- 1Department of Grassland and Livestock, Norwegian Institute of Bioeconomy Research (NIBIO), Aas, Norway
- 2Teagasc Food Research Centre, Ashtown, Dublin, Ireland
- 3Animal and Bioscience Research Department, Teagasc Grange, Meath, Ireland
- 4School of Biological and Chemical Sciences, Ryan Institute, University of Galway, Galway, Ireland
- 5Department of Biotechnology and Nanomedicine, SINTEF Industry, Trondheim, Norway
Introduction: The discovery of the methane-mitigating effect of the red seaweed Asparagopsis taxiformis has triggered a search for other seaweed species with similar effects. Brown seaweeds constitute the largest production volume of seaweeds in Europe. Some brown algae are known to inhibit methanogens and could potentially reduce enteric methane emissions. Use of by-products generated from industrial processing of plants are typically inedible for human consumption but well known as ruminant feeds. As fractions from Laminaria hyperborea showed significant reductions in methane emissions in vitro, a L. hyperborea by-product was chosen for an in vivo trial with sheep. The aim was to investigate the effect of L. hyperborea by-product inclusion in the diet of growing lambs on dry matter intake, methane emissions, growth rate and nitrogen digestibility.
Methods: Twenty-four Norwegian White Sheep lambs (12 ewe and 12 male lambs, 4 months; 36.8 kg live weight) were fed a Control diet (grass silage and control concentrate) or an Algae diet (grass silage and algae concentrate 2% inclusion rate). Lambs were fed a basic diet (grass silage and neutral concentrate) and, in staggered order, introduced to their respective diets for five weeks before entering one of six open circuit respiration chambers. Methane production was measured for three consecutive days. All lambs entered the chambers three time (Periods 1, 2 and 3). Feed intake was measured four consecutive days a week, and live weight (kg) was measured every two weeks. Twelve male lambs were used to investigate in vivo nitrogen digestibility using metabolism crates.
Results: The inclusion rate of L. hyperborea by-product was above the target and ended at 2.5% of DM. There was an increase in feed intake and live weight over the experimental period, consistent with the growth of the lambs. Methane production, yield, or intensity was not affected by diet, overall, but the Algae diet reduced methane in Period 1. Male lambs produced more methane than female lambs. Algae inclusion affected live weight negatively.
Discusssion: It is concluded that use of L. hyperborea by-products as a feed additive to sheep needs further investigation due to inconclusive results in the present study.
1 Introduction
Livestock supply chains account for approximately 14.5% of global anthropogenic greenhouse gas (GHG) emissions (FAO, 2023), of which Approximately two-thirds results from rumen fermentation during digestion of feed by ruminants. As a result, strategies to reduce enteric methane (CH4) emissions from ruminants is of high priority. Participants in the Global Methane Pledge have commitments to take voluntary actions to reduce global CH4 emissions by 30% from 2020 levels by 2030. The discovery of the methane-mitigating effect of the red seaweed Asparagopsis taxiformis (9 to 98% reduction; Wasson et al., 2022) has initiated a search for other seaweed species with similar effects. The CH4 reducing compound in this alga is bromoform, which inhibits methanogens without affecting other bacteria. However, bromoform is classified by the U.S Environmental Protection Agency (EPA) as a probable human carcinogen and is reported to be transferred to milk when fed to dairy cows (Muizelaar et al., 2021). Brown seaweeds, known as the Phaeophyceae, constitute the largest production volume of seaweeds in Europe. These species do not contain significant levels of bromoform, but other bioactive components including carbohydrates, lipids, peptides, iodine, and polyphenols known as phlorotannins. Some brown algae are known to inhibit methanogens (Archaea) and therefore could potentially reduce enteric CH4 emissions (Min et al., 2021). Exploration and characterization of any mitigating effects of these species are therefore of high interest.
To date, different species have been screened in vitro, using rumen fluid from sheep or cattle, as a cost-efficient and ethical first step to select a seaweed species for use in animal trials. Abbot et al. (2020) identified 21 seaweeds as candidates capable of mitigating CH4 in vitro, while other species had no mitigating effect. Wang et al. (2008) observed a 15% reduction in CH4 when Ascophyllum nodosum was included in an in vitro diet at 11%, but Roskam et al. (2022) observed no reduction in CH4 emission adding 10g/kg DM. Including 2% of daily DM intake of A. nodosum extract in diet to sheep, Roskam et al. (2024) found inconsistent results on CH4 emissions which was suggested to be due to differences in the content of phlorotannins in the diets.
Use of by-products generated from industrial processing of plants are well known as ruminant feeds, such as the protein-rich residues after oil extraction from oil seeds, grain residues from breweries and distilleries, and byproducts from sugar production (Salami et al., 2019). Typically, these by-products are not fit for human consumptions but used as feed by-products they can increase sustainable production of meat and milk. Some by-products contain bioactive compounds of interest for livestock. The seaweed industry extract hydrocolloids such as alginate, as well as high-value products for use as techno-functional food, pharmaceutical and cosmetic ingredients. Following hydrocolloid extraction process, a biomass residue or by-product remains, which usually has no economic value. Seaweed by-products have only, to a limited degree, been explored as feed additives in ruminant diets.
The by-product resulting from alginate extraction from Laminaria hyperborea contains residual alginate, cellulose, insoluble protein, and membrane lipids. Most water-soluble compounds, such as the carbohydrates used for storage of energy, are removed during the extensive washing steps, thus the composition of the by-product is less influenced by seasonal variations than the unprocessed biomass. However, minerals and other low-molecular weight compounds that are bound to, or embedded in, the insoluble parts of the seaweed will remain, including some polyphenols. The concentrations of iodine present in seaweed by-product resulting from alginate extractions were found to be in the same range and even higher, than concentration found in fresh biomass (Lind et al., 2023). However, Lind et al. (2023) investigated the iodine intake and excretion from sheep supplemented with the L. hyperborea by-product and found that most of the iodine was excreted in feces without compromising animal health.
In vitro analyses oxf CH4 and total gas production of various brown algae, including the commercial species A. nodosum, L. hyperborea, Saccharina latissima, and Alaria esculenta, performed at the Swedish Agricultural University, Umeå (data not published) showed significant reductions in CH4 for A. nodosum and one L. hyperborea stipe sample, however, with a tendency towards lower emissions for all L. hyperborea samples. Since the L. hyperborea byproduct used in this study is generated mainly from the stipes and has no current application or value, demonstration of a CH4 mitigating effect of this would be of scientific interest. The aim of this study was to investigate the effect of L. hyperborea by-product inclusion in the diet of growing lambs on dry matter intake (DMI), CH4 emissions, growth rate, and nitrogen digestibility. The hypotheses were 1) L. hyperborea inclusion in the diet of sheep reduces CH4 emissions; 2) L. hyperborea inclusion does not affect DMI or growth rate.
2 Material and methods
2.1 Concentrate feed
Laminaria hyperborea by-product from industrial alginate production were provided by IFF N&H Norway AS (Haugesund, Norway) as described in Lind et al. (2023). Briefly, the by-products were pressed and then dried from 3% solid dry weight to 93% dry weight. The dried biomass was ground to 2 mm and incorporated into a concentrate feed manufactured by Felleskjøpet Agri (Rindsem Mølle, Verdal, Norway). Two types of concentrate were produced, a control (Control) and an experimental (Algae). The concentrate composition was designed for this study and was based on commonly used organic concentrate for sheep available for Norwegian farmers. Dried L. hyperborea by-product was included in the concentrate at 6% (w/w) inclusion rate. The ingredient composition of the two concentrates and the chemical composition of the L. hyperborea by-product are presented in Lind et al. (2023) and in the Supplementary Material.
