed and incubated for 30 min with fresh DMEM media with Lysotracker Deep Red reagent [75 nm] (Molecular Probes, Eugene, Oregon, USA), after that cells were washed twice with DPBS. Then, samples were fixed and mounted as previously specified.
A. pleuropneumoniae Serine Protease Expression Analysis
Total proteins from App strains 4074, JL03, L20, and AP76, E. coli Bl21 (pUCmT::Vat) and E. coli Bl21 empty vector (positive and negative control, respectively) (49) were extracted by boiling for 3 min with staining buffer and TLCK. Protein concentration was measured by Bradford technique (50). Samples were electrophoresed on an 10% SDS-PAGE gel and transferred to a nitrocellulose membrane. Membrane was blocked with 5% skim milk (Gibco, BRL, Grand Island, NY), then was washed with PBS-Tween 0.1% three times, and incubated over night at 4°C with primary polyclonal antibody anti Vat SPATE [1:1,000] at 4°C, this used to test the Serine Protease presence. Then the membrane was washed three times with PBS-Tween 0.1% (51), and then was incubated with the secondary antibody anti rabbit IgG-HRP [2:10,000] (Molecular Probes, Eugene, Oregon, USA) for 1 h at room temperature. Finally the membrane was revealed with DAB (Sigma Chemical Co., St. Louis, Mo, USA).
Statistical Analysis
All experiments were performed by triplicate. The data was analyzed by ANOVA and two-way ANOVA tests for statistical significance at a P-value of 0.05 using GraphPad Prism version 7.0 (GraphPad Software, San Diego, CA, USA).
Results
Adhesion Assay
Actinobacillus pleuropneumoniae 4074, JL03, L20, and AP76 strain adhesion capacity to endothelial cells was determined. App 4074 shows higher adhesion rates compared with the rest of the serotypes studied, however the number of adhered CFUs decreases with increasing incubation period, contrary to L20 and AP76 which increase their adhesion in relation to incubation time; while JL03 strain shows an irregular pattern by decreasing the amount of adhesion at 1 h compared at 0.5 h and increasing again after 2 (Figure 1). Statical analysis data collected for each strain show that the adhesion difference between strains is not significant.
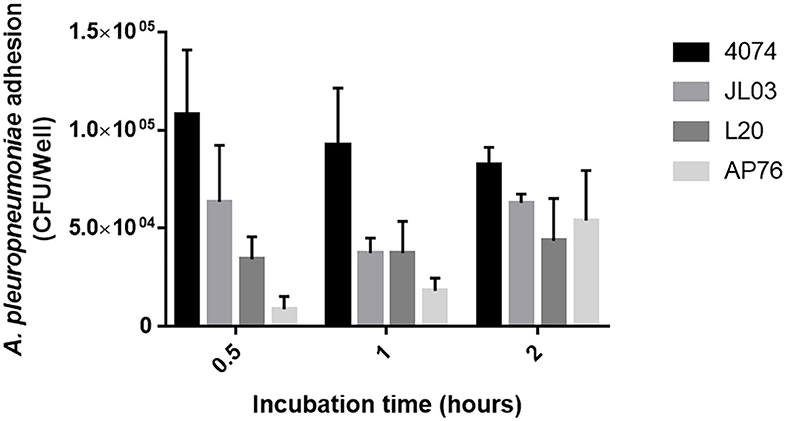
Figure 1. Adherence of A. pleuropneumoniae S4074, JL03, L20, and AP76 to swine aortic endothelial cells observed from 0.5 1 and 2 h of incubation. Error bars represent standard error (SE).
Internalization Assay and Immunofluorescent Labeling
The invasion capacity of A. pleuropneumoniae was investigated. CFU number confirm the ability to enter the host cell of the 4 App strains studied, although the internalization capacity of these strains was relatively low. A. pleuropneumoniae JL03 has a higher percentage of invasion (4.6%), followed by L20 (1.75%), AP76 (1.6%), and 4074 with an invasion of 1.3% (Table 1).
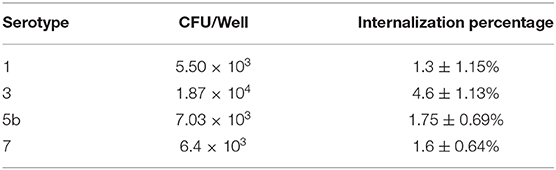
Table 1. Internalization percentage of A. pleuropneumoniae S4074, JL03, L20, and AP76 to swine aortic endothelial cells (MOI 10:1) at 3 h.
Once App's ability to enter the host was observed by CFU counts, the involvement of the actin and tubulin cytoskeleton in the internalization mechanism was analyzed. Figure 2 shows the normal distribution of actin microfilaments inside the endothelial cell, after the incubation of endothelial cells with the 4 App strains, there was no notable changes in actin rearrangement, but the bacteria was observed inside the endothelial cell, some of them housed in the perinuclear area, as well as their co-localization with the actin filaments in some areas. After internalization assay with endothelial cells pre-treated with CD, a well-known actin disruptor, the different App strains were still found inside the host regardless actin cytoskeleton presence.
