- 1School of Agriculture and Veterinary Sciences (FCAV), São Paulo State University (UNESP), Jaboticabal, SP, Brazil
- 2Department of Structural, Molecular Biology and Genetics, Ponta Grossa State University (UEPG), Ponta Grossa, PR, Brazil
- 3School of Veterinary and Animal Science (FMVZ), São Paulo State University (UNESP), Botucatu, SP, Brazil
- 4Range Cattle Research & Education Center, University of Florida, Ona, FL, United States
Introduction: To determine the impact of early weaning combined with concentrate supplementation on skeletal muscle energy metabolism, we profiled gene co-expression networks in Bos indicus (Nellore) calves.
Material and methods: Longissimus thoracis biopsies were collected from eight calves per treatment (conventional and early weaning) at 120 and 205 days of age (longitudinal sampling). All calves grazed Brachiaria decumbens pasture until 120 days. Subsequently, early weaned calves received a 1% body weight concentrate supplement (20% CP, 75% TDN) post-weaning. Total RNA was extracted, and 32 mRNA libraries were generated for RNA sequencing. Using normalized count matrix, we constructed gene co-expression modules using webCEMiTool and performed over-representation analysis (ORA) for pathway enrichment (KEGG). Additionally, we used Gene Set Enrichment Analysis (GSEA) to evaluate the regulatory activity (up- or downregulation) of identified gene modules.
Results: Results: Modules associated with pathways such as insulin signaling, unsaturated fatty acid biosynthesis, and PPAR signaling showed a significantly higher proportion of upregulated genes. Key hub genes within these early weaning-related modules were linked to lipid synthesis and adipocyte differentiation. Thus, early weaning followed by concentrate supplementation modified the gene expression profile, enhancing pathways involved in energy metabolism, adipogenesis, lipogenesis, and lipolysis inhibition.
Discussion: These findings suggest that early nutritional intervention can positively influence metabolic pathways associated with growth and body composition in Nellore calves. Despite the multifactorial nature of these traits and their susceptibility to modification during post-weaning phases, the current results demonstrate potential for long-term positive effects on carcass composition and final product quality.
1 Introduction
Zebu cattle, predominantly Nellore, represent 80% of Brazil’s 238 million beef herd (de Nadai Bonin et al., 2021; Brazilian Institute of Geography and Statistics, 2023) due to their adaptability to tropical climates conditions. However, seasonal forage variability significantly impacts production (Santana et al., 2016; Pereira-Flores et al., 2023). To mitigate weight loss and improve carcass finishing, animal breeding and nutritional strategies are crucial (Schmidt and Olson, 2007; Novais et al., 2022; Londoño-Gil et al., 2022; Berton et al., 2022). Early weaning emerges as an important nutritional intervention. By reducing the energy demands of lactation, early weaning facilitates faster recovery of cow body condition, directly enhancing pregnancy rates and overall herd reproductive efficiency (Lamb et al., 2010; Ayres et al., 2014; Da Silva et al., 2017; Moorey and Biase, 2020; Alforma et al., 2023; Nishimura et al., 2023).
Although primarily aimed at improving the reproductive indices of a herd, especially of young cows, early weaning provides the opportunity to supplement early weaned calves with a concentrate diet in a controlled environment and with better pastures (Rasby, 2007). Depending on supplementation levels, the intake of concentrate at this stage can improve the development of these calves and potentially accelerate weight gain rates during the postweaning phase (Fluharty et al., 2000; Myers et al., 1999b). Furthermore, in the case of European breeds, studies have shown that feeding early weaned calves a grain-rich diet promoted greater fat deposition, higher marbling scores, and higher carcass yield and quality at slaughter (Myers et al., 1999a; Sithyphone et al., 2011; Scheffler et al., 2014; Moriel et al., 2014). There is also evidence suggesting that feeding concentrate combined with roughage or starch during the preweaning period has great potential to affect the expression of genes related to adipogenesis in Zebu cattle (Reddy et al., 2017; Pedro et al., 2023; Abitante et al., 2024).
Phenotypic plasticity, driven by metabolic adaptations to nutrient availability, is governed by intricate signaling cascades and transcriptional modulation of key genes (Cantalapiedra-Hijar et al., 2018). High-resolution gene expression profiling of metabolically active tissues, including muscle, rumen, and liver, provides critical insights into the scope of metabolic remodeling induced by nutritional management strategies in beef cattle (Fernandes et al., 2024; Abbas et al., 2020; Zarek et al., 2017). In contrast to differential expression analysis, which relies on statistical significance and arbitrary relative expression (fold-change) thresholds, co-expression network analysis employs correlation-based module detection. This system-level approach, integrated with functional annotation, elucidates gene interaction networks and infers functional associations. Given the inherent correlation of expression profiles among genes participating in shared biological processes, co-expression analysis effectively identifies co-regulated gene sets, offering a comprehensive understanding of adaptive metabolic responses (Rau and Maugis-Rabusseau, 2018; Cheng et al., 2020). Approaches to inferring co-expressed gene modules vary with experimental design. Commonly, Weighted Gene Co-expression Network Analysis (WGCNA) are employed for broad co-expression profiling treating samples without regard to experimental groups. Essentially, WGCNA identifies gene sub-networks that may or may not correlate with the conditions being studied. The webCEMiTool uses WGCNA and add a post-hoc Gene Set Enrichment Analysis (GSEA) to statistically assess the enrichment of up- or downregulated genes within each module. This allows us to find co-expressed network modules that are either linked or unlinked to the experimental conditions, providing a comprehensive view of gene co-expression changes. Moreover, through the integration of co-expression and interaction data, this analytical framework generates a discrete set of hub genes for each identified module.
