- Center for Plant Nutrition and Environmental Research Hanninghof, Yara International, Dülmen, Germany
Calcium (Ca2+) is an important macronutrient in coffee and is involved in several physiological processes that influence crop growth, development, productivity, and stress response. This paper presents results from five experiments conducted on coffee under greenhouse and field conditions for over 5 years (2014–2018). The main objective of this study was to evaluate the influence of Ca+2 application on coffee growth, development, abiotic stress response, cation uptake, leaf cell structure, and productivity. The results show that Ca+2 directly influences the growth and development of plants and has a strong effect on root growth. Drought stress and low Ca+2 rates of 8 mg.L−1 showed no differences in photosynthetic rates (PN) and biomass accumulation; high Ca+2 rates between 75 and 150 mg.L−1 increased PN and biomass accumulation in plants under drought stress, with a positive correlation between Ca+2 content in the leaves and PN with and without drought stress. High air temperature (>30°C) reduced PN rates, and the treatment with proper Ca+2 application showed better PN compared to the treatments with low Ca+2. Ca+2 application showed a synergistic effect with potassium (K+) uptake and no influence on the magnesium (Mg+2) uptake but a reduction in the leaf concentration with the increase in Ca+2 application. Additionally, coffee plants with proper Ca+2 application showed thicker leaves, denser epidermis, and larger, more compact, and better-structured palisade parenchyma compared with the plants treated with Ca+2 at lower rates. After 5 years, the mean coffee yield showed a polynomial response with respect to the doses of Ca+2 applied, with optimum rate of 120 kg CaO ha−1year−1 and a peak of Ca+2 uptake by the coffee cherries during 110–220 days after flowering.
Introduction
Coffee is the second-most-traded commodity around the world (Talbot, 2004). The coffee crop provides livelihood to 20–25 million smallholder coffee farmers in 70 countries, and nearly 100 million people are involved in the production and processing of green coffee (Watts, 2016; Panhuysen and Pierrot, 2018). There is strong evidence that the climatic conditions in the coffee regions have been changing and that rising temperatures and altered rainfall patterns already affect coffee yields, quality, pests, and diseases (Bunn et al., 2015a,b; Craparo et al., 2015; Pérez-Rendón et al., 2016; Pham et al., 2019), with a strong impact on the economic and social sustainability of coffee. The regions most affected are those away from the equator and further up in the mountains (Bunn et al., 2015a). In addition, coffee production will most likely come into conflict with other land uses, including forests (Watts, 2016), which will further increase the risk of social imbalance in the coffee-growing regions. Coffee has 103 species (Davis et al., 2006); however, the most planted coffee varieties in the world are Coffea arabica L. (Arabica coffee) and C. canephora Pierre (Robusta coffee). Arabica coffee is well-known for its high cup quality and grows better in mean air temperatures between 18° and 23°. However, the bean and beverage quality will lessen when mean air temperatures increase beyond that level, and reductions in water supply occur (Bertrand et al., 2012; Watts, 2016; DaMatta et al., 2019); which strongly impacts farmer's income and the sustainability of the coffee crop, especially in countries such as Brazil, Colombia, Honduras, Mexico, Guatemala, Kenya, and Ethiopia.
The growth of the Robusta coffee variety is better adapted to higher air temperatures (24–30°C) (DaMatta et al., 2007), with optimal conditions between 22 and 26°C (Ferrão et al., 2012), but has a lower cup quality and lower value than Arabica coffee. However, an abrupt increase in air temperature and more prolonged drought periods can drastically reduce plant growth and productivity (Praxedes et al., 2006), which in turn affects the livelihood of a large number of farmers, especially in countries like Vietnam, Brazil, Uganda, India, and Indonesia.
Drought stress and heat stress are the two main sources of abiotic stress in coffee worldwide. Tailored adaptative strategies based on improved crop management, including nutrition, can increase the resilience capacity of coffee trees grown in the affected regions. In terms of stress, Ca+2 improves the resistance of the plants to stress conditions in two ways: (a) maintenance of the cell membrane and cell wall structure, and (b) acts as an intracellular messenger in the cytosol; which signals the effects of external factors at the cellular level and induces metabolic changes in the cells (White and Broadley, 2003). Ca+2 has unique properties and a universal ability to transmit diverse signals that trigger primary physiological actions in the cell in response to hormones, pathogens, and stress factors. Ca2+ in the cells has been recognized as the universal secondary messenger (Felle, 2001; White and Broadley, 2003; McAinsh and Pittman, 2009; Vanneste and Friml, 2013; van Bel et al., 2014; Choi et al., 2016; Gilroy et al., 2016), which is demonstrated by its involvement in multiple essential cellular processes, including cell division, cell growth/shrinkage, secretion, transcriptional regulation, cellular polarity, stomatal aperture regulation, response to light, response to biotic and abiotic stress, immunity, and response at multiple plant hormones (White and Broadley, 2003; Vanneste and Friml, 2013; Khushboo et al., 2018).
Some researchers suggested that Ca+2 might be involved in osmotic adjustment by increasing the soluble sugar and proline content in plants under drought stress (Jaleel et al., 2007; Khushboo et al., 2018). The Ca+2 ions bind to a calcium-modulate protein (calmodulin) at a minimum of four sites, forming a Ca+2-calmodulin complex. This complex activates numerous target proteins involved in a variety of cell processes, such as mediating plant responses to abiotic stress with the help of secondary messengers (Silva et al., 2011; Waraich and Ahmad, 2011). According to Tuteja and Mahajan (2007), a generic signaling transduction pathway follows four steps: (I) perception of the signal by the membrane receptors; (II) generation of second messengers; (III) a cascade of protein phosphorylation/dephosphorylation events that may target transcription factors controlling specific sets of stress-regulated genes; (IV) stress tolerance, plant adaptation, and other phenotypic responses.
Calcium-dependent protein kinase (CDPK) has been shown to be involved in signaling processes as well as other protein kinases, such as mitogen-activated protein kinase (MAPK) and calcineurin B-like protein-interacting protein kinases (CIPKs). All kinases are thought to be primarily involved in the drought tolerance metabolism of crops. Ca+2 cytosolic levels increase rapidly in plant cells in response to environmental stresses, such as drought and salinity (Moncrief et al., 1990; Hey et al., 2010; Cieśla et al., 2016; Kumar et al., 2018). Ca+2 has been considered to play a role in mediating stress response during injury, recovery from injury, and acclimation to stress (Palta, 1990). Ca+2 has a very prominent role in the maintenance of cell structure. It has been suggested that Ca+2 is necessary for recovery from drought by activating the plasma membrane enzyme ATPase, which pumps nutrients that were lost during cell damage (Moncrief et al., 1990; Palta, 1990; Bergmann, 1992; Waraich and Ahmad, 2011).
Asides from the risk of climate variability and climate change, coffee growers, are also in a vulnerable situation due to low yields mainly caused by high pressure from diseases, low soil fertility and poor or unbalanced nutritional management in plantations (Avelino et al., 2004; Wang et al., 2015; Tiemann et al., 2018). Hence, it is important that vulnerable coffee regions move forward and implement adaptation and mitigation strategies. From an agronomical point of view, proper nutrient management is one of the key components of climate adaptation strategies (Palutikov et al., 2013), because it can help alleviate the increasing drought and temperature stress caused by climate variability.
A Ca2+ deficit can interrupt the photosynthetic process and reduce carboxylation efficiency, stomatal behavior, photosynthesis capacity, and quantum yield (Atkinson et al., 1989; Ramalho et al., 1995; Eticha et al., 2017; Song et al., 2019). Ca2+ signaling and cytosolic acidification in guard cells trigger auxin-induced stomatal opening. The auxin also activates cation channels in the plasma membrane, which in turn facilitates Ca+2 influx and K+ efflux (Bergmann, 1992; Vanneste and Friml, 2013). Adequate concentrations of Ca2+ in the soil solution is an important factor for controlling the severity of specific ion toxicities, namely aluminum, manganese, and other heavy metal ions (Pavan et al., 1982; Hue et al., 2001; Fageria and Baligar, 2008).
Ca2+ is present in three major forms in the soils: in solution, bound to exchangeable sites of clay minerals and organic matter and as minerals. Only a small fraction of the total Ca2+ is present in soil solution. Plant roots can absorb only Ca2+ from soil solution. Several factors influence the availability of the Ca2+ in the soil solution, such as soil type, mineral fraction of the colloids, pH, organic carbon content, humid acids and cation exchangeable capacity (CEC). Ca2+ deficits are highest in acid and sandy soils and/or in soils with high aluminum (Al+3) saturation and a high Al+3 to Ca2+ ratio in soil solution (Bolan et al., 2007). Acid soils are the dominant soils of the coffee regions. Leaching of anions like nitrate (), sulfate () and chloride (Cl−) induce the leaching of basic cations like Ca2+ and Mg2+. When an excessive amount of potassium (K+) fertilizer is added to soil, some of the Ca2+ and Mg2+ at the cation exchangeable sites can be replaced by K+ ions (Bolan et al., 2007), resulting in an increase of Ca2+ in the soil solution.
Coffee trees respond to an increase of exchangeable Ca+2 in the soil. At five locations in Colombia, representing typical soils of the coffee regions, highest growth rates of coffee plants have been achieved with an exchangeable Ca+2 content of 5.1–11.2 cmolc.kg−1 soil (Sadeghian and Diaz, 2020). On 44 sites in Minas Gerais state in Brazil, 2.01–3.13 cmolc.dm−3 exchangeable Ca+2 in the soil was sufficient to reach highest coffee yield levels (90–100% of yield) (Silva et al., 2018). In Rwanda, a strong linear relationship between exchangeable Ca+2 in soil and coffee yield was observed, reaching the highest yield at an exchangeable Ca+2 content of 5.0–7.0 cmolc.kg−1 soil (Cyamweshi et al., 2014).
The Ca2+ demand for certain crop species is high and Ca2+ is one of the most demanded nutrients in coffee. In C. canephora, the most demanded macronutrients are: nitrogen> calcium> potassium>magnesium>sulfur>phosphorous (Bragança et al., 2007), while in C. arabica, the nutrient uptake is as follows: potassium>nitrogen>calcium>phosphorous>magnesium> sulfur (Ramírez et al., 2002; Laviola et al., 2007).
The present study shows results from different research trials on coffee plants conducted under greenhouse and field conditions aiming to evaluate the influence of Ca+2 application on coffee plant growth, abiotic stress response, cation uptake, leaf cell structure, and coffee yields.
Methods
Greenhouse Trials
Different trials with coffee plants were conducted between 2014 and 2018 under greenhouse and field conditions. All greenhouse trials were carried out at the Hanninghof Research Center of Yara International in Germany. Day/night temperature was adjusted to 23/16°C with 60–70% relative air humidity. Supplemental light (300 μmol m−2 s−1 photosynthetic photon flux density) with a 12–14 h light period was given when the natural light became insufficient.
