- 1Laboratorio de Estructura de Proteínas, Instituto Nacional de Medicina Genómica (INMEGEN), Mexico City, Mexico
- 2Laboratorio de Bioquímica Estructural, Escuela Nacional de Medicina y Homeopatía del Instituto Politécnico Nacional, Mexico City, Mexico
- 3Departamento de Infectómica y Patogénesis Molecular, Cinvestav, Zacatenco, Mexico City, México
Introduction: Asthma is a disease characterized by chronic inflammation of the airway mucosa that causes tissue remodeling and a reversible decrease in airflow. The causative agent of asthma is still unknown; however, several studies have shown that environmental factors such as allergens present in pollens are involved. This project's objective was to develop and evaluate a model of respiratory hypersensitivity in Vietnamese minipigs, which is closer in many aspects to humans than rodents, using Phleum pratense allergenic pollen extract.
Methods: In this hypersensitivity model, human-like signs were observed during a challenge with the allergens. Intradermal and passive anaphylaxis tests confirmed that specific IgE mediated the response.
Results: Significant changes in lung tissue remodeling, high levels of serum allergen-specific IgA, IgG, and to a lesser extent IgE were found in the sensitized pigs, which could favor tolerance and pathogenesis. However, since chronic pathology did not develop, elevated levels of cytokines were not proven.
Discussion: This work demonstrated that the immunization protocol in this experimental model can induce a type I respiratory hypersensitivity-like response mediated by antigen-specific IgE, with pathophysiological similarities to those of humans and prospective for translational basic and applied research.
1 Introduction
Asthma is a disease characterized by chronic airway mucosal inflammation and bronchial hyperresponsiveness, which results in tissue remodeling and reversible airflow reduction (1). Asthma has diverse clinical manifestations, highlighting episodes of repeated shortness of breath and wheezing, which are at least partially reversible, recurrent cough, and excess airway mucus production (2). The World Health Organization (WHO) estimates that this disease affects 262 million people and currently causes 455,000 asthma deaths per year (3), with a prevalence of 15% in first-world countries, while studies in Latin America have revealed figures ranging from 5.7%–16.5% in the population (4).
Clinical and laboratory data show asthma can be classified as nonallergic (intrinsic) or allergic (extrinsic). Non-allergic asthma is typically present in adulthood and is more severe but is not associated with elevated serum allergen-specific IgE titers, as it is often associated with nasal polyposis and rhinitis (5). Allergic asthma usually begins in childhood and is characterized by dependence on a constant allergen stimulus, positive skin tests, and elevated serum allergen-specific IgE titers. At present, the precise pathogenesis of allergic asthma is still unknown, but several studies have shown that environmental factors such as allergens are involved (6). Allergens are perceived by the body as harmful, which triggers an exaggerated reaction of the immune system, aeroallergens such as grass pollen are one of the main triggers of asthma exacerbations (7). Grass pollen from Phleum pratense is one of the most widespread grasses in the world and has been linked to allergic processes, asthma, and even hay fever (8). It is estimated that 80% of asthmatic children and 40%–50% of asthmatic adults have allergic mechanisms caused by allergens (9).
Currently, experimental animal models of asthma, primarily developed in mice and rats, have become an indispensable tool for histological and molecular research on this disease; however, due to structural differences as well as the size of the organs compared to humans, it has not been possible to deepen research where, for example, the drugs and allergen-specific immunotherapy dosages are not easily extrapolated from these animal models to humans (10–13). Pigs are most like humans in terms of anatomy, immunology, biochemistry, physiology, size, and genetics (14–18). The pig has been used as an animal model for cystic fibrosis, as porcine lungs have marked similarities to humans in tracheobronchial tree structure, lung physiology, airway morphology, abundance of airway submucosal glands, and glycoprotein synthesis patterns, allowing the model to be translational for various pathologies present in humans (18). Therefore, this model would help to elucidate some main unknowns of asthma, for example, to identify the early pathogenesis of the disease, the kinetics of the humoral response from the first contact with the antigen to the development of sensitization where the antibody response changes from classic IgG and IgA to an IgE profile, to show how tissue remodeling is generated, including the molecular mechanisms by which the allergen crosses the mucosal barrier, etc.
The porcine hypersensitivity model would allow testing new asthma treatments and desensitization protocols, making the dosage extrapolation easier due to its similar weight and size to humans (19, 20). Surprisingly, the pig has been used as a model for asthma only in limited instances, mainly using ovalbumin sensitization models, leaving aside the use of clinically relevant allergens in the development of asthma (21).
Hence, this research aimed to validate the porcine model of respiratory hypersensitivity to a clinically relevant human allergen such Phleum pratense, using a novel sensitization protocol to induce the early development of the serum and mucosal response to the allergen, evaluating key clinical signs and features of an IgE mediated response to elucidate the suitability of the model for the study of asthma.
2 Materials and methods
2.1 Animals
Thirty-two weaned male and female specific pathogen-free (SPF), Vietnamese mini-pigs (28 days of age) from the Experimental Animal Production Unit (UPEAL-Cinvestav) were used. They were provided ad libitum access to food and water and maintained in conditions under Mexican government regulations (NOM-062-ZOO-1999). The Institutional Committee for the Care and Use of Laboratory Animals (CICUAL) of Cinvestav approved the experimental protocol (0143-15).
2.2 Allergen sensitization protocol
The allergenic antigens came from a standardized protein extract of Phleum pratense (Standardized Timoty Grass, Alk Abello Pharm, USA, 100,000 BAU/ml, Lot 0001638321). Aluminum hydroxide [Al (OH)3] 64 mg (4.0 g/100 ml), (Alergel, suspension, 431M98 SSA, ARLEX Mexico, S.A. de C.V.) was used as an adjuvant. The immunization protocol was a modification of a pig allergen-induced lung inflammation (21) and a mouse respiratory system sensitization protocol (22). Pollen-treated animals received 1 mg of total protein from allergenic pollen extract+64 mg aluminum hydroxide per dose and control animals received the adjuvant and phosphate solution (PBS) only. The animals were divided into the following experimental groups (n = 32): Experiment I: Five pigs were immunized to evaluate infiltrating cells in the intradermal and mucosal areas and 6 control pigs; experiment II: Eleven immunized pigs to assess clinical signs and the cytokine profile at different times and 10 control pigs. Two additional pigs were used for IgE production and purification, to immunize mice to obtain porcine anti-IgE antiserum.
The routes of administration varied as the experiment progressed, as shown in the immunization scheme (Figure 1). The immunization protocol started on day 28 of birth (one week after weaning). Pigs were humanely euthanized at the end of the protocol.
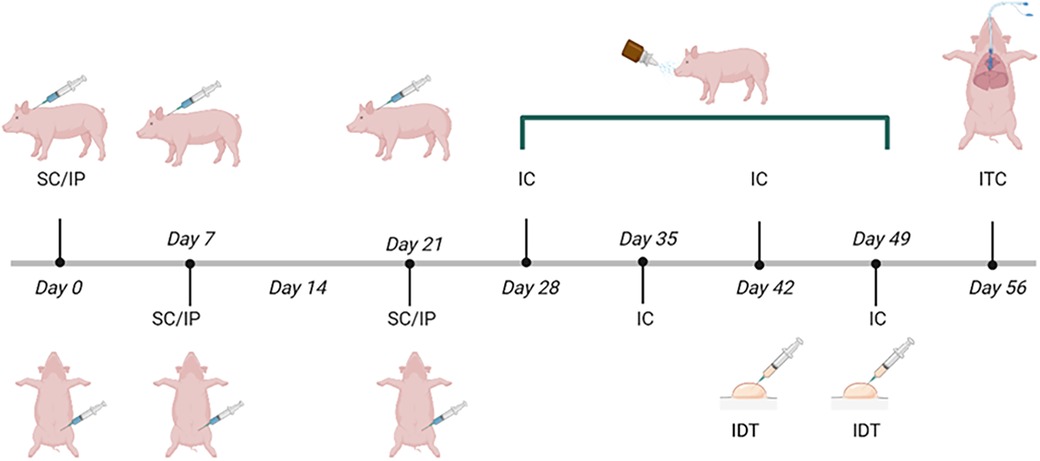
Figure 1. Immunization scheme with Phleum pratense pollen extract in Vietnamese minipigs. The onset of immunization (day 0) was at 28 days of age. The dose [1 mg proteins from pollen +64 mg Al(OH)3] was administered subcutaneously (SC) behind the ear and intraperitoneally (IP) on days 0, 7, and 21, intranasal challenges (IC) were performed on days 28, 35, 42 and 49. Intradermal Test (IDT) was performed on days 42 and 49. An intratracheal challenge (ITC) was carried out on day 56. Control groups only received adjuvant [64 mg Al (OH)3 + PBS].