Even though some efforts have been made to understand the impacts of feeding calves a whole grain-rich diet during the perinatal phase on phenotype and metabolism (Arthington and Kalmbacher, 2003; Meyer et al., 2005; Moriel et al., 2014; Myers et al., 1999a, b; Scheffler et al., 2014; Graugnard et al., 2009), studies involving tropical breeds are still scarce (Reddy et al., 2017; Pedro et al., 2023; Nishimura et al., 2023; Abitante et al., 2024). This study aims to compare co-expressed gene modules in Nellore calves under conventional vs. early weaning and evaluate metabolic pathway differences in skeletal muscle.
2 Material and methods
2.1 Animals and treatments
Forty male calves were divided into two groups: 20 early weaned animals (referred to as EW) and 20 conventionally weaned animals (CW). A field experiment was conducted on a properly regulated commercial farm located in the Pantanal biome, Mato Grosso, Brazil. All animals were born in the same month to contemporary cows of the same calving order, raised in the same batch and with similar weights to the beginning of the experiment 122.5 Kg ± 2.961 and 123.15 Kg ± 2.606 for CW and EW respectively. The CW group remained with their mothers on pasture until weaning at 205 days of age. The EW group stayed with their mothers on pasture until 120 days of age, when they were weaned and then transferred to a single Brachiaria decumbens paddock where they were supplemented with concentrate (Figure 1). The details of the diet are presented in Table 1.
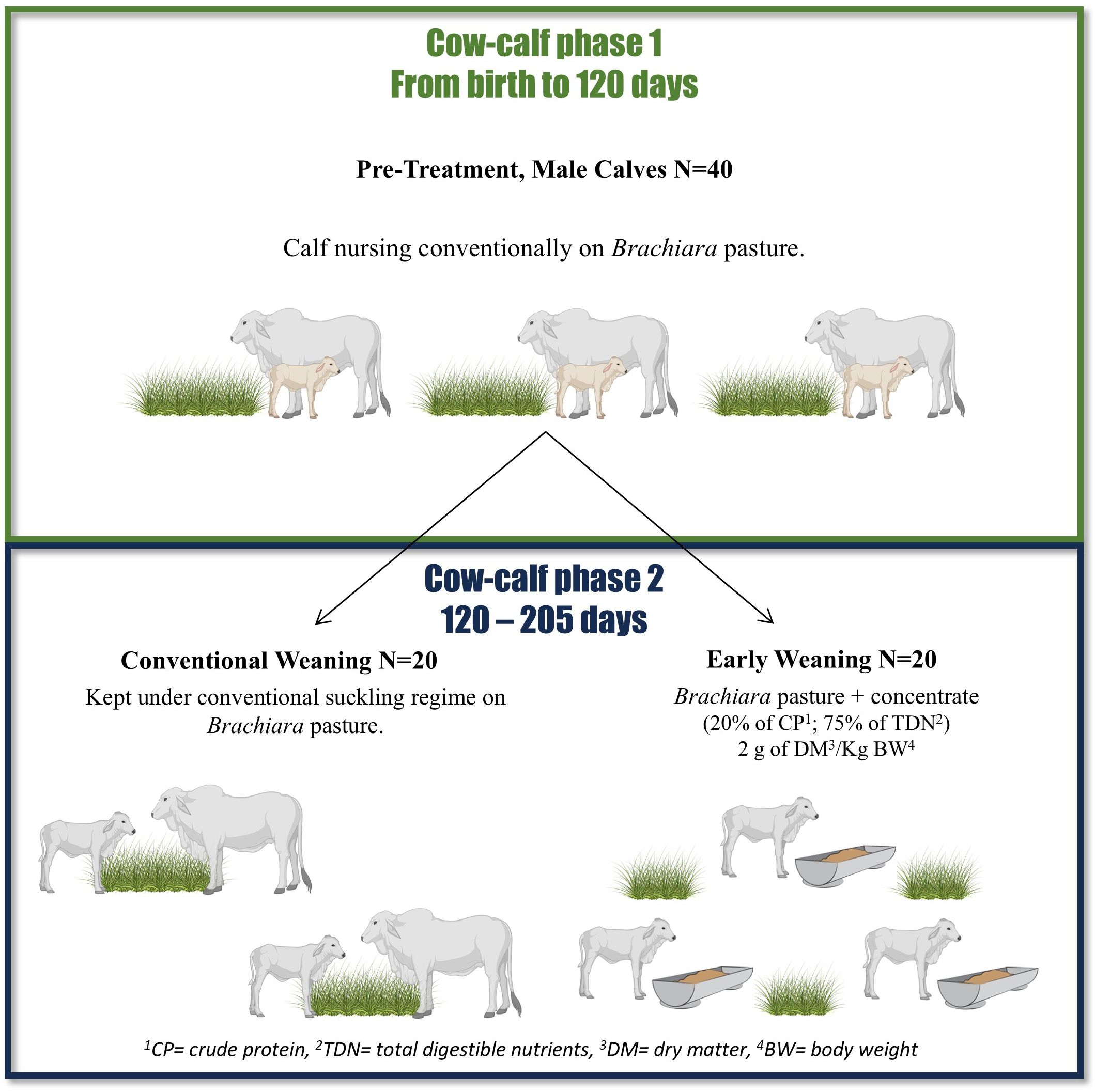
Figure 1. Representation of the experimental design. At 120 days of age, half of the calves from the initial group were weaned and moved to a Brachiaria paddock, where they received a diet based on natural pasture and supplementation composed of soybean meal, corn, additives, and minerals.