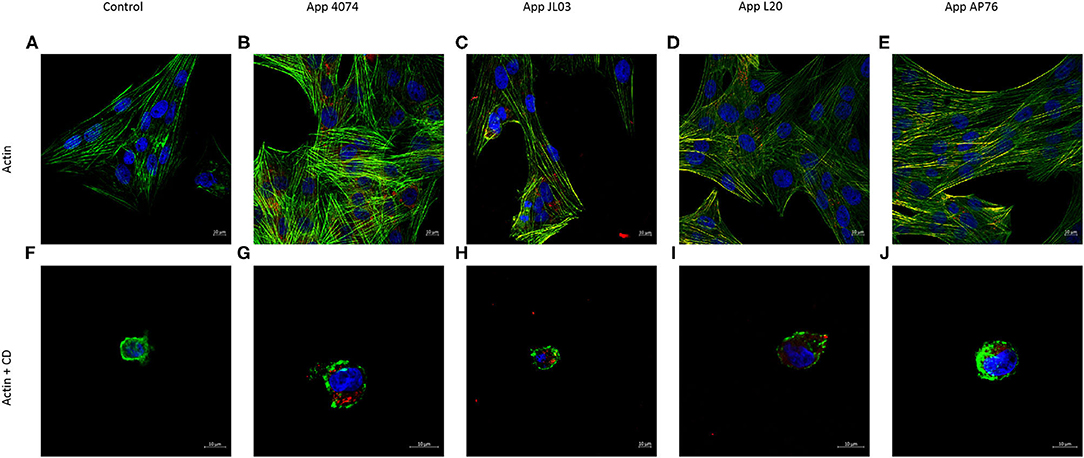
Figure 2. Actin cytoskeleton implication in A. pleuropneumoniae internalization to swine aortic endothelial cells after 3 h of incubation. (A) Actin cytoskeleton of swine endothelial cells stained in green with Alexa Fluor 488 Phalloidin; (B–E) A. pleuropneumoniae internalized in swine endothelial cells, labeled with primary polyclonal antibody, and secondary antibody Alexa Fluor 594 (red); (F) Actin cytoskeleton incubated with CD; (G–J) App internalized and lodge in perinuclear area of swine endothelial cells treated with CD.
The tubulin cytoskeleton participation in the App internalization is shown in Figure 3. Infected cultures showed the 4 App strains inside the endothelial cells, some of them forming clumps in the perinucleus. Bacteria can also was observed spreaded in the cell, inside round intracytoplasmic zones similar to vesicles, as well as in the circumference of these structures showing co-localization with microtubules in these and other areas. In pre-treated cultures with colchicine (tubulin disruptor) App was also observed inside the cells.
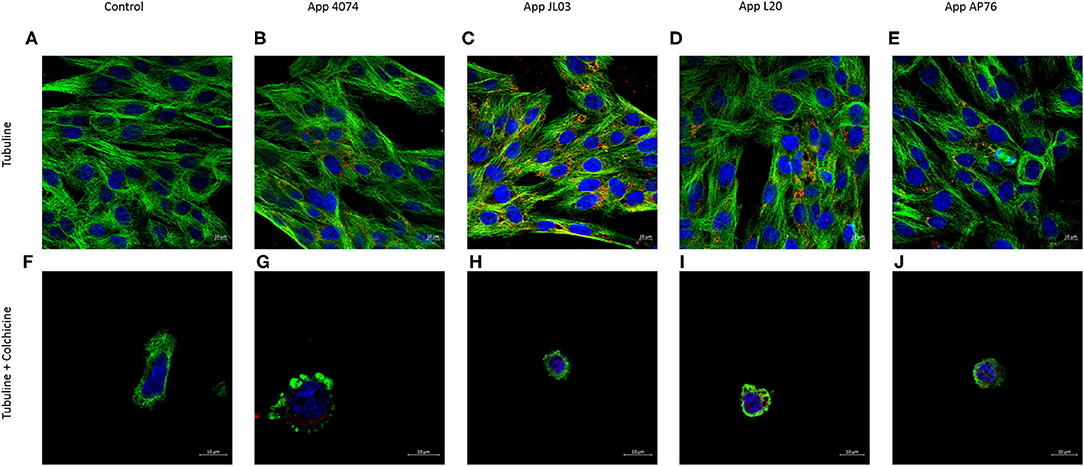
Figure 3. Tubulin cytoskeleton implication in A. pleuropneumoniae internalization to swine aortic endothelial cells after 3 h of incubation. (A) Tubulin cytoskeleton of swine endothelial cells stained in green with primary monoclonal antibody and secondary monoclonal antibody Alexa Fluor 488; (B–E) A. pleuropneumoniae internalized in swine endothelial cells labeled with primary polyclonal antibody and secondary antibody Alexa Fluor 594 (red); (F) Tubulin cytoskeleton treated with colchicine; (G–J) App maintains ability to internalized in endothelial cells treated with colchicine. Nuclei counterstained with Hoechst 33258.
The relationship of internalized A. pleuropneumoniae with organelles of the endothelial cell involved in the transport of substances was investigated. The labeling for endoplasmic reticulum (ER) was showed colocalization with bacteria in some regions, however, no changes in the organelle distribution in the cytoplasm was observed (Figure 4). App also were observed around the nucleus forming clumps, and was detected in round zones similar to vesicles. On the contrary, Golgi Apparatus' distribution was affected with the App internalization in the endothelial cell, showing a perinuclear distribution (Supplementary Figure 1).
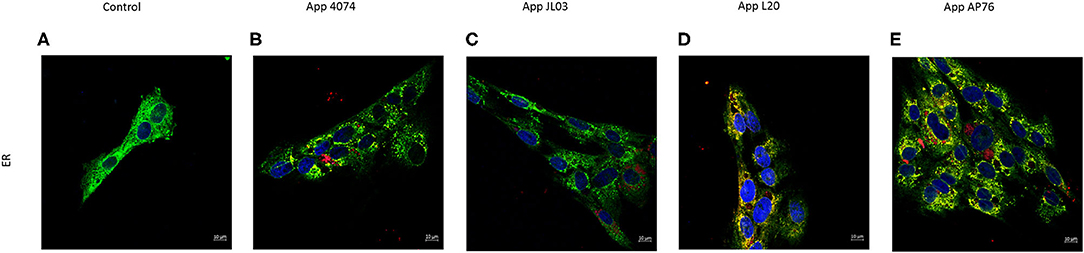
Figure 4. Actinobacillus pleuropneumoniae internalization effect on Endoplasmic Reticulum. (A) Normal distribution of endoplasmic reticulum stained with SelectFX Alexa Fluor 488 endoplasmic reticulum labeling kit (green); (B–E) App (red) internalized into endothelial cells. Co-localization of App and ER is observed however no major ER distribution changes are shown.