2.3 Subcutaneous, intraperitoneal, and intranasal immunizations
The dose [1 mg of proteins from pollen +64 mg Al (OH)3] was administered both subcutaneously (SC) behind the ear and intraperitoneally (IP), with subsequent intranasal (IN) challenges, based on a modification of the protocol reported by Mitchell et al. (21) and our previous experience with mucosal immunization (23–25). After immunization, vital signs, including heart and respiratory rates, were monitored to detect possible adverse effects such as discomfort or anaphylaxis. In addition, the application site was monitored for induration, redness, or itching. Control pigs received only the adjuvant [64 mg Al (OH)3 + PBS] under the same conditions. A total of 3 immunizations were applied on days 0, 7, and 21 (Figure 1). Intranasal instillations were performed with a 1 ml needleless syringe. Pollen-immunized pigs received a dose of 100 μg of pollen extract adjusted to a final volume of 500 μl with PBS, control pigs were instilled with 500 μl of PBS.
2.4 Intradermal testing and biopsy
The ventral area of the pigs was divided into quadrants to locate the area of application. PBS (200 μl) was administered as a negative control in one quadrant and pollen extract (100 μg of extract in 200 μl of PBS) in another. The test was read about 15 min post-application. A classical positive response was identified by erythema and induration. Skin biopsy collection with a sterile punch (Harris, Uni-Core 8.00 No. 7093508) was taken at the intradermal test site in all experimental animals after anesthesia with intramuscular (IM) Azaperone (2 mg/kg) (Sural, Laboratorios Chinoin. Mexico) and intravenous (IV) Tiletamine-Zolazepam (4.2 mg/kg) (Zoletil, Laboratorios Virbac, Mexico). Control pigs were tested under the same conditions 24 h before euthanasia to avoid prior pollen extract exposure. The intradermal test and skin biopsy were adapted and modified in our experimental model based on that previously described by Raszyk (26) and Nischal et al. (27).
2.5 Intratracheal challenge
The intratracheal challenge was performed in groups I and II using an orotracheal tube (Portex, oral/tracheal siliconized, 4.0 mm, Smiths Medical International Limited, UK), prior anesthesia and sedation with Azaperone (2 mg/Kg) and Tiletamine-Zolazepam (4.2 mg/Kg). The probe was introduced until reaching the bifurcation of the trachea and pollen extract (2 mg in 3 ml of PBS) was administered. At the end of the procedure, the probe was carefully removed, and the pigs were kept under constant surveillance and monitoring of signs (heart and respiratory rate) until their complete recovery. The procedure was adapted to our porcine respiratory hypersensitivity model based on that previously described by Nienhoff (28) and Judge et al. (18).
2.6 Passive cutaneous anaphylaxis (PCA) test
Sera from the pigs immunized with pollen proteins were collected, and an aliquot of each serum was inactivated at 56°C for 4 h, to determine whether the immune response was IgE mediated. Under the same conditions, sera from pigs unrelated to the project and PBS were used as negative controls. For the PCA test, two weaned pigs not included in the immunization protocol were employed. A volume of 200 μl of each test, and control sera were intradermally injected at different well-identified sites distributed along the ventral area of each animal. After 20 h, 1 mg/kg body weight of pollen extract was administered intravenously. A positive reaction, characterized by erythema and induration, was observed in about 15 min at the injection site (29, 30).
2.7 Sample collection
Blood, saliva, and nasal mucus samples were collected. A comprehensive review of the available literature was conducted to ascertain the optimal sampling times for Group II (31–33). Samples were taken before (time 0) and after immunization (5 and 24 h), to ensure the detection of cytokines released after the stimulus.
Blood samples (3–5 ml) were obtained by puncture in the jugular vein, these samples were centrifuged at 4,000 RPM to obtain serum, which was then aliquoted into microtubes and stored at −80°C until further analysis. Nasal mucus and saliva samples were collected using cotton swabs and kept on ice, for subsequent centrifugation at 4,000 RPM for 10 min. Protease inhibitors (PMSF, TPCK, and TLCK) were added to the mucosal sample for proper preservation, aliquoted, and stored at −80°C, as described elsewhere (23).
2.8 Euthanasia
All the animals in the experiment were humanely euthanized by prior anesthesia with intramuscular Azaperone (2 mg/kg) and intravenous Tiletamine-Zolazepam (4.2 mg/kg) until fully unconscious, followed by exsanguination severing the major blood vessels in the neck (carotid arteries and jugular veins) to induce rapid bleeding, accordingly with our protocol 0143-15, approved by our CICUAL.
2.9 Bronchoalveolar lavage (BAL) and determination of cellular infiltrates in the respiratory tract
After euthanasia, the lungs and trachea were dissected, pre-washed to remove residual blood, and processed as previously described (34). Briefly, the bronchoalveolar lavage (BAL) was obtained by ligating the trachea inserting a catheter in the first bronchial bifurcation and instilling 50 ml of PBS. The collected BAL fluid was centrifuged at 2,000 RPM for 10 min at 4°C. Cell counting was performed with an automated counter (Countess Automated Cell Counter C10227, Invitrogen, Carlsbad, CA. USA). Finally, the samples were diluted to obtain a total of 150,000 cells in a final volume of 80–100 μl and cytocentrifugation was performed at 2,000 rpm/10 min at room temperature (Cell Preparation System Cytospin 3, Shandon No. 7400001, USA), stained with Kwik Diff reagent (Thermo Fisher Scientific Kit Kwik Diff No. 9990700, USA), and a differential cell counting was performed with optical microscopy.
2.10 Morphological and histopathological analysis
Macroscopic morphological analysis of the lungs was performed to identify tissue damage, analyzing the area by planimetry using photographs of the dorsal and ventral views of the lungs with a computer-assisted image analyzer (Image J ver 1.54 h). Cuts in the lung were made at the level of the bronchus in the left lung parenchyma and another longitudinal cut from the apical to the basal zone. Lung samples and skin biopsies were fixed in 4% formalin for 24 h or in Carnoýs fixative for 16 h. The tissues were paraffin-embedded, cut in a microtome at 3 µm thick, and stained with toluidine blue (mast cells) or chromotrope 2R (eosinophils). The samples were observed under light microscopy (Nikon Eclipse E400, Japan) and the density of cells was recorded using a computer-assisted image analyzer (Image Pro Plus 7.0 software). The technique was based on our own experience (23) and the protocol described by Gandjeva et al. (35).
2.11 Determination of cytokines
Serum cytokine levels for TGFβ, IL-4, IL-6, IL-10, and IFNγ were determined by ELISA assays at 0, 5, and 24 h post intratracheal challenge. A commercial multiplex ELISA Kit (Porcine Cytokine Magnetic Bead Panel, Milliplex®) was used, at 1/5 serum dilution for TGFβ and neat samples for IL-4, 6, 10, and IFNγ. A commercial ELISA kit (Porcine IL-13, Ray Biotech, Inc.) and a 1/4 sample dilution was used for IL-13 determination. In all cases, the procedure recommended by the manufacturer of each kit was followed.
2.12 Obtention and characterization of porcine IgE
Two independent adult mini pigs were subcutaneous and intraperitoneally immunized at days 0, 7, 14, and 28 with Taenia hydatigena parasitic protein extract (746 μg/ml of Taenia hydatigena protein extract +Al (OH)3, kindly provided by Dr. Fernando Alba-Hurtado (FES-Cuautitlan-UNAM, Edo. de Mexico) to induce reaginic antibody production. The serum was obtained at the end of the experiment (day 35) after the animals were humanely euthanized. The serum was threefold precipitated with a 33% saturated ammonium sulfate solution (36, 37). The purity was verified by 12% polyacrylamide gel electrophoresis. Porcine anti-IgM (Clone 5C9) and anti-IgA (Clone F9) mice monoclonal antibodies were coupled to cyanogen bromide-activated Sepharose (CNBr-Activated Sepharose 4 Fast Flow GE Healthcare, Germany), and a Protein G column (Protein G Sepharose®, Fast Flow, Germany) coupled with Sepharose, were used to remove porcine IgM, IgA and IgG, respectively. IgE characterization was performed using native SDS-PAGE and Western blot techniques to confirm purity and molecular weight identification (38).