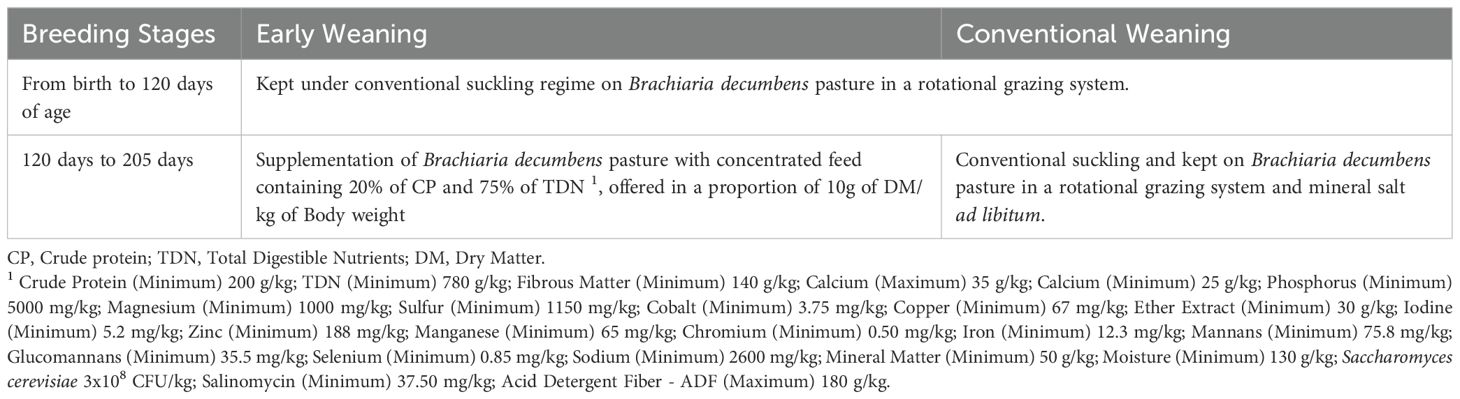
Table 1. Supplementation and diets based on natural forage, mineral salt and technical feed containing soybean meal, corn, additives and minerals, provided to Nellore cattle at different stages of development and subjected to different weaning protocols.
2.2 Collection of muscle tissue and RNAseq
Longissimus thoracis muscle aliquots were collected from eight steers of the EW group and eight animals of the CW group at two time points: 120 days of age (beginning of early weaning, time point 1 - T1) and 205 days of age (end of conventional weaning, time point 2 - T2) (Table 2). The samples were used for subsequent total RNA extraction using the TRIzol™ protocol (Invitrogen, Carlsbad, CA). To ensure adequate total RNA quality, only samples with an RNA integrity number (RIN) ≥ 7 were used for sequencing. The mRNA underwent purification and fragmentation steps, resulting in 32 cDNA libraries prepared with the TruSeq RNA Library Prep Kit v2 (Illumina, USA), 16 CW and 16 EW (8 per treatment and time point). Sequencing was performed on the NextSeq 550® System platform (Illumina, USA), in order to produce 150 bp paired-end (PE) reads, with an expected average coverage of 10 million PE reads per sample. The pipeline described by Pedro et al. (2023) was followed to obtain count data from RNAseq outputs. First, FastQC v. 0.11.9 (Andrews, 2010) was used to analyze the quality of raw reads. Sequencing adapters, indexes and low-quality sequences (Phred score < 20) were removed using the fastp v.0.20.0 (Chen et al., 2018), the reads obtained were mapped to the bovine reference genome ARS-UCD1.2 (https://www.ncbi.nlm.nih.gov/datasets/genome/GCF_002263795.1/) using STAR v.2.7.20 (Dobin et al., 2013). The reads were mapped independently for each sample and only sequences mapping to the reference genome and known chromosomes were considered. The MultiQC v. 1.13 software (Ewels et al., 2016) was used to evaluate the quality of alignment. The number of paired-end reads mapped per gene was then used to construct a count matrix (genes x samples) using the featureCounts v.2.0.3 software (Liao et al., 2014).
