- 1Department of Environmental Sciences - Botany, University of Basel, Basel, Switzerland
- 2Center for Sustainability and Climate Studies, Department of Ecology and Environmental Sciences, Pondicherry University, Puducherry, India
- 3Department of Agronomy, University of Agricultural Sciences, Bangalore, India
- 4Center for Natural Biological Resources and Community Development, Bangalore, India
Intercropping is a common cropping practice that takes advantage of plant diversity and plant complementarity to increase land-use efficiency. Biofertilizers centered around arbuscular mycorrhizal fungi (AMF) and plant growth promoting rhizobacteria (PGPR) are supplements to mineral fertilizers well-known for their far-reaching beneficial effects on plants such as increased drought resistance and increased yields. This study tested if the combination of intercropping and biofertilizers can enhance land-use efficiency (overyielding) using staple crops from southern India. Specifically, the role of different combinations of plant species (crop mixtures) and spatial arrangements mediating overyielding in intercropping was tested. Biofertilizer inoculation increased yields in both monocropping and intercropping. In intercropping, grain yield improved by an average of 23% over the control, regardless of the crop mixture or spatial arrangement. Positive crop-type-specific mycorrhizal growth responses (MGR) and overyielding across experimental treatments and sites indicate that combining biofertilizers with intercropping significantly enhances land-use efficiency in the semi-arid tropics. These findings have practical implications for improving the productivity of intercropping systems in dryland agricultural systems, particularly under resource-limited conditions.
1 Introduction
Dryland agriculture faces major challenges such as limited water availability, low soil fertility, and increasing climate variability, making conventional high-input practices unsustainable. Alternative approaches like intercropping combined with biofertilizers could improve resource efficiency, reduce environmental impacts, and boost productivity. This study investigated whether combining these approaches consistently enhances yields and land-use efficiency across different intercropping systems. Intercropping is a promising land-management practice for sustainable intensification of low-input agriculture that has positive ecosystem impacts such as increased biodiversity and soil health (Brooker et al., 2015; Wang et al., 2023). In intercropping two or more crop species are grown simultaneously on the same field for a substantial part of their growing period generally leading to improved land-use efficiency (Willey, 1990). Intercropped plant species grown in close proximity can profit from plant complementary effects, in terms of resource partitioning, abiotic facilitation and biotic feedbacks (Yu et al., 2015; Zhang et al., 2019a). Plant complementary effects in intercropping are determined by the plant species identity or crop mixtures, their planting distance, and spatial arrangements (Stefan et al., 2022). Crop mixtures and their spatial arrangement are generally selected to leverage complementary effects against interspecific competition to enhance productivity. However, increasing the planting distance between crop mixtures to reduce interspecific competition can also limit beneficial rhizosphere interactions, leading to reduced facilitation and decreased land-use efficiency of intercropping (Zhang and Li, 2003).
Biofertilizers consisting of arbuscular mycorrhizal fungi (AMF) and plant growth promoting rhizobacteria (PGPR) are soil microorganisms that can increase plant resistance to environmental challenges and facilitate plant nutrient uptake (Mäder et al., 2011; Schütz et al., 2018; Mathimaran et al., 2020). Moreover, biofertilizers can have a positive effect on the environment as they can improve the soil structure and aggregation (Smith and Read, 2010). Most importantly, field inoculations with biofertilizers have been shown to improve grain yields of crops, such as wheat, rice, maize, as well as marginal crops such as sorghum, a variety of legumes and millets from regions of Africa and Asia (Zhang et al., 2019b; Mathimaran et al., 2020; Singh et al., 2021). Biofertilizer effects on yield vary between crop species, depending on a wide variety of factors such as the quality of the inoculum and the identity of the AMF taxa infecting crop roots as well as abiotic factors such as soil types, soil fertility and climatic conditions (Tawaraya, 2003; Romero et al., 2023; Lutz et al., 2023). For example, in cereal crops, which have fine fibrous, highly branched roots and dense thin root hairs typically display lower reliance upon soil microorganisms for nutrient acquisition (Ryser and Lambers, 1995; Wilson and Hartnett, 1998). In contrast, legume crops with coarse, thick, and less branched roots generally require more nutrients to achieve the same yield as cereals because legumes expend more energy on respiration and nitrogen fixation (Sadras, 2006). As a result, legumes generally rely more heavily upon AMF and other soil microorganisms to acquire nutrients from the soil through their thin and extensively branched hyphal networks (Duponnois et al., 2008; Unger et al., 2016).
Combining intercropping and biofertilization holds potential to intensify yields in marginal dryland agriculture, where conventional high-input land-management strategies are not optional or desired. AMF form interconnected fungal networks known as common mycorrhizal networks (CMNs). CMNs can enhance nutrient exchange, particularly phosphorus and nitrogen, between neighboring crops, helping plants cope better with competition and environmental stress, thereby increasing the productivity and stability of intercropping (Wagg et al., 2011; Walder et al., 2012; Zhu et al., 2023). CMNs can further enhance plant diversity effects leading to overyielding by promoting resource transfer across different plant species (van der Heijden et al., 1998). However, the formation of a CMN between plants depends on the distance at which AMF hyphae can connect the root systems of different plant species (Giovannetti et al., 1999, van der Heijden et al., 2015). Singh et al. (2021) found that in a dryland cereal‐legume intercropping system using pigeon pea (Cajanus cajan) and finger millet (Eleusine coracana), the combined use of biofertilizers with intercropping arranged at varied planting distances resulted in higher yields than when either practice was applied alone. Furthermore, the study demonstrated that biofertilizers exerted crop‐type specific positive effects on yields in both monocropping and intercropping systems, regardless of spatial arrangement or site. Still, it remains unclear whether the effects of crop mixtures involving different species, spatial arrangements, and biofertilizers are consistent across different sites and seasons; this underlines the critical role of species selection in optimizing intercropping systems in combination with biofertilizers.
Building on previous research (Singh et al., 2021), which indicated the potential for biofertilizers to improve intercropping effects, this study tested additional crop mixtures consisting of three dryland legumes—pigeon pea (Cajanus cajan, PP), cowpea (Vigna unguiculata, CP), and lablab (Lablab purpureus, LL)—in addition to the cereal finger millet (Eleusine coracana, FM). These crop mixtures were evaluated under two spatial arrangements (row and mosaic) and on different soil types in varying climates. Specifically, the study aimed to determine: (1) whether biofertilizer effects remain consistent across different intercropping systems grown at different sites; (2) whether the spatial arrangement within intercropping mixtures influences the magnitude of biofertilizer effects; and (3) whether combining biofertilizers with intercropping leads to enhanced land-use efficiencies compared to monocropping, as well as the extent of variation in these effects across different crop mixtures and spatial arrangements.
2 Methods
2.1 Field site and conditions
Field trials were conducted at two sites in southern India, the University of Agricultural Sciences campus in Bangalore (GKVK), site 1, (Karnataka) and Kolli Hills, site 2 (Tamil Nadu). Both sites were studied during the first season (July 2018 to January 2019) and only site 1 during the second season (July 2019 to March 2020). Supplementary Table S1 shows the soil properties of both field sites, as well as their climatic conditions for the respective study years.
2.2 Experimental design
Experimental treatments ranged from T01 to T10. Each treatment was paired with a control (labeled “-”) and with biofertilizer inoculation (labeled “+”). Treatments T01-T04 were monocropping and T05-T10 were intercropping. Following recommendations from local agronomists, one cereal, finger millet (Eleusine coracana) (FM) variety GPU-28, and three local legumes, pigeon pea (Cajanus cajan) (PP) variety BRG-2, cowpea (Vigna unguiculata) (CP) variety C-152 and lablab (Lablab purpureus) (LL) local variety 1 were selected. During the second season, a new lablab (Lablab purpureus) local variety 2 was used. Seeds were provided by the Seed Center from the University of Agricultural Sciences campus in Bangalore. Supplementary Table S2 shows key agronomic traits for each species. Monocropping of cereal was defined as T01, with monocropping of each legume, PP, CP, LL, always in the same order defined as T02, T03, and T04, respectively. T05–T10 were intercropping, with T05–07 involving row-wise spatial arrangements with each legume species in the same order as in the monocropping, and T08–10 involving mosaic spatial arrangement of the same legume combinations (Figure 1). Intercropping spatial arrangements were based on previous research (Singh et al., 2021). Row spatial arrangement was adjusted as follows: in monocropping, cereal rows were 30 cm apart and plants were separated by 7.5 cm within each row. Legume monocropping rows were 60 cm apart and plants were 30 cm apart within each row. The distance between cereals and legume rows in row-wise spatial arrangement was 45 cm, and the mosaic spatial arrangement had an approximate spacing of 90 cm between legumes and cereals. To achieve the same plant densities of legumes and cereals in both intercropping spatial arrangements, always 8 cereal plants were substituted by one legume leading to 4 rows of 12 legumes in the row-wise arrangement and 12 rows of 4 legumes in the mosaic spatial arrangement. Monocropping were planted at plant densities of 1152 cereal plants and 144 legume plants per plot, in intercropping a ratio of 2:8 (legume: cereal) with respective plant densities was adjusted to 768 cereal plants and 48 legume plants per plot. The experimental design was a randomized complete block with r = 4 complete blocks. The plot size was 7.2 x 3.6 m, with a net plot area of 3.6 x 1.8 m (Table 1).