Plants were pre-germinated in dark condition, and before the radicle emerged (BBCH scale 03), the pre-germinated seeds were moved to small containers with perlite as a growing medium. The seeds were allowed to germinate until the plants reached three pairs of leaves that were completely open (BBCH scale 13) and were transplanted in pots of 4,5 L with quartz-sand as a growing medium.
The aim of the greenhouse trials was to test the response of young coffee plants to increasing Ca+2 application rates, drought and heat stress, and different pH levels in the substrate. A total of 3 greenhouse trials were conducted. Trial 1 evaluated the response of the growth of young coffee trees to three rates of soluble Ca+2 (70, 140, and 210 mg L−1) and a control without Ca+2. All treatments in trial 1 were setup in a complete randomized design. Trial 2 was initiated to test the effect of the three Ca+2 rates used in trial 1 in interaction with three soil moisture levels (35–40, 55–60, and 80–90% of field capacity) on coffee growth and photosynthetic activity (PN). A treatment with zero Ca was not installed in trial 2, because trial 1 showed that plants without Ca+2 do not grow well and finally die. In trial 3 the influence of three Ca+2 rates (8, 40, and 80 mg.L−1) interacting with two pH levels in the substrate (pH4 and pH6) on coffee growth and nutrient uptake has been assessed. A maximum of 80 mg.L−1 Ca+2 was applied in this trial, because the results of the previous trials showed that higher Ca+2 rates result only in small and insignificant increases in growth. In the same trial 3 the effect of increasing Ca+2 rates on the growth and temperature stress tolerance of young coffee trees were tested in selected treatments (treatments with soil pH adjusted to 6). The PN was measured at two temperature levels: 23.3°C (±0.5), which is in the optimal temperature range for coffee, and 31.3°C (±0.6), which represents heat stress for the plants. A complete randomized 2-factorial design has been used for the trials 2 and 3. The trials 1, 2 and 3 were harvested 10, 13 and 11 months after transplanting, respectively. Twelve replications per treatment were used in the trials.
All nutrients in the greenhouse trials were applied as nutrient solutions to the soil surface, without any foliar application. Pots were watered with a complete nutrient solution containing N (13 mM), P (1.0 mM), K (4.5 mM), Mg (1.2 mM), S (0.9 mM), Fe (12.87 μM), Mn (7.2 μM), Zn (2.5 μM), Cu (1.4 μM), B (2.4 μM), and Mo (0.5 μM), and the nutrient solution was applied once per week with application volumes between 80 and 110 mL, according to the water demand of the plants.
To determine field capacity (FC) of the pots, the substrate was saturated with water and pots were covered with plastic to avoid evaporation. Once the free drainage stopped, the weight of the pot has been measured and considered as the point of FC. For trials 1 and 3, the gravimetric soil moisture content was measured daily in each of the pots and constantly maintained at 55–60% of the field capacity (FC). For trial 2, the pots were placed into a computer-controlled irrigation system, with the capacity to irrigate 260 pots, continuously moving, and irrigating automatically up to 10 times per day. The water consumption recorded and the water stress patterns were set up based on the desired holding capacity as described before. In trial 3, the pH was adjusted with 1,0 N H2SO4 and 1,0 M KOH solutions.
The photosynthesis rates (PN) were measured after 2 months of water deficit in trial 2; selecting sunny days with mean air temperatures between 23 and 25°C. In trial 3, the PN was measured during sunny days in the summer with the high and low temperatures of 31.3°C (±0.5) and 23.3°C (±0.6), respectively. The PN readings were made between 10:30 a.m. and 12:10 p.m., randomly among the treatments. The pots were irrigated early morning to avoid drought stress in order to measure the influence of the air temperature on the photosynthesis of coffee plants growing in low and optimum Ca+2 rates (8 and 80 mg L−1 of Ca+2).
The photosynthesis rates (PN) for trials 2 and 3 were measured when four pairs of leaves from the apex to the stem fully expanded using the Li-6400 Portable Photosynthesis System (Li-Cor Biosciences, Inc., Lincoln, NE, USA). The equipment was set up with the following parameters: temperature = 25°C; CO2 concentrations 370 μmol.mol−1, PAR = 1.000 umol photons m−2 s−1. Measurements were made after 3 min equilibration between 10:00 and 12:00 h.
For trial 3, the rate of the cation uptake was calculated according to the equation (1), presented by Kumar et al. (2010), performing intermedium harvest at 5 and 11 months after transplanting.
Ur: Net rate of ion uptake relative to dry weight.
WR: Dry weight, WR2 at 11 months and WR1 at 5 months after transplanting.
M: Nutrient content of plants, M2 at 11 months and M1 at 5 months after transplanting.
t: Time t2 at 11 months and t1 at 5 months after transplanting.
In the trial 1, stem diameter at 30 cm height and plant height were measured at harvest time. In all three trials, dry biomass at harvest was analyzed separately for roots, stems, old leaves, special (leaf samples from the third and fourth pair leaves of each branch counted from the top of the branch), and youngest leaves (first two pairs of leaves from each branch and principal stem). All samples were dried in an oven at 65°C until a constant weight was attained. The dried plant material was then finely ground for nutrient analysis in the lab.
Finely milled plant materials were used for elemental analysis after wet digestion in a microwave digester (MLS 1200 mega; MLS GmbH, Leutkirch, Germany). All micro- and macronutrients (excluding nitrogen) were analyzed using inductively coupled plasma optical emission spectrometry (Perkin-Elmer Optima 3000 ICP-OES; Perkin-Elmer Corp., Norwalk, CT, USA).
The morphology and cell structure of leaf and petiole samples were analyzed by selecting mature leaves with Ca+2 deficiency symptoms from plants supplied with low Ca+2 rates and leaves without Ca+2 deficiency symptoms from plants with optimum Ca+2 rates. Scanning electron microscopy at different magnifications has been applied to detect differences between the samples. The magnification can be found at the bottom of each image. The scanning electron microscope (SEM) was equipped with a cold stage for cryo-microscopy. Therefore, cryofixation was used, and the cryo-fixed specimens were cryo-fractured under a vacuum in a special chamber to reveal internal structure, sputter-coated, and then transferred onto the SEM cryo-stage and scanned while still frozen (Location: University of Applied Sciences Münster- Campus Steinfurt; Stegerwaldstraße 39; 48565 Steinfurt, Germany).
Field Trials
Two trials were carried out under field conditions in the southeast region of Colombia, The parental material for the soil for both trials is a biotite-granite classified as a Typic Tropothents and Typic Dystrudepts (González and Salamanca, 2008). The trial 1 was located in El Pital-Huila in a farm located at 02°20,1'62” N-75°50,1'41” W and at 1.700 m elevation. The soil conditions (0–20 cm) at the first trial were: pH 4.0; organic matter of 4.78%; and P, K, Ca, and Mg contents of 80, 141, 448, and 150 mg kg−1, respectively, with a soil particle distribution of 70% sand, 24% silt and 6% clay. The trial 2 was located in Garzón-Huila in a farm located a 02°10,40” N-75°35'99” W and at 1.43700 m elevation The soil conditions during trial 2 were: pH 4.12; organic matter at 4.17%; and P, K, Ca, and Mg contents of 1.8, 62, 267, and 46 mg kg−1, respectively, with a soil particle distribution of 75.3% sand, 17.4% silt and 7.3% clay. The pH was determined in CaCl2, organic-matter by Walkley-Black, P by Bray-II, and the exchangeable fraction of K, Mg and Ca with 1 N ammonium acetate extraction (1 N NH4C2H3O2, pH 7.0). The cations in the extracts were detected using an ICP (Perkin Elmer, Optima 8300), soil texture analyses using the hygrometer-Bouyoucos method. The mean climatic conditions observed in the region from 2014 to 2019 are shown in Table 1.
The aim of the first trial was to evaluate the yield response of the coffee plants to different Ca+2 rates during the whole season from 2014 to 2018 (5 years-4 harvests), and test the influence on C. arabica L. cv. Castillo® productivity with resistance to the Coffee leaf rust (CLR) disease generated by the fungi Hemileia vastatrix Verkeley & Brome. The plantation was established without shade and the coffee was planted with a plant density of 5.100 plants ha−1 at 1.4 m distance between plants and 1,4 m distance between rows.
The experiment was set up in a randomized complete block design with four replications, each plot into the blocks had 54.88 m2 with a total of 28 plants and 10 effective plants for yield evaluation. The nutrients N, P2O5, K2O, MgO, and S in trial 1 were applied at rates of 260, 110, 230, 77, and 66 kg ha−1 year−1, respectively. Calcium has been applied at rates of 0, 50, 100, and 150 kg CaO ha−1 year−1. The CaO rates were soil applied and split in three applications during the year: at pre-flowering and at 90 and 180 days after flowering. Calcium nitrate was used as a Ca+2 source, the other nutrients were applied using an ammonium-nitrate based NPK fertilizer, also providing Mg, S, B, and Zn.
The second trial was carried out in the same coffee variety over 2 years 2017–2018 from stem trimmings to the first harvest, with the aim of determining the Ca+2 uptake dynamics after flowering up to the harvest. The plantation was established without shade and the coffee was planted with a plant density of 6.600 plants ha−1 at 1.5 m distance between plants and 1.0 m distance between rows. The nutrient application rates in trial 2 for N, P2O5, K2O, MgO, and CaO were 190, 68, 190, 40, and 100 kg ha−1 year−1, respectively. In the first year, the CaO rates were soil applied and split in three applications during vegetative growth at 2, 5, and 8 months after stem trimming. In the second year, the applications were split in 3 rates: pre-flowering, 90 and 180 days after flowering. Calcium nitrate was used as a Ca+2 source. The other nutrients were applied using Urea (46%N), ammonium-diphosphate (18%N-46%P2O5) and potassium chloride (60%K2O).
The experiment was set up in a randomized block design with four replications, each plot in the blocks occupied 45 m2 of space with a total of 30 plants and 8 effective plants used for data assessment. In each effective plant the branch number 10 counted from the apex to the base was labeled at pre-flowering stage (BBCH scale 54–57) (Arcila et al., 2002). Each effective plant served as a replicate and the cherries of a labeled branch were harvested in intervals of 30 days from 0 to 240 days after flowering. Cherries were harvested when reaching maturity at BBCH scale 88.
The samples were dried in an oven at 65°C for a few days until a constant weight was attained, and finely ground for nutrient analysis. At harvest time 240 days after flowering, red cherries (BBCH scale 88) were sampled, and the external part of the cherry was removed. The dry parchment was removed once it reached 11% moisture.
Statistical Analysis
All data were submitted to their respective analysis of variance (ANOVA) test according to the experimental design. Statistical analysis was conducted using the Statgraphics Centurion software package (Statgraphics Technologies, Inc.). Shapiro-Wilks modified test was applied for normality tests were carried out using the and the heterogeneity of variances using the residuals vs. prediction test for each of the variables. The Fisher's LSD test was used to detect the treatments that had a significant effect in the ANOVA.