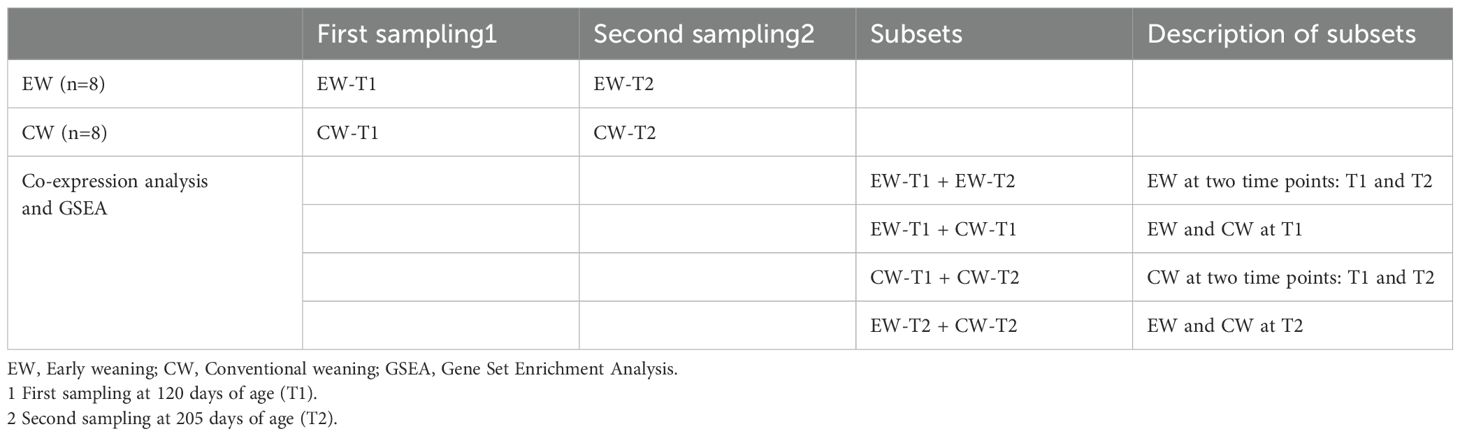
Table 2. Sample collection and subsets used for co-expression and enrichment analyses of gene modules in the experimental groups.
2.3 Identification of co-expressed gene modules
Co-expressed gene modules were determined using the webCEMiTool software (Cardozo et al., 2019). WebCEMiTool is a web-based version developed from the CEMiTool of the R package (Russo et al., 2018). For analysis, a count matrix file (genes x samples), a sample annotation file (to distinguish the experimental groups to which each sample belongs), a gene interactions file, and a gene set file based on the KEGG database for subsequent functional analysis were used. Briefly, in the first step, the software automatically filters the input genes. It assumes by default an inverse gamma distribution of the genes and selects genes based on a predetermined p-value, the most restrictive value was chosen to improve the reliability of the results (p = 0.01). Also, a variance-stabilizing transformation (VST), was applied this transformation is required when the data set shows mean-variance dependence. Additionally, the package removes by default 25% of the genes with the lowest mean expression across samples (Russo et al., 2018). In the next step, webCEMiTool determines the correlation values between each pair of genes present in the expression file; the method chosen for this study was Pearson correlation. First, we conducted correlation analysis between EW and CW at T2 (EW-T2 + CW-T2) and between the two different time points in the EW group (EW-T1 + EW-T2). To corroborate the results, we also performed correlation analysis between CW and EW at T1 (EW-T1 + EW-T2) and between the two time points in the CW group (CW-T1 + CW-T2). Each of these subsets contained 16 samples, eight per group. For further details, see Table 2.
2.4 Enrichment of functional terms
Using webCEMiTool (https://cemitool.sysbio.tools/), over-representation analysis (ORA) was performed based on the genes found in each module. For this purpose, a file containing KEGG pathway annotations was provided. This step was performed using the Enrichr tool (Chen et al., 2013) integrated in webCEMiTool. The analysis was based on the hypergeometric test corrected for false discovery rate using the Benjamini-Hochberg method, with significance considered at p-adj. ≤ 0.05.
2.5 Gene set enrichment analysis
Finally, to understand the relationship among gene modules and treatments applied in this study. Enrichment Analysis (GSEA) was performed using webCEMiTool to represent module activity within each experimental group. For this, a ranked list of all input genes (L) is generated based on z-score normalization of gene expression within each experimental group and a list of module genes (S). These lists were overlapped and then the module genes position on L is considered to calculate enrichment scores (ES). These values are used to perform a weighted Kolmogorov–Smirnov statistic test comparing the ranks of genes in S with the uniform distribution. If most of the genes in S are found at the top or the bottom of the list L, the set is considered to be enriched. However, if the distribution of genes in S was homogeneous across L, the set was not considered enriched. Then a normalized enrichment score is calculated, so that multiple modules can be compared in the same plot. The normalized enrichment score (NES) is visually represented by the intensity of the color and the size of the circles. Positive NES values indicate that genes in S were found at the top of L (upregulated), while negative values indicate they were at the bottom (downregulated). A value of zero indicates no enrichment (Subramanian et al., 2005; Russo et al., 2018).