Subsequently, purified IgE bands were excised from polyacrylamide gels for immunization of BALB/c mice according to standardized protocols to obtain porcine anti-IgE mouse antiserum (39, 40). The optimal dilution of the mouse porcine anti-IgE antiserum was determined by serial dilution using a pre-immune serum as a reference and comparing optical densities. The specificity of the antiserum was evaluated by Western blotting and IgE was specifically identified. Two weaned pigs that were not part of the protocol were used for the PCA test as previously described in this study.
2.13 Quantitative determination of allergen-specific IgA, IgG, and IgE
Antigen-specific ELISA assays were performed to measure IgA, IgG, and IgE concentrations in pig serum, saliva, and nasal mucosal samples. Briefly, 96-well ELISA microplates were sensitized with 0.2 μg (2 µg/ml) of the commercial extract of Phleum pratense per well. For immunodetection, peroxidase-coupled anti-porcine IgG and IgA antibodies (goat anti-Pig IgG-Fc Fragment Antibody Affinity Purified, A100-104 and goat anti-Pig IgA Antibody Affinity Purified, A100-102, Bethyl, USA), were used by the protocols previously described for this technique and our patented method (22, 23, 25, 41, 42).
Previously, a linear regression analysis was performed to compare the commercial porcine IgE standard with the one obtained in this study. The optimal conditions for antigen coating concentration (Phleum pratense) per well were also determined. IgE quantification was performed with the mouse anti-pig IgE antiserum obtained in this work at a 1/500 dilution, for immunodetection an anti-mouse IgG antibody coupled to HRP was used at a 1/1,500 dilution (HRP Goat anti-mouse IgG minimal x-reactivity BioLegend Cat 405306, USA). The 3,3′,5,5,5,5′-tetramethylbenzidine (TMB) (Sigma-Aldrich®) was used as substrate and the absorbance was read at 450 nm after adding 1M HCl to stop the peroxidase reaction.
3 Results
3.1 Intradermal reaction (IDR)
All the pollen extract-sensitized pigs were positive for the intradermal test performed on days 43 and 50 of the protocol (Figure 2A), the control group did not show any reaction (Figure 2B). The positive test was characterized by a rapid erythema reaction (observed within approximately 15 min) and, in some cases, induration in the areas tested with the pollen extract (Figure 2C).
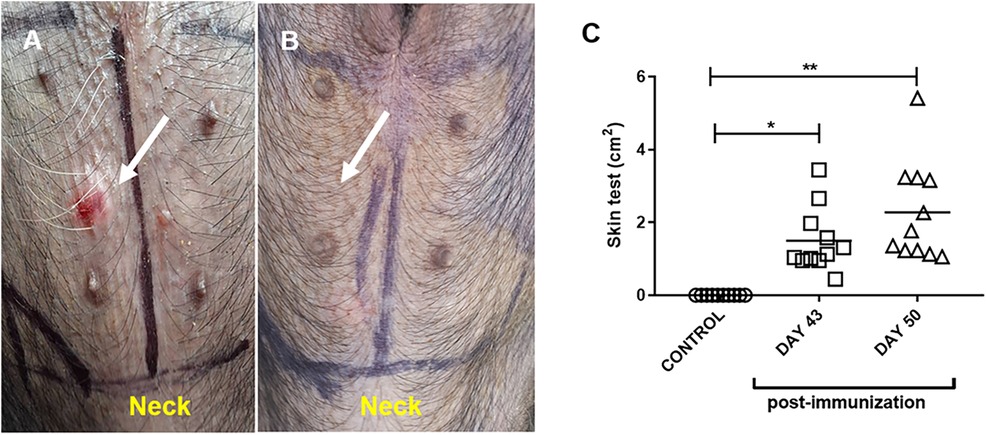
Figure 2. Representative intradermal test in pollen-sensitized (A) and control (adjuvant-only treated) (B) pigs. The arrow indicates the area of ID induration after 15 min of inoculation. (C) Area of erythema and induration in control (adjuvant) and pollen-sensitized (day 43 and day 50 PI) pigs after pollen inoculation. Each symbol represents one pig in two independent experiments. Horizontal bars represent the mean (n = 11). Significance calculated by Unpaired t-test. *p ≤ 0.05, **p < 0.01.
3.2 Passive cutaneous anaphylaxis (PCA) test
Sensitization to pollen extract was confirmed by a passive cutaneous anaphylaxis (PCA) test, to evaluate whether the hypersensitivity response was mediated by specific IgE against pollen. Only positive responses were seen with fresh sera from sensitized pigs, presenting erythema within a few minutes after the test. Heat-inactivated positive sera gave a negative result, showing that the reaction was due to thermo-sensitive specific IgE for pollen. Fresh and inactivated sera from control pigs gave a negative PCA reaction (Figure 3).
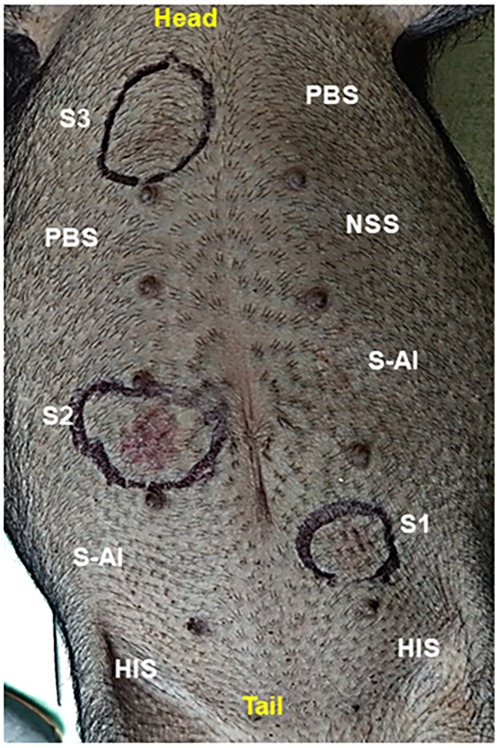
Figure 3. Passive cutaneous anaphylaxis (PCA) reaction in a naive non-sensitized pig. S1, S2, and S3 = fresh sera from pollen-sensitized pigs; HIS = heath-inactivated pollen-positive serum; NSS = non-sensitized pig serum; S-Al = pig control serum; PBS = inoculation control. After 24 h of the ID inoculation, the pig received intravenously 1 mg/Kg of pollen extract. An erythematous reaction (encircled) was evident within 15 min after administration.
3.3 Intratracheal challenge
In pigs immunized with pollen, clinical signs of anaphylactic reaction (bronchospasm, bilateral wheezing, intercostal twitching, peripheral cyanosis) were observed within minutes after the orotracheal administration of pollen extract. In one of the sensitized pigs, anaphylactic shock led to death (Table 1). The macroscopic morphology of the lungs of the pollen-treated pigs was analyzed and the presence of reddish multifocal stippling in different areas of the organ (petechial hemorrhages) was observed, in addition to the presence of tissue consolidations. The damage represented an average of 18% of the total visible area in both (ventral and dorsal) sides of the lung, finding a higher incidence of deterioration in the cranial and intermediate lobes with a significant difference (p ≤ 0.01) compared to the other experimental conditions (Figure 4). No tissue damage was observed in the control groups.
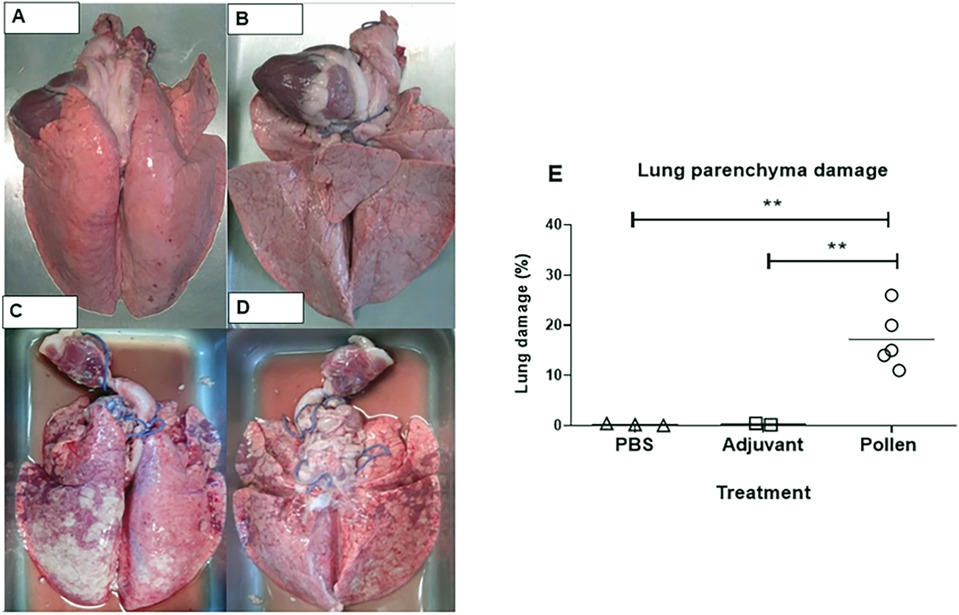
Figure 4. Representative dorsal and ventral images of lungs from control (A,B) and pollen-treated (C,D) pigs. (E) Percentage of tissue damage in lungs of control (PBS and Adjuvant, n = 3 each) and experimental (n = 5) pigs. Each figure represents one pig. Significance calculated by Unpaired t-test. **p ≤ 0.01.