Results
Effect of Ca+2 on Coffee Growth in a Controlled Environment
The coffee plant responded to increasing Ca+2 application rates. The rates had a significant effect on total dry biomass, stem diameter, plant height (Table 2). Ca+2 directly influenced root growth and total dry biomass, stem diameter, and plant height (Figures 1A,C,D). The lack of calcium reduced root growth and consequently, the root: shoot ratio (Figure 1B).
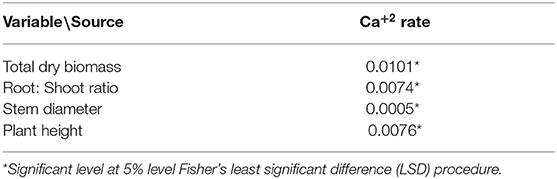
Table 2. Pr > F-values from the statistical output (Anova, 5%) for Calcium (Ca+2) rates in biomass accumulation.
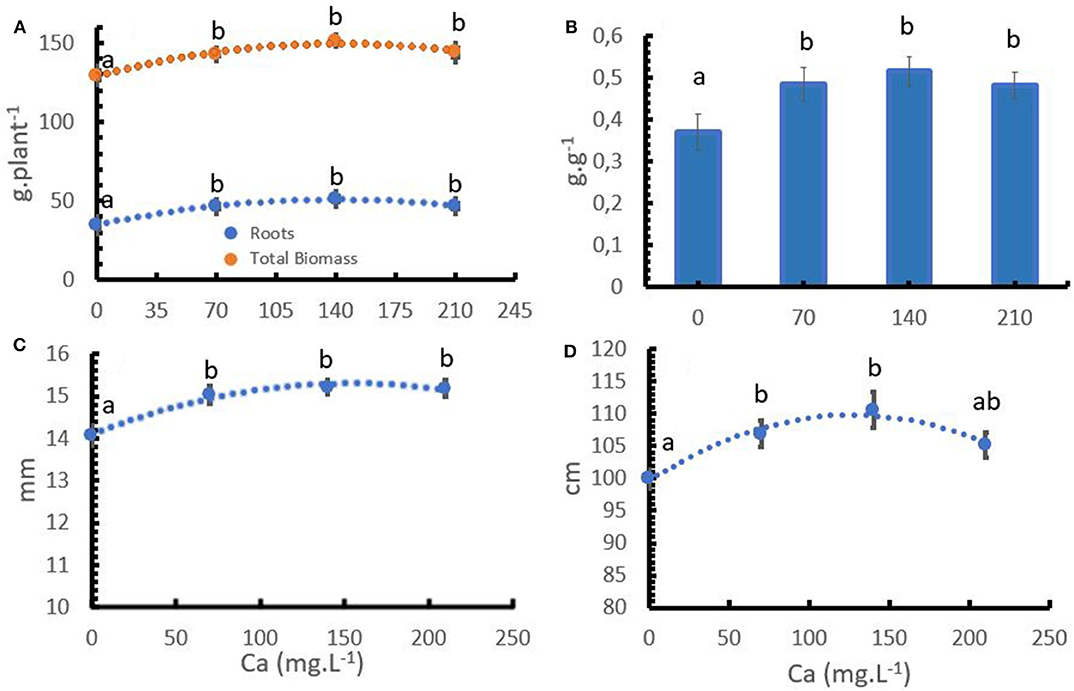
Figure 1. Effect of the calcium (Ca+2) and coffee dry biomass accumulation (A), root: shoot ratio (B), stem diameter (C), and plant height (D). Points and bars represent the mean values for each of the replicates± standard error. Different letters represent statistically significant differences among the treatments (P < 0.05, LSD fisher).
Interaction of Ca+2 Rates and Soil Moisture Levels in a Controlled Environment
The coffee plants responded positively to the soil moisture changes (mainly from 35–40 to 55–60% FC) and to Ca+2 rates (10, 75, and 150 mg L−1). The interaction between soil moisture and Ca+2 rate had a significant influence on the aboveground biomass (Table 3).
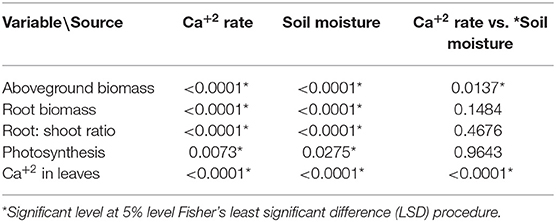
Table 3. Pr > F-values from the statistical output (Anova, 5%) for influence of the Calcium (Ca+2) rates and soil moisture levels on the biomass accumulation.
Low soil moisture levels (35–40% FC) reduced the Photosynthesis rate (PN) (Figure 2A) compared to optimum water supply (55–60% FC), which in turn resulted in a decrease of the aboveground and root biomass (Figures 2B,D). Water over-supply (80–90% FC) significantly reduced root growth and PN at medium and high Ca+2 rates (Figures 2D,A), but aboveground biomass development was improved compared to the optimum water supply. As a result of the abovementioned findings, the over-supply of water resulted in the lowest root-shoot ratio, while the difference in the ratio between optimum and low water supply was relatively low at low Ca+2 rates and higher at medium and higher Ca+2 rates (Figure 2C).
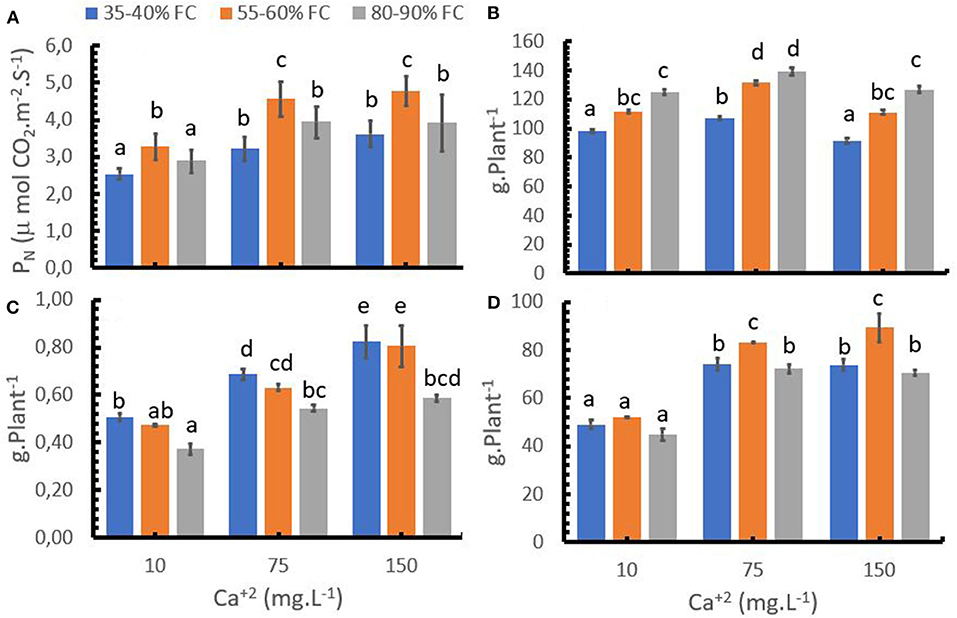
Figure 2. Effect of the calcium (Ca+2) rates with different water levels with respect to the field capacity (FC) on photosynthesis (PN, A) aboveground biomass (B), root: shoot ratio (C), root biomass (D). Bars represent the mean values for each of the replicates ± standard error. Different letters represent statistically significant differences among the treatments (P < 0.05, LSD fisher).
An increase in the Ca+2 rate from 10 to 75 mg L−1 improved PN activity at any given water supply level (Figure 2A). A similar pattern was observed for the effect of Ca+2 rates on aboveground and root growth (Figures 2B,D). A further increase to 150 mg L−1 Ca+2 led only to small increases of PN, which were not significant, and to a reduction of crop growth compared to 75 mg L−1 Ca+2. The results indicate that Ca+2 can reduce the negative effect of inadequate water supply (water deficit or water excess) on PN, root biomass accumulation and the root: shoot ratio.
Increasing Ca+2 supply increased the root: shoot ratio while an increase in soil moisture reduced the ratio (Figure 2C).
Interaction Between Ca+2 in the Leaves, Photosynthesis, and Soil Moisture in a Controlled Environment
The interaction between Ca+2 application rate, Ca+2 in the leaves, and the water level was statistically significant (Table 3). Figure 3B shows that an increase in Ca+2 application from 10 to 150 mg L−1 resulted in increasing concentrations of Ca+2 in the leaves at any given water supply level. A reduction in leaf Ca+2 concentration was observed with the increasing water supply at rates of 75 and 150 mg L−1 Ca+2. This can be explained by the increase in aboveground biomass production with increasing water supply (Figure 2B), which resulted in a dilution of Ca+2 in the leaves. However, the soil moisture content had no impact on the leaf Ca+2 concentration at a low Ca+2 rate (10 mg L−1).
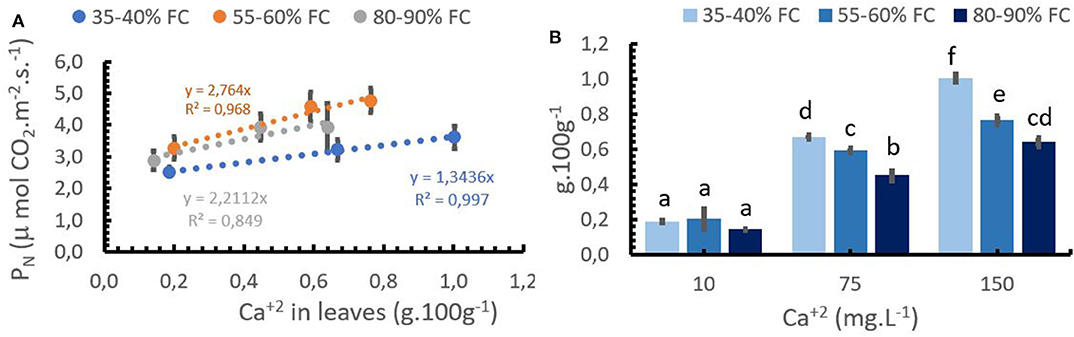
Figure 3. Relationship between Ca+2 concentration in the leaves and photosynthetic activity –(PN,A), and Ca+2 application rates and leaf Ca+2 concentrations at 3 soil moisture levels (B). Bars represent the mean values for each of the replicates ± standard error. Different letters represent statistically significant differences among the treatments (P < 0.05, LSD fisher).