3 Results
Sixteen modules and a total of 1,883 genes were inferred from the EW-T2 + CW-T2 subset, while 11 modules comprising 1,522 genes were inferred from the EW-T1 + EW-T2 subset. In EW-T1 + CW-T1 and CW-T1 + CW-T2, four and five modules comprising a total of 1,030 and 1,096 genes were inferred, respectively (Supplementary Table S1).
All modules in each subset were submitted to functional enrichment of metabolic pathways (KEGG). In the EW-T2 + CW-T2 subset, there were eight modules with at least one enriched pathway, although the sum of all significantly enriched metabolic pathways in its eight modules was the smallest compared to the other subsets, with only 50% of the modules containing some enriched pathway (Figure 2B). At least one enriched pathway per module was observed in the EW-T1 + CW-T1 and CW-T1 + CW-T2 subsets (Figures 2A, C). In the datasets including supplemented individuals, some modules did not contain enriched pathways (Modules: 4, 7, 9, 11, 12, 13, 14, 15 and 16 in EW-T2 + CW-T2; Modules: 4, 6, 7 and 10 in EW-T1 + EW-T2) (Figures 2B, D).
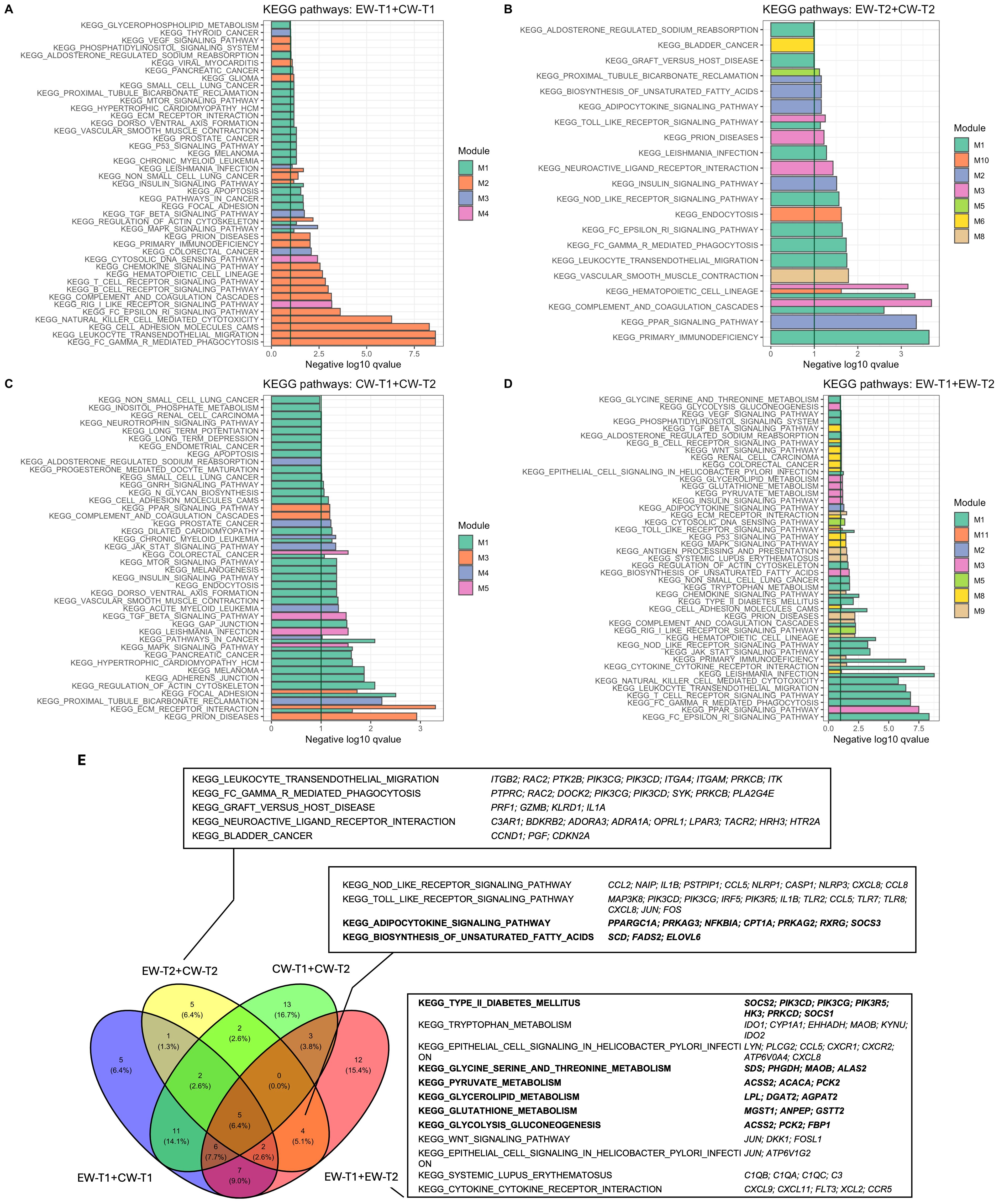
Figure 2. Functional enrichment based on over-representation analysis for modules prospected for each subset data: (A) EW and CW at T1; (B) EW and CW at T2; (C) T1 and T2 (CW); (D) T1 and T2 (EW). Venn diagram indicating overlapped KEGG pathways for data subset containing early-weaning information (E).