2.2 Diet composition
Lambs were offered first cut grass silage composed of Bromus inermis, Poa pratensis, Festuca pratensis and Trifolium repens. The grass was wilted for 24 hours and subsequently baled in large bales (700 kg raw weight) without the use of an additive. During the experiment all animals received a fixed amount of concentrate with grass silage offered ad libitum. From week 1-2 the animals received 300g of concentrate, week 3-9 they received 400g and from week 10-17 they received 600g.
The inclusion rate of L. hyperborea by-product was limited by the iodine content of the by-product (Lind et al., 2023) and aimed to be 2% of the total diet (fresh matter). The inclusion was thus adjusted by increasing the concentrate level in parallel with the increased level of grass silage.
2.3 Animal trial
2.3.1 Animals, diets, and methane emissions
An in vivo sheep trial was initiated using 24 Norwegian White Sheep lambs (4 months of age). The trial was carried out in accordance with the Norwegian Ministry of Agriculture and Food regulation for use of animals in experiments and approved by the Ethics Commission on Animal Use application number FOTS ID 19314. It complies with the EU Directive 2010/63/EU on the use of experimental animals.
The animals consisted of 12 male lambs (37.8 kg live weight (LW) +/- SD 1.2 kg) and 12 female lambs (35.8 kg LW +/- SD 0.6 kg). The lambs were born in April 2021, milk fed for two months, then weaned and let out on a pasture where they additionally were fed a commercial concentrate. In the middle of August, 4 months of age, the lambs were collected and individually housed in stalls in an uninsulated barn at NIBIO Tjøtta in Nordland County, Norway (65° 49’22 N 12° 25’37 E). They were adapted to the indoor conditions fed grass silage ad libitum, 300 g commercial concentrate and given free access to water. The lambs were weighed and within sex, randomly allocated to either a Control diet (grass silage and Control concentrate, 36.4 kg LW +/- 1 kg) or an Algae diet (grass silage and Algae concentrate, 37.2 kg LW +/- 1 kg). This resulted in six male and six female lambs receiving each of the diets. Animals were fed their daily allowances in two equal portions at 08:30 and 14:30 h. Concentrate was offered separately from the grass silage. Grass silage was weighed for four consecutive days each week (Monday through to Thursday) and refused grass silage was measured before morning feeding (Tuesday through to Friday) to calculate daily feed intake. Samples of grass silage were taken weekly and dried for 48 h at 60°C for dry matter (DM) analysis. Fresh grass silage and concentrate samples were collected weekly and stored at -18 °C for further analysis.
Six open circuit respiration chambers at NIBIO Tjøtta were used during the experiment. Each chamber had a 25 x 25 mm frame of coated steel covered with a steel mesh that protected the exterior polycarbonate sheeting (Hart et al., 2014). The chambers measured 1.8 m wide, 1.8 m deep and 1.5 m high, comprising a total volume of approximately 5 m3. Enteric CH4 was sampled into a seven port Servopro Multiexact 4100 Analyzer (Servomex Group Inc., Woburn, MA, USA) as described in Lind et al. (2020). Methane concentrations in ambient air and in exhaust gas leaving each chamber (in total seven ports) were measured on a rotational basis, taking one gas sample reading after 2.5 min per port, measuring ambient air and each chamber every 17.5 min. The gas Servomex analyzer was calibrated before the animals entered the chambers using a zero gas (pure nitrogen) and a span gas (50 ppm CH4). The average CH4 recovery rate for the chambers was determined before and after the experiment and was 89%.
Animals were kept on the basic diet until five weeks prior to their entry to one of the respiration chambers. In a staggered order over four weeks, lambs (six each week) were introduced and adapted to their diets with three lambs receiving the Control and three lambs receiving the Algae diet each week. The lambs were kept in the chambers for 72 h, during which they were fed and managed in a similar way as in the barn. Grass silage intake was measured daily. Concentrate was offered separately from the grass silage and increased from 300 g/animal per day to 400 g/animal per day during Period 1 (see below) and further increased to 600 g/animal per day during Period 2 and 3. The increase in concentrate was following the nutritional needs for growing lambs and regulated according to grass silage intake to reach the target of 2% inclusion rate of L. hyperborea in the total diet. Water was available in buckets. Chambers were cleaned daily during morning feeding.
All the animals entered the respiration chambers in the same order, three times (Period 1, 2 and 3) to record enteric methane emissions. The total experimental period was 17 weeks including the adaptation period of five weeks and three periods of four weeks. Lambs were weighted every two weeks during the experimental period in an electronic scale (BioControl, WSS3000, Rakkestad, Norway).
2.3.2 Nitrogen digestibility
In vivo nitrogen digestibility was determined using the 12 male lambs assigned to the study as previously described by Lind et al. (2023). Briefly, lambs were placed individually in metabolism crates for three consecutive days during week 5 and week 10 of the present experiment and fed as described above. Samples of grass silage and refusals were collected daily, dried at 48 °C for 48 h and ground to pass 1-mm screen using a Tecator Cyclotec Sample Mill (Foss Analytical Co., Ldt., Suzhou, China). Samples were stored in plastic zipped bags at -18 °C until further analysis.
Buckets were placed under each of the metabolism crates to collect facilitate the separate collection of feces and urine One hundred milliliters (ml) of 10% sulfuric acid were added to each urine bucket to prevent loss of ammonia (Özkan-Gülzari et al., 2019). Acidity of urine was measured using a pH indicator strips non-bleeding pH 0 – 6.0 (Teststrips, pH, MColorpHast™, product number: 1.09531.0001, VWR International, Merck KGaA, Darmstadt, Germany). Feces and urine were collected, and total amount measured from each animal in the morning before feeding. Samples of feces (g) and urine (l), 10% of total volume was collected and stored at −18°C for later analysis. Frozen fecal samples were course ground and freeze-dried for 48 h, using a Labconco FreeZone 4.5 Plus® freeze-drier (Kansas City, Missouri, USA). The samples were then ground to pass through a 1-mm screen using a Tecator Cyclotec Sample Mill® (Foss Analytical Co., Ltd., Suzhou, China) and stored in plastic zipped bags at -18°C until further analyses.
2.4 Analysis
2.4.1 Chemical composition grass silage, refusals, feces and urine
Samples of grass silage were analyzed using NIR by Eurofins Norway performed at Eurofins Agro Testing Wageningen (Binnenhaven 5, NL-6709 PD, Wageningen ISO/IEC 17025:2005 RvA L122) for DM, ash, crude protein (CP) and neutral detergent fiber (NDF) content. Nitrogen in feed, refusals, feces, and thawed urine was measured as Kjeldahl N (AOAC, 2002; method 2001.11, KjeltecTM 8400; Foss Electric, Hillerød, Denmark) by the laboratory at NMBU, Ås, Norway.
2.4.2 Polyphenol content of L. hyperborea by-product
The total polyphenol content (TPC) of the algal concentrate and control (without algae) was determined as described previously by Kupina et al. (2018) and is based on the AOAC recognized method of Singleton and Rossi (1965). Test extracts with algae and without (control) were prepared as follows: 100 mg of the test extract was transferred into a 100 mL volumetric flask. To this, 75 mL of water was added. The solution was sonicated for 10 min until all solids were dissolved. 5.0 mL of this solution was added to a 100 mL volumetric flask and diluted to volume with water. The weight of the test material was adjusted to achieve an absorbance within the calibration curve range. Phenolic content was determined by measuring the absorbance at 765 nm of the test extract in solution and comparing it with a calibration curve using Gallic acid (GA) as a standard. The concentration of Gallic acid for stock solutions was calculated using the equation:
where; M = mass of Gallic acid (g); P = purity of Gallic acid (%); and W = water content of Gallic acid. The concentration of Gallic acid of the stock standard solutions was then used to calculate the actual concentration of each calibration standard solution as follows: Calibration standard solution (mg/L) = s v mL × 25 where S = the concentration of the stock standard solution (mg/L) and V = the volume of stock standard solution (mL). Absorbance at 765 nm vs concentration of the calibration standards was used to determine the mg/L GAE for test samples. Results were determined as statistically different using the two-tailed P value test where the value was less than 0.0001 (P<0.0001) calculated using GraphPad Prism and are reported as mg GAE/100 mg of extract (the amount of extract used in the study).