PN was positively correlated with the Ca+2 concentration in the leaves at each water supply level (Figure 3A). However, the strongest increases in PN were observed in the optimum supply of water (55–60% FC). At optimum soil moisture, the PN increased by 2.74 μmols CO2 m−2 s−1 per unit increase in foliar Ca+2, compared with 2.21 μmols CO2 m−2 s−1 increase in the treatment with high soil moisture content. At low water supply (35–40% FC), the PN increased only by 1.34 μmols CO2 m−2 s−1 for each unit of foliar Ca+2 increase (Figure 3A), although leaf Ca+2 was significantly different between medium and higher Ca+2 rates at any given water supply (Figure 3B). Low Ca+2 rates (10 mg.L−1) reduced PN rates and leaf Ca+2 content at any given water supply as compared to higher Ca+2 application rates. The differences, however, were not significant. The lower PN at low soil moisture can be explained by a reduction in the gas exchange over the leaves due to pronounced stomata closure during the water stress scenario.
The highest PN rates were obtained from Ca+2 concentrations between 0.6 and 0.8% in the leaves without soil water limitations.
Calcium (Ca+2), Air Temperature, and Photosynthesis (PN) in Controlled Environments
In the trial 3, both, Ca+2 rates and air temperature, had a significant influence on PN rates. Increasing the mean air temperature from 23.3 to 31.3°C resulted in a reduction of the PN rate at any given Ca+2 application rate (Figure 4A). Increasing Ca+2 applications improved PN activity at both temperatures, confirming the potential of Ca+2 to ameliorate coffee stress tolerance. At the optimum temperature for coffee (23.3°C), the PN strongly increased up to a rate of 80 mg L−1 Ca+2, while under stress conditions (31.3°C), an increase from 8 to 40 mg L−1 Ca+2 significantly improved PN from 0.54 to 2.1 μmol CO2 m−2 s−1. However, a further increase to 80 mg L−1 Ca+2 only resulted in a moderate increase of PN to 2.88 μmol CO2 m−2 s−1.
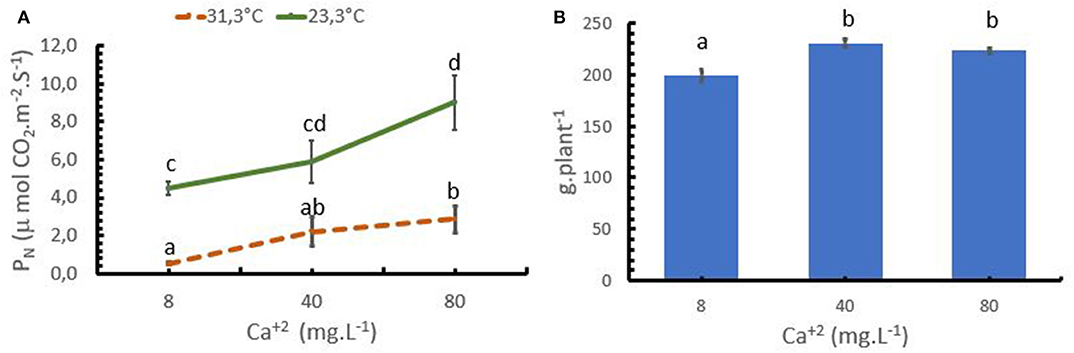
Figure 4. Effect of the calcium (Ca+2) on the photosynthesis (PN) of coffee plants at 2 temperature levels (A), and in biomass accumulation (B). Points and bars represent the mean values for each of the replicates ± standard error. Different letters represent statistically significant differences among the treatments (P < 0.05, LSD fisher).
The lower PN rates in the treatment with low Ca+2 rates significantly reduced the biomass accumulation in the coffee plants, where the treatment with 8 mg L−1 presented lower biomass accumulation compared with the treatment with 40 and 80 mg L−1 (Figure 4B).
Interaction Between Soil pH, Ca+2 Rates, and Cation Uptake in a Controlled Environment
The application of Ca+2 had a direct and significant influence on the Ca+2, Mg+2, and K+ concentrations in the tissues. At low Ca+2 concentrations in the solution, Mg+2 and K+ concentrations in the leaves and stems were relatively high, while the Ca+2 concentration was on a low level. Increasing Ca+2 application rates significantly increased the Ca+2 concentration in the tissues following a linear trend. Mg+2 and K+ tissue concentrations decreased significantly with increasing Ca+2 application rates. However, both, Mg+2 and K+ concentration, showed a stabilization at Ca+2 rates higher than 40 mg.L−1 (Figure 5).
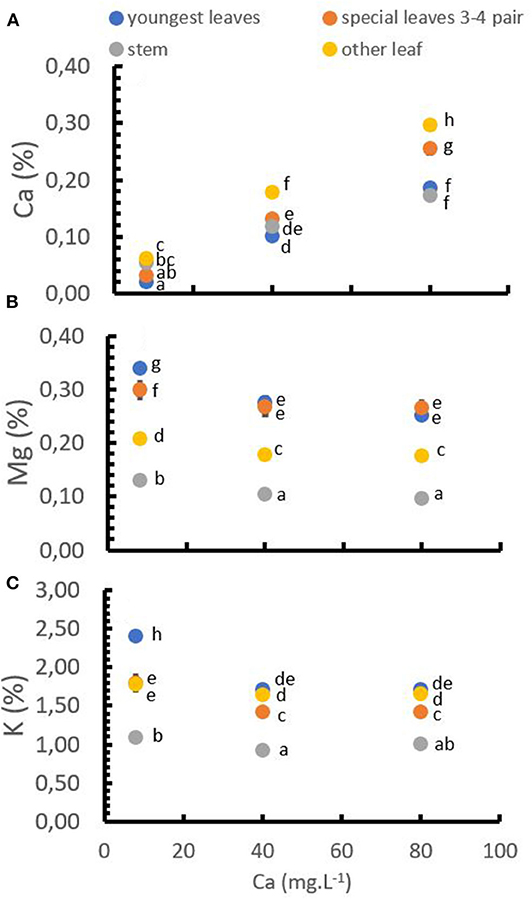
Figure 5. Cation distribution in different parts of the plant at different Ca+2 rates. Ca+2 in tissues (A), Mg+2 in tissues (B), and K+ in tissues (C). Points represent the mean values for each of the replicates ± standard error. Different letters represent statistically significant differences among the treatments (P < 0.05, LSD fisher).
The Ca+2 accumulation in the tissues was higher in the older and “special” leaves (third and fourth pair) and lower in youngest leaves and stems (Figure 5A). Additionally, contrary to Ca+2, the concentration of Mg+2 tended to be higher in the youngest and special leaves and lower in the old leaves (Figure 5B), which was similar to the K+ concentration that was also higher in the youngest leaves (Figure 5B).
Only the concentration of K+ in the leaves showed a significant interaction between Ca+2 doses and soil pH (Table 4). The data show that in low doses of Ca+2 (8 mg.L−1) and high pH (pH = 6) the K+ in leaves was higher with a mean value of 2.51%, compared to the treatment with Low pH (pH = 4) in which the K+ concentration in the leaves was lower with an average value of 2.39%.
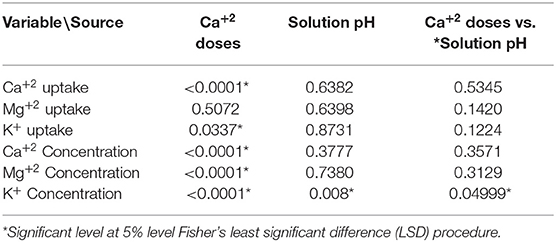
Table 4. Pr >F-values from the statistical output (Anova, 5%) for Calcium (Ca+2) and solution pH on cation uptake rates.
In the same trial, nutrient uptake of the trees was analyzed. Table 5 presents a strong significant relationship between Ca+2 rates and Ca+2 uptake, and a lower significant relationship between Ca+2 rate and K+ uptake. The Mg+2 uptake was not influenced by the Ca+2 rate. The Ca+2 uptake increased from ~2 to more than 11 g per month and plants with an increase in Ca+2 application from 8 to 80 mg L−1 Ca+2. K+ uptake also increased from 30 to 40 mg, while Mg+2 uptake did not change as Ca+2 application increased (Figure 6).
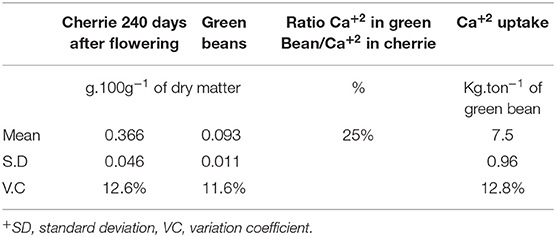
Table 5. Calcium concentration in cherries (including beans, husk, pulp, and mucilage) and in green beans, and the estimation of Ca uptake per ton for Coffea arabica L. cv. Castillo®.
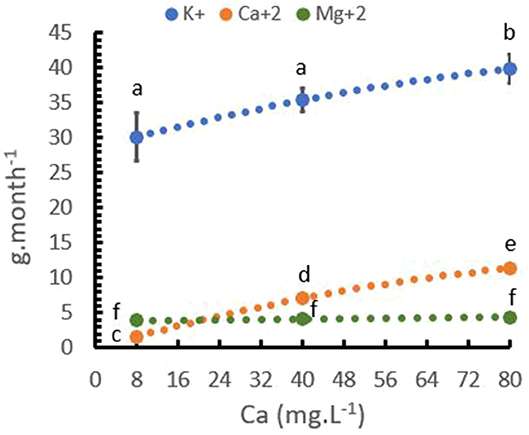
Figure 6. Relationship between calcium (Ca+2) rates and cation uptake rate per plant. Points represent the mean values for each of the replicates ± standard error. Different letters represent statistically significant differences among the treatments (P < 0.05, LSD fisher).
Calcium (Ca+2) and Coffee Leaf Cell Structure
Figure 7 shows a cross-section of coffee leaves with low and optimum Ca+2 rates (Figures 7A,B). Leaves with optimum Ca+2 present thicker leaves at 286.4 μm ± 11.8 on average compared to 265.0 μm ± 26.7 in the leaves with low Ca+2. Furthermore, leaves with optimum Ca+2 presented a dense epidermis, and a large, compact, better-structured palisade parenchyma (83.3 μm ± 5.1) (Figure 7D) compared with leaves with low Ca+2 (65 μm ± 5.3) (Figure 7C). The optimum Ca+2 had a higher diameter in the xylem vessels and leaf petioles with mean value of (20.7 μm ± 1.0) (Figure 7F) compared with the leaves with lower Ca+2 with mean value of (18.3 μm ± 1.9) (Figure 7E).