The metabolic pathways overlapped from the experimental groups revealed pathways shared by the datasets containing the supplemented experimental group (EW-T2 + CW-T2 and EW-T1 + EW-T2), including adipocytokine signaling, TOLL- and NOD-like receptors, and saturated fatty acid biosynthesis. In addition, exclusive pathways related to energy metabolism were found in EW-T1 + EW-T2 (glycolysis-gluconeogenesis and type II diabetes pathways) (Figure 2E).
The visualization of the enriched pathways (PPAR, insulin and adipocytokine signaling pathway; biosynthesis of unsaturated fatty acids; pyruvate, glutathione, glycerolipid metabolism, and glycolysis gluconeogenesis) from modules 2 and 3 in EW-T2 + CW-T2 and EW-T1 + EW-T2 indicated an effect of the early weaning treatment applied (Figures 2B, D).
GSEA (Figures 3A–D) demonstrated positive activity for these modules to lipid metabolism, suggesting an active role in energy homeostasis, indicating increased relative expression of the gene sets from module 2 in EW-T2 + CW-T2 and module 3 in EW-T1 + EW-T2 in the experimental group supplemented with the concentrate diet (EW-T2), with values of normalized enrichment score NES = 2.22 and adjusted p-value = 0.02133 for M2 in EW-T2 + CW-T2, and NES = 2.65 and adjusted p-value = 0.0006 for M3 in EW-T1 + EW-T2 (Figures 3E, F), in which a large part of the gene sets responsible for positive and significant scores were also enriched in energy metabolic pathways, especially those linked to lipids and fatty acids.
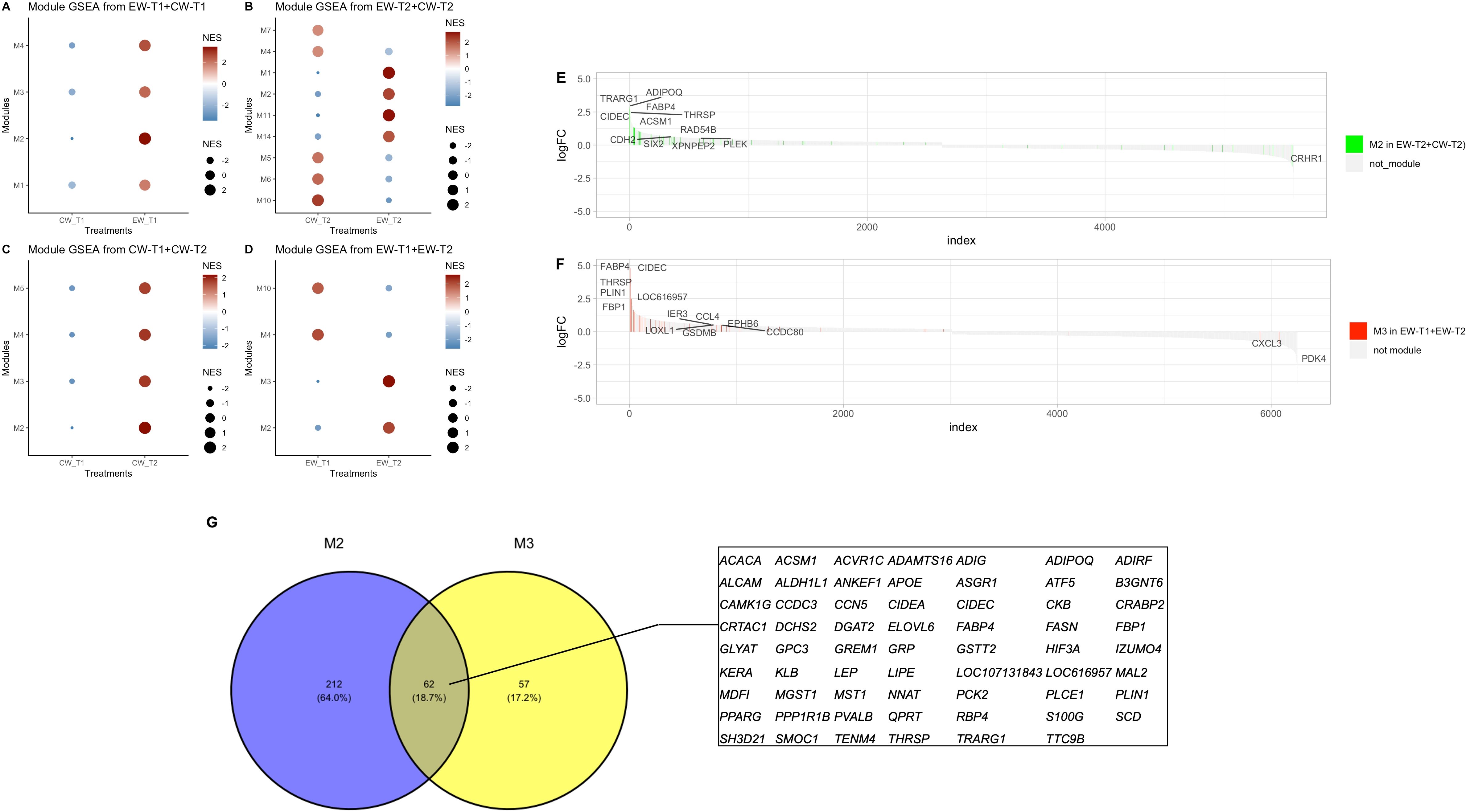
Figure 3. Gene Set Enrichment Analysis (GSEA) representing the activity of modules within each experimental group and between the four comparisons performed. (A) EW and CW at T1; (B) CW and EW at T2; (C) T1 and T2 (CW); (D) T1 and T2 (EW). Normalized enrichment score (NES), color, and size indicate the direction (up or downregulated) and extent of normalized enrichment score; GSEA plot of modules associated to energetic metabolism pathways (E, F); and overlapped M2 and M3 genes (G).