3.4 Cellular infiltrates in bronchoalveolar lavage (BAL)
The bronchoalveolar lavage (BAL) allowed us to identify the different cell populations in each experimental condition on a proportional and representative basis. In control pigs, macrophages and epithelial cells were normally found whereas in the pigs immunized with pollen, in addition to the presence of these cells, a significant increase in eosinophils was demonstrated (Figure 5), suggesting an inflammatory reaction caused by bronchial hyperreactivity and tissue remodeling. In contrast, the percentage of lymphocytes, neutrophils, and mast cells was not significantly different from control pigs (data not shown).
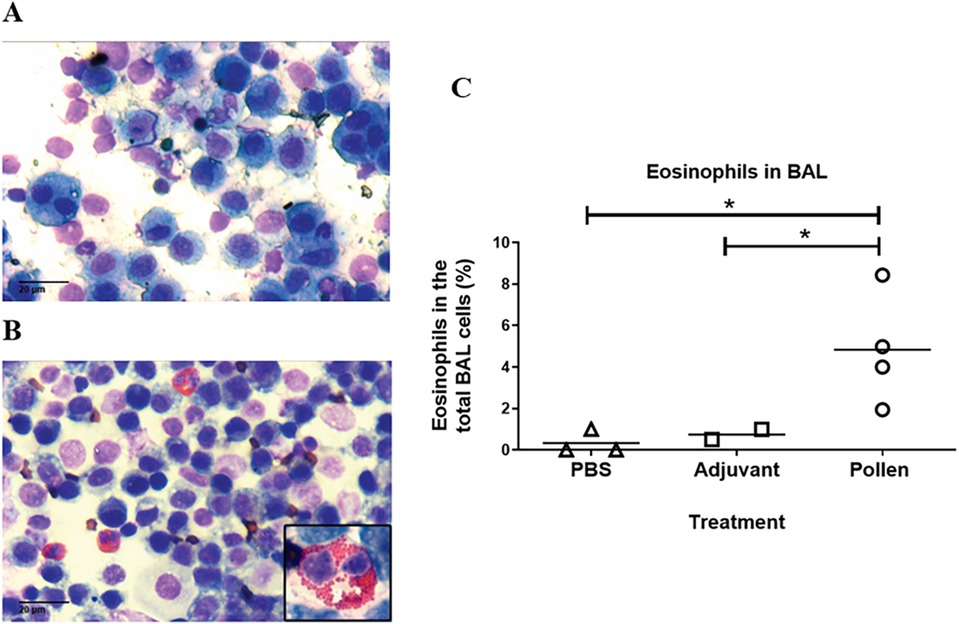
Figure 5. Representative bronchoalveolar lavage (BAL) in control (A) (PBS) and pollen-sensitized (B) pigs (40X, inset 100X). (C) Eosinophils in BAL from control (PBS and adjuvant) and pollen-sensitized pigs Each symbol represents an animal. Significance calculated by Unpaired t-test. *p ≤ 0.05.
3.5 Histopathological analyses of lungs
Analysis of lung tissue from control pigs revealed a healthy structure of the bronchial parenchyma, well-defined alveolar spaces, and no damage (Figures 6A,C). Tissue from pigs treated with the pollen extract showed significant epithelial restructuring, bronchial congestion, increased mucus within the bronchi and decreased alveolar spaces (Figures 6B,D). In addition, degranulation of the infiltrate of both cell types was observed in the lung tissue of pollen-sensitized pigs (Figure 6D inset). The cell density was determined in each of the microscopic fields, calculating the total number of mast cells stained in blue-purple and eosinophils stained in red, per square millimeter of tissue. The area of bronchial epithelium and alveolar spaces were excluded to homogenize the count. Statistical analysis revealed a significantly increased infiltrate of these cells in the lung tissue of pollen-sensitized pigs compared to control pigs (Figure 6E).
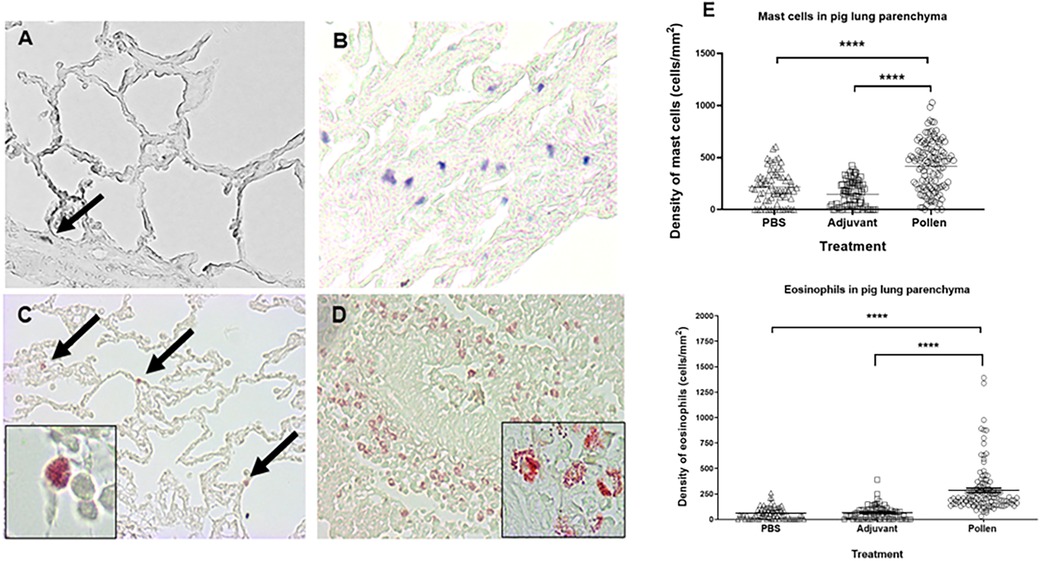
Figure 6. Histological images of lung parenchyma from control (A,C) and pollen sensitized (B,D) pigs (40X magnification). Toluidine blue mast cell staining (A,B) and chromotrope 2R neutrophil staining (C,D). Insets (100x) show eosinophils in diverse stages of degranulation. (E) Density (cells/mm2) of mast cells and eosinophils in lung parenchyma. Each symbol represents the count of a microscopic field of at least 5 animals per group. Significance calculated by Unpaired t-test. ****p ≤ 0.0001.
3.6 Serum cytokine profile
The cytokine response between pollen-sensitized and control pigs at 0, 5 and 24 h post-intratracheal challenge showed no significant difference in interleukins 4, 10, and 13 (data not shown) while for TGFβ and IFNγ, significant reductions were observed at various times (p ≤ 0.05) (Figure 7). In the case of IFNγ, the response decreased significantly at 5 h in both experimental groups concerning the time of 0 h (p ≤ 0.05) (Figure 7A), in TGFβ a significant decrease was found at 5 h and 24 h concerning the time of 0 h in both experimental groups (p ≤ 0.05) (Figure 7B).