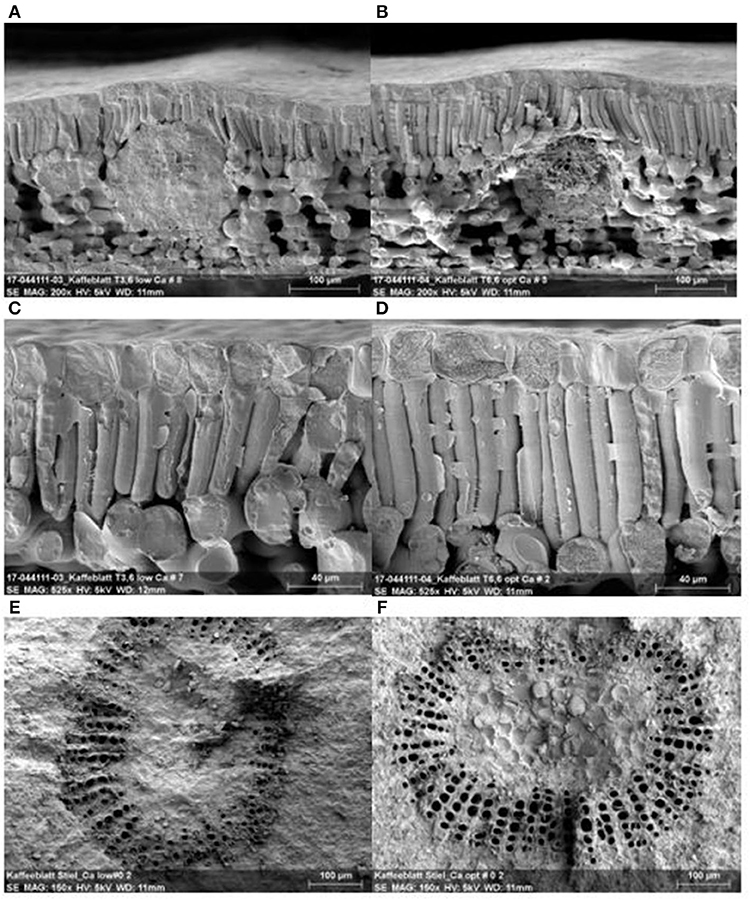
Figure 7. Leaf structure with low and optimum Ca+2 applications. Leaf cross section with low Ca+2 (A), and optimum Ca+2 (B), leaf epidermal and palisade cell with low Ca+2 (C) and optimum Ca+2 (D), leaf petiole vascular bundle for low Ca+2 (E) leaf petiole vascular and optimum Ca+2 (F).
Calcium (Ca+2) Dynamics After Flowering and Yield Response Under Field Conditions
Under field conditions in Colombia, the Ca+2 concentration of coffee cherries presented a negative exponential slope and Ca+2 uptake presented a positive polynomial increase. The Ca+2 concentration in the cherry showed a fast reduction during the first 120 days after flowering, reaching an almost constant level between 150 and 240 days after flowering, while the Ca+2 uptake by the coffee cherry during the first 90 days after flowering was low, with <10% of the total uptake. The Ca+2 uptake accelerated between 120 and 210 days after flowering, where 67% of the total Ca+2 was taken up by the growing coffee cherries (Figures 8A,B).
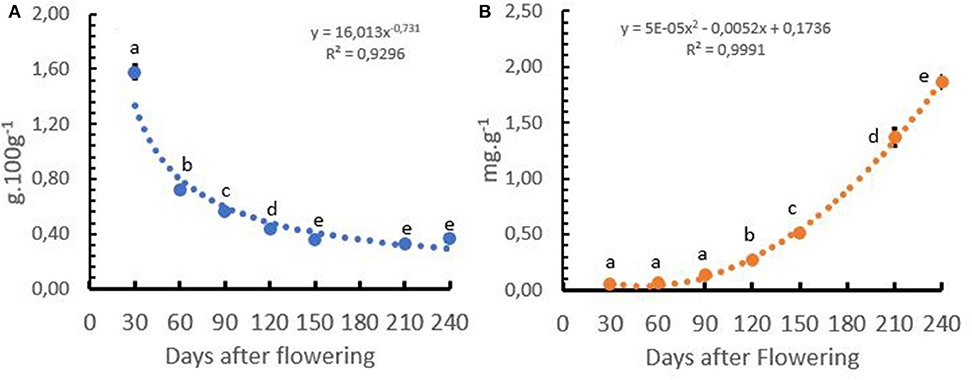
Figure 8. Calcium (Ca+2) concentration (A) and uptake of Ca+2 (B) by the coffee cherries after flowering to harvest in Coffea arabica L. cv. Castillo®. Points represent the mean values for each of the replicates ± standard error. Different letters represent statistically significant differences among the treatments (P < 0.05, LSD fisher).
Once the cherries reached their harvest period 240 days after flowering, 25% of the total Ca+2 uptake in cherries was accumulated in the green coffee beans, which are then used to prepare the coffee beverage (Table 5).
The mean Ca+2 uptake of green coffee beans in C. arabica L. cv. Castillo® was 7.5 kg Ca+2 ton−1 of green bean, including the husk, pulp, and mucilage (Table 5). It was observed that the coffee crop had a positive response to the Ca+2 application after 5 years of the field trial, and the sigmoidal model showed that the optimum yield response of the crop to Ca+2 was at the rate of 120 kg CaO ha−1 year−1 (Figure 9).
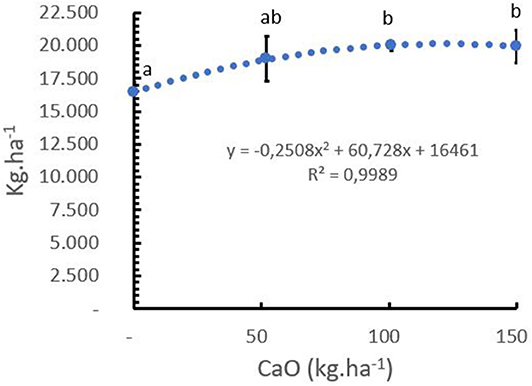
Figure 9. Effect of calcium rates (kg CaO/ha) on yield in Coffea arabica L. cv. Castillo® (mean yield from 2014 to 2018). Points represent the mean values for each of the replicates ± standard error. Different letters represent statistically significant differences among the treatments (P < 0.05, LSD fisher).
Discussion
Calcium (Ca+2) and Growth
The formation of new root tissues always depends on continuous Ca+2 uptake. Root growth is controlled by Ca+2, which regulates cell growth, increases the elasticity and stability of the cell walls, encourages metabolic activity, and binds CO2 from respiration in the nutritive medium (Bergmann, 1992). The role of Ca+2 is thought to be involved in the development of lateral and primary root meristems. The number of growing meristems ultimately determines the relative sink strength of the root system (Picchioni et al., 2001). Ca+2 improved the growth and development parameters of young coffee plants in controlled environments (Figure 1A). The aboveground biomass increased by 5.7% with 140 m L−1 of Ca+2 compared to the treatment without Ca+2, and root biomass increased by 47% in the same trial. The higher increase in root biomass compared to aboveground development explains the differences in the root: shoot ratio with variable Ca+2 applications (Figure 1B).
Ramalho et al. (1995), studied C. arabica L. cv. Catuai under a controlled environment and found a 26% reduction in the total biomass and a 15% reduction in the root biomass in treatments without Ca+2. Shoot biomass reduction was closely related to the reduction in the new leaf area after 40 days of Ca+2 reduction. Eticha et al. (2017), investigated the controlled environmental conditions in citrus (Citrus sinensis (L) Osbeck) reported that Ca+2 deficiency resulted in higher root tip mortality and root system decay, compared to a healthy and prolific root formation in plants that received Ca+2 treatment.
With regard to other developmental parameters, it is important to observe that Ca+2 increases stem diameter (Figure 1C). This finding has practical relevance as a larger stem diameter provides the plants more stress resistance during the harvest periods and increases the vigor of the vegetative buds, which in turn promotes the formation of new branches after stem pruning.
Calcium (Ca+2), Abiotic Stress, and Photosynthesis
Root biomass accumulation is the main effect of Ca+2 in the phenotypic response of coffee under drought stress (Figure 2D). This means that the perception of the signal began in the roots under drought stress, and all the cascading responses were generated by this organ. Another reason why Ca+2 promotes root growth with and without drought stress is linked to the antagonism between Ca+2 and auxin. Auxin promotes shoot growth and inhibits root growth, whereas Ca+2 inhibits shoot growth and promotes root growth (Hepler, 2005). Duan et al. (2010) demonstrated that FERONIA (FER) receptor-like kinase had been involved in root hair development, a process that correlates with reduced levels of auxin-regulated reactive oxygen species (ROS) accumulation in roots and root hairs. Bascom and Bezanilla (2018) claimed that the modulation of many processes and structures in root tip growth is driven by specific ion concentrations, such as Ca+2 and protons, in the cytoskeleton and in the cytoplasm, and finally generating an apical Ca+2 gradient. The authors also mention that many channels in the plasma membrane, as well as organelles, are likely to be involved in establishing and maintaining the apical Ca+2 gradient.
Water stress reduced the photosynthetic activity (PN), but the reduction was lower in the treatments with Ca+2 (Figure 2A), indicating that Ca+2 plays a role in activating the signaling process to control the photosynthesis rates. The essential role of Ca+2 has been confirmed by the positive correlation between Ca2+ in the leaves and PN rates (Figure 3A). Hence, an optimal calcium supply is very important in maintaining a sufficient Ca+2 concentration in coffee plants to reduce the negative effect of drought stress and heat stress (Figure 4A).
Drought stress affects several plant processes that lead to membrane disorganization, metabolic toxicity, reduction in turgor, photosynthetic rate, and stomatal conductance, as well as the generation of reactive oxygen species (ROS), including superoxide radicals (O2−), hydrogen peroxide (H2O2), and hydroxyl radicals (OH−) (Khushboo et al., 2018; Kumar et al., 2018). It was observed in several studies that Ca+2 supplementation resulted in lower accumulation of superoxide radicals and H2O2. Furthermore, enhanced accumulation of calcium in wheat was correlated with higher activities of antioxidant enzymes under drought stress conditions (Khushboo et al., 2018). Sakhonwasee and Phingkasan (2017) reported that the foliar application of CaCl2 and CaNO3 in tomatoes under heat stress resulted in a lower production of hydrogen peroxide and superoxide, which in turn resulted in reduced cell death compared to the control plants.
Ramalho et al. (1995) examined coffee under controlled environments and found a positive correlation between leaf Ca+2 concentration and the photochemical efficiency and total chlorophyll concentration in coffee, and concluded that a shortage in photo assimilates and leaf growth had direct involvement in the significant decrease in shoot biomass.
In coffee plants, Ca2+deficiency induces the impairment of photosynthesis both at the stomatal and mesophyllic levels. Ramalho et al. (1995) showed evidence regarding the role of Ca2+ at the mesophyllic level. In fact, Ca2+ concentrations showed a reasonable (r2 = 0.71) positive correlation with Fv/Fm; that is, a gradual decrease in the photochemical efficiency of PS II accompanied the decrease in Ca2+ leaf concentrations. The authors suggest that such a loss in photochemical efficiency is linked to the lower O2 evolution rates observed under Ca+2-deficient conditions.