The analysis of hub genes revealed the presence of the following lipid metabolism genes within the modules, 2 in EW-T2 + CW-T2 and 3 in EW-T1 + EW-T2: Perilipin 1 (PLIN1), an important gene that significantly contributes to the protection of lipid droplets from lipase enzymes; Cell death inducing DFFA like effector C (CIDEC), the product of this gene is a lipid droplet-associated protein that acts together with PLIN1 and that is involved in the suppression of lipolysis and increase in lipid droplet size; Acyl-coenzyme A synthetase short-chain family member 2 (ACSS2), this gene encodes an enzyme known as acetyl-CoA synthetase 2, which catalyzes the conversion of acetate to acetyl-CoA, a fundamental building block for the synthesis of fatty acids and cholesterol; Fatty acid binding protein 4 (FABP4), this protein binds to fatty acids and transports them into adipocytes, and ADIPOQ, the product of this gene, adiponectin, is secreted by adipocytes and promotes adipocytes differentiation, and is associated with increased lipid deposition in mature adipocytes (Supplementary Table S1) the presence of these genes in the context of early weaning possibly indicates the positive effect of supplementation on increased lipid storage and adipocyte differentiation.
The genes shared by module 2 in EW-T2 + CW-T2 and of module 3 in EW-T1 + EW-T2 (Figure 3G) included stearoyl-CoA desaturase (SCD1), fatty acid desaturase 1 (FADS1), ELOVL fatty acid elongase 6 (EVOLV6), phosphoenolpyruvate carboxykinase 1 (PCK1), phosphoenolpyruvate carboxykinase 2 (PCK2), protein kinase AMP-activated non-catalytic subunit gamma 2 (PRKAG2), protein kinase AMP-activated non-catalytic subunit gamma 3 (PRKAG3), carnitine palmitoyltransferase 1A (CPT1A), suppressor of cytokine signaling 3 (SOCS3), and PPARG coactivator 1 alpha (PPARGC1A), in addition to the transcription factor retinoid X receptor gamma (RXRG) genes, all of them essential components for fatty acid synthesis, fat deposition, adipocyte differentiation, and regulation of energy metabolism.
4 Discussion
The data obtained here demonstrated a change in the expression of genes related to energy and lipid metabolism in Nellore animals submitted to early weaning. Nutrition affects the expression of genes involved in lipid metabolism and studies on taurine breeds have shown that early weaning and dietary manipulation can alter the fat composition and marbling of beef (Myers et al., 1999a; Sithyphone et al., 2011; Scheffler et al., 2014; Moriel et al., 2014).
A larger number of co-expression modules was detected in the EW-T2 + CW-T2 and EW-T1 + EW-T2 subsets compared to EW-T1 + CW-T1 and CW-T1 + CW-T2. The last two sets were formed by data from animals that did not receive concentrate diet as nutritional supplementation. This is not necessarily a pattern, but at time point 1, when analyzing data from both experimental groups, no treatment effect is observed in either group. Similarly, when using CW data across two time points, no treatment effect is detected. As a result, in both cases, there is no variability in gene expression due to changes in metabolic pathways, unlike when data from supplemented animals are included.
In the same way, pathways enriched from modules derived solely from CW or EW pre-treatment result in fewer but larger modules, containing a greater number of genes. These genes are associated with pathways commonly activated during development, making them more easily enriched. Conversely, incorporating post-supplementation data reveals a greater number of metabolic pathways influenced by supplementation, generating many more modules, some with only a few genes, which often leads to no enrichment of pathways. Thus, the focus of this discussion will be in the EW-T2 + CW-T2 subset, which is the main comparison of this study, and in the module 2 which is more related to lipid and energy metabolism.
The insulin signaling pathway, which triggers a cascade of intracellular events in response to binding of insulin to its receptors (Abe et al., 1997), this pathway was upregulated in the EW group after supplementation with concentrate feed, possibly as a result of increased energy availability from the diet. In their late stage of differentiation, adipocytes acquire insulin sensitivity as a result of increased availability of the glucose transporter GLUT4 (Ladeira et al., 2016). GLUT4 belongs to the family of insulin-sensitive glucose transport proteins, which are exclusively expressed in muscle and adipose tissue (Abe et al., 1997). This increases intracellular glucose levels which, in the context of carbohydrate metabolism, result in enhanced glycolytic activity (adipose and muscle tissue), stimulate glycogen synthesis (adipose tissue, muscle, and liver), reduce the rate of glycogen depletion in muscle tissue and liver, and inhibit the rate of glycogenolysis and gluconeogenesis in the liver (Dimitriadis et al., 2011).