2.5 Statistical analysis
To determine daily dry matter intake, and enteric methane emissions between diets and periods, data were analyzed using the ANOVA repeated measures mixed model in Minitab for Windows version 19.2. Diet, period, and sex were considered fixed effects and animal a random effect. Individual animal were nested under the respective diets.
Animal live weight and average daily weight gain were analyzed using the ANOVA general linear model in Minitab. Diet and sex were considered fixed and individual animal random effects. Initial start weight was used as covariate when calculating average daily weight gain.
Nitrogen digestibility was analyzed using the ANOVA general linear model in Minitab. Diet was considered fixed and individual animal random effects.
When a significant effect of diet, period or sex was found, post hoc comparison of means was made using the Tukey test. Differences were considered significant at P < 0.05.
3 Results
3.1 Feed composition
The chemical composition (g/kg DM) of grass silage and concentrate offered within each of the three periods and overall trial, during the 17 weeks is presented in Table 1. The two concentrates were formulated to be isonitrogenous and isocaloric.
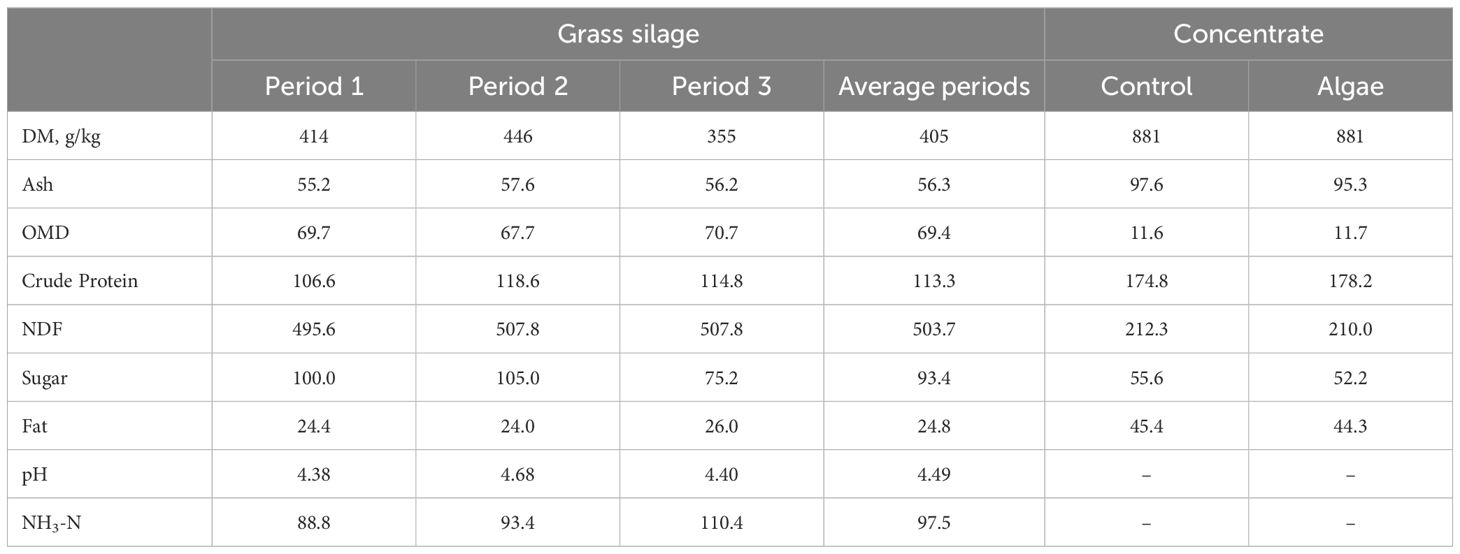
Table 1. Average chemical composition (g/kg DM) of grass silage in Period 1, 2 and 3 and average over the three periods, Control and Algae concentrates.
3.1.1 Total polyphenols
As shown in Figure 1, the content of polyphenols in the algae fed diet (84.7 mg GAE/100 mg extract) was greater than the control (51.3 mg GAE/100 mg extract; P < 0.0001).
3.2 Feed intake
The inclusion of L. hyperborea in the Algae diet on fresh matter, including concentrate and grass silage, was on average 2.5% per animal per day during the 17 weeks of experiment (Table 2). The inclusion was significantly higher in Period 3 compared to Period 1 and Period 2 (P < 0.001) and higher for female lambs (maximum intake was 3.9%) compared to male lambs (maximum intake was 3.3%; P < 0.01), due to increased intake of concentrate relatively to the silage. No refusals of concentrate were observed throughout the experiment from any lambs. The proportion of concentrate consumed relative to grass silage was higher in female lambs (P < 0.001) compared to male lambs (43% versus 35% DM).

Table 2. Average percentage (%) L. hyperborea by-product in the Algae diet consumed by male (M) and female (F) lambs, and all lambs for Period 1, 2 and 3.
Dry matter intake (DMI) of grass silage and total DMI (TDMI) over the three periods, within period and sex is presented in Table 3. The differences in total DMI (TDMI) between diets (total and within Periods) are related to differences in DMI from grass silage, as the DMI from concentrate was the same for all animals within Period. The DMI from concentrate increased from Period 1 (0.44 kg DM/lamb per day) to Period 2 and 3 (0.56 g DM/lamb per day). The proportion of concentrate (DM basis) in the diet was 38.0% in the Control diet and 39.8% in the Algae diet (P = 0.153). the proportion of concentrate in Period 1, 2, and 3 was 38.9%, 36.3% and 41.6% respectively (P < 0.003). Dry matter intake was similar between diets (P = 0.16). However, there were differences in DMI between diets within Periods (P < 0.001) with animals having the highest intake in Period 2, followed by Period 3 and Period 1. The inclusion of concentrate was highest in Period 3 (41% DM), followed by Period 2 (36% DM) and Period 1 (33% DM).