Ca+2 is required for the proper functioning of the photosynthetic enzyme, Rubisco. Ca2+ supply hinders rubisco degradation under stress conditions (Lavon et al., 1999; Dolatabadian et al., 2013). Based on the positive correlation between quantum efficiency (QE) and Ca+2, Ramalho et al. (1995) argued that a rise in pH gradient occurs in the thylakoid with lower Ca+2 levels. The rise in the intrathylakoidal proton gradient indicates both the existence of electron transport after PSII (responsible for its build-up) and a relative decrease in the utilization of that proton gradient. Thus, the impairment of photosynthesis does not occur only at the PS II level. The decrease in the utilization of the pH gradient could be due to a decrease in ATPase activity or due to the use of ATP, for example, in carboxylation. Furthermore, it was suggested that the build-up of the pH gradient is a result of a decrease in the activity of ATPase more than the reduction of Rubisco activity. The presence of Ca+2 will then provoke a decrease in photoinactivation by controlling the redox balance at the PS II level (Krieger and Weis, 1993). Semin et al. (2008) stated that Ca+2 restored the electron transport activity and fluorescence induction kinetics in PSII membranes and thylakoid membranes (TM) when the pH in the thylakoid drops.
The foliar application of CaCl2 and CaNO3 improved the operating efficiency of photosystem II (PSII) in the light-adapted state, maximized quantum efficiency of PSII in the dark-adapted state (Fv/Fm), and maximized the CO2 assimilation rate under heat-stress conditions in tomato. In contrast, the application of MgCl2 and KNO3 had no significant effect on those parameters (Sakhonwasee and Phingkasan, 2017). Similarly, Dolatabadian et al. (2013) reported that the foliar application of Ca+2 reduced the antioxidant enzyme activity and lipid peroxidation in wheat leaves.
In contrast to Ramalho et al. (1995), who found that at least Ca+2 leaf concentrations of 1% were required to promote modifications in the photosynthetic parameters, in this study, a positive correlation between leaf Ca+2 and PN was observed at concentrations between 0.2 and 1.1%. Under drought stress conditions, the leaf content of Ca+2 was higher than that under non-stress conditions (Figure 3B). Under drought stress conditions, 1% Ca+2 in the leaves was measured to reach the highest level of PN (3.0 μmol CO2 m−2.s−1), while under non-stress conditions, 0.8% of Ca+2 resulted in a high PN level at 4.5 μmol CO2 m−2.s−1 (Figure 3A).
Different authors working under field conditions in bermudagrass (Cynodon dactylon (L) Pers.) and trees (Dalbergia sissoo Roxb) report an increase in the Ca+2 concentration in the tissues under water stress conditions (Utrillas et al., 1995; Singh and Singh, 2004). Other authors have shown contradictory results; for instance, Sardans et al. (2008) in the Mediterranean evergreen (Quercus ilex) forest indicated that drought tends to decrease the Ca+2 concentration in the aboveground biomass. They attributed this effect to the reduction in transpiration flux under drought stress. In our study on coffee, we hypothesized that higher Ca+2 concentrations in the leaves under water stress conditions are linked with a lower aboveground biomass development compared to the non-stressed treatment. A lower transpiration rate, stomata closure and reduction on PN under drought stress resulted in reduced biomass accumulation and, in turn, to a higher Ca+2 concentration in the biomass.
Calcium (Ca+2) and Cation Uptake
Ca+2 uptake by plants is influenced by the Ca+2 status and pH values of the soil and the concentration of other cations. It is absorbed by plants in its ionic form and is transported through the plant via transpiration flow in the xylem. Ca+2 mobility in the phloem is low, and remobilisation of the Ca+2 to the older leaves or fruits over the phloem is very limited (Bergmann, 1992; Gilliham et al., 2011). Hence, Ca+2 deficient symptoms are usually observed in young tissues (Tang and Luan, 2017). In this study, it was observed that the Ca+2 concentration in the coffee tissues at lower Ca+2 rates is low and similar in stems and old (other) leaves. The accumulation in all tissues increased linearly as the Ca+2 rates increased, but was much faster in old leaves (other leaves) and special leaves (third and fourth pair leaves) compared with the youngest leaves and stems (Figure 5A).
The reduction in the Mg+2 and K+ concentrations in the tissues with increasing Ca+2 rates did not follow a linear tendency. A strong reduction in Mg+2 and K+ uptake at lower Ca+2 rates indicated that coffee plants compensate for the lack of Ca+2 in the soil solution by uptake of other cations such as Mg+2 and K+ (Figures 5B,C). However, higher concentrations of K+ and Mg+2 did not result in better growth (Figure 4B).
According to Gilliham et al. (2011), it is likely that Ca+2 significantly contributes to the osmotic potential of mesophyll cells, and when mesophyll [Ca+2]vac is reduced, mesophyll specific accumulation of magnesium (Mg+2) is substituted for the osmotic role of Ca+2. In serpentine soil or low Ca+2 growth conditions, MRS2-1 and MRS2-5 proteins that encode vacuole-localized Mg+2 transporters were upregulated and appeared to play a key role in maintaining optimal growth. This can at least partly explain why the Mg+2 concentration in the leaves is highest in the treatments with low Ca+2, which lessens as Ca+2 supply increases, and remains more or less constant at the medium to high Ca+2 supply level.
Ca+2 is usually regarded as the counterpart to K+. Owing to its dehydrating properties, it opposes the plasma expanding action of K+ ions. Ca+2 ions are, therefore, important as a factor that influences the stability of the protoplasm structure (dehydrating effect of the Ca+2) (Bergmann, 1992). In their regulation of ion uptake by plants, Ca+2 ions have both synergistic and antagonistic effects. Low Ca+2 concentration encourages ion uptake, probably because Ca+2 has a membrane-stabilizing effect and through the fact that it encourages respiratory metabolism, synthetic activity, and electron transport (Bergmann, 1992). More recently, it was demonstrated at the molecular level by Xu et al., 2006 that Ca+2 activates calcineurin B-like (CBL) proteins and protein kinase family such as CIPK23, which serves as an activator and regulator of K+ transporters, directly influencing K+ uptake.
Ramalho et al. (1995) found that an increase in Ca+2 concentration in the soil solution reduced the concentration of K+ and Mg+2 in the leaves and roots in C. arabica L. cv. Catuai under a controlled environment. Since Ca+2, Mg+2, K+ (and also Na+2) are cations that may substitute for each other in case of a lack or excess of one of them; these reductions may represent the maintenance of the electrical and chemical balance of the cell. However, in our study, K+ uptake directly increased proportionally to Ca+2 supply and Ca+2 leaf concentration (Figure 6). This allowed us to hypothesize that at low Ca+2 concentrations in the soil solution, K+ and Mg+2 compensation occurs. As Ca+2 increases in the solution, Ca+2-dependent proteins are activated at the molecular level, thus improving root growth and activating transporters that directly influence K+ uptake, as previously discussed.
The causes for poor plant growth on acidic soils include the immobilization of phosphorous and molybdenum, the inhibition of the Mg+2, Ca+2, and K+ uptake, and the increase in the concentration of Mn+2 and Al+3 ions. Although a drop in soil pH leads to those consequences, the low pH value, as such, is usually not a limiting factor for growth. Plants can grow under acidic conditions (pH values of 3.5–4.5) as long as the soil solution contains enough Ca+2 and other essential nutrients and the concentration of highly toxic elements (especially Mn+2 and Al+3) remain low (Bergmann, 1992). A toxic concentration of H+ ions can only be expected at very low pH values (3.0 or lower), whereas Mn+2 and Al+3 toxicity are to be reckoned with at pH values <4.5 (Bergmann, 1992).
Calcium (Ca+2) and Coffee Leaf Cell Structure
The leaf vacuole is widely regarded as the major Ca+2 storage organelle in plant tissues. Ca+2 is preferentially secreted into the vacuoles of specific cell types in leaves (Gilliham et al., 2011). Once Ca+2 is deposited into the vacuoles, it is not redistributed because, with the exception of the small amounts involved in signaling, most intracellular Ca+2 is relatively immobile (White and Broadley, 2003; Paiva, 2019).
A reduction in Ca+2 accumulation in the mesophyll cell shows a severe effect on leaf physiology, such as perturbation of cell wall synthesis and activation of genes, which ultimately results in thicker and less extensible cell walls. Furthermore, the reduction in stomatal apertures is observed, which reduces transpiration and CO2 assimilation with a negative effect on plant growth (Gilliham et al., 2011). In this study, we demonstrated the effect of Ca+2 on cell wall growth and structure, which resulted in thicker leaves, dense epidermal cells, and better-structured palisade parenchyma. In leaf petioles, a better structure and higher number of xylem vessels were observed (Figure 7). All these findings had a direct influence on photosynthetic rates and stomatal conductance (data not shown), which in turn was translated into increased dry biomass accumulation with and without abiotic stress. A close linear correlation between leaf Ca+2 content and cell wall dry matter was observed and reported by Eticha et al. (2017) in citrus.
Ca+2 Dynamics After Flowering and Coffee Yield
Dubberstein et al. (2016) in C. canephora and Laviola et al. (2007) and Sadeghian et al. (2013) in C. arabica report that the Ca+2 accumulation in coffee cherries follows a sigmoidal model, with a slow accumulation during the first 60 days and exponential accumulation during 60 to 200 days after flowering. In this study, the Ca+2 concentration in the cherries reduced from 1.6 g.100 g−1 at 30 days after flowering to 0.4 g.100 g−1 at 240 days after flowering (harvest time), which was slightly different from the values reported by Sadeghian et al. (2013) for the same variety; with a reduction from 1.07 g.100 g−1 at 30 days after flowering to 0.4 g.100 g−1 at 240 days after flowering (harvest time).
Dubberstein et al. (2016) reported that the Ca+2 concentration in coffee beans in C. canephora was lower in fertilized than in unfertilized plants. This can be explained by a) the application of NH4+, K+, and Mg+2, which can result in a decrease in the absorption of Ca+2 and also because the rate of Ca+2 applied was too low (12.5 kg CaO ha−1) compared with higher N and K2O rates at 440 and 270 kg ha−1, respectively, and b) the dilution of the nutrient Ca+2 caused by higher cherry yield in the fertilized plants.
Understanding the dynamics of Ca+2 uptake by the cherries is important because it allows growers to better understand the critical periods of Ca+2 application to the crop. The results of this study revealed that the application of a soluble Ca+2 fertilizer source must be performed continuously during the growing season because of the rather low mobility of this cation in the plant and the importance for abiotic stress tolerance. Next, Ca+2 must be available in sufficient amounts in the productive growth phase during the first 60 days after flowering to ensure quick cherry development (Figure 8B).
The trial results show that the total Ca+2 uptake required to produce 3.3 ton of green coffee beans was 35 kg CaO ha−1 year−1, while the optimal yield response was achieved at a rate of 120 kg CaO ha−1. year−1 (Figure 9). This means that 32% of the total CaO applied has been taken up by the beans, and the rest was distributed in the vegetative tissues, cherry pulp, held by the soil, and/or lost by leaching and runoff.