Interestingly, an elongation of the nuclei of infected cells was observed in all trial samples. The average length of nuclei at control samples was 14277.063 nm ± 21.85 nm, whilst for the endothelial cells interacted with App 4074 was 18344.528 ± 11.66 nm, 17389.621 ± 16.61 nm for JL03, 18324.956 ± 13.84 nm when L20 was internalized and 18109.861 nm ± 17.41 in the presence of AP76. The differences in lengths of control nuclei compared with the problem samples was statistically significant.
Vacuole Presence Evaluation by Acidic Organelles Labeling
As is shown in Supplementary Figure 3, the Lysotracker labeling results demonstrated that when the endothelial cells were infected with App an increased quantity of acidic vesicles (lysosomes) were produced in comparison with the control. This was observed for App JL03 and App L20, this result was similar to the effect that E. coli Bl21 (pUCmT::Vat) produced. On the contrary, no effect was observed for App 4074 neither for AP76.
A. pleuropneumoniae Serine Protease Expression Analysis
Westernblot results (Supplementary Figure 2) showed the recognition of a protein with E. coli vacuolizing toxin Vat antibody, in A. pleuropneumoniae. All App strains presented a 100 kDa band and other two bands of ~50 and 33 kDa. Negative control (E. coli Bl21 empty vector) only shows one band at 37 kDa whilst positive control E. coli Bl21 (pUCmT::Vat) show several bands including the ones present in App.
Discussion
Endothelium is important in the pathogenesis of many bacteria. Based in a previous unpublished work carried out by this group, endothelium was one of the tissues that showed greater damage in post-mortem lung samples of pigs with swine pleuropneumonia, a finding in concordance with App's proven citotoxicity to endothelial cells (52). Therefore, studying App closely in relation with the endothelium could help better understand its pathogenesis.
As shown in the results obtained here, App strain 4074 had the higher adherence results, simiar to the results obtained for epithelial cells by Auger et al. (6). App JL03 and AP76 strains showed a linear behavior where adhesion increases over time, however the adhesion levels of App 4074 and L20 vary. Perhaps these observations are explainded by a weak affinity of these strains for the endothelium (53), but also due to the nature of endothelial cells, the continue process of pinocytosis that present, bacteria can enter and exit of the cell constantly, as is observed in other more distant bacteria as E. coli and Shigella flexneri (54).
As is known, App can remain in other tissues and organs of asymptomatic pigs, such as tonsils (55). Rare occurrence of App isolated from organs outside the respiratory system without causing sepsis (26, 36), led to analyze if it has the ability to invade host cells and spread to other organs using the endothelium to reach the bloodstream due to its intrinsic relationship as a material exchange barrier and involvement in the pathogenesis of several intracellular pathogens (28, 29, 56–58).
Internalization assay demonstrated low invasion rates for 4074, JL03, L20, and AP76 App strains, suggesting that few CFUs are necessary to cause the disease, however, this may be due to usage of its various virulence factors to infect and persist within the host and resorts to its invasion capacity in a lesser way (18). In a similar work Haemophilus parasuis also presented low invasion to aorta endothelial cells, nevertheless this is attributed by the authors due to possible low tropism to PBMEC cell line and this not being an optimal model for this particular bacteria (59, 60).
S4074 holds the lowest invasion percentage but highest adherence. Serotype 3 is considered a low virulence serotype and does not have high adhesion levels to EC, however shows greater adhesion than serotypes 5b and 7 and the highest invasion rate of all the 4 strains used in this work; evidencing differences in virulence and infection mechanisms between the serotypes.
It is worth to mention that when the interaction was made for 4 h, a considerable amount of detached and rounded EC was observed, mainly in the presence of serotype 5b (L20), followed by serotype 3 (JL03) due to App virulence and endothelial cytotoxicity (52). This results was less observed for serotypes 1 (4074) and 7 (AP76).
Invasion of non-phagocytic cells through rearrangement of the actin cytoskeleton is a common immune evasion mechanism used by most intracellular bacteria. Also, some pathogens modulate host microtubules and endocytic route as invasion mechanism, indicating that this organelle has a fundamental role in the infection process (20, 61).
As mentioned, in many cases the bacteria capable to invade cause a stimulus to the host that generates a rearrangement of the cytoskeleton resulting in the engulfing of the bacteria by non-phagocytic host cells. Therefore, once the internalization capacity of App was checked by CFU (6, 18, 38, 62), the experiments performed to analyze the possible involvement of the actin and tubulin cytoskeleton showed that even when an interaction of the bacterium with both kind of cytoskeleton was observed through co-localization, there was no visible rearrangement of any of them actin or tubulin, after App internalization. In addition at the time of using drugs capable of inhibiting the polymerization of these and therefore losing their function, the bacteria continued appearing inside of endothelial cells, showing that neither actin or tubulin cytoskeleton are necessary for App internalization. The results obtained shows remarkable differences with Haemophilus parasuis, A. suis and Streptococcus suis, whose invasion capacity is greatly diminished when a pretreatment of cytochalasin D is made, demonstrating an actin dependent internalization. On other hand, these differences with App are clear in comparison with H. parasuis and A. suis whose invasion requires also microtubules (53, 59, 60, 63).
The results show all 4 strains scattered inside the host and around to the perinuclear region forming clumps, this behavior is observed in other intracellular bacteria such as Bartonella henselae when invading human endothelial cells and in Burkholderia organism invading respiratory epithelium being accredited the phenomenon to microtubular trafficking (64, 65) the reason some intracellular bacteria use clumps rearrangement is currently unknown.
The tubular endolysosomal network is a system is in charge of bringing internalized molecules to their intracellular destination and is involved in nutrition processes, various cellular functions and maintaining cellular homeostasis. Due to their importance, opportunistic pathogens highjack these protein sorting pathways which allows them to internalize and replicate within the host cell avoiding degradation. In some cases, bacteria reside within vacuoles producing proteins that are transported by these sorting pathways, interfering with the function of endogenous regulators and endosomal substance trafficking systems (66).