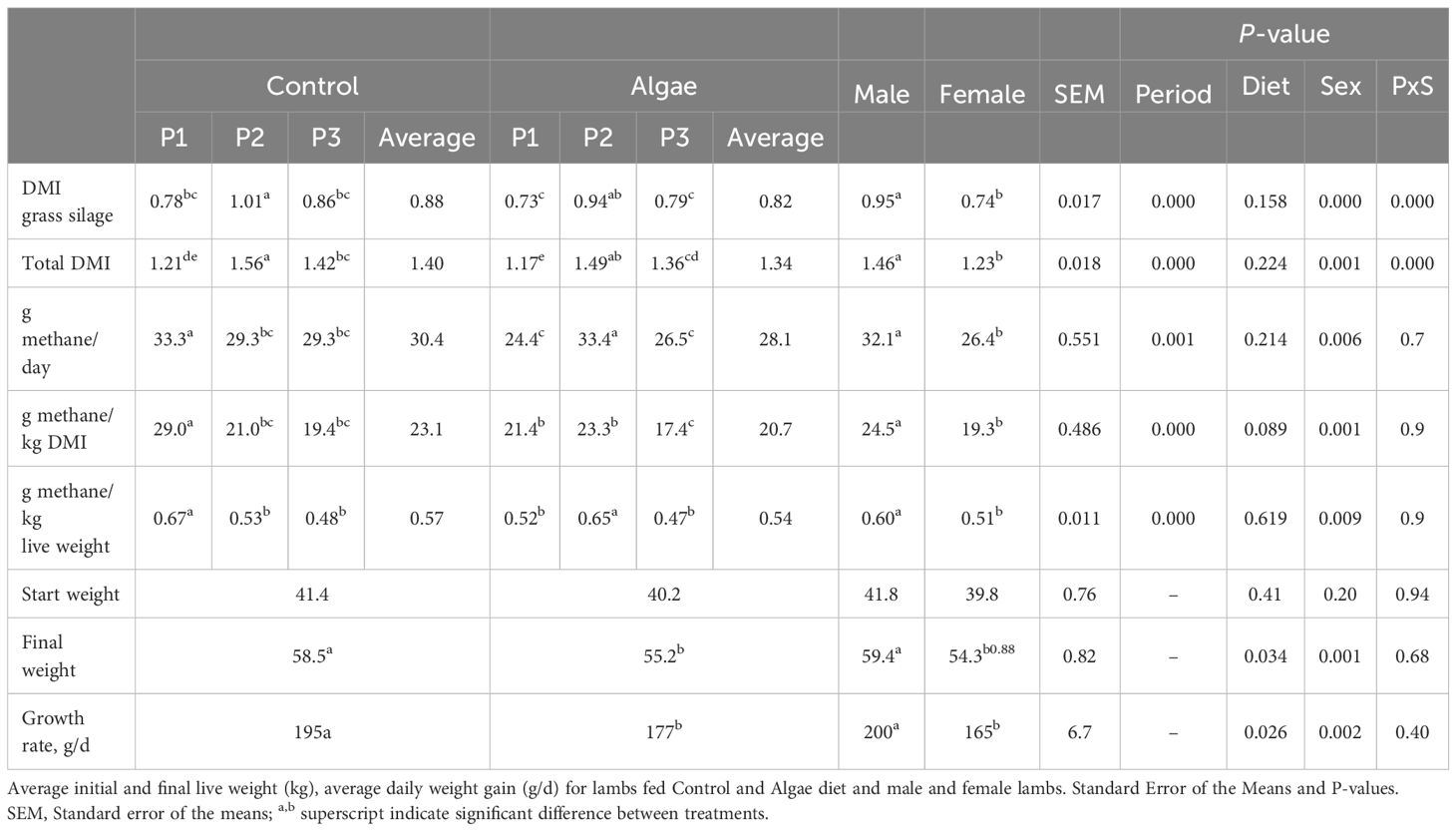
Table 3. Average daily dry matter intake (DMI, kg) of grass silage, total dry matter intake (kg), average CH4 production (g/d), CH4 yield (g/kg DMI) and CH4 intensity (g/kg live weight) for lambs fed Control and Algae diet in Period 1, 2 and 3, and male and female lambs.
Total dry matter intake of grass silage and concentrate by male and female lambs for Period 1, 2, 3, respectively, are shown in Figure 2. The highest intake was by female lambs during Period 2 (1.02 kg DMI/lamb per day) and the lowest by female lambs during Period 1 (0.56 g DMI/lamb per day).
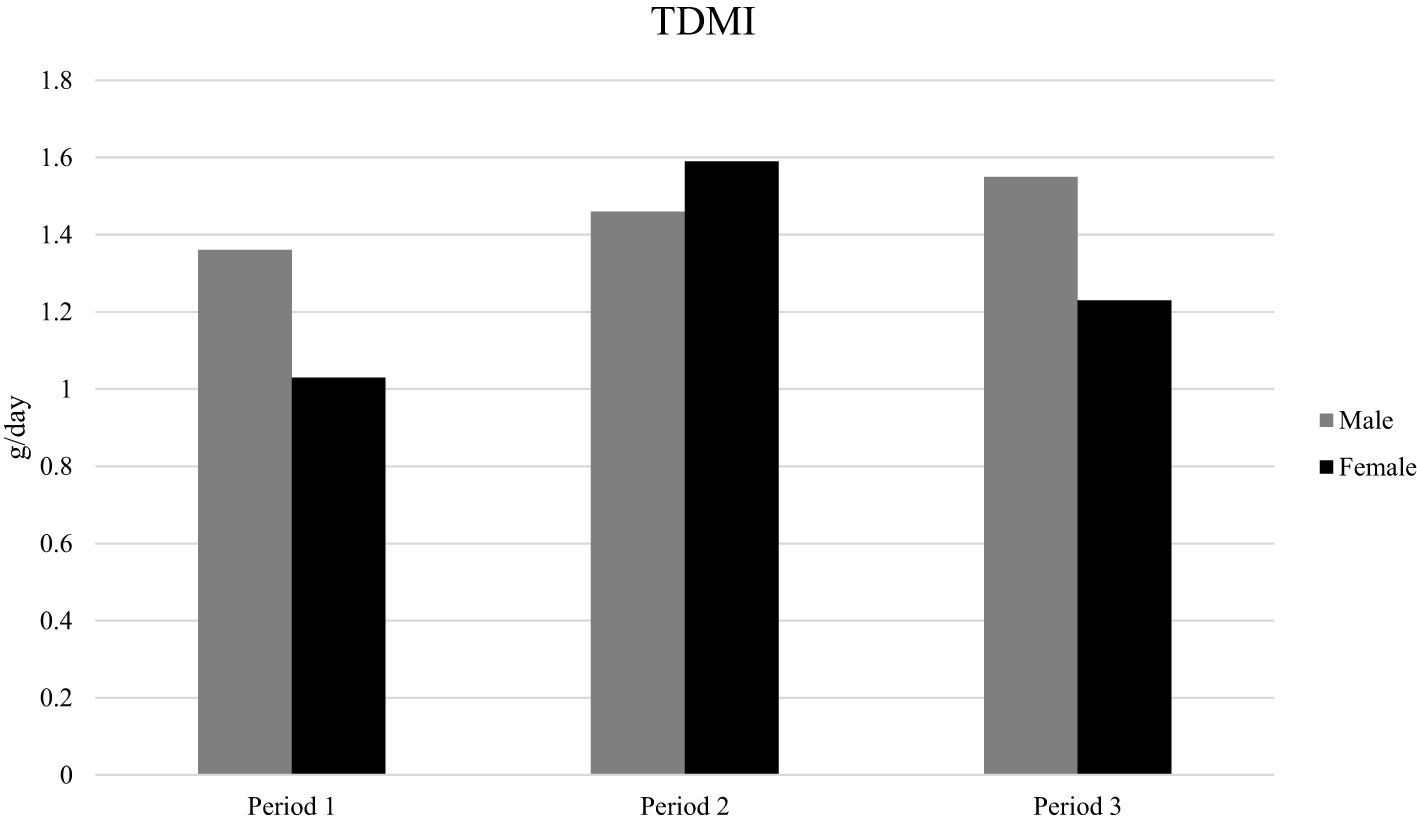
Figure 2. Total dry matter intake of grass silage and concentrate by male and female lambs for Period 1, 2, and 3.
3.3 Methane emission
Table 3 shows the CH4 production (g CH4/animal per day), CH4 yield (g CH4/kg DMI) and intensity (g CH4/kg LW) as an average over the 3 periods and within each period, split on diets and on male and female lambs. There were no differences in CH4 production, yield and intensity overall between diets. However, between periods, there were significant differences, where lambs emitted more CH4 in Period 1 compared to Period 3 (P < 0.001). Methane production was highest for lambs during Period 2 (P < 0.001). Male lambs had greater CH4 production (g CH4/animal; P = 0.006), yield (g CH4/kg DMI; P = 0.001) and intensity (g CH4/kg LW; P = 0.009) compared to female lambs.