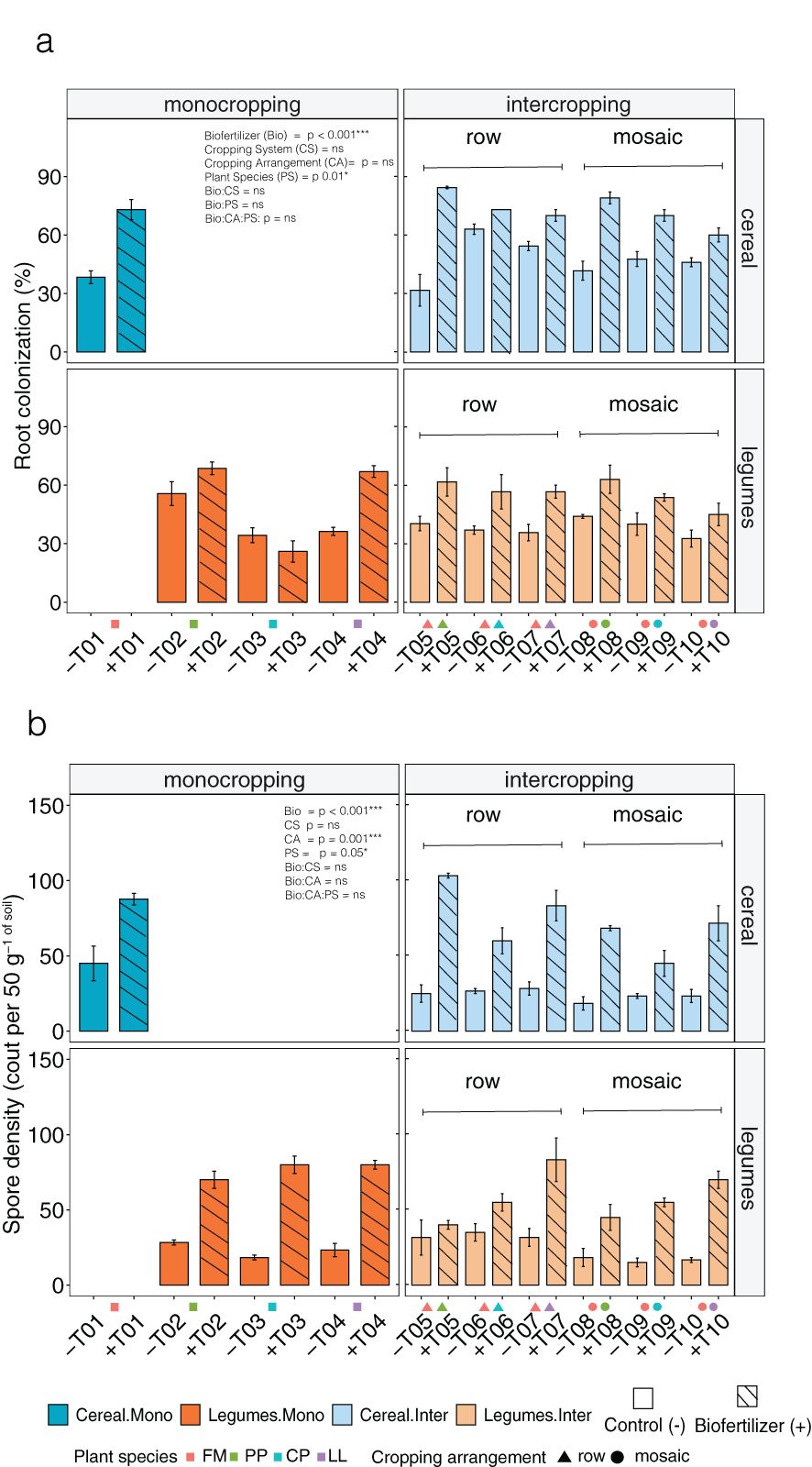
Figure 1. Effects of biofertilizer inoculation on (A) root colonization and (B) spore density at Site 1 during Season 2. Bars show finger millet (cereal, blue) and legume (orange) crops grown either in monocropping (dark shades) or intercropping (light shades). Control treatments are shown with empty bars (-), and biofertilizer treatments with striped bars (+) and cropping spatial arrangements are presented as row (triangle) and mosaic (circles). Species abbreviations: FM, Finger millet; PP, Pigeon pea; CP, Cowpea; LL, Lablab. Error bars show standard errors (SE).
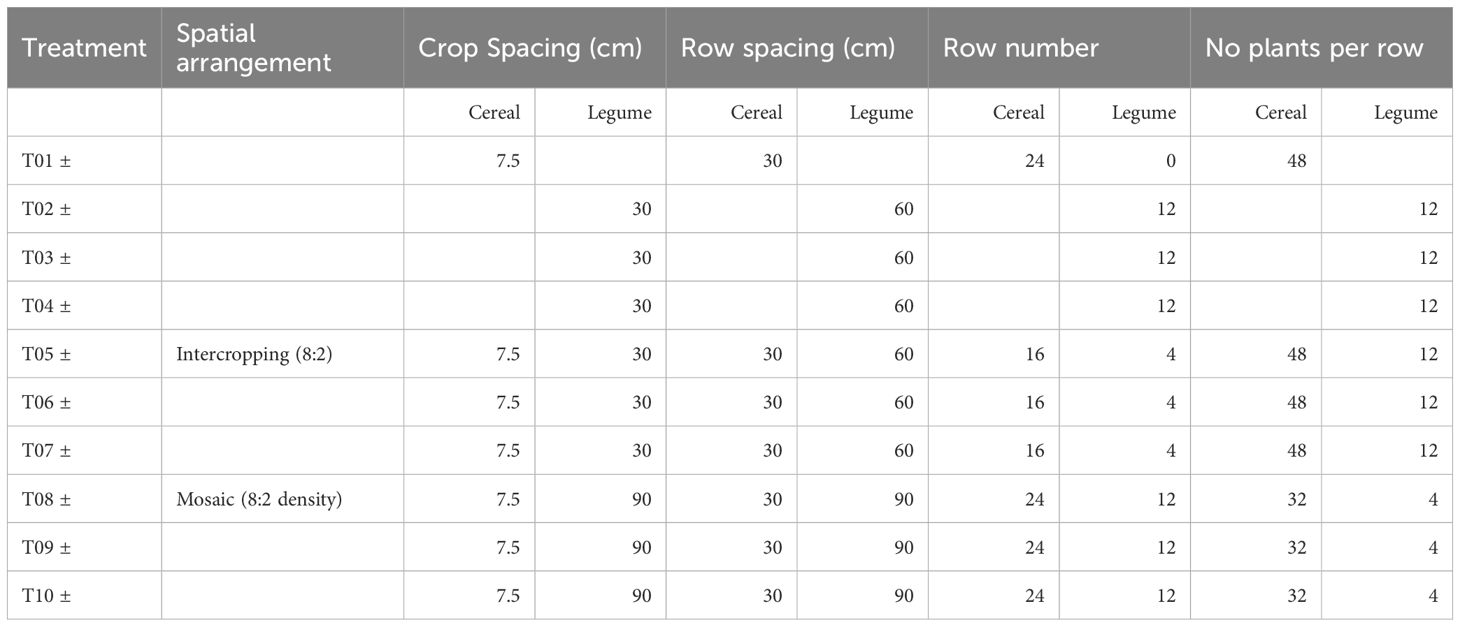
Table 1. Layout of experimental plots, spatial arrangements, and plant densities for different crop mixtures.
2.3 Microbial inoculants
Biofertilizer consisted of two AMF strains and plant growth promoting rhizobacteria (PGPR). Rhizophagus fasciculatus (formerly called Glomus fasciculatum) and Ambispora leptoticha (formerly called Glomus leptotichum) which were chosen for FM and PP/CP/LL, respectively (Govinda Rao et al., 1983; Byra Reddy and Bagyaraj, 1991). The PGPR strains (Pseudomonas sp. MSSRFD41 and Rhizobium liquid formulation) were chosen as a complement to the AMF strains as shown in other previous studies (Sekar and Prabavathy, 2014; Mathimaran et al., 2020; Singh et al., 2021). The AMF strains were obtained from Centre for Natural and Biological Resources and Community Development (CNBRCD), Bengaluru.