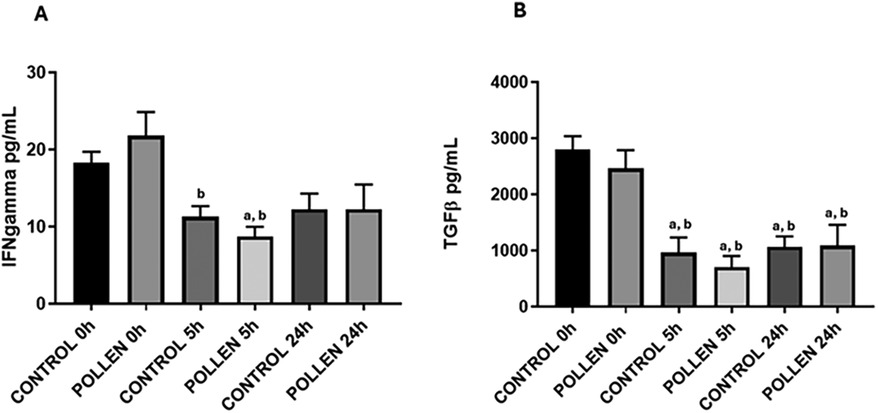
Figure 7. Serum IFNg (A) and TGFb (B) cytokine profile of control (n = 10) and sensitized (n = 11) pigs. Data expressed as mean ±SEM. Significance calculated by one-way ANOVA analysis, and Tukey's multiple analysis. ap ≤ 0.05 vs. control 0 h, bp ≤ 0.05 vs. pollen 0 h.
3.7 Quantitative determination of allergen-specific IgA, IgG, and IgE
Analysis of the production of different isotypes of specific antibodies against pollen proteins showed significant differences in serum, nasal mucus, and saliva between sensitized pigs and the control group. Only sensitized pigs showed anti-pollen IgG and IgA antibodies in serum reaching a maximum at day 49 post-immunization. Serum IgE was detected at low levels until day 49, but, in contrast to IgG and IgA, its concentration kept rising over time (Figure 8A). In nasal mucus and saliva samples, a significant difference was observed in the production of IgA and IgG antibodies in sensitized pigs compared to the control group. In contrast, IgE was not detected in these samples (Figures 8B,C).
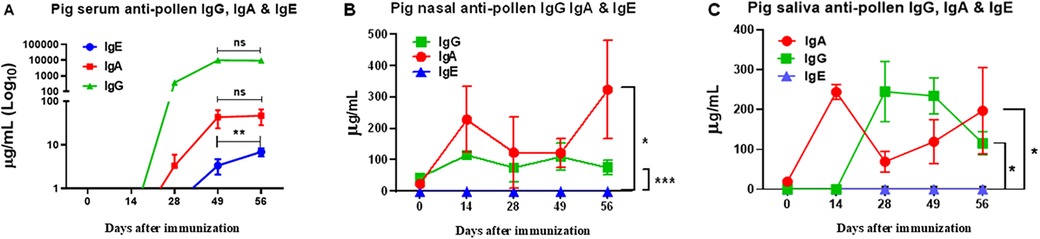
Figure 8. ELISA quantitative determination of allergen-specific IgA, IgG, and IgE in serum (A), nasal (B), and saliva (C) samples of pollen-sensitized pigs. Data expressed as mean ±SEM (n = 12). Significance calculated by one-way ANOVA analysis, and Tukey's multiple analysis. **p < 0.01 between dates in (A) *p ≤ 0.05 and ***p ≤ 0.001 vs. control groups in (B,C) Control groups were all negative (not shown). The graph scales are different.
4 Discussion
Asthma is a chronic disease that has a significant impact on the quality of life of the world population, particularly by its high incidence in children, adolescents, and older adults (41). The pig has great similarities with human anatomy and physiology, making it an experimental model that could provide information to complement the data obtained in more conventional models such as the mouse, rat, rabbit, and guinea pig (43), especially for pre-clinical studies.
The porcine respiratory tract has been accepted as a translational model for respiratory research (16–18), which offers the advantage of deepening studies in public health problems such as asthma and respiratory allergies. The bronchial structure and ventilatory patterns of pigs allow assessment of lung function with clinical techniques applicable to humans, such as spirometry and bronchoscopy. Furthermore, allergen-induced airway inflammation and remodeling in pigs more accurately reflect the human asthmatic phenotype compared to rodents (16, 19). Although their cost and handling are higher than murine models, this is compensated for the quantity and quality of the data obtained, as the porcine model provides more detailed information due to its higher biological complexity. It also allows for more advanced techniques and the collection of larger sample volumes, which improves the reproducibility and depth of the analyses.
Currently, this is the first study to establish a model of respiratory hypersensitivity in Vietnamese pigs and our findings provide an initial basis for future research on the applicability of this model in allergy studies. Therefore, in this study a novel immunization protocol was carried out in our experimental model (Vietnamese mini-pig), using Phleum pratense allergenic pollen extract, to induce a type-I-like respiratory hypersensitivity, to provide a model to future investigate the onset, development, diagnosis, treatment and prevention of such pathology. In our model, the intradermal reaction demonstrated an antigen-specific IgE-mediated response. This phenomenon is due to the degranulation of mast cells, which, during the process of immunization with Phleum pratense pollen, became sensitized with specific IgE against the pollen antigens, which bind to their high-affinity receptors on the mast cell membrane. Thus, when the test is performed and the antigen is administered, it interacts between two adjacent IgE, causing receptors cross-linking and, consequently, activation and degranulation of mast cells, releasing proinflammatory mediators that generate erythema and induration, as observed in our model. Moreover, these results are consistent with what has been reported in animal models with food allergy and intradermal tests used for allergy diagnosis in humans (44–47).
On the other hand, passive cutaneous anaphylaxis is a classic test used to confirm antigen-specific IgE-mediated responses. Heat inactivation allows the identification of the nature of the antibody involved. In our work, fresh sera from sensitized pigs gave a positive skin reaction, but a negative response was seen when these sera were heat-inactivated (Figure 3), confirming that an anti-Phleum pratense IgE mediated the response (47, 48). In the intratracheal challenge, clinical signs were highly variable in the porcine model as in an open human population. However, it was possible to discern between the effects caused by pollen instillation in control pigs (pulmonary edema only) and pollen-sensitized pigs, in which an exacerbated reaction was obtained upon challenge, with a series of clinical manifestations corresponding to anaphylactic shock (49, 50) (Table 1). The ability to observe and measure such clinical signs in pigs offers a significant advantage over rodent models, where these signs, particularly those indicative of severe allergic reactions, can be difficult to quantify or manifest less reliably (51, 52). For example, in rodents, anaphylactic reactions often require invasive procedures to accurately measure vital signs, which can be challenging or ethically controversial. In contrast, the porcine model allows non-invasive and more accurate real-time monitoring of clinical parameters such as heart rate, respiratory rate and blood pressure, which are critical for assessing the severity of anaphylactic reactions (53, 54). This model therefore not only provides a more robust platform for assessing allergic responses but also reflects the clinical complexity of anaphylaxis in humans. Hence, in some cases, drug administration was necessary to prevent fatal consequences, as in humans with respiratory anaphylactic shock.
Several studies have demonstrated changes in tissue architecture in lung biopsies from asthmatic individuals, like those observed in our experimental model. These structural changes are a consequence of the inflammatory process caused by pollen hypersensitivity, which has been reported as part of the pathophysiology of asthma, leading to tissue remodeling and an increase in inflammatory cells (mast cells, lymphocytes and eosinophils) (22, 55, 56). As observed in BAL cytology and lung histology, eosinophil infiltration indicated a quick response caused by specific pollen allergens. In addition, increased degranulated eosinophils were observed, characteristic of patients with allergic asthma and in established mouse models of asthma, where chronic bronchopulmonary inflammation with an abundant infiltrate of mast cells and eosinophils, localized even at the tissue epithelium is often found (57). This is closely associated with severe forms of asthma (55, 56, 58).
The study of inflammatory and anti-inflammatory cytokines is of great relevance for understanding immune responses. In the case of IL-4, IL-6, IL-10, and IL-13 levels in serum samples, no significant differences were observed between the control and treated groups. This finding is consistent with previous studies that evaluated the prevalence of the Th1 and Th2 cytokine profiles in serum samples from individuals with stable asthma (59–61). Similarly, other authors have reported that elevated cytokine levels only occur under specific conditions, such as during chronic phases of asthma accompanied by bronchial hyperresponsiveness, prior to receiving pharmacological treatment (46, 55, 59). On the other hand, significant changes in TGF-β and IFN-γ levels were observed with respect to the time of immunization, but not between the treated and control groups. These findings are consistent with previous studies, for example, in childhood populations with asthma and risk factors such as obesity, where it has been reported that modulators of the immune response, such as serum IFN-γ, do not show significant differences compared to non-overweight individuals (62). A similar phenomenon has been described in pregnant women with and without asthma (63).