3.4 Live weight
Initial and final LW (kg) and average daily growth rate (g/d) for lambs in the Control and Algae treatments and for male and female lambs, are shown in Table 3. There were no differences in start weight between diets (P = 0.41) or sex (P = 0.20) (Table 3, Figure 3). Lambs fed the Control diet had a higher daily growth rate (P = 0.034) and final LW (P = 0.026). Male lambs were heavier (P = 0.001) at the end of the experiment and had higher growth rate (P = 0.002) than female lambs.
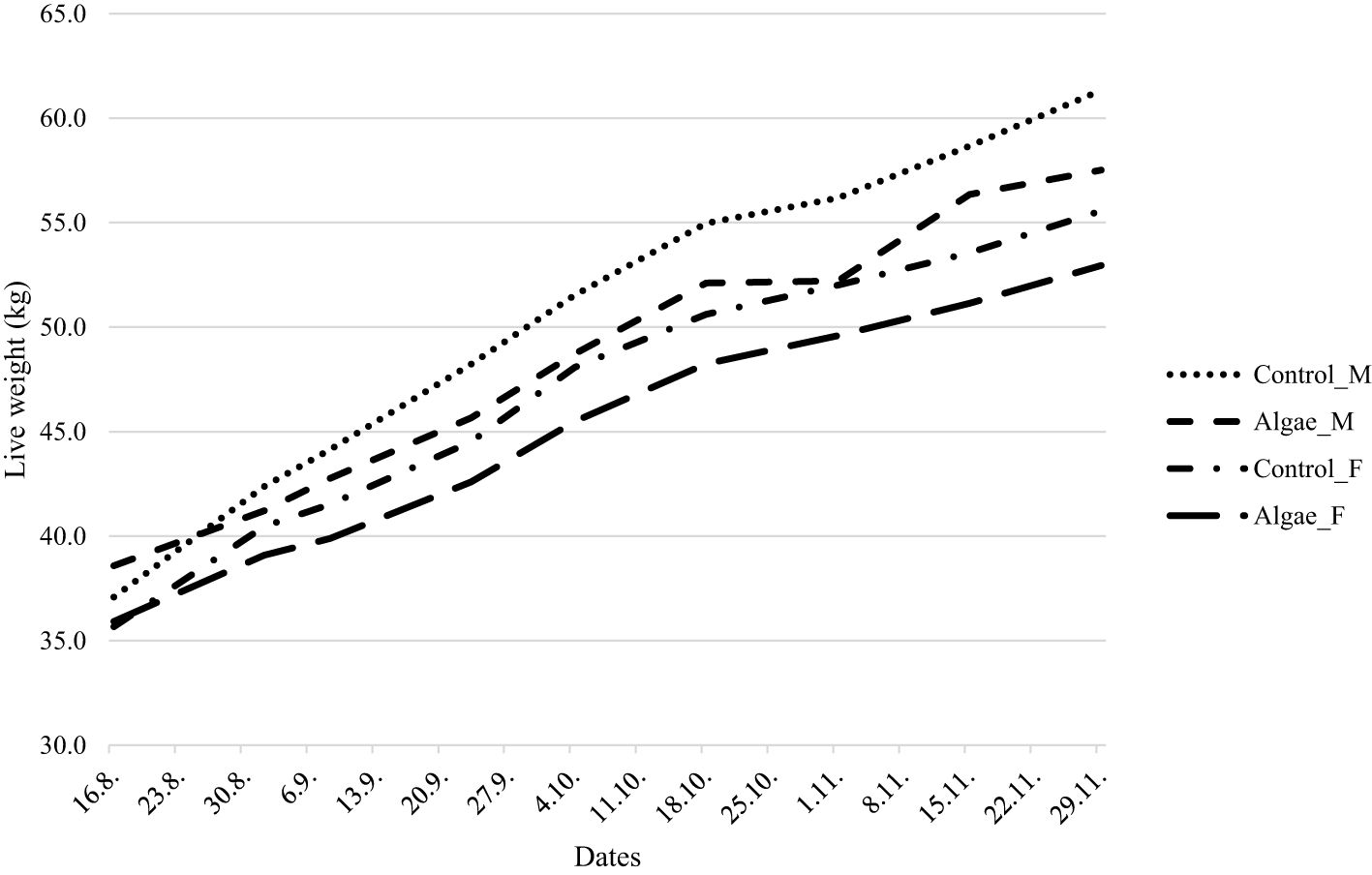
Figure 3. Live weight (kg) during the experimental period for male (M) and female (F) lambs fed Control or Algae diet.
3.5 Nitrogen digestibility
Total N intake (g/day) was similar between diets (P = 0.388) and no differences in N output (feces or urine) were observed (Table 4).
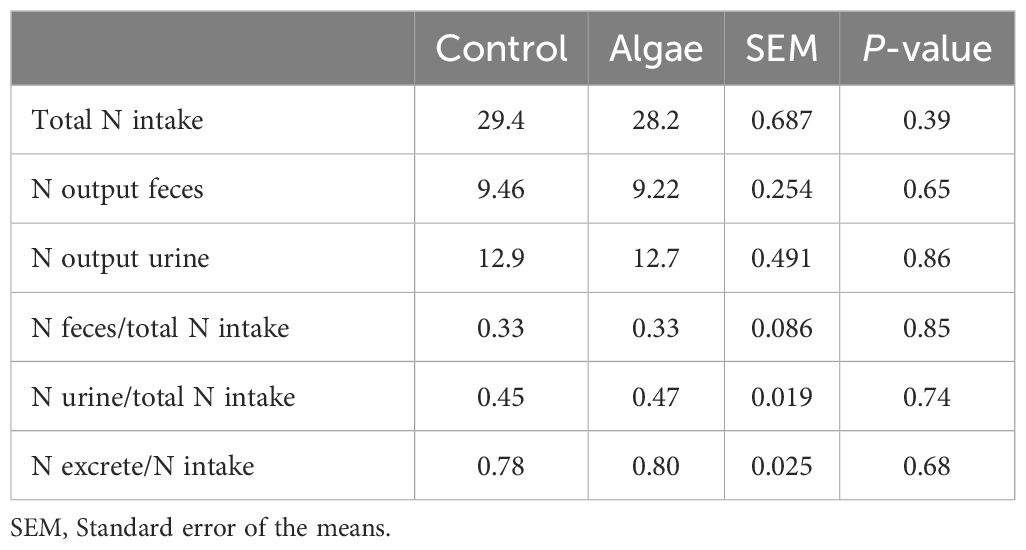
Table 4. Average intake (g/d) and excretion (g/g intake) of nitrogen in urine and feces of male lambs fed Control and Algae diet.