2.4 Microbial cultivation
AMF species were propagated in a vermiculite-based carrier material using Rhodes grass (Chloris gayana) as host plant for 40 to 45 days. The mycorrhizal inoculum quality was improved by setting the grass to a short drought period (last week of cultivation) after which the roots were chopped into small segments (ca., 0.5–1 cm), and mixed homogenously in the same substrate in which the grass was grown. The harvested Rhizophagus fasciculatus AMF inoculum consisting of 15 spores per g substrate was applied at the rate of 444 kg inoculum per hectare as a band application (along the planting rows). Glomus leptotichum inoculum consisting of 24 spores per g substrate was applied at the rate of 278 kg inoculum per hectare during sowing. PGPR pseudomonas strain was obtained from MS Swaminathan Research Foundation (MSSRF), Chennai and Rhizobium sp. was obtained from Agricultural Research Station, Acharya N. G. Ranga Agricultural University, Amaravati. The PGPR strains were multiplied in King`s B medium. The liquid culture consisting of 1x109 CFU ml-1 of Pseudomonas sp. MSSRFD41 was applied as seed coating at the rate of 10 ml per kg seed (Sekar and Prabavathy, 2014). Additionally, a band application was applied at the rate of 50 liters together with farmyard manure (FYM) 7.5 t per hectare. Rhizobium was applied as seed inoculation at the rate of 10 ml kg-1 (1x109 CFU ml-1) to all legume seeds.
2.5 Mineral fertilization
All plots received 50% of the recommended dose of fertilizer (RDF) during sowing. Finger millet had a NPK RDF of 50:40:25 kg per ha-1, while all legumes had an RDF of 25:50:25 kg per ha-1. nitrogen (N) was applied in the form of Urea (46% N-0P2 O5 - 0K2 O, SPIC India Fertilizer Company), phosphate (P) was applied in the form of Single Super Phosphate (normal 16% P2O5, SPIC India Fertilizer Company) and potassium (K) was given in the form of Muriate of Potash (normal 60% K2O, SPIC India Fertilizer Company).
2.6 Harvest, root colonization and spore density
Plant material in the net plot area was harvested row by row after the growing period of each individual species was completed (Supplementary Table S2). For analysis, straw and grains were separated. To determine the total biomass, grains and straw were sun-dried for ten days and subsamples were oven dried for 24 hours at 80°C. To assess mycorrhizal root colonization, roots from three randomly selected plants from either cereals or legumes per plot were harvested and pooled. Root samples were thoroughly washed with water and cleared overnight in KOH (10% w/v) at room temperature. The percentage of mycorrhizal root colonization was estimated using the gridline intersect method after staining fine and large lateral roots with trypan blue (0.05% w/v in 0.8% acetic acid solution) at 121°C for 3 minutes (Giovannetti and Mosse, 1980). AM fungal spore population was estimated using the wet sieving and decantation method (Gerdemann and Nicolson, 1963). Briefly, 50 g of sieved sun-dried soil from three randomly selected soil plots was combined with 500 mL of water. After briefly allowing heavier particles to settle, the supernatant was strained through several sieves (2-mm, 750 µm, 500 µm and 250 µm), removing larger organic materials but allowing AMF spores to pass. The solution was then stirred and allowed to settle again before being decanted through final sieves (pore sizes 125 µm and 45 µm), retaining only the last fractions. Debris were examined in a thin layer in a petri dish using a stereoscopic microscope at 25x and 50x magnifications in bright light. There was no species characterization and both colonization and spore densities were only investigated at one site (site 1) during one growing season (season 2).
2.7 Mycorrhizal growth response
MGR was determined by expressing the dry total yield of each biofertilizer treatment as a percentage of the total yield of the control treatments (Köhl et al., 2016). MGR was calculated using the following equation:
2.8 Land equivalent ratio, competitive ratio, and harvest index
LER was calculated using the following equation, where (-) refers to control treatments and (+) with biofertilizer inoculation, and Yinter to intercropping grain yield and Ymono to the respective monoculture:
CR was calculated using the following equation:
Harvest index was calculated using the following equation, where (-) refers to control treatments and (+) with biofertilizer inoculation:
2.9 Statistics
Analysis of Variance (ANOVA) was used to investigate the main effects and interactions between biofertilizer treatment, on yields, LER, CR, MGRs in relation to cropping systems, spatial arrangement, and crop mixtures across sites. Subplot was used as constant-variance random term. Individual Student’s T-tests were used to determine whether LER were significantly different from the null expectation of one. All calculations and analysis were performed in R version 4.1.0 (R Core Team, 2022). Normality assumptions were tested with histograms and plotting standardized residuals.
3 Results
Biofertilizer inoculation significantly increased AMF root colonization compared to control, independently of the cropping system and spatial arrangement in site 1 season 2 (Table 2). AMF root colonization varied across crop types independently of the legume species. When cereals in monocropping were inoculated with biofertilizer, their average root colonization increased from 38 ± 3.3% (mean ± se) in the control to 73 ± 5.1% in the biofertilizer treatment. On average, legumes in monocropping showed a similar effect with increasing root colonization from 42 ± 4% in the control to 53 ± 7.2% in biofertilizer treatment (Figure 1A). In intercropping, cereals displayed a similar colonization increase as in monocropping averaging 47 ± 2.8% in control which increased to 72 ± 2% in the biofertilizer treatments. Also, legumes in intercropping showed a general increase of root colonization from 38 ± 1.5% in the control to 56 ± 2.5% in the biofertilizer treatment. Overall, mean percentage change of root colonization after biofertilizer inoculation in monocropping was + 44.4% for cereals and + 13.3% for legumes. In intercropping, the overall mean percentage change was + 33.7% in cereals and + 23.8% in legumes.
Spore density (per 50 g soil) was also significantly affected by inoculation with biofertilizers in site 1 with no significant interactions across the other factors (Table 2). Cereal monocropping demonstrated a substantial increase in spore density after biofertilizer inoculation from 45 ± 11 spores in the control to 87 ± 3.9 in the biofertilizers treatment. Similarly, legumes in monocropping showed increasing spore densities from 23 ± 2 spores in control to an average of 76 ± 3 in the biofertilizer treatment. This pattern was consistent across individual legume species and no significant effect at species level was recorded (Figure 1B). Cereals in intercropping showed an increment from average 24 ± 1 spores in control to 72 ± 5 in biofertilizer treatment. Legumes in intercropping also exhibited an increase after biofertilizer inoculation in the spore density of 58 ± 4 compared to 24 ± 2 in control. Overall, average percentage change of the spore density after biofertilizer inoculation in monocropping was + 33.4% for cereals and + 70.3% for legumes. In intercropping, the overall mean percentage increase was + 60.8% for cereals and + 53.4% for legumes.
Biofertilizer inoculation consistently led to higher yields than the control in both monocropping and intercropping systems, regardless of crop mixture, site, or season (Table 3). Across cropping systems, cereal monocropping grain yields in control ranged from 1.48 ± 0.15 to 3.03 ± 0.30 tons per hectare, compared to 1.48 ± 0.28 to 3.40 ± 0.26 tons per hectare in biofertilizer treatments (Figure 2A). Similarly, control legumes in monocropping yielded on average between 0.46 ± 0.05 to 3.03 ± 0.30 tons per hectare, while biofertilizer treatment ranged from an average 0.52 ± 0.05 to 3.48 ± 0.78 tons per hectare. Accordingly, in monocropping percentage change after biofertilizer inoculation in site 1 was + 19.2% during season 1, + 11% during season 2 and + 5.7% in site 2 (Figure 2B). In intercropping, row spatial arrangement in control ranged between 1.27 ± 0.06 to 3.25 ± 0.18 tons per hectare compared to 1.51 ± 0.08 to 3.98 ± 0.29–18 tons per hectare in biofertilizer treatment and mosaic spatial arrangement control ranged from 1.34 ± 0.07 to 2.79 ± 0.16 tons per hectare compared to 1.59 ± 0.06 to 3.48 ± 0.18 in biofertilizer treatments. Percentage increase following inoculation with biofertilizers of intercropping systems where therefore for site 1, 29% during season 1, 23% for season 2, and 19.3% in site 2. Across crop mixtures, the highest total grain percentage increase after biofertilizer inoculation compared to respective control was 42.7% for FM X PP (+T05) in site 1, season 1 while the lowest was 3.57% for FM X LL (+T10) in site 2. Other noteworthy percentage increases included FM X CP (+T06) with 39.47% in site 2 and FM X LL (+T07) with a 27.48% increase in site 1, season 2.