The group A Streptococcus use LC3-associated phagocytosis (LAP) and enter the cell through vacuoles. After internalization pore-forming toxins damage the endosomal membrane allowing bacteria to escape into the cytoplasm where they induce reactive oxygen species (ROS), LAP formation and blocks ubiquitination inhibiting autophagy maintaining a neutral pH inside the autophagosome structure allowing cell proliferation (67). It is suspected a similar mechanism in App due to the round zones similar to vesicles observed in Figures 2, 3, showing bacteria inside them and at the circumference of the aforementioned structures, which indicate a possible form of internalization of the bacteria and transport method inside the host. App capacity to form lysosomes inside EC was analyzed. After 3 h interaction an increase of acidic organelles presence is seen in the case of App JL03 and L20 whereas for strains 4074 and AP76 shows basal lysosomal function, compared to negative control (EC without bacteria) implying a possible vacuolization function. Results where compared using as positive control E. coli Bl21 pUCmT::Vat a mutant that over expresses the characterized vacuolizing toxin Vat which induces vacuole formation and changes in permeability in epithelial cells (49). The increased number of acidic organelles in EC interacted with App imply the vacuolizing function for App leading to think a probable protein similar to Vat, present in App.
Polyclonal antibody against Vat SPATE toxins shows affinity to App protein extract indicating recognition to conserved epitopes of SPATEs (51). Westernblot results showed a band at approximately 100 kDa presumably App Subtisilin-like autotransporter protein AasP (104 kDa) that has homology with E. coli autotransporter proteins' binding site (68). Autotransporter proteins containing a serine protease motive are in volved in diverse functions like adhesion, colonization, cell host invasion, biofilm formation, and toxicity. AasP protein universally expressed in App is autocatalytic and has a maturation protease function. It shows homology to surface-localized autotransporters from various bacterial pathogens including Mannheimia haemolytica, Bordetella pertussis, and Neisseria meningitidis; suggesting a possible role in adhesion, colonization, internalization, and persistence in App (68, 69) although recombinant AasP antigen did not affected App virulence and did not protected pigs from App's colonization and infection (70). The other two bands recognized by anti-Vat antibody possibly indicate expression of more than one serine protease autotransporter or the presence of OmlA wich is maturated and released in the presence of AasP and is seen in SDS-PAGE gels as band of 50 kDa and a band of 33 kDa (69). Whether Aasp is responsible of inducing vacuole formation and has an active role in App invasion is yet to be determined.
Even when the true nature of the aforementioned intracytoplasmic structures similar to vesicles is unknown, they confirm the presence of App within the endothelial cytoplasm. Further investigation should be performed in order to elucidate the true nature of these structures.
Since intracellular pathogens exploit host cell signaling pathways to facilitate their uptake and survival within host cells (71, 72) and cytoskeleton does not seem to have a key role in App internalization; the possibility of bacteria entering the host through some other type of mechanism was analyzed. Therefore, the involvement of other cellular organelles with substance transport function was studied.
In cases such as reported for Salmonellae bacteria reach the perinuclear area an interacts with the host signaling pathways in order to reach the Golgi Apparatus where the intracellular bacterial replication begins (73), in Bordetella bacilliformis case if the Golgi Apparatus is disrupted invasion in highly diminished (64) showing a very important role in these bacteria invasion mechanisms. As seen in Figure 4 App continues to be observed within the endothelial cell. Golgi Apparatus normal distribution is altered and can be seen located in a predominant perinuclear area. Endoplasmic reticulum distribution is not disturbed by the presence of any of the 4 App strains, however we can find co-localization in some regions indicating a possible interaction between the organelle and the bacteria. ER is used by bacteria as a niche inside the host cell that ensures biosynthesis of nutrients such as lipids, carbohydrates and proteins and a safe place ideal to niche intracellularly (74). Whether direct or indirectly, an affection to these organelles is generated in the presence of intracellular App. How App interacts and relates to cellular organelles is yet to be known.
Figures 2–4 show in many areas enlarged nuclei on the endothelial cells when interaction with App occurs, this phenomenon has been previously reported in PAMs apoptosis induced by App (75), suggesting that the bacterium is interacting and causing morphological changes in them.
Many Pasteurellaceae organisms are identified as invasive pathogens but machanisms involved are not fully understood. With this work we state that Actinobacillus pleuropneumoniae is able to internalize in endothelial cells and could possibly be considered as an intracellular pathogen.
Although App can be found inside the cell and making apparent interactions with various organelles it is still unknown the specific route or mechanism it uses to achieve invasion. It is worth mentioning strains JL03 and L20 (serotypes 3 and 5b) show greater virulence against EC by presenting the highest adherence and internalization percentages and greater presence of acidic organelles after infection.
App might possess virulence factors enabling to achieve invasion through autophagy induction and transport through vesicles or it might benefit from cellular molecule exchange mechanisms, such as pinocytosis and caveolins, as an in and out way to host cell (21).
Further experiments must be performed in order to comprehend and elucidate Actinobacillus pleuropneumoniae invasion mechanism and test intracellular survival. Fully understanding of its pathogenesis will help developed better measures and diagnostic tools to prevent outbreaks of A. pleuropneumoniae.
Data Availability Statement
The raw data supporting the conclusions of this article will be made available by the authors, without undue reservation.
Author Contributions
BP-M and AG-B conceived the main research idea and designed the methodology. Experiments where performed by BP-M with support from AM-F. MD, FA-G, and MJ helped with the statistical analysis of the data. AG-B supervised the project. BP-M took the lead in writing the manuscript. All authors provided critical feedback and helped shape the research, analysis, and manuscript.
Funding
This work was funded through the support of CONACyT scholarship (602733) and also was supported by the program of Becas Mixtas, CONACYT. This project was also supported by Universidad Autonoma de Aguascalientes, Mexico Award Number: PIBT16-3 to AG-B. The project was supported by Special Resource UAA for Research 2017 and 2018.
Conflict of Interest
The authors declare that the research was conducted in the absence of any commercial or financial relationships that could be construed as a potential conflict of interest.