4 Discussion
Seaweed is considered a natural product and as such can be referred to as a supplement rather than a feed additive. Inclusion of seaweed, or natural by-products from seaweed, in a diet to livestock, thus do not need to go through a time-consuming European Food Safety Authority (EFSA) or FDA regulatory approval processes. Managing seaweed in the diets of livestock, however, needs attention due to toxic levels of heavy metals including arsenic, iodine, or other compounds, such as bromoform, that could affect animal health or if detected as residues in milk and meat products could be unsuitable or dangerous for human consumption. Although there are promising findings describing the use of the red seaweed Asparagopsis sp. as a CH4 mitigation feed supplement in ruminants, there are also report of negative attributes in terms of the bromoform content which is toxic to animal and human health (Glasson et al., 2022). Also, harvested batches of Asparagopsis are inconsistent in their mitigating ability, as it ages over time and thus can lose effectiveness and activity in addition to being expensive to purchase (Zhu et al., 2021). Due to these challenges, focus has been changed to brown European coastal species. Several of the brown species have shown contents of polyphenols and other possible bioactive compounds to potentially mitigate enteric CH4 (Wang et al., 2008), however with inconsistent results (Min et al., 2021; Roskam et al., 2022). Hansen et al. (2003) investigated the primitive sheep breed North Ronaldsay from the Orkney islands in Scotland which survives with seaweeds as their sole feed source. They report the occurrence of in vitro gas production by Laminaria ssp. which indicate these seaweeds contain phenolic compounds. Furthermore, the authors show that Laminaria spp. accounted for approximately 90% of the sheep daily feed intake and they therefore suggest that Laminaria spp have the potential to be used as an alternative feed source for other sheep breeds. North Ronaldsay sheep have adapted to these extreme conditions, and their metabolism may differ from larger intensive breeds which are bred for composite purposes (meat and wool) and as such are not comparable. Most of the results reported to date on use of brown seaweed species to mitigate enteric CH4 from ruminants are from in vitro studies as few in vivo studies have been reported (e.g., Roskam et al., 2024). Due to the inconsistencies associated with dietary supplementation with whole brown seaweeds, there has been a renewed focus on the examination of by-products and bioactives derived from seaweed for their ability to consistently mitigate enteric CH4 emissions.
4.1 Feed intake
In the current study, a by-product from L. hyperborea, was incorporated into a concentrate pellet. The inclusion rate of the by-product in the diets to sheep was 2.5%, but varying between male and female animals, and during the experiment. The increase in feed intake over time is explained by the growth of the lambs even though feed intake was highest in Period 2. The amount of concentrate inclusion in the total diet increased from Period 1 to Period 2 and was unchanged from Period 2 to Period 3. The largest differences are related to intake of grass silage but is not explained by differences in quality of the silages over the three periods (results not shown). As lambs grow, their demand for intake of high-quality feed increases. There were numerical differences in feed intake between animals of the two diets. Roskam et al. (2024) observed no differences in DMI with mature ewes supplemented with the brown seaweed Ascophyllum nodosum. Palatability was not an issue in the present experiment as all offered concentrate (which contained the seaweed biomass) was consumed.
4.2 CH4 emissions
Overall, there were no difference in CH4 emissions between the diets but differences were observed between experimental periods. The proportion of concentrate consumed was lower in period 1 which would explain the higher CH4 emissions and yield observed in Period 1.,Similar differences were observed between male and female lambs with male lambs emitting more CH4 compared to the female lambs. The proportion of concentrate in the diet to females were higher (42% DM) than that of diet to the male lambs (35% DM).
Ascophyllum nodosum is the most tested brown seaweed for nutritional and CH4 mitigation purposes. The mitigation effect of inclusion as seaweed or as an extract are inconclusive as shown for Roskam et al. (2024) who in one experiment found that inclusion of the seaweed and extract, independently in the diet of sheep, reduced CH4 emissions while similar results were absent in a repeat experiment. Use of L. hyperborea to mitigate CH4 emissions in ruminants has not been previously reported. Literature referring to this brown seaweed species are focused on their effects in anaerobic digesters on biogas production. Inclusion of L. hyperborea by-product in the current study showed inconsistent results. In Period 1, algae fed lambs had a significant reduction in enteric CH4 emission and yield compared to those fed the control diet. However, in Period 2, this was reversed and for Period 3, no differences were discovered. The rumen microbiome is the main driver for CH4 production (Tapio et al., 2017) and via the diet it is possible to manipulate their efficiency. The reported effects of polyphenols to mitigate CH4 thus is assumed to have an effect on the lower emissions and yield for Period 1.
Bioactive substances like polyphenols can modify metabolism in the rumen as identified previously by Vasta et al. (2019). Polyphenols, like phlorotannins, that are known to be exclusively found in brown seaweeds (e.g., Laminaria hyperborea), are thought to impact enteric CH4 emissions through their bioactive antimicrobial or antioxidant actions, which can interfere with the metabolism of microorganisms including methanogens in the rumen. In the present study, the phlorotannin content of the polyphenols in L. hyperborea were not quantified specifically. Further characterization work on the algal extracts is therefore required. In An in vitro study, Vissers et al. (2017) examined phlorotannin extracts from Laminaria digitata at various concentrations and observed reductions in CH4 production at dosages of 40 g/kg and greater (38-72% reduction). The concentrations of polyphenols reported in this work, are in line with values reported in previous studies focusing on the polyphenol content of L. hyperborea. For example, Wekre et al. (2022) found the content of polyphenols in L. hyperborea (expressed as mg GAE/g DW) to vary from 5.51 to 8.32 mg GAE/g DW. Concentrations of polyphenols were identified using qNMR in our study while the total polyphenol content (TPC) was determined by Wekre et al. (2019).
Considering it has been reported that the microorganisms adapt to the new environment when the diet is altered (McGovern et al., 2020), one would expect to observe less of a difference in the reduction in CH4 emissions in the lambs fed the algae diet as the rumen microbes had time to adapt. However, higher CH4 emissions and yield were recorded for lambs fed the algal supplemented diet compared to the controls in Period 2, which was unexpected. Based on these results, it seems that the main effect on the CH4 emissions is related to the level of concentrate in the diets rather than the inclusion of L. hyperborea. This is consistent with findings of Olijhoek et al. (2018) who found that increased levels of concentrate relative to forage reduced CH4 emissions by 14 – 30%.
The largest differences in CH4 emissions between the diets were found in the first period, and could possibly have been influenced by the development of the rumen and the rumen microbiota. However, in the Norwegian sheep production system, lambs are reared by their dams and follow onto pasture for four to five months before they are slaughtered. The rumen development of lambs occurs within the first two months of life with rumen microbial colonization achieved during the first month, rumen function at 2 months and anatomic development after 2 months (Jiao et al., 2015). Weaning time and access to solid feed have implications on the rumen development (Herath et al., 2021). The pre-experimental management of lambs in the present study included milk and concentrate feeding for two months before the lambs had access to pasture where they additionally were fed concentrate. Therefore, it is possible, but unlikely, that weaning time and pre-feeding influenced our results in the present experiment.
4.3 Weight
The inclusion of L. hyperborea in the diet affected lambs’ growth rate resulting in algae supplemented lambs being 3 kg lighter following feeding for 4 months. Similarly, Roskam et al. (2024) found a tendency towards the inclusion of seaweed or seaweed extract affecting daily weight gain negatively. It is not ideal to feed growing livestock ingredients or feed additives that affects performance negatively as determined in this study. The maintenance of animal performance is essential for farmers as it directly impacts farm economy which, among other things, is based on the carcass weight. However, the lambs were not slaughtered, so this is an assumption based on previous experience of an observed high correlation between animal live weight and fat free carcass weight (Brooks, 2017; Nicol et al., 1968). In the in vitro trial by Wang et al. (2008) ruminal fermentation and protein degradation was reduced with inclusion of phlorotannins. As tannins are considered to reduce ruminal degradation of proteins (Frutos et al., 2004) it could be hypothesized that the phlorotannin content in the algae diet resulted in reduced protein utilization. On the other hand, nitrogen digestibility was not affected by the inclusion of L. hyperborea in the diet which leaves more questions to be investigated to find the true mechanisms. We did not expect L. hyperborea to be a source of protein as previous analysis of different seaweed species show that brown species in general are low in digestible protein (Tayyab et al., 2016) and their amino acid composition is not beneficial for livestock growth (Gaillard et al., 2018). Red species, on the other hand, may have a more beneficial protein content as we previously determined that lambs fed Porphyra sp or soybean meal as the primary protein sources had similar growth rate (Lind et al., 2020).