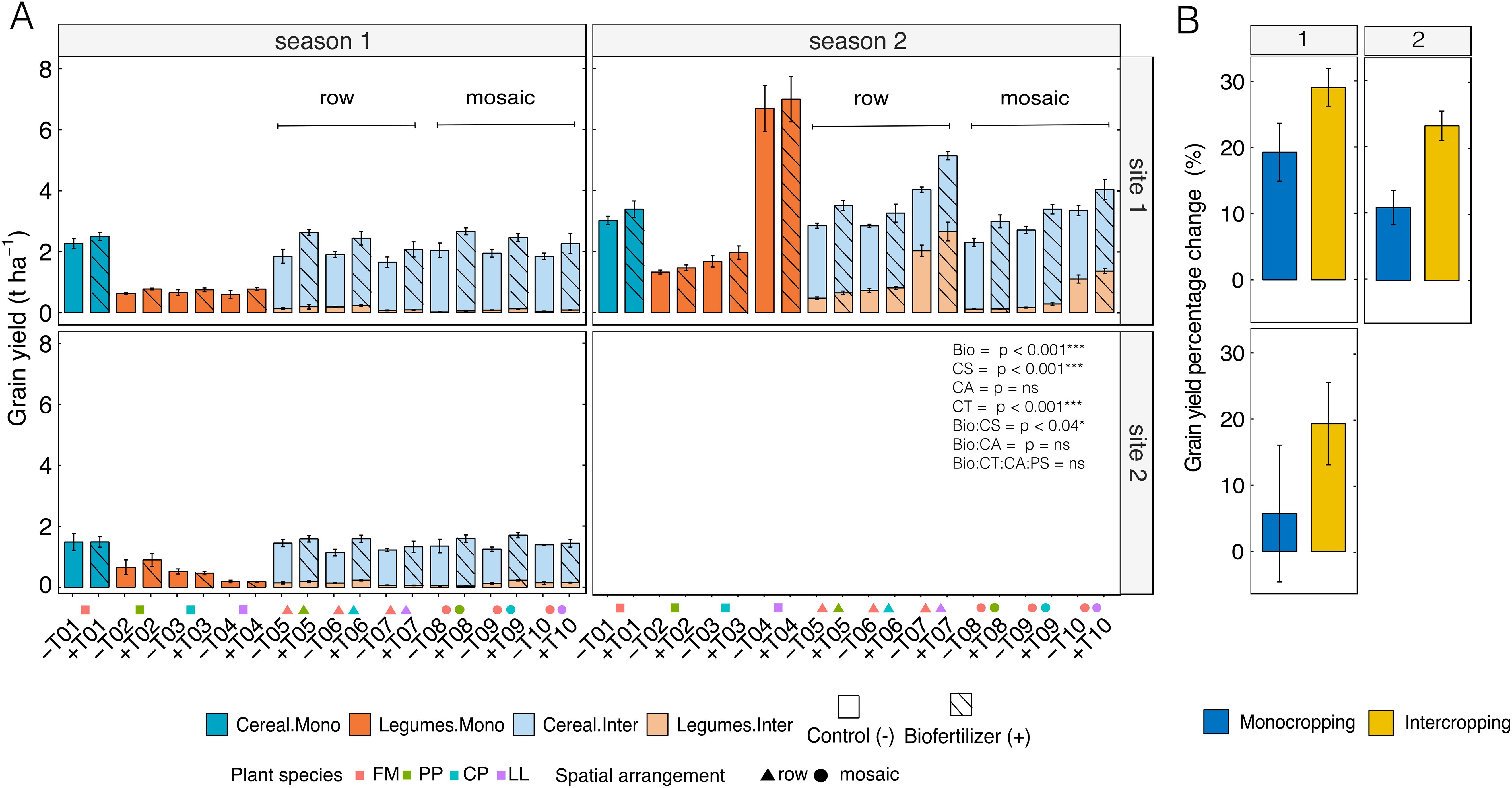
Figure 2. Grain yields under monocropping (dark shades) and intercropping (light shades) systems across two seasons (Season 1, Season 2) and two experimental sites (Site 1, Site 2). (A) shows grain yield (tons ha-1) for finger millet (cereal, blue) and legumes (orange) with control treatments (empty bars, -) and biofertilizer inoculation (striped bars, +). (B) illustrates the percent grain yield increase due to biofertilizer treatments relative to controls for monocropping (blue bars) and intercropping (yellow bars). Species abbreviations as in Figure 1. Error bars indicate SE.
The harvest index (HI) was not influenced by biofertilizer treatment, either alone or in combination with spatial arrangement or crop mixtures. While site 1 and season 1 had significantly higher harvest indexes, these effects were not associated with biofertilizer application (Supplementary Table S3). The effects of AMF-biofertilizers on total biomass in the different crop mixtures and spatial arrangements across sites and seasons were assessed using the mycorrhizal growth response (MGR). MGRs were generally positive with significant effects only across cropping systems regardless of the site and season (Table 4). For cereals, intercropping showed greater MGR than monocropping. At site 1, in season 1, row-wise intercropping increased MGR by 21.6% and mosaic by 14.6%, while in season 2, row intercropping increased MGR by 7.7% and mosaic by 11.8% compared to monocropping (Figure 3; Table 5). At site 2, cereal row-wise intercropping increased MGR relative to monocropping by 18% and 17.4% in mosaic arrangement. Legumes also showed higher MGRs than monocropping, at site 1, in season 1, row intercropping increased MGR by 15.4% and mosaic by 39.3% compared to respective monocropping, in season 2, row-wise intercropping increased legume MGR by 15.7% and mosaic by 23.4%, and at site 2, row intercropping increased MGR by 14.7% and 22.3% in mosaic arrangement. Across individual legume species, no significant effects were recorded; however, CP displayed the most pronounced change in MGR in intercropping relative to monocropping, averaging 45.3%.
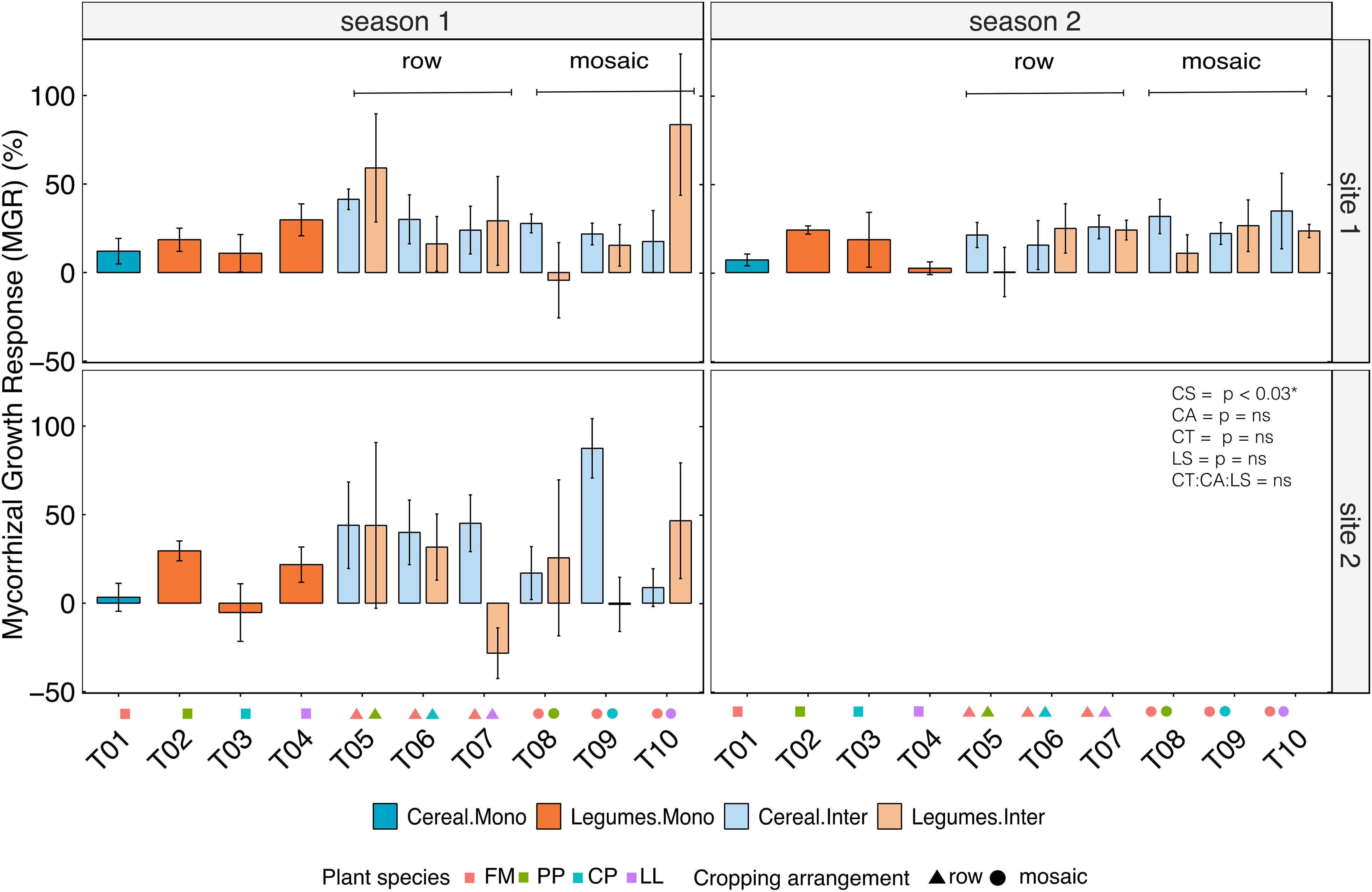
Figure 3. Mycorrhizal Growth Response (MGR) expressed as percent increase in total biomass of finger millet (cereal, blue) and legumes (orange) following biofertilizer inoculation relative to controls. Data represent monocropping (dark shades) and intercropping (light shades) systems across two experimental seasons and two study sites. Species abbreviations as in Figure 1. Error bars show SE.