Acknowledgments
We thank Fabiola Galindo and Juan Manuel Diaz for their technical assistance and collaboration in this work as well we acknowledge the support provided by Karen Sánchez with animal handling.
Supplementary Material
The Supplementary Material for this article can be found online at: https://www.frontiersin.org/articles/10.3389/fvets.2020.569370/full#supplementary-material
Supplementary Figure 1. Actinobacillus pleuropneumoniae internalization effect on Golgi Apparatus. (A) Golgi Apparatus (red) normal distribution in swine endothelial cell, (B–E) Golgi Apparatus distribution is altered after App entry, migrating into a perinuclear position.
Supplementary Figure 2. Recognition of App total proteins with antibody anti- vacuolizing toxin Vat (100 kDa). (1) Molecular weight marker, (2) E. coli Bl21 empty vector total proteins (negative control), (3) E. coli Bl21 pUCmT::Vat total proteins (positive control), (4) App 4074 total proteins, (5) App JL03 total proteins (6) App L20 total proteins, (7) App AP76 total proteins. All App samples show a band at 100 kDa, one between 52 and 37 kDa and another one between 37 and 25 kDa. Positive control shows same bands as App whilst negative control only has one band at 37 kDa.
Supplementary Figure 3. Acidic organelles formation after internalization assay. (A) Endothelial cells without bacteria, (B) Endothelial cells + E. coli Bl21 pUCmT::Vat, (C) Endothelial cells + App 4074, (D) Endothelial cells + App JL03, (E) Endothelial cells + App L20, (F) Endothelial cells + App AP76. Endothelial cells interacted with each bacterial strain for 3 h followed by labeling for 30 min with Lysotracker Deep Red [75 nm]. A remarkable increased of acidic organelles is seen when interacted with E. coli and with App JL03 and L20 but no notable difference between negative control and cells interacted with App 4074 and AP76.
References
1. Hathroubi S, Beaudry F, Provost C, Martelet L, Segura M, Gagnon C, et al. Impact of Actinobacillus pleuropneumoniae biofilm mode of growth on the lipid a structures and stimulation of immune cells. Innate Immunity. (2016) 22:353–62. doi: 10.1177/1753425916649676
2. Hsu C, Li S, Chang N, Chen Z, Liao J, Chen T, et al. Involvement of Nf-κB in regulation of Actinobacillus pleuropneumoniae exotoxin ApxI-induced proinflammatory cytokine production in porcine alveolar macrophages. Vet Microbiol. (2016) 195:128–35. doi: 10.1016/j.vetmic.2016.09.020
3. Williams J, Torres-León M, Echeverria-Coelo P, Matos-Medina M. Aislamiento e identificación de Actinobacillus pleuropneumoniae en pulmones de cerdos con pleuroneumonía crónica sacrificados en el rastro municipal de Mérida, Yucatán, México. Rev Biomed. (2000) 11:175–81. doi: 10.32776/revbiomed.v11i3.234
4. Frey J. Detection, identification, and subtyping of actinobacillus pleuropneumoniae. PCR detection of microbial pathogens. Methods Mol Biol. (2003) 216:87–95. doi: 10.1385/1-59259-344-5:87
5. Enriquez-Verdugo I, Guerrero AL, Serrano JJ, Godinez D, Rosales JL, Tenorio V, et al. Adherence of Actinobacillus pleuropneumoniae to swine-lung collagen. Microbiology-Sgm. (2004) 150:2391–400. doi: 10.1099/mic.0.27053-0
6. Auger E, Deslandes V, Ramjeet M, Contreras I, Nash JHE, Harel J, et al. Host-pathogen interactions of Actinobacillus pleuropneumoniae with porcine lung and tracheal epithelial cells. Infect Immun. (2009) 77:1426–41. doi: 10.1128/IAI.00297-08
7. Gottschalk M, Lacouture S. Canada: distribution of Streptococcus suis (from 2012 to 2014) and Actinobacillus pleuropneumoniae (from 2011 to 2014) serotypes isolated from diseased pigs. Can Vet J. (2015) 56:1093–4.
8. Bossé J, Li Y, Sárközi R, Fodor L, Lacouture S, Gottschalk M, et al. Proposal of serovars 17 and 18 of Actinobacillus pleuropneumoniae based on serological and genotypic analysis. Vet Microbiol. (2018) 217:1–6. doi: 10.1016/j.vetmic.2018.02.019
9. Paradis MA, Vessie GH, Merrill JK, Dick CP, Moore C, Charbonneau G, et al. Efficacy of tilmicosin in the control of experimentally induced Actinobacillus pleuropneumoniae infection in Swine. Can J Vet Res. (2004) 68:7–11.
10. Fittipaldi N, Klopfenstein C, Gottschalk M, Broes A, Paradis MA, Dick CP. Assessment of the efficacy of tilmicosin phosphate to eliminate Actinobacillus pleuropneumoniae from carrier pigs. Can J Vet Res. (2005) 69:146–50.