Conclusions
This is a holistic study on the importance and the role Ca+2 in the coffee crop nutrition through the physiological and agronomical perspective. After 5 years of trials, we concluded that:
• Coffee plants respond widely to Ca2+ application during the vegetative and productive stages.
• Ca2+ application in coffee has a stronger positive influence on root biomass accumulation than on the development of aboveground biomass.
• The Ca2+ concentration in the leaves shows a positive correlation with photosynthesis.
• Under abiotic stress conditions, Ca2+ reduces the negative influence of heat and drought stress in coffee plants.
• The optimum Ca2+ rate for Arabica coffee is ~120 kg CaO ha−1 year−1.
• Ca2+ was the third most demanded nutrient in coffee cherries.
• Proper Ca2+ management in coffee plants improves stem diameter, which in turn improves the capacity of the plant to respond better to stem trimming and reduce plant death after pruning.
In the productive stages, the peak in Ca2+ uptake by the plant was during pre-flowering and 120 days after flowering, but the low mobility of Ca2+ in the plant requires the availability of sufficient soluble Ca2+ in soil solution throughout the season.
Data Availability Statement
The raw data supporting the conclusions of this article will be made available by the authors, without undue reservation.
Author Contributions
VR-B contributes with the field and greenhouse trial, data analysis, and manuscript preparation. JK contributes with the trial set up in field and greenhouse, data analysis, and manuscript preparation. TdS and CS contribute with the greenhouse trials implementation. All authors contributed to the article and approved the submitted version.
Funding
The authors declare that this study received funding from Yara International. The funder was not involved in the study design, collection, analysis, interpretation of data, the writing of this article or the decision to submit it for publication.
Conflict of Interest
The authors declare that the research was conducted in the absence of any commercial or financial relationships that could be construed as a potential conflict of interest.
Supplementary Material
The Supplementary Material for this article can be found online at: https://www.frontiersin.org/articles/10.3389/fagro.2020.590892/full#supplementary-material
References
Arcila, P. J., Buhr, L., Bleiholder, H., Hack, H., Meier, U., and Wicke, H. (2002). Application of the extended BBCH scale for the description of the growing stages of coffee (Coffea spp.). Ann. Appl. Biol. 141, 19–27. doi: 10.1111/j.1744-7348.2002.tb00191.x
Atkinson, J. C., Mansfield, T. A., Kean, A. M., and Davies, W. J. (1989). Control of stomatal aperture by calcium in insolated epidermal tissue and whole leaves of Commelina communis L. New Phytol. 111, 9–17. doi: 10.1111/j.1469-8137.1989.tb04212.x
Avelino, J., Willocquet, L., and Savary, S. (2004). Effects of crop management patterns on coffee rust epidemics. Plant Pathol. 53, 541–547. doi: 10.1111/j.1365-3059.2004.01067.x
Bascom, C. S. Jr, Hepler, P. K., and Bezanilla, M. (2018). Interplay between ions, the cytoskeleton, and cell wall properties during tip growth. Plant Physiol. 176, 28–40. doi: 10.1104/pp.17.01466
Bergmann, W. (1992). “Nutritional disorders of plants,” in Gustav Fisher Verlag Jena (Stuttgart), 741.
Bertrand, B., Boulanger, R., Dussert, S., Ribeyre, F., Berthiot, L., Descroix, F., et al. (2012). Climatic factors directly impact the volatile organic compound fingerprint in green Arabica coffee bean as well as coffee beverage quality'. Food Chem. 135, 2575–2583. doi: 10.1016/j.foodchem.2012.06.060
Bolan, N. S., Loganathan, P., and Saggar, S. (2007). “Calcium and magnesium in soils,” in: Encyclopedia of Soils in the Environment. 1st Edn. (New York, NY:Academic Press Editor in chief Daniel Hillel), 149–154. doi: 10.1016/B0-12-348530-4/00223-X
Bragança, S. M., Martinez, P. H. E., Leite, G. H., Santos, P. L., Sediyanna, C. S., and Alvarez, V. H. (2007). Accumulation of macronutrients for the Conilon coffee tree. J. Plant Nutr. 31,103–120. doi: 10.1080/01904160701741990
Bunn, C., Läderach, P., Ovalle Rivera, O., and Kirschke, D. (2015b). A bitter cup: climate change profile of global production of Arabica and Robusta coffee. Clim. Change 129, 89–101. doi: 10.1007/s10584-014-1306-x
Bunn, C., Läderach, P., Pérez Jimenez, J. G., Montagnon, C., and Schilling, T. (2015a). Multiclass classification of agro-ecological zones for arabica coffee: an improved understanding of the impacts of climate change. PLoS ONE 10:e0140490. doi: 10.1371/journal.pone.0140490
Choi, W. G., Hilleary, R., Swanson, S. J., Kim, S. H., and Gilroy, S. (2016). Rapid, long-distance electrical and calcium signaling in plants'. Annu. Rev. Plant Biol. 67, 287–307. doi: 10.1146/annurev-arplant-043015-112130
Cieśla, A., Mituła, F., Misztal, L., Fedorowicz-Strońska, O., Janicka, S., Tajdel-Zielińska, M., et al. (2016). A role for barley calcium-dependent protein kinase CPK2a in the response to drought. Front. Plant Sci. 7:1550. doi: 10.3389/fpls.2016.01550
Craparo, A. C. W., van Asten, P. J. A., Läderach, P., Jassogne, L. T. P., and Grab, S. W. (2015). Coffea arabica yields decline in Tanzania due to climate change: global implications. Agric. Forest Meteorol. 207, 1–10. doi: 10.1016/j.agrformet.2015.03.005
Cyamweshi, R. A., Nabahungu, N. L., Mukashhema, A., Rugunzu, V., Gatarayiha, M. C., Nduwumuremyi, A., et al. (2014). Enhancing nutrient availability and coffee yield on acid soil of the central plateau of southern Rwanda. Glob. J. Agric. Res. 2, 44–55.
DaMatta, F. M., Rahn, E., Läderach, P., Ghini, R., and Ramalho, J. C. (2019). Why could the coffee crop endure climate change and global warming to a greater extent than previously estimated? Clim. Change 152, 167–178. doi: 10.1007/s10584-018-2346-4
DaMatta, F. M., Ronchi, C. P., Maestri, M., and Barros, R. S. (2007). Ecophysiology of coffee growth and production. Braz. J. Plant Physiol. 19, 485–510. doi: 10.1590/S1677-04202007000400014
Davis, A. P., Govaerts, R., Bridson, D. M., and Stoffelen, P. (2006). An annotated taxonomic conspectus of the genus Coffea (Rubiaceae). Bot. J. Linn. Soc. 152, 465–512. doi: 10.1111/j.1095-8339.2006.00584.x
Dolatabadian, A., Sanavy, S. A., Gholamhoseini, M., Joghan, A. K., Majdi, M., and Kashkooli, A. B. (2013). The role of calcium in improving photosynthesis and related physiological and biochemical attributes of spring wheat subjected to simulated acid rain. Physiol. Mol. Biol. Plants 19, 189–198. doi: 10.1007/s12298-013-0165-7
Duan, Q., Kita, D., Li, C., Cheung, A. Y., and Wu, H. M. (2010). FERONIA receptor-like kinase regulates RHO GTPase signaling of root hair development. Proc. Natl. Acad. Sci. U.S.A. 107, 17821–17826. doi: 10.1073/pnas.1005366107
Dubberstein, D., Partelli, F. L., Dias, J. R. M., and Espindola, M. C. (2016). Concentration and accumulation of macronutrients in leaf of coffee berries in the Amazon, Brazil. Aust. J. Crop Sci. 10, 701–710. doi: 10.21475/ajcs.2016.10.05.p7424
Eticha, D., Kwast, A., de Souza, C. T., Horowitz, N., and Stützel, H. (2017). Calcium nutrition of orange and its impact on growth, nutrient uptake and leaf cell wall. Citrus R&T 38, 62–70. doi: 10.4322/crt.ICC096
Fageria, N. K., and Baligar, V. C. (2008). Ameliorating soil acidity of tropical oxisols by liming for sustainable crop production. Adv. Agron. 99, 345–399. doi: 10.1016/S0065-2113(08)00407-0
Felle, H. H. (2001). pH: signal and messenger in plant cells. Plant Biol. 3, 577–591. doi: 10.1055/s-2001-19372
Ferrão, G. R., Almeida, F. A. F., Gava, F. M. A., Verdin, F. A. C., Volpi, P. S., de Muner, L. H., et al. (2012). “Conilon coffee: production techniques with improve varieties,” in Revised and Update, Victória, 4th Edn. (Espiritu Santo: Instituto Capixaba de Pesquisa, Assisténcia Têcnica e Extensão Rural), 74.
Gilliham, M., Dayod, M., Hocking, B. J., Xu, B., Conn, S. J., Kaiser, B. N., et al. (2011). Calcium delivery and storage in plant leaves: exploring the link with water flow. J. Exp. Bot. 62, 2233–2250. doi: 10.1093/jxb/err111
Gilroy, S., Białasek, M., Suzuki, N., Górecka, M., Devireddy, A. R., Karpiński, S., et al. (2016). ROS, calcium, and electric signals: key mediators of rapid systemic signaling in plants. Plant Physiol. 171, 1606–1615. doi: 10.1104/pp.16.00434
González, O. H., and Salamanca, J. A. (2008). Representative soil units of the Colombian coffee zone. Chinchiná:Cenicafé. 25.
Hepler, P. K. (2005). Calcium: a central regulator of plant growth and development. Plant Cell 17, 2142–2155. doi: 10.1105/tpc.105.032508
Hey, S. J., Byrne, E., and Halford, N. G. (2010). The interface between metabolic and stress signaling. Ann. Bot. 105, 197–203. doi: 10.1093/aob/mcp285
Hue, N. V., Vega, S., and Silva, J. A. (2001). Manganese toxicity in a hawaiian oxisol affected by soil pH and organic amendments . Soil Sci. Soc. Am. J. 65, 153–160. doi: 10.2136/sssaj2001.651153x
Jaleel, C. A., Manivannan, P., Sankar, B., Kishorekumar, A., and Panneerselvam, R. (2007). Calcium chloride effects on salinity-induced oxidative stress, proline metabolism and indole alkaloid accumulation in Catharanthus roseus. C. R. Biol. 330, 674–683. doi: 10.1016/j.crvi.2007.07.002
Khushboo, B. K., Singh, P., Raina, M., Sharma, V., and Kumar, D. (2018). Exogenous application of calcium chloride in wheat genotypes alleviates negative effect of drought stress by modulating antioxidant machinery and enhanced osmolyte accumulation. In Vitro Cell. Dev. Biol. Plant 54, 495–507. doi: 10.1007/s11627-018-9912-3
Krieger, A., and Weis, E. (1993). The role of calcium in the pH-dependent control of photosystem II. Photosynth. Res. 37, 117–130. doi: 10.1007/BF02187470
Kumar, F. N., Baligar, C. V., and Jones, C. A. (2010). Growth and Mineral Nutrition of Field Crops. 3rd Edn. Boca Raton, FL: CRC Press-Taylor & Francis Group.