The overall mean grain LER value was 1.09 pointing towards an enhanced land-use efficiency of intercropping compared to monocropping (Figure 4). The inoculation with biofertilizer resulted in significantly higher LER values than 1 through sites and seasons compared to control and, most importantly, biofertilizer inoculation increased the average LER from 1.01 in the controls to an average value of 1.17 (Table 6). This effect was not affected by the intercropping spatial arrangement nor by the crop mixture composition and their interaction (Table 7). Across sites, the highest LER values were observed in site 2, with mean control LER values of 1.12 and 1.33 for the biofertilizer treatment, while site 1 showed mean values of 0.95 for the control and 1.08 for the biofertilizer treatments. Across seasons, there was a slight improvement during season 2 for the control, ranging from LER 0.92 to 0.95, and stable values in biofertilizer treatment, maintaining a mean LER of 1.08. Mosaic spatial arrangement with biofertilizer displayed the highest LERs in site 2, with LER values of 1.18 in control and of 1.42 in the biofertilizer treatment. Despite cereals displaying higher competitiveness and suppling the highest contribution to the LERs, the biofertilizer treatment did not significantly affect the competitive ratios of either cereals or legumes nor for the interaction with intercropping spatial arrangements, crop mixtures and sites (Supplementary Table S4).
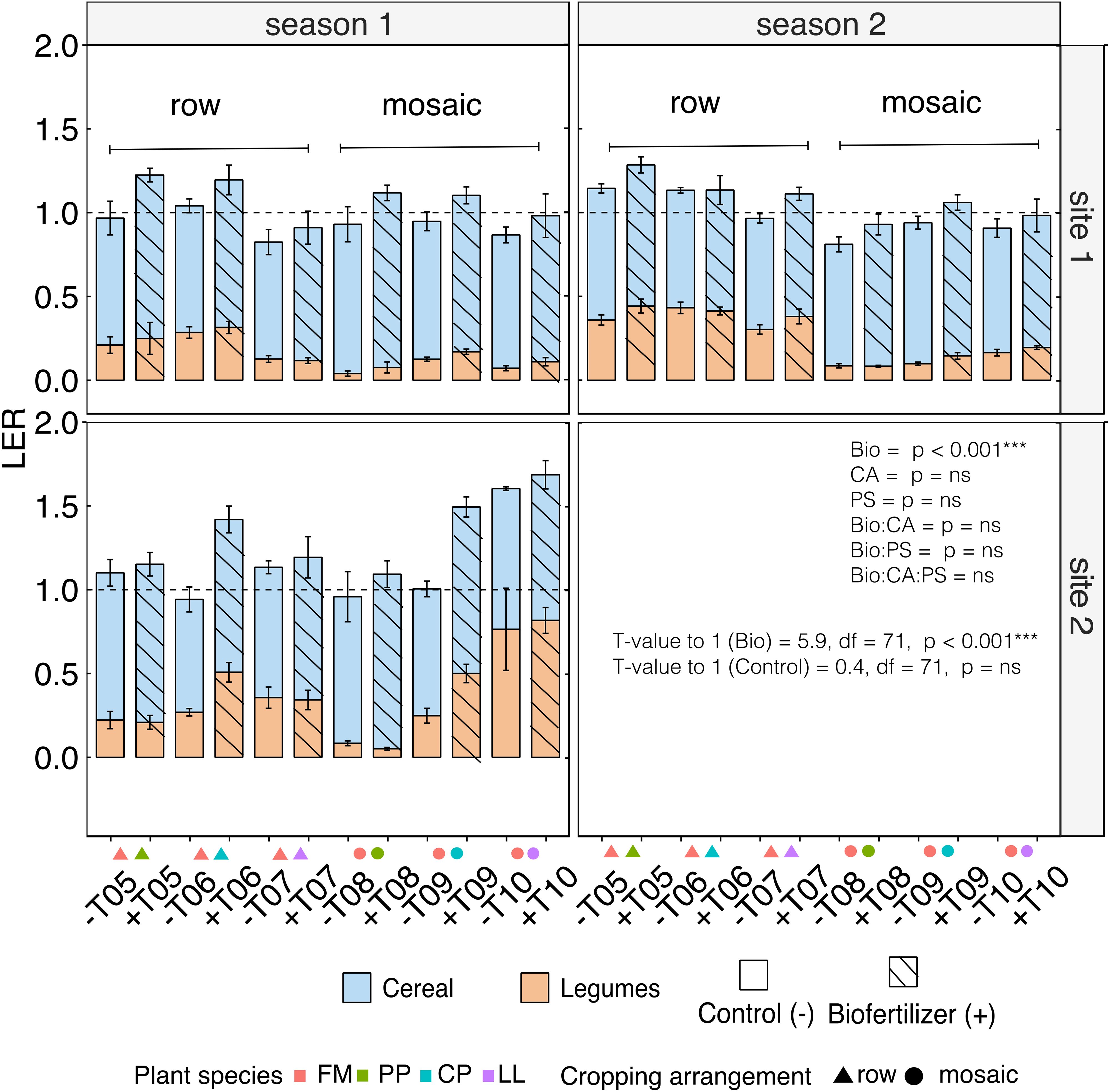
Figure 4. Land Equivalent Ratio (LER), indicating land-use efficiency, calculated for finger millet (cereal, blue) and legumes (orange) grown in row (triangle symbols) and mosaic (circle symbols) spatial arrangements. Control treatments (empty symbols, -) are compared to biofertilizer treatments (striped symbols, +). LER values greater than one indicate enhanced land-use efficiency compared to monocropping. Statistical significance is indicated based on T-tests against LER = 1. Error bars denote SE.
4 Discussion
Inoculation with biofertilizers led to a higher root colonization and spore production than observed in the controls independent of cropping system and the spatial arrangement in intercropping during one season in one site, where these variables were assessed. The percentage increase in root colonization after biofertilizers inoculation, despite background colonization, aligns with similar studies from semi-arid tropics (Mäder et al., 2011). However, they are lower than those reported in other studies, such as Bender et al. (2019), who observed up to 66% increase after biofertilizer inoculation compared to controls in maize roots in Swiss fields. AMF are non-host-specific but they generally display host preferences, in which the largest symbiotic responses are achieved by both symbionts when selected plant species are colonized by favored AMF taxa (Bagyaraj, 2014; van der Heijden et al., 2003). The AMF taxa used in this study as well as the PGPRs were previously screened and selected based on their maximum symbiotic responses for some of the crop species used in this study (Mathimaran et al., 2020). Moreover, the combined inoculation of AMF and PGPRs has been also shown to act synergistically and to lead to increased fungal colonization and spore germination (Frey-Klett et al., 2007; Mäder et al., 2011; Hoeksema et al., 2010). Stronger colonization and spore production were expected in intercropping because of the higher plant diversity. However, biofertilizer inoculation resulted in comparable increases in both cropping systems. This may be related to literature reporting that biofertilizers can alter indigenous microbial communities, often reducing native AMF colonization (Janoušková et al., 2017; Bender et al., 2019).