11. Antenucci F, Fougeroux C, Deeney A, Ørskov C, Rycroft A, Holst P, et al. In vivo testing of novel vaccine prototypes against Actinobacillus pleuropneumoniae. Vet Res. (2018) 49:1. doi: 10.1186/s13567-017-0502-x
12. Loera-Muro A, Avelar-González FJ, Loera-Muro VM, Jacques M, Guerrero-Barrera AL. Presence of Actinobacillus pleuropneumoniae, Streptococcus suis, Pasteurella multocida, Bordetella bronchiseptica, Haemophilus parasuis and Mycoplasma hyopneumoniae in upper respiratory tract of swine in farms from aguascalientes, Mexico. Open J Anim Sci. (2013) 3:132–7. doi: 10.4236/ojas.2013.32020
13. Loera-Muro VM, Loera-Muro A, Morfín-Mata M, Jacques M, Avelar-González FJ, Ramírez-Castillo F, et al. Porcine respiratory pathogens in swine farms environment in Mexico. Open J Anim Sci. (2014) 4:196–205. doi: 10.4236/ojas.2014.44025
14. Li Y, Cao S, Zhang L, Lau G, Wen Y, Wu R, et al. A TolC-like protein of Actinobacillus pleuropneumoniae is involved in antibiotic resistance and biofilm formation. Front Microbiol. (2016) 7:618. doi: 10.3389/fmicb.2016.01618
15. Sassu E, Ladinig A, Talker S, Stadler M, Knecht C, Stein H, et al. Frecuency of Th17 cells correlates with the presence of lung lesions in pigs chronically infected with Actinobacillus pleuropneumoniae. Vet Res. (2017) 48:4. doi: 10.1186/s13567-017-0411-z
16. Dreyfus A, Schaller A, Nivollet S, Segers R, Kobisch M, Mieli L, et al. Use of recombinant Apxiv in serodiagnosis of Actinobacillus pleuropneumoniae infections, development and prevalidation of the ApxIV ELISA. Vet Microbiol. (2004) 99:227–38. doi: 10.1016/j.vetmic.2004.01.004
17. Sassu E, Bossé J, Tobias T, Gottschalk M, Langford P, Henning-Pauka I. Update on Actinobacillus pleuropneumoniae knowledge, gaps and challenges. Transbound Emerg Dis. (2017) 65:72–90. doi: 10.1111/tbed.12739
18. Chiers K, De Waele T, Pasmas F, Ducatelle R, Haesebrouck F. virulence factors of Actinobacillus pleuropneumoniae involved in colonization, persistence and induction of lesions in its porcine host. Vet Res. (2010) 41:65. doi: 10.1051/vetres/2010037
19. Brogaard L, Klitgaard K, Heegaard P, Hansen M, Jensen T, Skovgaard K. Concurrent host-pathogen gene expression in the lungs of pigs challenged with Actinobacillus pleuropneumoniae. BMC Genomics. (2015) 16:417. doi: 10.1186/s12864-015-1557-6
20. Pizarro-Cerdá J, Cossart P. Bacterial adhesion and entry into host cells. Cell. (2006) 124:715–27. doi: 10.1016/j.cell.2006.02.012
21. St Geme J. Molecular and cellular determinants of non-typeable Haemophilus influenzae adherence and invasion. Cell Microbiol. (2002) 4:191–200. doi: 10.1046/j.1462-5822.2002.00180.x
22. Ojha S, Lacouture S, Gottschalk M, MacInnes J. characterization of colonization-deficient mutants of Actinobacillus suis. Vet Microbiol. (2010) 140:122–30. doi: 10.1016/j.vetmic.2009.07.014
23. Bercier P, Gottschalk M, Grenier D. Effects of Actinobacillus pleuropneumoniae on barrier function and inflammatory response of pig tracheal epithelial cells. Pathog Dis. (2019) 77:fty079. doi: 10.1093/femspd/fty079
24. Knudsen L, Ochs M. The micromechanics of lung alveoli: structure and function of surfactant and tissue components. Histochem Cell Biol. (2018) 150:661–76. doi: 10.1007/s00418-018-1747-9
25. Millar FR, Summers C, Griffiths MJ, Toshner MR, Proudfoot AG. The pulmonary endothelium in acute respiratory distress syndrome: insights and therapeutic opportunities. Thorax. (2016) 71:462–73. doi: 10.1136/thoraxjnl-2015-207461
26. Lubkin A, Torres VJ. Bacteria and endothelial cells: a toxic relationship. Curr Opin Microbiol. (2017) 35:58–63. doi: 10.1016/j.mib.2016.11.008
27. Valbuena G, Walker DH. The endothelium as a target for infections. Ann Rev Pathol. (2006) 1:171–98. doi: 10.1146/annurev.pathol.1.110304.100031
28. Michiels C. Endothelial cell functions. J Cell Physiol. (2003) 196:430–43. doi: 10.1002/jcp.10333
29. Rajendran P, Rengarajan T, Thangavel J, Nishigaki Y, Sakthisekaran D, Sethi G, et al. The vascular endothelium and human diseases. Int J Biol Sci. (2013) 9:1057–69. doi: 10.7150/ijbs.7502
30. Weidensdorfer M, Chae J, Makobe C, Stahl J, Averhoff B, Müller V, et al. Analysis of endothelial adherence of Bartonella henselae and Acinetobacter baumannii using a dynamic humanex vivo infection model. Infect Immun. (2015) 84:711–22. doi: 10.1128/IAI.01502-15
31. Gonzales J, Verin A. Pulmonary vascular endothelial cells. In: Endothelial Dysfunction - Old Concepts and New Challenges. (2018). doi: 10.5772/intechopen.76995
32. Letsiou E, Bauer N. Endothelial extracellular vesicles in pulmonary function and disease. Curr Topics Membranes. (2018) 82:197–256. doi: 10.1016/bs.ctm.2018.09.002
33. Lemichez E, Lecuit M, Nassif X, Bourdoulous S. Breaking the wall: targeting of the endothelium by pathogenic bacteria. Nat Rev Microbiol. (2009) 8:93–104. doi: 10.1038/nrmicro2269
34. Salo J, Pietikäinen A, Söderström M, Auvinen K, Salmi M, Ebady R, et al. Flow-tolerant adhesion of a bacterial pathogen to human endothelial cells through interaction with biglycan. J Infect Dis. (2016) 213:1623–31. doi: 10.1093/infdis/jiw003