Metabolomic studies have also evaluated serum TGF-β and IFN-γ levels in patients with chronic obstructive respiratory disease (COPD) and in those with both COPD and asthma, compared to smokers. These cytokines were found to be significantly altered in patients with COPD and asthma, but not in those with asthma or in smokers (64). TGF-β is closely related to structural changes in the respiratory epithelium and fibrotic processes, so its increase depends on disease severity and lung function impairment (65–67). Finally, an increase in serum TGF-β levels has been associated with irreversible processes of pulmonary fibrosis in asthmatics, and IFN-γ has been described to have an immunomodulatory effect. A decrease in this cytokine during acute episodes has been reported in asthmatics. It is likely that IFN-γ binds to cellular receptors during inflammatory processes to exert its anti-inflammatory function (68, 69).
In asthma, the kinetics of the appearance of immunoglobulins IgA, IgG, and IgE play a fundamental role in modulating the immune response. The IgE, central to the allergic response, is rapidly activated upon allergen exposure, with levels peaking after approximately 14 days (70). This elevation triggers mast cell and basophil degranulation, promoting airway inflammation (50). We measured the antigen-specific IgE, IgA, and IgG, providing insights into the sequential immune dynamics. The initial IgG response may serve to moderate inflammation, functioning as a regulatory mechanism to limit antigen entrance and excessive hypersensitivity (71). Moreover, IgA primarily operates at the mucosal level, neutralizing inhaled allergens and pathogens, thus providing a protective barrier, as observed during pollen instillations in the porcine tonsil (72). Our results indicate that, although serum IgG and IgA responses increased initially to prevent sensitization potentially, these responses plateau after approximately five to seven weeks, coinciding with the late appearance of allergen-specific IgE, albeit at low serum concentrations (Figure 8A). Given IgE's high cytotropic nature, it may bind predominantly to mast cell membranes, with concentrations rising over time increasing the chances of a hypersensitivity reaction. Further long-term studies are needed to understand these immunoglobulin kinetics and their relationship to cytokine dynamics, particularly in chronic models of respiratory hypersensitivity. Therefore, our model may offer valuable insights into the onset, progression, and regulation of the humoral immune response in respiratory hypersensitivity mediated by IgE, giving clues for future treatments.
The porcine model has multiple advantages over other animal models, among them: its high genome and protein sequence homologies with humans, its close resemblance of human immune parameters (>80% vs. <10% for mice), many tools are available (cell lines, antibodies, ELISA and microarrays), cloning and transgenic technology advances, its docility, relatively short gestational and developmental periods, numerous offspring, monogastric and omnivorous nature, size and weight close to the human allowing translational results for treatments, and cheaper and ethically more acceptable use than primates (15–17). These advantages might outweigh the cost of animals, feed and housing in pre-clinical studies for pathologies as important as asthma. In our hands, we could recognize clinical asthma signs like those in humans, and the sample size was large enough for more comprehensive studies (BAL, histology, mucosal fluids).
5 Conclusions
Our immunization protocol in the swine model induced a respiratory hypersensitivity type I-like reaction against Phleum pratense antigens mediated by antigen-specific IgE. The model also showed clinical and histopathological similarities in skin and lungs, and the production of antibodies, like human physiopathology in the face of the allergenic challenge with a complex allergen such as Phleum pratense pollen. It would be necessary to establish a chronic state of the disease using a longer protocol in the swine model, to study the molecular mechanisms involved in the beginning, development, and establishment of the condition resembling human asthma. This model will help in pre-clinical studies to develop early diagnostic tools and therapeutic and prophylactic measures to treat this pathology.
Data availability statement
The raw data supporting the conclusions of this article will be made available by the authors, without undue reservation.
Ethics statement
The animal study was approved by Institutional Committee for the Care and Use of Laboratory Animals (CICUAL) of Cinvestav. The study was conducted in accordance with the local legislation and institutional requirements.
Author contributions
JL-A: Formal analysis, Investigation, Methodology, Writing – original draft, Writing – review & editing. GS-G: Formal analysis, Investigation, Methodology, Writing – review & editing. AS-M: Formal analysis, Investigation, Methodology, Writing – review & editing. CR-E: Formal analysis, Investigation, Methodology, Writing – review & editing. MG-E: Conceptualization, Funding acquisition, Resources, Writing – review & editing. JR-G: Visualization, Writing – review & editing. CR-L: Conceptualization, Formal analysis, Funding acquisition, Investigation, Methodology, Project administration, Resources, Supervision, Writing – original draft, Writing – review & editing. MV-L: Conceptualization, Formal analysis, Funding acquisition, Investigation, Methodology, Project administration, Resources, Supervision, Writing – original draft, Writing – review & editing.
Funding
The author(s) declare that financial support was received for the research and/or publication of this article. This work was partially funded by César A. Reyes-López, PhD, and Marco A. Vega-López, PhD.
Acknowledgments
The authors acknowledge the support of CONAHCYT for Postgraduate Fellowship No. 746721, 635757, and 862848. We thank the Instituto Politécnico Nacional (grant SIP-20161388, SIP-20170907, and SIP-2018068). We thank DVM Gustavo Franco, DVM Octavio Ramos and DVM Diana Peláez for technical assistance with the animals.
Conflict of interest
The authors declare that the research was conducted in the absence of any commercial or financial relationships that could be construed as a potential conflict of interest.
Generative AI statement
The author(s) declare that no Generative AI was used in the creation of this manuscript.
Publisher's note
All claims expressed in this article are solely those of the authors and do not necessarily represent those of their affiliated organizations, or those of the publisher, the editors and the reviewers. Any product that may be evaluated in this article, or claim that may be made by its manufacturer, is not guaranteed or endorsed by the publisher.
References
1. Global Initiative for Asthma — GINA. 2022 GINA Main Report — Global Initiative for Asthma — GINA (2023, 8 March). Global Initiative for Asthma — GINA. Available at: https://ginasthma.org/gina-reports/ (Accessed March 08, 2023).
2. Holgate ST, Wenzel S, Postma DS, Weiss ST, Renz H, Sly PD. Asthma. Nat Rev Dis Primers. (2015) 1:15025. doi: 10.1038/nrdp.2015.25
3. World Health Organization. “Asthma.” WHO. Last modified May 16, (2023). Available at: https://www.who.int/news-room/fact-sheets/detail/asthma (Accessed May 16, 2023).
4. Estela B, Río-Navarro D, María Hidalgo-Castro E, José J, Sienra-Monge L. Asthma. Bol Med Hosp Infant Mex. (2009) 66(1):3–33.