Grain yields increased significantly in response to biofertilizer inoculation in both cropping systems at both sites, with intercropping consistently showing greater grain yields than monocropping, independent of crop mixtures or spatial arrangements. While the precise mechanisms behind these yield improvements were not explicitly addressed in this study, higher yield after biofertilizer inoculation across both cropping systems could have been due to the well-known associated benefits of biofertilizers such as better root-growth, enhanced nutrient uptake and increased resistance to environmental stresses (Mathimaran et al., 2020; Singh et al., 2021; Daniel et al., 2022; Kumar et al., 2022). Also, biofertilizers may have enhanced yields through the formation of common mycorrhizal networks (CMNs), facilitating nutrient exchange such as phosphorus and nitrogen between intercropped plants, thus improving resource use efficiency and alleviating competition stress. While biofertilizer inoculation did not alter the harvest index, other studies suggest it may increase nutrient density in both grain and straw (Zhang et al., 2019b).
Literature suggests that the greater biofertilizer effects observed in intercropping at both sites may stem from increased soil microbial activity, stronger biotic feedbacks, and enhanced interactions between AMF and the associated microbiomes. This is likely because of the increased belowground plant diversity and the varied root architectures characteristic of intercropping systems (Wahbi et al., 2016; Ingraffia et al., 2019; Grünfeld et al., 2022). Previous studies have shown that intercropping commonly promote higher diversity of AMF species than monocropping (Lee et al., 2023). Diversity of AMF species has been shown to be an important predictor of plant productivity and is generally associated in the literature with an increase in the length of AMF extraradical hyphae resulting in a more efficient mining of the soil (van der Heijden et al., 1998; Oehl et al., 2010). Extensive hyphal networks and CMNs may also enhance exchanges between intercropped plants and their associated bacteria (Ingraffia et al., 2019). While greater yields have been reported across different crop mixtures in intercropping, and outcomes of responsiveness to AMF have been shown to vary across crop species, no clear pattern across the different legume species tested was observed. The conserved effects across crop mixtures could be attributed to the intensive breeding history of modern crops, where traits for maximum nutrient uptake are selected. This is hypothesized to have reduced the capacity of modern crops to gain full benefit from their associated soil microorganisms (Lauk and Lauk, 2008; Sawers et al., 2018). Testing combinations of more distantly related crops and those with less breeding history may provide further insights into the effects of biofertilizers on various cropping systems.
Crop-type-specific responses, where cereals displayed a greater magnitude of biofertilizer effects than legumes in intercropping, might have been partially because of the establishment of CMNs tends to be more reliant upon cereals as AMFs can generally sequester more carbon of leaf photosynthates from cereals than from legumes (Johnson et al., 2002). Moreover, larger cereal plants in this study might have applied a stronger sink strength for resources available in the established CMN leading to more pronounced growth responses, similar to the findings by Walder et al. (2012). Accordingly, the allocation of plant carbon through extraradical hyphae has been shown to influence nutrient dynamics and microbial communities in the soil which in turn might further facilitate nutrient uptake and protect against environmental stressors for both crop species in intercropping or increase the size of the underground fungal mycelium (Jones et al., 2004).
Spatial arrangements did not affect the biofertilizer effects at either site. It was initially hypothesized that different spatial arrangements would lead to varying root intermingling patterns, influencing AMF-mediated interspecific facilitation and competition, and thereby potentially increasing yields (Singh et al., 2021). While previous studies suggest that plant arrangement affects root colonization, with more heterogeneous and connected habitats enhancing AMF colonization (Grünfeld et al., 2022), in this study, the mosaic arrangement, despite its higher heterogeneity, did not result in significant effects after inoculation with biofertilizers in colonization, spore production, or grain yields. Conserved biofertilizer effects across crop mixtures and spatial arrangements may be attributed to the wide differences in soil microbial activities in the soil (Duchene et al., 2017). AMF communities are influenced by soil types, climatic conditions and the land-use intensity (Johnson, 1993; Oehl et al., 2010). In dryland soils, characteristics such as high clay content, low organic matter and reduced vegetative growth periods are frequently regarded as unstable habitats for many soil microorganisms (Parr and Papendick, 1997; Verbruggen et al., 2013; Adenan et al., 2021). Intercropping may contribute to a more favorable environment for microbial diversity and activity. Site two, characterized by lower soil nutrient levels and higher clay content compared to site one, likely experienced a shift in AMF community composition. Higher clay content, which limits root expansion and also the growth of extraradical hyphae, has been shown to favor genera such as Gigaspora over Glomus, the main component of the biofertilizers. This shift may contribute to greater instability in the microbial communities at site two, as reflected in the higher variability of biofertilizer effects (Lekberg et al., 2007; Moebius-Clune et al., 2013). The lack of AMF species identification limits predicting the general applicability of these findings, as different AMF species often differ in their effectiveness. Future studies should characterize AMF communities to enhance predictive power. For practical application, future research should also include cost-benefit analyses across diverse agroecological settings. This would help farmers evaluate economic viability and effectively integrate biofertilizer-intercropping strategies into their management practices.
In this study enhanced overyielding effects after inoculation with biofertilizers across all intercropping settings were recorded. AMF-mediated overyielding can be partially due to an easing of interspecific plant competition through AMF mediated uneven belowground allocation of resources between interconnected plants (van der Heijden et al., 2015). While biofertilizer inoculation did not significantly impact aboveground interspecific competition across crop mixtures or spatial arrangements, it likely enhanced belowground facilitation among intercropped plants. For example the acidification of legume rhizospheres, a process amplified by AMF hyphae producing extracellular phosphatases, can lead to overall greater nutrient availabilities throughout the growing seasons (Li et al., 2007; Ordoñez et al., 2016). Synergies between legumes, AMF and rhizobacteria could have also improved N fixation and interspecific nutrient transport in intercropping (Hestrin et al., 2019; Ingraffia et al., 2019; Makoi and Ndakidemi, 2009). A limitation of this study is the absence of root morphological and biochemical analyses, which could have provided deeper insights into the plant-microbial interactions underlying yield improvements. Future studies should incorporate measurements of root nodulation, and soil biochemical properties to enhance understanding of biofertilizer effects.
In summary, biofertilizer inoculation combined with intercropping resulted in higher land-use efficiencies, possibly due to increased diversity effects. This enhanced diversity likely contributed to greater nutrient availability for the entire system. These effects were consistent across different spatial arrangements and crop mixtures, suggesting a broad benefit of biofertilizers for yield improvements across cropping systems. These findings demonstrate that biofertilizers combined with intercropping enhance land-use efficiency and crop productivity. This approach contributes to sustainable agriculture by reducing dependency on mineral fertilizers and promoting resource efficiency. Future studies should validate these findings across more diverse agroecological zones, perform cost-benefit analyses for farmers, and investigate the long-term effects of continuous biofertilizer inoculation.
Author’s note
A video abstract for this article is available at: https://vimeo.com/manage/videos/1082338275/09823df9e8.
Data availability statement
The original contributions presented in the study are included in the article/Supplementary Material. Further inquiries can be directed to the corresponding author.
Author contributions
SP-B: Writing – original draft, Conceptualization, Formal analysis, Investigation. NM: Conceptualization, Project administration, Writing – review & editing. MT: Project administration, Writing – review & editing. DB: Methodology, Project administration, Writing – review & editing. AK: Conceptualization, Investigation, Writing – original draft, Writing – review & editing.
Funding
The author(s) declare that financial support was received for the research and/or publication of this article. Syngenta-PSC Fellowship -Zurich-Basel Plant Science Center. SP-B received additional support from the Department of Environmental Sciences at the University of Basel.
Acknowledgments
The authors thank the Plant Ecophysiology group at the University of Basel for their valuable discussions and support throughout the project as well as the reviewers.
Conflict of interest
The authors declare that the research was conducted in the absence of any commercial or financial relationships that could be construed as a potential conflict of interest.
Generative AI statement
The author(s) declare that no Generative AI was used in the creation of this manuscript.
Publisher’s note
All claims expressed in this article are solely those of the authors and do not necessarily represent those of their affiliated organizations, or those of the publisher, the editors and the reviewers. Any product that may be evaluated in this article, or claim that may be made by its manufacturer, is not guaranteed or endorsed by the publisher.