35. Pedersen R, Grønnemose R, Stærk K, Asferg C, Andersen T, Kolmos H, et al. A method for quantification of epithelium colonization capacity by pathogenic bacteria. Front Cell Infect Microbiol. (2018) 8:16. doi: 10.3389/fcimb.2018.00016
36. Hoeltig D, Rohde J, Frase R, Nietfeld F, Waldmann K, Valentin-Weigand P, et al. Multi-organ spreading of Actinobacillus pleuropneumoniae serovar 7 in weaned pigs during the first week after experimental infection. Vet Res. (2018) 49:97. doi: 10.1186/s13567-018-0592-0
37. Burciaga-Nava JA, Reyes-Romero MA, Avelar-González FJ, Guerrero-barrera AL. Establishment and characterization of porcine aortic endothelial cell cultures with prolonged replicative lifespan by a non-enzymatic method. In vitro Cell Dev Biol Anim. (2009) 45:15–8. doi: 10.1007/s11626-008-9146-5
38. Letourneau J, Lévesque C, Berthiaume F, Mourez M, Jacques M. In vitro assay of bacterial adhesion onto mammalian epithelial cells. J Visual Exp. (2011) 51:2783. doi: 10.3791/2783
39. Lévesque C, Provost C, Labrie J, Hernandez-Reyes Y, Burciaga-Nava J, Gagnon C, et al. Actinobacillus pleuropneumoniae possesses an antiviral activity against porcine reproductive and respiratory syndrome virus. PLoS ONE. (2014) 9:e98434. doi: 10.1371/journal.pone.0098434
40. Hamad MA, Skeldon AM, Valvano MA. Construction of Aminoglycoside-sensitive Burkholderia cenocepacia strains for use in studies of intracellular bacteria with the gentamicin protection assay. Appl Environ Microbiol. (2010) 76:3170–6. doi: 10.1128/AEM.03024-09
41. Edwards AM, Massey RC. Invasion of human cells by a bacterial pathogen. J Visual. (2011) 49:2693. doi: 10.3791/2693
42. Burns J, Jonas M, Chi E, Clark D, Berger A, Griffith A. Invasion of respiratory epithelial cells by Burkholderia (pseudomonas) cepacia. Infect Immun. (1996) 64:4054–9. doi: 10.1128/IAI.64.10.4054-4059.1996
43. Jamur MC, Oliver C. Permeabilization of cell membranes. Immunocytochem Methods Protocols. (2010) 588:63–6. doi: 10.1007/978-1-59745-324-0_9
44. Mammadzada P, Gudmundsson J, Kvanta A, Andre H. Differential hypoxic response of human choroidal and retinal endothelial cells proposes tissue heterogeneity of ocular angiogenesis. Acta Ophthalmol. (2016) 94:805–14. doi: 10.1111/j.1755-3768.2016.0399
45. Risinger AL, Westbrook CD, Encinas A, Mülbaier M, Schultes CM, Wawro S, et al. ELR510444, a novel microtubule disruptor with multiple mechanisms of action. J Pharmacol Exp Ther. (2011) 336:652–60. doi: 10.1124/jpet.110.175331
46. Gao M, Wu S, Ji J, Zhang J, Liu Q, Yue Y, et al. The influence of actin depolymerization induced by cytochalasin d and mechanical stretch on interleukin-8 expression and jnk phosphorylation levels in human retinal pigment epithelial cells. BMC Ophthalmol. (2017) 17:43. doi: 10.1186/s12886-017-0437-z
47. Weber A, Iturri J, Benitez R, Zemljic-Jokhadar S, Toca-Herrera J. Microtubule disruption changes endothelial cell mechanics and adhesion. Sci Rep. (2019) 9:14903. doi: 10.1038/s41598-019-51024-z
48. Guerrero-Barrera A, de la Garza M, Mondragón R, García-Cuéllar C, Segura-Nieto M. Actin-related proteins in Actinobacillus pleuropneumoniae and their interactions with actin-binding proteins. Microbiology. (1999) 145:3235–44. doi: 10.1099/00221287-145-11-3235
49. Díaz J, Dozois C, Avelar-González F, Hernández-Cuellar E, Pokharel P, de Santiago A, et al. The vacuolating autotransporter toxin (vat) of Escherichia coli causes cell cytoskeleton changes and produces non-lysosomal vacuole formation in bladder epithelial cells. Front Cell Infect Microbiol. (2020) 10:299. doi: 10.3389/fcimb.2020.00299
50. Hammond J, Kruger N. The bradford method for protein quantitation. N Protein Tech. (1988) 126:25–32. doi: 10.1385/0-89603-126-8:25
51. Pokharel P, Díaz J, Bessaiah H, Houle S, Guerrero-Barrera A, Dozois C. The serine protease autotransporters tagb, tagc, and sha from extraintestinal pathogenic Escherichia coli are internalized by human bladder epithelial cells and cause actin cytoskeletal disruption. Int J Mol Sci. (2020) 21:3047. doi: 10.3390/ijms21093047
52. Serebrin S, Rosendal S, Valdivieso-Garcia A, Little P. Endothelial cytotoxicity of Actinobacillus pleuropneumoniae. Res Vet Sci. (1991) 50:18–22. doi: 10.1016/0034-5288(91)90047-R
53. Vanier G, Szczotka A, Friedl P, Lacouture S, Jacques M, Gottschalk M. Haemophilus parasuis invades porcine brain microvascular endothelial cells. Microbiology. (2006) 152:135–42. doi: 10.1099/mic.0.28312-0
54. Philpott D, Edgeworth J, Sansonetti P. The pathogenesis of shigella flexneri infection: lessons from in vitro and in vivo studies. Phil Trans R Soc Lond B. (2000) 355:575–86. doi: 10.1098/rstb.2000.0599
55. Kalita A, Hu J, Torres AG. Recent advances in adherence and invasion of pathogenic Escherichia coli. Curr Opin Infect Dis. (2014) 27:459–64. doi: 10.1097/QCO.0000000000000092
56. Langford P, Loynds B, Kroll S. Cloning and molecular characterization of cu,zn superoxide dismutase from Actinobacillus pleuropneumoniae. Infect Immun. (1996) 64:5035–41. doi: 10.1128/IAI.64.12.5035-5041.1996
57. Sheehan BJ, Langford PR, Rycroft AN, Kroll JS. [Cu,Zn]-Superoxide dismutase mutants of the swine pathogen Actinobacillus pleuropneumoniae are unattenuated in infections of the natural host. Infect Immun. (2000) 68:4778–81. doi: 10.1128/IAI.68.8.4778-4781.2000