5. Walker C, Bode E, Boer L, Hansel TT, Blaser K, Virchow JC Jr. Allergic and nonallergic asthmatics have distinct patterns of T-cell activation and cytokine production in peripheral blood and bronchoalveolar lavage. Am Rev Respir Dis. (1992) 146(1):109–15. doi: 10.1164/ajrccm/146.1.109
6. Jeffery P. Inflammation and remodeling in the adult and child with asthma. Pediatr Pulmonol Suppl. (2001) 21:3–16. doi: 10.1002/ppul.2001
7. Radauer C, Bublin M, Wagner S, Mari A, Breiteneder H. Allergens are distributed into few protein families and possess a restricted number of biochemical functions. J Allergy Clin Immunol. (2008) 121(4):847–52. doi: 10.1016/j.jaci.2008.01.025
8. Motta A, Peltre G, Dormans JAMA, Withagen CET, Lacroix G, Bois F, et al. Phleum pratense pollen starch granules induce humoral and cell-mediated immune responses in a rat model of allergy. Clin Exp Allergy. (2004) 34(2):310–4. doi: 10.1111/j.1365-2222.2004.01872.x
9. Papapostolou N. Allergic asthma in the era of personalized medicine. J Pers Med. (2022) 12(7):1162. doi: 10.3390/jpm12071162
10. Kirschvink N, Reinhold P. Use of alternative animals as asthma models. Curr Drug Targets. (2008) 9(6):470–84. doi: 10.2174/138945008784533525
11. Shin YS, Takeda K, Gelfand EW. Understanding asthma using animal models. Allergy Asthma Immunol Res. (2009) 1(1):10–8. doi: 10.4168/aair.2009.1.1.10
12. Blume C. In vitro and ex vivo models of human asthma. Eur J Pharm Biopharm. (2013) 84(2):394–400. doi: 10.1016/j.ejpb.2012.12.014
13. Mullane K, Williams M. Animal models of asthma: reprise or reboot? Biochem Pharmacol. (2014) 87(1):131–9. doi: 10.1016/j.bcp.2013.06.026
14. Ibrahim Z, Busch J, Awwad M, Wagner R, Wells K, Cooper DKC. Selected physiologic compatibilities and incompatibilities between human and porcine organ systems. Xenotransplantation. (2006) 13(6):488–99. doi: 10.1111/j.1399-3089.2006.00346.x
15. Hart EA, Caccamo M, Harrow JL, Humphray SJ, Gilbert JG, Trevanion S, et al. Lessons learned from the initial sequencing of the pig genome: comparative analysis of an 8 Mb region of pig chromosome 17. Genome Biol. (2007) 8(8):R168. doi: 10.1186/gb-2007-8-8-r168
16. Meurens F, Summerfield A, Nauwynck H, Saif L, Gerdts V. The pig: a model for human infectious diseases. Trends Microbiol. (2012) 20(1):50–7. doi: 10.1016/j.tim.2011.11.002
17. Swindle MM, Makin A, Herron AJ, Clubb FJ Jr, Frazier KS. Swine as models in biomedical research and toxicology testing. Vet Pathol. (2012) 49(2):344–56. doi: 10.1177/0300985811402846
18. Judge EP, Hughes JL, Egan JJ, Maguire M, Molloy EL, O’Dea S. Anatomy and bronchoscopy of the porcine lung. A model for translational respiratory medicine. Am J Respir Cell Mol Biol. (2014) 51(3):334–43. doi: 10.1165/rcmb.2013-0453TR
19. Bertho N, Meurens F. The pig as a medical model for acquired respiratory diseases and dysfunctions: an immunological perspective. Mol Immunol. (2021) 135:254–67. doi: 10.1016/j.molimm.2021.03.014
20. Woodrow JS, Sheats MK, Cooper B, Bayless R. Asthma: the use of animal models and their translational utility. Cells. (2023) 12:1091. doi: 10.3390/cells12071091
21. Mitchell HW, Turner DJ, Gray PR, McFawn PK. Compliance and stability of the bronchial wall in a model of allergen-induced lung inflammation. J Appl Physiol. (1999) 86(3):932–7. doi: 10.1152/jappl.1999.86.3.932
22. Fraga-Iriso R, Núñez-Naveira L, Brienza NS, Centeno-Cortés A, López-Peláez E, Verea H, et al. Desarrollo de un modelo murino de inflamación y remodelación de vías respiratorias en asma experimental. Arch Bronconeumología. (2009) 45(9):422–8. doi: 10.1016/j.arbres.2009.01.014
23. Guzman-Bautista ER, Garcia-Ruiz CE, Gama-Espinosa AL, Ramirez-Estudillo C, Rojas-Gomez OI, Vega-Lopez MA. Effect of age and maternal antibodies on the systemic and mucosal immune response after neonatal immunization in a porcine model. Immunology. (2014) 141(4):609–16. doi: 10.1111/imm.12222
24. Salinas-Zacarias I, Guzman-Bautista E, Ramírez-Estudillo MC, Chacón-Salinas R, Vega-López MA. Mucosal and systemic immune responses to Aujeszky’s disease virus (ADV) in early vaccinated piglets. Comp Immunol Microbiol Infect Dis. (2020) 68:101400. doi: 10.1016/j.cimid.2019.101400
25. Fragoso-Saavedra M, Ramírez-Estudillo C, Pelaez-Gonzalez D, Ramos-Flores G, Torres-Franco G, Nuñez-Muñoz L, et al. Combined subcutaneous-intranasal immunization with epitope-based antigens elicits binding and neutralizing antibody responses in serum and mucosae against PRRSV-2 and SARS-CoV-2. Front Immunol. (2022) 13:848054. doi: 10.3389/fimmu.2022.848054
26. Raszyk J. A practical immunologic skin test for piglets. Vet Med (Praha). (1992) 37(5-6):269–79.1413389
27. Nischal U, Nischal KC, Khopkar U. Techniques of skin biopsy and practical considerations. J Cutan Aesthet Surg. (2008) 1(2):107–11. doi: 10.4103/0974-2077.44174
28. Nienhoff H. Obtención de Muestras de Lavado Pulmonar en Granja (2008). Asís Veterinaria. ISBN: 978-84-612-3074-7.
29. Roe JM, Patel D, Morgan KL. Isolation of porcine IgE, and preparation of polyclonal antisera. Vet Immunol Immunopathol. (1993) 37(2):83–97. doi: 10.1016/0165-2427(93)90057-b
30. Rupa P, Hamilton K, Cirinna M, Wilkie BN. A neonatal swine model of allergy induced by the major food allergen chicken ovomucoid (Gal d 1). Int Arch Allergy Appl Immunol. (2008) 146(1):11–8. doi: 10.1159/000112498
31. Madden J, Howarth PP, Godfrey RR, Frew AA. The kinetics and stimulant dependence of cytokine production by blood and bronchoalveolar lavage T cells evaluated at the single cell level. Cytokine. (1999) 11(7):510–7. doi: 10.1006/cyto.1998.0456
32. Marsella R, Olivry T, Maeda S. Cellular and cytokine kinetics after epicutaneous allergen challenge (atopy patch testing) with house dust mites in high-IgE beagles. Vet Dermatol. (2006) 17(2):111–20. doi: 10.1111/j.1365-3164.2006.00508.x
33. Liravi B, Piedrafita D, Nguyen G, Bischof RJ. Dynamics of IL-4 and IL-13 expression in the airways of sheep following allergen challenge. BMC Pulm Med. (2015) 15:101. doi: 10.1186/s12890-015-0097-9
34. Balam-May AJ, Ramírez-Estudillo C, Lazo-Vázquez G, Vega-López MA. Postnatal development of lung T lymphocytes in a porcine model. Lung. (2014) 192(5):793–802. doi: 10.1007/s00408-014-9622-5
35. Gandjeva A, Tuder RM. Lung histological methods. Lung Innate Immun Inflammation. (2018) 1809:315–29. doi: 10.1007/978-1-4939-8570-8_20
36. Garvey JS, Cremer NE, Sussdorf DU. Isolation of immunoglobulins, antibodies, and their subunits. In: Shaffer VF, editor. Methods in Immunology (3rd. Ed). IV. New York, NY: W.A. Benjamin, Inc (1977). p. 218–9.
37. Wingfield P. Protein precipitation using ammonium sulfate. Curr Protoc Protein Sci. (1998) 13(1):A-3F. doi: 10.1002/0471140864.psa03fs13
38. Kavran JM, Leahy DJ. Coupling antibody to cyanogen bromide-activated sepharose. Methods Enzymol. (2014) 541:27–34. doi: 10.1016/B978-0-12-420119-4.00003-3
39. Fuller SA, Takahashi M, Hurrell JG. Immunization of mice. Curr Protoc Molecular Biol. (1992) 18(1):11–4. doi: 10.1002/0471142727.mb1104s18
40. Greenfield EA. Standard immunization of mice, rats, and hamsters. Cold Spring Harb Protoc. (2020) 2020(3):82–4. doi: 10.1101/pdb.prot100297
41. de Benedictis D. Asthma in adolescence: is there any news? Pediatr Pulmonol. (2017) 52(1):129–38. doi: 10.1002/ppul.23498
42. Vega-López MA, Ramírez-Estudillo MC. Mexican Patent No. 355566 “Métodos de Estandarización de la Técnica de ELISA Para Cuantificar Inmunoglobulinas Específicas de Origen Porcino (IgG e IgA)” (2018) [Quantitative ELISA Standarization to Evaluate Specific Porcine IgG and IgA in Biological Samples], Otorgada en Abril 19 de 2018 por el Instituto Mexicano de la Propiedad Industrial, Expediente MX/s/2012/008563. Expiration date: Jul/23/2032.