Supplementary material
The Supplementary Material for this article can be found online at: https://www.frontiersin.org/articles/10.3389/fagro.2025.1562589/full#supplementary-material
References
Adenan S., Oja J., Shraim A. M., Alsafran M., Tedersoo L., Zobel M., et al. (2021). Diversity of arbuscular mycorrhizal fungi and its chemical drivers across dryland habitats. Mycorrhiza 31, 685–697. doi: 10.1007/s00572-021-01052-3
Bagyaraj D. (2014). Mycorrhizal fungi. Proc. Indian Natl. Sci. Acad. 80, 415. doi: 10.16943/ptinsa/2014/v80i2/55118
Bender S. F., Schlaeppi K., Held A., and Van der Heijden M. G. A. (2019). Establishment success and crop growth effects of an arbuscular mycorrhizal fungus inoculated into Swiss corn fields. Agricult. Ecosys. Environ. 273, 13–24. doi: 10.1016/j.agee.2018.12.003
Brooker R. W., Bennett A. E., Cong W.-F., Daniell T. J., George T. S., Hallett P. D., et al. (2015). Improving intercropping: A synthesis of research in agronomy, plant physiology and ecology. New Phytol. 206, 107–117. doi: 10.1111/nph.13132
Byra Reddy M. S. and Bagyaraj D. J. (1991). The symbiotic efficiency of pigeonpea to VA mycorrhizal inoculation in an alfisol and a vertisol. Biol. Agric. Horticult. 8, 177–182. doi: 10.1080/01448765.1991.9754588
Daniel A. I., Fadaka A. O., Gokul A., Bakare O. O., Aina O., Fisher S., et al. (2022). Biofertilizer: the future of food security and food safety. Microorganisms 10, 1220. doi: 10.3390/microorganisms10061220
Duchene O., Vian J. F., and Celette F. (2017). Intercropping with legume for agroecological cropping systems: Complementarity and facilitation processes and the importance of soil microorganisms. A review. Agricult. Ecosys. Environ. 240, 148–161. doi: 10.1016/j.agee.2017.02.019
Duponnois R., Galiana A., and Prin Y. (2008). “The Mycorrhizosphere Effect: A Multitrophic Interaction Complex Improves Mycorrhizal Symbiosis and Plant Growth,” in Mycorrhizae: Sustainable Agriculture and Forestry. Eds. Siddiqui Z. A., Akhtar M. S., and Futai K. (Dordrecht: Springer Netherlands), 227–240. doi: 10.1007/978-1-4020-8770-7_10
Frey-Klett P., Garbaye J., and Tarkka M. (2007). The mycorrhiza helper bacteria revisited. New Phytol. 176, 22–36. doi: 10.1111/j.1469-8137.2007.02191.x
Gerdemann J. W. and Nicolson T. H. (1963). Spores of mycorrhizal Endogone species extracted from soil by wet sieving and decanting. Trans. Br. Mycol. Soc. 46, 235–244. doi: 10.1016/S0007-1536(63)80079-0
Giovannetti M., Azzolini D., and Citernesi A. S. (1999). Anastomosis formation and nuclear and protoplasmic exchange in arbuscular mycorrhizal fungi. Appl. Environ. Microbiol. 65 (12), 5571–5575. doi: 10.1128/AEM.65.12.5571-5575.1999
Giovannetti M. and Mosse B. (1980). An evaluation of techniques for measuring vesicular arbuscular mycorrhizal infection in roots. New Phytol. 84, 489–500. doi: 10.1111/j.1469-8137.1980.tb04556.x
Govinda Rao Y. S., Bagyaraj D. J., and Rai P. V. (1983). Selection of an efficient VA mycorrhizal fungus for finger millet: I. Glasshouse screening. Zentralblatt Für Mikrobiol. 138, 409–413. doi: 10.1016/S0232-4393(83)80038-9
Grünfeld L., Skias G., Rillig M. C., and Veresoglou S. D. (2022). Arbuscular mycorrhizal root colonization depends on the spatial distribution of the host plants. Mycorrhiza 32, 387–395. doi: 10.1007/s00572-022-01087-0
Hestrin R., Hammer E. C., Mueller C. W., and Lehmann J. (2019). Synergies between mycorrhizal fungi and soil microbial communities increase plant nitrogen acquisition. Commun. Biol. 2 (1), 233. doi: 10.1038/s42003-019-0481-8
Hoeksema J. D., Chaudhary V. B., Gehring C. A., Johnson N. C., Karst J., Koide R. T., et al. (2010). A meta-analysis of context-dependency in plant response to inoculation with mycorrhizal fungi. Ecol. Lett. 13, 394–407. doi: 10.1111/j.1461-0248.2009.01430.x
Ingraffia R., Amato G., Frenda A. S., and Giambalvo D. (2019). Impacts of arbuscular mycorrhizal fungi on nutrient uptake, N2 fixation, N transfer, and growth in a wheat/faba bean intercropping system. PloS One 14, e0213672. doi: 10.1371/journal.pone.0213672
Janoušková M., Krak K., Vosátka M., Püschel D., and Štorchová H. (2017). Inoculation effects on root-colonizing arbuscular mycorrhizal fungal communities spread beyond directly inoculated plants. PloS One 12, e0181525. doi: 10.1371/journal.pone.0181525
Johnson N. C. (1993). Can fertilization of soil select less mutualistic mycorrhizae? Ecol. Appl. 3, 749–757.
Johnson D., Leake J. R., Ostle N., Ineson P., and Read D. J. (2002). In situ13CO2 pulse-labelling of upland grassland demonstrates a rapid pathway of carbon flux from arbuscular mycorrhizal mycelia to the soil. New Phytol. 153, 327–334. doi: 10.1046/j.0028-646X.2001.00316.x
Jones D. L., Hodge A., and Kuzyakov Y. (2004). Plant and mycorrhizal regulation of rhizodeposition. New Phytol. 163, 459–480. doi: 10.1111/j.1469-8137.2004.01130.x
Köhl L., Lukasiewicz C. E., and van der Heijden M. G. A. (2016). Establishment and effectiveness of inoculated arbuscular mycorrhizal fungi in agricultural soils. Plant Cell Environ. 39, 136–146. doi: 10.1111/pce.12600
Kumar S., Sindhu S. S., and Kumar R. (2022). Biofertilizers: An ecofriendly technology for nutrient recycling and environmental sustainability. Curr. Res. Microbial. Sci. 3, 100094. doi: 10.1016/j.crmicr.2021.100094
Lauk R. and Lauk E. (2008). Pea-oat intercrops are superior to pea-wheat and pea-barley intercrops. Acta Agricult. Scandinavica Sect. B — Soil Plant Sci. 58, 139–144. doi: 10.1080/09064710701412692
Lee A., Neuberger P., Omokanye A., Hernandez-Ramirez G., Kim K., and Gorzelak M. A. (2023). Arbuscular mycorrhizal fungi in oat-pea intercropping. Sci. Rep. 13, 390. doi: 10.1038/s41598-022-22743-7
Lekberg Y., Koide R. T., Rohr J. R., Aldrich-Wolfe L., and Morton J. B. (2007). Role of niche restrictions and dispersal in the composition of arbuscular mycorrhizal fungal communities. J. Ecol. 95, 95–105. doi: 10.1111/j.1365-2745.2006.01193.x
Li L., Li S.-M., Sun J.-H., Zhou L.-L., Bao X.-G., Zhang H.-G., et al. (2007). Diversity enhances agricultural productivity via rhizosphere phosphorus facilitation on phosphorus-deficient soils. Proc. Natl. Acad. Sci. 104, 11192–11196. doi: 10.1073/pnas.0704591104
Lutz S., Bodenhausen N., Hess J., Valzano-Held A., Waelchli J., Deslandes-Hérold G., et al. (2023). Soil microbiome indicators can predict crop growth response to large-scale inoculation with arbuscular mycorrhizal fungi. Nat. Microbiol. 8, 12. doi: 10.1038/s41564-023-01520-w
Mäder P., Kaiser F., Adholeya A., Singh R., Uppal H. S., Sharma A. K., et al. (2011). Inoculation of root microorganisms for sustainable wheat–rice and wheat–black gram rotations in India. Soil Biol. Biochem. 43, 609–619. doi: 10.1016/j.soilbio.2010.11.031
Makoi J. and Ndakidemi P. (2009). The agronomic potential of vesicular-arbuscular mycorrhiza (VAM) in cereals – legume mixtures in Africa. Afr. J. Microbiol. Res. 3 (11), 664–675. Available online at: https://www.semanticscholar.org/paper/The-agronomic-potential-of-vesicular-arbuscular-in-Makoi-Ndakidemi/e5ffc92a66664d60ea12b4759a509a6bb1a21dac.