58. Bossé J, Janson H, Sheehan B, Beddek A, Rycroft A, Simon Kroll J, et al. Actinobacillus pleuropneumoniae: pathobiology and pathogenesis of infection. Microbes Infect. (2002) 4:225–35. doi: 10.1016/S1286-4579(01)01534-9
59. Frandoloso R, Pivato M, Martínez-Martínez S, Rodríguez-Ferri E, Kreutz L, Martín, et al. Differences in Haemophilus parasuis adherence to and invasion of AOC-45 porcine aorta endothelial cells. BMC Vet Sci. (2013) 9:207. doi: 10.1186/1746-6148-9-207
60. Aragon V, Bouchet B, Gottschalk M. Invasion of endothelial cells by systemic and nasal strains of Haemophilus parasuis. Vet J. (2010) 186:264–7. doi: 10.1016/j.tvjl.2009.08.013
61. Sana T, Baumann C, Merdes A, Soscia C, Rattei T, Hachani A, et al. Internalization of Pseudomonas aeruginosa strain pao1 into epithelial cells is promoted by interaction of a t6ss effector with the microtubule network. mBio. (2015) 6:e00712. doi: 10.1128/mBio.00712-15
62. Kuehn M, Kesty N. Bacterial outer membrane vesicles and the host-pathogen interaction. Genes Dev. (2005) 19:2645–55. doi: 10.1101/gad.1299905
63. Vanier G, Segura M, Friedl P, Lacouture S, Gottschalk M. Invasion of porcine brain microvascular endothelial cells by Streptococcus suis serotype 2. Infect Immun. (2004) 72:1441–9. doi: 10.1128/IAI.72.3.1441-1449.2004
64. Verma A, Davis G, Ihler G. Infection of human endothelial cells with bartonella bacilliformis is dependent on rho and results in activation of rho. Infect Immun. (2000) 68:5960–9. doi: 10.1128/IAI.68.10.5960-5969.2000
65. Taylor J, Hogue L, LiPuma J, Walter M, Brody S, Cannon C. Entry of Burkholderia organisms into respiratory epithelium: cftr, microfilament and microtubule ependence. J Cystic Fibr. (2010) 9:36–43. doi: 10.1016/j.jcf.2009.10.002
66. Wang J, Fedoseienko A, Chen B, Burstein E, Jia D, Billadeau D. Endosomal receptor trafficking: retromer and beyond. Traffic. (2018) 19:578–90. doi: 10.1111/tra.12574
67. Cheng Y, Kuo C, Lu S, Hiroko O, Wu Y, Hsieh C, et al. Group a streptococcus induces laposomes via SLO/β1 Integrin/NOX2/ROS pathway in endothelial cells that are ineffective in bacterial killing and suppress xenophagy. mBio. (2019) 10:e02148–19. doi: 10.1128/mBio.02148-19
68. Baltes N, Buettner F, Gerlach G. selective capture of transcribed sequences (scots) of Actinobacillus pleuropneumoniae in the chronic stage of disease reveals an hlyx-regulated autotransporter protein. Vet Microbiol. (2007) 123:110–21. doi: 10.1016/j.vetmic.2007.03.026
69. Ali T, Oldfield N, Wooldridge K, Turner D, Ala'Aldeen D. Functional characterization of aasp, a maturation protease autotransporter protein of Actinobacillus pleuropneumoniae. Infect Immun. (2008) 76:5608–14. doi: 10.1128/IAI.00085-08
70. Oldfield N, Worrall K, Rycroft A, Ali T, Wooldridge K, Ala'Aldeen, et al. Aasp autotransporter protein of Actinobacillus pleuropneumoniae does not protect pigs against homologous challenge. Vaccine. (2009) 27:5278–83. doi: 10.1016/j.vaccine.2009.06.047
71. Pierini R, Cottam E, Roberts R, Wileman T. Modulation of membrane traffic between endoplasmic reticulum, ergic and golgi to generate compartments for the replication of bacteria and viruses. Semin Cell Dev Biol. (2009) 20:828–33. doi: 10.1016/j.semcdb.2009.03.015
72. Choi S, Maeng Y, Kim T, Lee Y, Kim Y, Kim E. Lysosomal trafficking of tgfbip via caveolae-mediated endocytosis. PLoS ONE. (2015) 10:e0119561. doi: 10.1371/journal.pone.0119561
73. McGhie E, Brawn L, Hume P, Humphreys D, Koronakis V. Salmonella takes control: effector-driven manipulation of the host. Curr Opin Microbiol. (2009) 12:117–24. doi: 10.1016/j.mib.2008.12.001
74. Celli J, Tsolis R. Bacteria, the ER and the unfolded protein response: friends or foes? Nat Rev Microbiol. (2015) 132:71–82. doi: 10.1038/nrmicro3393
Keywords: Actinobacillus pleuropeumoniae, cytoskeleton, bacterial cell adhesion, bacterial cell internalization, endothelial cell
Citation: Plasencia-Muñoz B, Avelar-González FJ, De la Garza M, Jacques M, Moreno-Flores A and Guerrero-Barrera AL (2020) Actinobacillus pleuropneumoniae Interaction With Swine Endothelial Cells. Front. Vet. Sci. 7:569370. doi: 10.3389/fvets.2020.569370
Received: 03 June 2020; Accepted: 28 September 2020;
Published: 29 October 2020.
Edited by:
Maureen T. Long, University of Florida, United StatesReviewed by:
Adina R. Bujold, University of Alberta, CanadaJanet MacInnes, University of Guelph, Canada
Copyright © 2020 Plasencia-Muñoz, Avelar-González, De la Garza, Jacques, Moreno-Flores and Guerrero-Barrera. This is an open-access article distributed under the terms of the Creative Commons Attribution License (CC BY). The use, distribution or reproduction in other forums is permitted, provided the original author(s) and the copyright owner(s) are credited and that the original publication in this journal is cited, in accordance with accepted academic practice. No use, distribution or reproduction is permitted which does not comply with these terms.
*Correspondence: Alma L. Guerrero-Barrera, alguerre@correo.uaa.mx