4.4 By-product as feed ingredient
By-products used in diets to livestock are low-input and sustainable feeds. The costs of by-products are often less than that of whole unprocessed materials (Salami et al., 2019) and the authors summarize the benefits of plant by-products for ruminant meat production. Using the by-products from alginate production requires pressing and drying, as the dry matter is below 5%, in our case was only 3%. Most of the water-soluble compounds of the L. hyperborea biomass were removed during the extensive washings during the alginate extraction, resulting in a lower ash content, lower content of toxic heavy metals, and higher protein content than in the native seaweed. However, the content of iodine, bound to insoluble parts, was similar to the original material or even higher in concentrations. Lind et al. (2023) investigated the pathway for iodine in sheep fed L. hyperborea by-products and found that most of the iodine was excreted in feces and none of the animals’ showed signs of clinical intoxication. Costs and energy for the drying process must be considered when calculating the entire cost and benefits of the feed product. However, in a recent LCA analysis, the L. hyperborea residue performed better than other seaweed species and fractions due to the minimal processing requirements and lower allocated burdens (Thomas et al., 2025).
Salami et al. (2019) highlight that the content of bioactive compounds such as unsaturated fatty acids and phytochemicals may decrease GHG emissions while improving meat nutritional composition and shelf-life. However, there is little impact of A. nodosum inclusion on meat quality (chemical composition and sensory attributes) which was the only species reviewed when Costa et al. (2021) did their review. Salami et al. (2019) suggest that increased use of plant by-products may replace conventional forages and thus improve the environmental performance of meat production. In general, use of plant by-products can be a low-cost and low-input feed.
This study addresses new knowledge in understanding how brown seaweed species may affect enteric CH4 emissions in small ruminants. To further understand the potential anti-methanogenic effects of brown seaweed species, additional research on the supplementation of the diets of adult ewes with brown seaweeds and their by-products at different level should be performed. This will address how they respond to the seaweed inclusion. As mentioned, the content of iodine and toxic heavy metals are the most limiting factors in the amount of some brown seaweed species that can be included in the diet of ruminants. Extraction of these compounds from the seaweeds must be achieved before we can recommend higher inclusion rates of the brown species.
5 Conclusion
Discovering new feed ingredients or feed additives with anti-methanogenic properties that are applicable at farm level, are essential to meet our global targets in reducing enteric methane emissions from ruminants. This study included a by-product from the seaweed Laminaria hyperborea as a potential to mitigate enteric methane emissions from sheep. We tested if the inclusion of this by-product would reduce methane but have no negative effect on DMI or growth rate. The results showed that while methane was reduced due to dietary supplementation with the algae diet in the first period of the experiment, the overall production, yield, or intensity of methane was not affected by diet over the entire duration of the study. However, factors other than the algae inclusion may have contributed to the observed effects, and more research, with more comprehensive analyses, is needed to understand the effects on growth and methane emissions.
Data availability statement
The raw data supporting the conclusions of this article will be made available by the authors, without undue reservation.
Ethics statement
The animal studies were approved by Norwegian Ministry of Agriculture and Food Ethics Commission on Animal Use. The studies were conducted in accordance with the local legislation and institutional requirements. Written informed consent was obtained from the owners for the participation of their animals in this study.
Author contributions
VL: Conceptualization, Data curation, Formal Analysis, Funding acquisition, Investigation, Methodology, Project administration, Resources, Validation, Visualization, Writing – original draft, Writing – review & editing. MH: Conceptualization, Data curation, Methodology, Project administration, Writing – original draft, Writing – review & editing. SW: Conceptualization, Data curation, Formal Analysis, Writing – original draft, Writing – review & editing. SK: Data curation, Writing – original draft, Writing – review & editing. IA: Conceptualization, Data curation, Formal Analysis, Funding acquisition, Investigation, Methodology, Writing – original draft, Writing – review & editing.
Funding
The author(s) declare that financial support was received for the research and/or publication of this article. The project was funded by the SeaSolutions project, through the ERA-GAS cofound under Horizon 2020 with funding from the Research Council in Norway (308942).
Acknowledgments
The authors want to thank Trond Helgerud and team at IFF N&H Norway AS, who provided the Laminaria hyperborea by-product for the experiment and Felleskjøpet Fôrutvikling for producing the concentrates.
Conflict of interest
The authors declare that the research was conducted in the absence of any commercial or financial relationships that could be construed as a potential conflict of interest.
Publisher’s note
All claims expressed in this article are solely those of the authors and do not necessarily represent those of their affiliated organizations, or those of the publisher, the editors and the reviewers. Any product that may be evaluated in this article, or claim that may be made by its manufacturer, is not guaranteed or endorsed by the publisher.
Supplementary material
The Supplementary Material for this article can be found online at: https://www.frontiersin.org/articles/10.3389/fanim.2025.1430709/full#supplementary-material
References
Abbot D., Aasen I. M., Beauchemin K. A., Grøndahl F., Gruninger R., Hayes M., et al. (2020). Seaweed and seaweed bioactives for mitigation of enteric methane: Challenges and opportunities. Animals 10, 2432. doi: 10.3390/ani10122432
Brooks C. (2017). The relationship between lean meat yield and birth weight in New Zealand Romney sheep (New Zealand: Dissertation. Dep. Agricultural Sciences, Lincoln university). Available at: https://hdl.handle.net/10182/9031. (Assessed May 03, 2024)
Costa M., Cardoso C., Afonso C., Bandarra N. M., Prates J. A. (2021). Current knowledge and future perspectives of the use of seaweeds for livestock production and meat quality: a systematic review. J. Anim. Physiol. Anim. Nutr. 105, 1075–1102. doi: 10.1111/jpn.13509
FAO (2023). “Methane emission in livestock and rice systems,” in Sources, quantification, mitigation and metrics((FAO: Rome, Italy). doi: 10.4060/cc7607en
Frutos P., Hervás G., Giráldez F. J., Mantocón A. R. (2004). Review. Tannins and ruminant nutrition. Span. J. Agric. Res. 2, 191–202. doi: 10.5424/sjar/2004022-73
Gaillard C., Bhatti H. S., Novoa-Garrido M., Lind V., Roleda M. Y., Weisbjerg M. R. (2018). Amino acid profiles of nine seaweed species and their in situ degradability in dairy cows. Anim. Feed Sci. Technol. 241, 210–222. doi: 10.1016/j.anifeedsci.2018.05.003
Glasson C. R. K., Kinley R. D., de Nys R., King N., Adams S. L., Packer M. A., et al. (2022). Benefits and risks of including the bromoform containing seaweed Asparagopsis in feed for the reduction of methane production from ruminants. Algal Res. 64, 102673. doi: 10.1016/j.algal.2022.102673
Hansen H. R., Hector B. L., Feldmann J. (2003). A qualitative and quantitative evaluation of the seaweed diet of North Ronaldsay sheep. Anim. Feed Sci. Technol. 105, 21–28. doi: 10.1016/S0377-8401(03)00053-1
Hart K. J., Yanez-Ruiz D. R., Martin-Garcia A. I., Newbold C. J. (2014).Chapter 5: Sheep methane chambers at Aberystwyth University (UK) and CSIC (Spain). In: Technical manual on respiration chambers designs (New Zealand: Ministry of Agriculture and Forestry). Available online at: https://www.globalresearchalliance.org/wp-content/uploads/2012/03/GRA-MAN-Facility-BestPract-2012-ch51.pdf (Accessed 09 April 2024).