Mathimaran N., Jegan S., Thimmegowda M. N., Prabavathy V. R., Yuvaraj P., Kathiravan R., et al. (2020). Intercropping transplanted pigeon pea with finger millet: arbuscular mycorrhizal fungi and plant growth promoting rhizobacteria boost yield while reducing fertilizer input. Front. Sustain. Food Syst. 4. doi: 10.3389/fsufs.2020.00088
Moebius-Clune D. J., Moebius-Clune B. N., van Es H. M., and Pawlowska T. E. (2013). Arbuscular mycorrhizal fungi associated with a single agronomic plant host across the landscape: Community differentiation along a soil textural gradient. Soil Biol. Biochem. 64, 191–199. doi: 10.1016/j.soilbio.2012.12.014
Oehl F., Laczko E., Bogenrieder A., Stahr K., Bösch R., van der Heijden M., et al. (2010). Soil type and land use intensity determine the composition of arbuscular mycorrhizal fungal communities. Soil Biol. Biochem. 42, 724–738. doi: 10.1016/j.soilbio.2010.01.006
Ordoñez Y. M., Fernandez B. R., Lara L. S., Rodriguez A., Uribe-Vélez D., and Sanders I. R. (2016). Bacteria with phosphate solubilizing capacity alter mycorrhizal fungal growth both inside and outside the root and in the presence of native microbial communities. PloS One 11, e0154438. doi: 10.1371/journal.pone.0154438
Parr J. F. and Papendick R. I. (1997). Soil quality: relationships and strategies for sustainable dryland farming systems. Ann. Arid Zone 36, 181–191.
R Core Team (2022). R: A language and environment for statistical computing (Vienna, Austria: R Foundation for Statistical Computing).
Romero F., Argüello A., de Bruin S., and van der Heijden M. G. A. (2023). The plant–mycorrhizal fungi collaboration gradient depends on plant functional group. Funct. Ecol. 37, 2386–2398. doi: 10.1111/1365-2435.14395
Ryser P. and Lambers H. (1995). Root and leaf attributes accounting for the performance of fast- and slow-growing grasses at different nutrient supply. Plant Soil 170, 251–265. doi: 10.1007/BF00010478
Sadras V. O. (2006). The N:P stoichiometry of cereal, grain legume and oilseed crops. Field Crops Res. 95, 13–29. doi: 10.1016/j.fcr.2005.01.020
Sawers R. J. H., Ramírez-Flores M. R., Olalde-Portugal V., and Paszkowski U. (2018). The impact of domestication and crop improvement on arbuscular mycorrhizal symbiosis in cereals: Insights from genetics and genomics. New Phytol. 220, 1135–1140. doi: 10.1111/nph.15152
Schütz L., Boller T., Mäder P., and Mathimaran N. (2018). Improving crop yield and nutrient use efficiency via biofertilization—A global meta-analysis. Front. Plant Sci. 8. doi: 10.3389/fpls.2017.02204
Sekar J. and Prabavathy V. R. (2014). Novel Phl-producing genotypes of finger millet rhizosphere associated pseudomonads and assessment of their functional and genetic diversity. FEMS Microbiol. Ecol. 89, 32–46. doi: 10.1111/1574-6941.12354
Singh D., Mathimaran N., Sekar J., Ramalingam P. V., Perisamy Y., Raju K., et al. (2021). Spatial arrangement and biofertilizers enhance the performance of legume—Millet intercropping system in rainfed areas of southern India. Front. Sustain. Food Syst. 5. doi: 10.3389/fsufs.2021.711284
Smith S. E. and Read D. J. (2010). Mycorrhizal Symbiosis (San Diego, California, USA: Academic Press).
Stefan L., Engbersen N., and Schöb C. (2022). Using spatially-explicit plant competition models to optimise crop productivity in intercropped systems. Basic Appl. Ecol. 63, 1–15. doi: 10.1016/j.baae.2022.05.004
Tawaraya K. (2003). Arbuscular mycorrhizal dependency of different plant species and cultivars. Soil Sci. Plant Nutr. 49, 655–668. doi: 10.1080/00380768.2003.10410323
Unger S., Friede M., Hundacker J., Volkmar K., and Beyschlag W. (2016). Allocation trade-off between root and mycorrhizal surface defines nitrogen and phosphorus relations in 13 grassland species. Plant Soil 407, 279–292. doi: 10.1007/s11104-016-2994-y
van der Heijden M. G. A., Klironomos J. N., Ursic M., Moutoglis P., Streitwolf-Engel R., Boller T., et al. (1998). Mycorrhizal fungal diversity determines plant biodiversity, ecosystem variability and productivity. Nature 396, 69–72. doi: 10.1038/23932
van der Heijden M. G. A., Martin F. M., Selosse M.-A., and Sanders I. R. (2015). Mycorrhizal ecology and evolution: The past, the present, and the future. New Phytol. 205, 1406–1423. doi: 10.1111/nph.13288
van der Heijden M. G. A., Wiemken A., and Sanders I. R. (2003). Different arbuscular mycorrhizal fungi alter coexistence and resource distribution between co-occurring plant. New Phytol. 157, 569–578. doi: 10.1046/j.1469-8137.2003.00688.x
Verbruggen E., van der Heijden M. G. A., Rillig M. C., and Kiers E. T. (2013). Mycorrhizal fungal establishment in agricultural soils: Factors determining inoculation success. New Phytol. 197, 1104–1109. doi: 10.1111/j.1469-8137.2012.04348.x
Wagg C., Jansa J., Stadler M., Schmid B., and Van Der Heijden M. G. (2011). Mycorrhizal fungal identity and diversity relaxes plant–plant competition. Ecology 92 (6), 1303–1313. doi: 10.1890/10-1915.1
Wahbi S., Maghraoui T., Hafidi M., Sanguin H., Oufdou K., Prin Y., et al. (2016). Enhanced transfer of biologically fixed N from faba bean to intercropped wheat through mycorrhizal symbiosis. Appl. Soil Ecol. 107, 91–98. doi: 10.1016/j.apsoil.2016.05.008
Walder F., Niemann H., Natarajan M., Lehmann M. F., Boller T., and Wiemken A. (2012). Mycorrhizal networks: common goods of plants shared under unequal terms of trade. Plant Physiol. 159, 789–797. doi: 10.1104/pp.112.195727
Wang Z., Dong B., Stomph T. J., Evers J. B., van der Putten P. E., Ma H., et al. (2023). Temporal complementarity drives species combinability in strip intercropping in the Netherlands. Field Crops Res. 291, 108757. doi: 10.1016/j.fcr.2022.108757
Willey R. W. (1990). Resource use in intercropping systems. Agric. Water Manage. 17, 215–231. doi: 10.1016/0378-3774(90)90069-B
Wilson G. W. T. and Hartnett D. C. (1998). Interspecific variation in plant responses to mycorrhizal colonization in tallgrass prairie. Am. J. Bot. 85, 1732–1738. doi: 10.2307/2446507
Yu Y., Stomph T. J., Makowski D., and van der Werf W. (2015). Temporal niche differentiation increases the land equivalent ratio of annual intercrops: a meta-analysis. Field Crops Res. 184, 133–144. doi: 10.1016/j.fcr.2015.09.010
Zhang C., Dong Y., Tang L., Zheng Y., Makowski D., Yu Y., et al. (2019a). Intercropping cereals with faba bean reduces plant disease incidence regardless of fertilizer input; a meta-analysis. Eur. J. Plant Pathol. 154, 931–942. doi: 10.1007/s10658-019-01711-4
Zhang S., Lehmann A., Zheng W., You Z., and Rillig M. C. (2019b). Arbuscular mycorrhizal fungi increase grain yields: A meta-analysis. New Phytol. 222, 543–555. doi: 10.1111/nph.15570
Zhang F. and Li L. (2003). Using competitive and facilitative interactions in intercropping systems enhances crop productivity and nutrient-use efficiency. Plant Soil 248, 305–312. doi: 10.1023/A:1022352229863
Keywords: mycorrhiza, AMF, biofertilizer, intercropping, land-use efficiency, nature based solutions, biodiversity, sustainability
Citation: Pérez-Bernal S, Mathimaran N, Thimmegowda MN, Bagyaraj DJ and Kahmen A (2025) Biofertilizers enhance land-use efficiency in intercropping across crop mixtures and spatial arrangements. Front. Agron. 7:1562589. doi: 10.3389/fagro.2025.1562589
Received: 17 January 2025; Accepted: 22 April 2025;
Published: 16 May 2025.
Edited by:
Ahmed M. Abdelghany, Chinese Academy of Agricultural Sciences, ChinaReviewed by:
Eman Elremaly, Agricultural Research Center (Egypt), EgyptKipkorir Koech, Cranfield University, United Kingdom
Copyright © 2025 Pérez-Bernal, Mathimaran, Thimmegowda, Bagyaraj and Kahmen. This is an open-access article distributed under the terms of the Creative Commons Attribution License (CC BY). The use, distribution or reproduction in other forums is permitted, provided the original author(s) and the copyright owner(s) are credited and that the original publication in this journal is cited, in accordance with accepted academic practice. No use, distribution or reproduction is permitted which does not comply with these terms.
*Correspondence: Santiago Pérez-Bernal, c2FudGlhZ28ucGVyZXpiZXJuYWxAZmlibC5vcmc=