Kumar, S., Sachdeva, S., Bhat, K. V., and Vats, S. (2018). Plants Responses to Drought Stress: Physiological, Biochemical and Molecular Basis in Biotic and Abiotic Stress Tolerance in Plants. ed S. Vats (Singapore: Springer Nature Singapore Pte. Ltd.). doi: 10.1007/978-981-10-9029-5_1
Laviola, G. B., Prieto, M. G., Bartolomeu de Souza, R., and Alvarez, V. H. (2007). Dinámica de cálcio e magnésio en folhas e frutos de Caffea arabica. R. Bras. Si. Solo. 31, 319–329. doi: 10.1590/S0100-06832007000200014
Lavon, R., Salomon, R., and Goldschmidt, E. E. (1999). Effect of potassium, magnesium, and calcium deficiencies on nitrogen constituents and chloroplast components in Citrus leaves. JASHS 124, 158–162. doi: 10.21273/JASHS.124.2.158
McAinsh, M. R., and Pittman, J. K. (2009). Shaping the calcium signature. New Phytol. 181, 275–294. doi: 10.1111/j.1469-8137.2008.02682.x
Moncrief, N. D., Kretsinger, R. H., and Goodman, M. (1990). Evolution of EF-hand calcium-modulated proteins. I. Relationships based on amino acid sequences. J. Mol. Evol. 30, 522–562. doi: 10.1007/BF02101108
Paiva, E. A. S. (2019). Are calcium oxalate crystals a dynamic calcium store in plants? New Phytol. 223, 1707–1711. doi: 10.1111/nph.15912
Palta, J. P. (1990). Stress interactions at the cellular and membrane levels. HortScience 25, 1377–1381. doi: 10.21273/HORTSCI.25.11.1377
Palutikov, J., Parry, M., Smith, S. M., Ash, J. A., Boulter, L. S., and Waschka, M. (2013). The Past, Present and Future of Adaptation: Setting the Context and Naming the Changes in Climate Adaptation Futures. eds J. P. Palutikof, S. L. Boulter, A. J. Ash, M. S. Smith, M. Parry, M. Waschka, et al. (Jersey City, NJ: John Wiley & Sons, Ltd.). doi: 10.1002/9781118529577
Panhuysen, S., and Pierrot, J. (2018). Coffee barometer. Editing Pascal Kuipers and Tijmen de Vries (Schuttelaar &Partners). Available online at: https://www.hivos.org/assets/2018/06/Coffee-Barometer-2018.pdf.36p. (accessed September 12, 2020).
Pavan, A. M., Bingham, F. T., and Pratt, P. F. (1982). Toxicity of aluminum to coffee in ultisols and oxisols amended with CaCO3, MgCO3 and CaSO4.2H2O. Soil Sci. Soc. Am. J. 45, 1201–1206. doi: 10.2136/sssaj1982.03615995004600060017x
Pérez-Rendón, E. P., Ramírez-Builes, V. H., and Peña-Quiñones, A. J. (2016). Variabilidad espacial y temporal de la temperatura del aire en la zona cafetera colombiana. Investigaciones Geográficas 2016, 23–40. doi: 10.14350/rig.38707
Pham, Y., Reardon-Smith, K., Mushtaq, S., and Cockfield, G. (2019). The impact of climate change and variability on coffee production: a systematic review. Clim. Change 156, 609–630. doi: 10.1007/s10584-019-02538-y
Picchioni, G. A., Valenzuela-Vazquez, M., and Armenta-Sanchez, S. (2001). Calcium-activated root growth and mineral nutrient accumulation of lupinus havardii: ecophysiological and horticultural significance. Jashs 126, 631–637. doi: 10.21273/JASHS.126.5.631
Praxedes, S. C., DaMatta, F. M., Loureiro, M. E. G., Ferrão, M. A., and Cordeiro, A. T. (2006). Effects of long-term soil drought on photosynthesis and carbohydrate metabolism in mature robusta coffee (Coffea canephora Pierre var. kouillou) leaves. Environ. Exp. Bot. 56, 263–273. doi: 10.1016/j.envexpbot.2005.02.008
Ramalho, J. C., Rebelo, M. C., Santos, M. E., Antunes, M. L., and Nunes, M. A. (1995). Effects of calcium deficiency on Coffea arabica. Nutrient changes and correlation of calcium levels with some photosynthetic parameters. Plant Soil 172, 87–96. doi: 10.1007/BF00020862
Ramírez, F., Bertsch, F., and Mora, L. (2002). Nutrient consumption by Caturra coffee fruits and bandolas during a development and maturation cycle in Aquiares, Turrialba, Costa Rica (in Spanish). Agron. Costarricense 26, 33–42.
Sadeghian, K. S., and Diaz, M. C. (2020). Soil acidity correction: effects on the initial coffee growth. Cenicafé 71, 21–31. doi: 10.38141/10778/1117
Sadeghian, K. S., Mejia, M. B., and Gonzalez, O. H. (2013). Accumulation of calcium, magnesium and sulfur by the coffee fruits (Coffea arabica L.) variety Castillo® (in Spanish). Cenicafé 64, 1–18.
Sakhonwasee, S., and Phingkasan, W. (2017). Erratum to: effects of the foliar application of calcium on photosynthesis, reactive oxygen species production, and changes in water relations in tomato seedlings under heat stress. Hortic. Environ. Biotechnol. 58:310. doi: 10.1007/s13580-017-1194-x
Sardans, J., Peñuelas, J., and Ogaya, R. (2008). Drought's impact on Ca, Fe, Mg, Mo and S concentration and accumulation patterns in the plants and soil of a Mediterranean evergreen Quercus ilex forest. Biogeochemistry 87, 49–69. doi: 10.1007/s10533-007-9167-2
Semin, K. B., Ivanov, I. L., Rubin, B. A., and Carpentier, R. (2008). pH-Depended extraction of Ca+2 from photosystem II membranes and Thylakoid membranes: indication of the Ca+2 sensitive site on the acceptor side of photosystem II. Photochem. Photobiol. 68, 538–544. doi: 10.1111/j.1751-1097.1998.tb02511.x
Silva, E. C., Nogueira, R. J. M. C., Silva, M. A., and Alburquerque, M. B. (2011). Drought stress and plant nutrition. Plant Stress. 5, 32–41.
Silva, S. J., Lima, N. J. C., Prieto, M. H. E., and Alvarez, V. V. H. (2018). Relationship between coffee leaf analysis and soil chemical analysis. Rev. Bras. Cienc. Solo. 42. [on line]13. doi: 10.1590/18069657rbcs20170109
Singh, B., and Singh, G. (2004). Influence of soil water regime on nutrient mobility and uptake by Dalbergia sissoo seedlings. Trop. Ecol. 45, 337–340.
Song, Q., Liu, Y., Pang, J., Yong, J. W. H., Chen, Y., Bai, C., et al. (2019). Supplementary calcium restores peanut (Arachis hypogaea) growth and photosynthetic capacity under low nocturnal temperature. Front. Plant Sci. 10:1637. doi: 10.3389/fpls.2019.01637
Talbot, J. M. (2004). Grounds for Agreement: the Political Economy of the Coffee Commodity Chain (Oxford: Rowman & Littlefield Publisher, Inc.).
Tang, R. J., and Luan, S. (2017). Regulation of calcium and magnesium homeostasis in plants: From transporters to signaling network. Curr. Opin. Plant Biol. 39, 97–105. doi: 10.1016/j.pbi.2017.06.009
Tiemann, T., Maung Aye, T., Duc Dung, N., Minh Tien, T., Fisher, M., Nalin de Paulo, E., et al. (2018). Crop nutrition for Vietnamese robusta coffee. Better Crops 102, 20–23. doi: 10.24047/BC102320
Tuteja, N., and Mahajan, S. (2007). Calcium signaling network in plants: an overview. Plant Signal. Behav. 2, 79–85. doi: 10.4161/psb.2.2.4176
Utrillas, M. J., Alegre, L., and Simon, E. (1995). Seasonal changes in production and nutrient content ofCynodon dactylon (L.) Pers. subjected to water deficits. Plant Soil 175, 153–157. doi: 10.1007/BF02413021
van Bel, A. J., Furch, A. C., Will, T., Buxa, S. V., Musetti, R., and Hafke, J. B. (2014). Spread the news: systemic dissemination and local impact of Ca2? signals along the phloem pathway. J. Exp. Bot. 65, 1761–1787. doi: 10.1093/jxb/ert425
Vanneste, S., and Friml, J. (2013). Calcium: the missing link in auxin action. Plants 2, 650–675. doi: 10.3390/plants2040650
Wang, N., Jassogne, L., van Asten, P. J. A., Mukasa, D., Wanyama, I., Kagezi, G., et al. (2015). Evaluating coffee yield gaps and important biotic, abiotic, and management factors limiting coffee production in Uganda. Eur. J. Agron. 63, 1–11. doi: 10.1016/j.eja.2014.11.003
Waraich, A. E., and Ahmad, R. (2011). “Role of mineral nutrition in the alleviation of drought stress in plants,” in Australian Journal of Crop Science. (Saifullah, Ashraf, MY) 5, 764–777.
Watts, C. (2016). A Brewing Storm: the Climate Change Risk to Coffee. By the Climate Institute. 16p: ISBN: 978-1-921611-35-3.
White, P. J., and Broadley, M. R. (2003). Calcium in plants. Review article. Ann. Bot. 92, 487–511. doi: 10.1093/aob/mcg164
Keywords: coffee, Calcium (Ca+2), drought and heat stress, photosynthesis, productivity, cations uptake
Citation: Ramírez-Builes VH, Küsters J, de Souza TR and Simmes C (2020) Calcium Nutrition in Coffee and Its Influence on Growth, Stress Tolerance, Cations Uptake, and Productivity. Front. Agron. 2:590892. doi: 10.3389/fagro.2020.590892
Received: 03 August 2020; Accepted: 11 November 2020;
Published: 01 December 2020.
Edited by:
Ayman EL Sabagh, Siirt University, TurkeyReviewed by:
Yajun Hu, Chinese Academy of Sciences, ChinaDaniel Sacristán Moraga, University of the Balearic Islands, Spain
Copyright © 2020 Ramírez-Builes, Küsters, de Souza and Simmes. This is an open-access article distributed under the terms of the Creative Commons Attribution License (CC BY). The use, distribution or reproduction in other forums is permitted, provided the original author(s) and the copyright owner(s) are credited and that the original publication in this journal is cited, in accordance with accepted academic practice. No use, distribution or reproduction is permitted which does not comply with these terms.
*Correspondence: Victor Hugo Ramírez-Builes, dmljdG9yLnJhbWlyZXpAeWFyYS5jb20=