Herath H. M. G. P., Pain S. J., Kenyon P. R., Blair H. T., Morel P. C. H. (2021). Rumen Development of artificially-reared lambs exposed to three different rearing regimens. Animals 11, 3606. doi: 10.3390/ani11123606
Jiao J., Li X., Beauchemin K. A., Ta Z., Tang S., Zhou C. (2015). Rumen development process in goats as affected by supplemental feeding v. grazing: age-related anatomic development, functional achievement and microbial colonisation. Br. J. Nutri. 113, 888–900. doi: 10.1017/S0007114514004413
Kupina S., Fields C., Roman M. C., Brunelle S. (2018). Determination of total phenolic content using the Folin-C Assay: Single-Laboratory validation, First Action 2017.13. J. AOAC Int. 101, 1466–1472. doi: 10.5740/jaoacint.18-0031
Lind V., Opheim M., Sandvik J. T., Aasen I. M. (2023). Iodine intake and excretion from sheep supplemented with macroalgae (Laminaria hyperborea) by-product. Front. Anim. Sci. 4. doi: 10.3389/fanim.2023.1213890
Lind V., Weisbjerg M. R., Jørgensen G. M., Fernandez-Yepes J. E., Arbesú L., Molina-Alcaide E. (2020). Ruminal fermentation, growth rate and methane production in sheep fed diets including white clover, soybean meal or Porphyra sp. Animals 10, 79. doi: 10.3390/ani10010079
McGovern E., McGee M., Byrne C. J., Kenny D. A., Kelly A. K., Waters S. M. (2020). Investigation into the effect of divergent feed efficiency phenotype on the bovine rumen microbiota across diet and breed. Sci. Rep. 10, 15317. doi: 10.1038/s41598-020-71458-0
Min B. R., Parker D., Brauer D., Waldrip H., Lockard C., Hales K., et al. (2021). The role of seaweed as a potential dietary supplementation for enteric methane mitigation in ruminants: Challenges and opportunities. Anim. Nutri. 7, 1371–1387. doi: 10.1016/j.aninu.2021.10.003
Muizelaar W., Groot M., Van Duinkerken G., Peters R., Dijkstra J. (2021). Safety and transfer study: Transfer of bromoform present in Asparagopsis taxiformis to milk and urine of lactating dairy cows. Foods 10, 584. doi: 10.3390/foods10030584
Nicol A. M., Thomson G. G., McLean J. W. (1968). The prediction of carcass composition of lambs from live weight and red cell volume. New Z. J. Agric. Res. 11, 330–340. doi: 10.1080/00288233.1968.10431432
Olijhoek D. W., Løvendahl P., Lassen J., Hellwing A. L. F., Höglund J. K., Weisbjerg M. R., et al. (2018). Methane production, rumen fermentation, and diet digestibility of Holstein and Jersey dairy cows being divergent in residual feed intake and fed a 2 forage-to-concentrate ratio. J. Dairy Sci. 101, 9936–9940. doi: 10.3168/jds.2017-14278
Özkan-Gülzari S., Lind V., Aasen I. M., Steinshamn H. (2019). Effect of supplementing sheep diet with macroalgae species in in vivo nutrient digestibility, rumen fermentation and blood amino acid profile. Animal 13, 2792–2801. doi: 10.1017/S1751731119001502
Roskam E., Kirwan S. F., Kenny D. A., O’Donnell C., O’Flaherty V., Hayes M., et al. (2022). Effect of brown and green seaweeds on diet digestibility, ruminal fermentation patterns and enteric methane emissions using the rumen simulation technique. Front. Anim. Sci. 3. doi: 10.3389/fanim.2022.1021631
Roskam E., O’Donnell C., Hayes M., Kirwan S. F., Kenny D. A., O’Flaherty V., et al. (2024). Enteric methane emission reduction potential of natural feed supplements in ewe diets. J. Anim. Sci. 11, skad421. doi: 10.1093/jas/skad421
Salami S. A., Luciano G., O’Grady M. N., Biondi L., Newbold C. J., Kerry J. P., et al. (2019). Sustainability of feeding plan by-products: A review of the implications for ruminant meat production. Anim. Feed Sci. Technol. 251, 37–55. doi: 10.1016/j.anifeedsci.2019.02.006
Singleton V. L., Rossi J. A. (1965). Colorimetry of total phenolics with phosphomolybdic-phosphotungstic acid reagents. Am. J. Enol. Vitic. 16, 144–158. doi: 10.5344/ajev.1965.16.3.144
Tapio I., Snelling T. J., Strozzi F., Wallace R. J. (2017). The ruminal microbiome associated with methane emission from ruminant livestock. J. Anim. Sci. Biotechnol. 8, 7. doi: 10.1186/s40104-017-0141-0
Tayyab U., Novoa-Garrido M., Roleda M. Y., Lind V., Weisbjerg M. R. (2016). Ruminal and intestinal protein degradability of various seaweed species measured in situ in dairy cows. Anim. Feed Sci. Technol. 213, 44–54. doi: 10.1016/j.anifeedsci.2016.01.003
Thomas J. B., Xu V., Krizsan S. J., Aasen I. M., Oliveira A., Ramos H., et al. (2025). Seaweed as a climate fix for meat and dairy production: An LCA perspective submitted.
Vasta V., Daghio M., Cappucci A., Buccioni A., Serra A., Viti C., et al. (2019). Invited review: Plant polyphenols and rumen microbiota responsible for fatty acid biohydrogenation, fiber digestion, and methane emission: Experimental evidence and methodological approaches. J. Dairy Sci. 102, 3781–3804. doi: 10.3168/jds.2018-14985
Vissers A. M., Caligiani A., Sforza S., Vincken J.-P., Gruppen M. (2017). Phlorotannin composition of Laminaria digitata. Phytochem. Anal. 28, 487–495. Available at: https://edepot.wur.nl/418098. (Assessed May 03, 2024)
Wang Y., Xu Z., Bach S. J., McAllister T. A. (2008). Effects of phlorotannins from Ascophyllum nodosum (brown seaweed) on in vitro ruminal digestion of mixed forage or barley grain. Anim. Feed Sci. Technol. 145, 375–395. doi: 10.1016/j.anifeedsci.2007.03.013
Wasson D. W., Yarish C., Hristov A. N. (2022). Enteric methane mitigation through Asparagopsis taxiformis supplementation and potential algal alternatives. Front. Anim. Sci. 3. doi: 10.3389/fanim.2022.999338
Wekre M. E., Hellesen Brunvoll S., Jordheim M. (2022). Advancing quantification methods for polyphenols in brown seaweeds-applying a selective qNMR method compared with the TPC assay. Phytochem. Anal. 33, 1099–1110. doi: 10.1002/pca.3162
Wekre M. E., Kåsin K., Underhaug J., Holmelid B., Jordheim M. (2019). Quantification of polyphenols in seaweeds: A case study of ulva intestinalis. Antioxidants 8 (12), 612. doi: 10.3390/antiox8120612
Keywords: methane abatement, sheep, seaweed, feed intake, ruminants
Citation: Lind V, Hayes M, Waters SM, Kirwan SF and Aasen IM (2025) Methane emissions and growth rate of lambs fed Laminaria hyperborea supplemented diet. Front. Anim. Sci. 6:1430709. doi: 10.3389/fanim.2025.1430709
Received: 10 May 2024; Accepted: 27 March 2025;
Published: 15 April 2025.
Edited by:
Marcos Inacio Marcondes, William H. Miner Agricultural Research Institute, United StatesReviewed by:
Gemma A Miller, Scotland’s Rural College, United KingdomAndrea Vitali, University of Tuscia, Italy
Copyright © 2025 Lind, Hayes, Waters, Kirwan and Aasen. This is an open-access article distributed under the terms of the Creative Commons Attribution License (CC BY). The use, distribution or reproduction in other forums is permitted, provided the original author(s) and the copyright owner(s) are credited and that the original publication in this journal is cited, in accordance with accepted academic practice. No use, distribution or reproduction is permitted which does not comply with these terms.
*Correspondence: Vibeke Lind, dmliZWtlLmxpbmRAbmliaW8ubm8=