43. Schook LB, Collares TV, Darfour-Oduro KA, De AK, Rund LA, Schachtschneider KM, et al. Unraveling the swine genome: implications for human health. Annu Rev Anim Biosci. (2015) 3:219–44. doi: 10.1146/annurev-animal-022114-110815
44. Helm RM, Furuta GT, Stanley JS, Ye J, Cockrell G, Connaughton C, et al. A neonatal swine model for peanut allergy. J Allergy Clin Immunol. (2002) 109(1):136–42. doi: 10.1067/mai.2002.120551
45. Rupa P, Schmied J, Wilkie BN. Porcine allergy and IgE. Vet Immunol Immunopathol. (2009) 132(1):41–5. doi: 10.1016/j.vetimm.2009.09.013
46. Stone SF, Cotterell C, Isbister GK, Holdgate A, Brown SGA. Elevated serum cytokines during human anaphylaxis: identification of potential mediators of acute allergic reactions. J Allergy Clin Immunol. (2009) 124(4):786–92. doi: 10.1016/j.jaci.2009.07.055
47. Ring J, Beyer K, Biedermann T, Bircher A, Duda D, Fischer J, et al. Guideline for acute therapy and management of anaphylaxis. Allergo J Int. (2014) 23(3):96–112. doi: 10.1007/s40629-014-0009-1
48. Poulsen OM, Hau J. Murine passive cutaneous anaphylaxis test (PCA) for the “all or none” determination of allergenicity of bovine whey proteins and peptides. In: Beynen AC, Solleveld HA, editors. New Developments in Biosciences: Their Implications for Laboratory Animal Science. Dordrecht: Springer (1988). p. 245–52. doi: 10.1007/978-94-009-3281-4_17.
49. Hamelmann E, Tadeda K, Oshiba A, Gelfand EW. Role of IgE in the development of allergic airway inflammation and airway hyperresponsiveness—a murine model. Allergy. (1999) 54(4):297–305. doi: 10.1034/j.1398-9995.1999.00085.x
50. Stone KD, Prussin C, Metcalfe DD. IgE, mast cells, basophils, and eosinophils. J Allergy Clin Immunol. (2010) 125(2):S73–80. doi: 10.1016/j.jaci.2009.11.017
51. Nauta A, Knippels L, Garssen J, Redegeld F. Animal models of anaphylaxis. Curr Opin Allergy Clin Immunol. (2007) 7(4):355–9. doi: 10.1097/ACI.0b013e32825ea543
52. Gouel-Chéron A, Dejoux A, Lamanna E, Bruhns P. Animal models of IgE anaphylaxis. Biology (Basel). (2023) 12(7):931. doi: 10.3390/biology12070931
53. Szebeni J, Bawa R. Human clinical relevance of the porcine model of pseudoallergic infusion reactions. Biomedicines. (2020) 8(4):82. doi: 10.3390/biomedicines8040082
54. Reber LL, Hernandez JD, Galli SJ. The pathophysiology of anaphylaxis. J Allergy Clin Immunol. (2017) 140(2):335–48. doi: 10.1016/j.jaci.2017.06.003
55. Saetta M, Turato G. Airway pathology in asthma. European respiratory journal. Supplement. (2001) 18(34):18s–23. doi: 10.1183/09031936.01.00229501
56. Brightling CE, Gupta S, Gonem S, Siddiqui S. Lung damage and airway remodelling in severe asthma. Clin Exp Allergy. (2012) 42(5):638–49. doi: 10.1111/j.1365-2222.2011.03917.x
57. Wardlaw AJ, Dunnette S, Gleich GJ, Collins JV, Kay AB. Eosinophils and mast cells in bronchoalveolar lavage in subjects with mild asthma. Relationship to bronchial hyperreactivity. Am Rev Respir Dis. (1988) 137(1):62–9. doi: 10.1164/ajrccm/137.1.62
58. Bousquet J, Jeffery PK, Busse WW, Johnson M, Vignola AM. Asthma. From bronchoconstriction to airways inflammation and remodeling. Am J Respir Crit Care Med. (2000) 161(5):1720–45. doi: 10.1164/ajrccm.161.5.9903102
59. Cho SH, Stanciu LA, Holgate ST, Johnston SL. Increased interleukin-4, interleukin-5, and interferon-γ in airway CD4+ and CD8+ T cells in atopic asthma. Am J Respir Crit Care Med. (2005) 171(3):224–30. doi: 10.1164/rccm.200310-1416OC
60. Ceyhan BB, Enc FY, Sahin S. IL-2 and IL-10 levels in induced sputum and serum samples of asthmatics. J Investig Allergol Clin Immunol. (2004) 14(1):80–5.15160446
61. Rogala B, Bozek A, Gluck J, Jarzab J. Prevalence of IgE-mediated allergy and evaluation of Th1/Th2 cytokine profiles in patients with severe bronchial asthma. Postepy Dermatol Alergol. (2015) 32(4):274–80. doi: 10.5114/pdia.2015.53323
62. Shailesh H, Noor S, Hayati L, Belavendra A, Van Panhuys N, Abou-Samra AB, et al. Asthma and obesity increase inflammatory markers in children. Front Allergy. (2025) 5:1536168. doi: 10.3389/falgy.2024.1536168
63. Osei-Kumah A, Smith R, Clifton VL. Maternal and cord plasma cytokine and chemokine profile in pregnancies complicated by asthma. Cytokine. (2008) 43(2):187–93. doi: 10.1016/j.cyto.2008.05.008
64. Ghosh N, Choudhury P, Kaushik SR, Arya R, Nanda R, Bhattacharyya P, et al. Metabolomic fingerprinting and systemic inflammatory profiling of asthma COPD overlap (ACO). Respir Res. (2020) 21(1):126. doi: 10.1186/s12931-020-01390-4
65. Litonjua AA, Sparrow D, Guevarra L, O'Connor GT, Weiss ST, Tollerud DJ. Serum interferon-gamma is associated with longitudinal decline in lung function among asthmatic patients: the normative aging study. Ann Allergy Asthma Immunol. (2003) 90(4):422–8. doi: 10.1016/S1081-1206(10)61827-3
66. Cao Y, Gong W, Zhang H, Liu B, Li B, Wu X, et al. A comparison of serum and sputum inflammatory mediator profiles in patients with asthma and COPD. J Int Med Res. (2012) 40(6):2231–42. doi: 10.1177/030006051204000621
67. Rybka J, Korte SM, Czajkowska-Malinowska M, Wiese M, Kędziora-Kornatowska K, Kędziora J. The links between chronic obstructive pulmonary disease and comorbid depressive symptoms: role of IL-2 and IFN-γ. Clin Exp Med. (2016) 16(4):493–502. doi: 10.1007/s10238-015-0391-0
68. Makinde T, Murphy RF, Agrawal DK. The regulatory role of TGF-β in airway remodeling in asthma. Immunol Cell Biol. (2007) 85(5):348–56. doi: 10.1038/sj.icb.7100044
69. Al-Alawi M, Hassan T, Chotirmall SH. Transforming growth factor β and severe asthma: a perfect storm. Respir Med. (2014) 108(10):1409–23. doi: 10.1016/j.rmed.2014.08.008
70. Gould HJ, Sutton BJ. Ige in allergy and asthma today. Nat Rev Immunol. (2008) 8(3):205–17. doi: 10.1038/nri2273
71. Williams JW, Tjota MY, Sperling AI. The contribution of allergen-specific IgG to the development of Th2-mediated airway inflammation. J Allergy (Cairo). (2012) 2012:1–9. doi: 10.1155/2012/236075
Keywords: asthma, allergy, minipig, pollen, hypersensitivity
Citation: Ledesma-Aparicio J, Salazar-Guerrero G, Soto-Muñoz A, Ramírez-Estudillo C, Gómez-Esquivel ML, Reyes-Grajeda JP, Reyes-López CA and Vega-López MA (2025) Evaluation of a translational swine model of respiratory hypersensitivity induced by exposure to Phleum pratense pollen allergens. Front. Allergy 6:1557656. doi: 10.3389/falgy.2025.1557656
Received: 8 January 2025; Accepted: 24 March 2025;
Published: 7 April 2025.
Edited by:
Saptarshi Roy, Temple University, United StatesReviewed by:
Josefina Zakzuk, University of Cartagena, ColombiaYaqi Yang, Tongji Medical College of Huazhong University of Science and Technology, China
Lei Fang, Yangzhou University, China
Copyright: © 2025 Ledesma-Aparicio, Salazar-Guerrero, Soto-Muñoz, Ramírez-Estudillo, Gómez-Esquivel, Reyes-Grajeda, Reyes-López and Vega-López. This is an open-access article distributed under the terms of the Creative Commons Attribution License (CC BY). The use, distribution or reproduction in other forums is permitted, provided the original author(s) and the copyright owner(s) are credited and that the original publication in this journal is cited, in accordance with accepted academic practice. No use, distribution or reproduction is permitted which does not comply with these terms.
*Correspondence: César A. Reyes-López, Y2FyZXllc0BpcG4ubXg=; Marco A. Vega-López, bWF2ZWdhQGNpbnZlc3Rhdi5teA==