In adipocytes, increased glucose availability can lead to a decrease in lipolysis (Dimitriadis et al., 2011). Furthermore, saturation of the glycolytic pathway triggers the activation of de novo lipogenesis (Ameer et al., 2014) and provides pyruvate for conversion to acetyl-CoA, feeding the tricarboxylic acid cycle, which consequently promotes upregulation of the entire fatty acid synthase (FASN) biosynthetic pathway (Baldwin et al., 2006; Ameer et al., 2014). Thus, the impact of early weaning on the insulin signaling pathway observed in the Zebu animals studied here corroborates the proposal by Schoonmaker et al. (2003) that activation of de novo lipogenesis improves the performance of intramuscular fat deposition. In protein metabolism, the insulin pathway signals an increase in the rate of transport of some amino acids to tissues, increases the rates of protein synthesis in muscle, adipose tissue, liver and other tissues, and decreases the rates of protein degradation in muscle (Dimitriadis et al., 2011).
Another pathway highlighted in module 2 of this study was the peroxisome proliferator-activated receptor (PPAR) signaling pathway, which is involved in the regulation of lipid and glucose metabolism (Bionaz et al., 2013; Ladeira et al., 2016). PPAR are a family of nuclear receptors activated by fatty acids. Their isoforms form a heterodimer with the retinoid X receptor (RXR) for activation and consequent binding to regulatory sites of numerous energy metabolism genes, where they can induce or repress expression (Lemay and Hwang, 2006). The PPARα isoform is highly expressed in the liver, followed by the intestine, adipose tissue and heart (Bunger et al., 2007), and plays a key role in fatty acid oxidation (Tyagi et al., 2011). PPARγ is encoded by the PPARG gene, which was identified as a hub gene in module 2 of this study. This isoform is highly expressed in adipose tissue and, to a lesser extent, in muscle tissue (Kersten, 2014) where it plays a fundamental role in the control of adipogenesis, lipogenesis, and insulin sensitivity (Olefsky and Saltiel, 2000).
PPARγ plays a central role in adipogenesis, and its upregulation in cattle is likely responsible for the observed increase in intramuscular fat deposition, as reported by Lim et al. (2011) Studies using in vitro cell cultures suggested that PPARγ and CCAAT/enhancer-binding proteins (C/EBP) are crucial factors for controlling gene expression during adipogenesis, from multipotent stem cell commitment to adipocyte differentiation (Cho and Jefcoate, 2004; Fernyhough et al., 2007).
Moisá et al. (2014) also found altered expression of PPARG in early weaned Angus and Angus × Simmental calves. In that study, early weaned animals (141 days, day 0 of treatment) were fed an exclusive high-starch diet, while animals that remained with the cows on pasture received high-starch feed in a creep-feeding regimen until they were weaned at 222 days of age (Moisá et al., 2014). The authors observed alterations in the expression of PPARG and other adipogenic and lipogenic activators in the early weaning group, in addition to genes encoding enzymes that participate in intramuscular fat deposition (Moisá et al., 2014). There was a gradual increase in these fat deposition pathways in early weaned animals, reaching its peak between 96 and 167 days of treatment, which was not observed in the conventional weaning group. Furthermore, after slaughter, the highest marbling scores occurred in the group of early weaned crossbred Angus × Simmental animals, followed by the group of early weaned purebred Angus animals (Moisá et al., 2014). Also comparing early and conventional weaning (70 and 250 days of age, respectively) of beef calves with Zebu genetic influence, Moriel et al. (2014) found that early exposure to a high-concentrate diet improved the growth performance of early weaned calves and induced adipocyte differentiation, concomitant with higher expression of PPARG compared to the conventional weaning group. Thus, the alteration in the PPAR pathway observed in our study indicates positive regulation of genes and pathways involved in adipocyte differentiation and lipogenesis in the early weaned group supplemented with concentrate.
One of the lipid metabolism genes highlighted in our study in early weaned animals is PLIN1, which was identified as a hub gene in module 2 and was enriched in the PPAR signaling pathway). Under basal conditions, PLIN1 (hypo-phosphorylated) protects lipid droplets from lipases present in the cytosol by acting as a valve that prevents the enzyme from entering the droplets, thus contributing to lipid storage (Maurizi et al., 2018). PLIN1 is phosphorylated by protein kinase A (PKA). After phosphorylation, it promotes lipolytic activity by transporting hormone-sensitive lipase (HSL) to the surface of lipid droplets to facilitate lipolysis under energy-deficient conditions (Raza et al., 2020; Shijun et al., 2020).
A detailed analysis of the PLIN1 gene by Shijun et al. (2020) provided information about its function in cattle. The highest expression levels of PLIN1 are observed in subcutaneous fat, followed by heart and muscle (Longissimus). Peak expression occurs during adipocyte differentiation from day 2 to day 6, followed by a decrease from day 6 to day 10 (Shijun et al., 2020). Knockdown of PLIN1 and of its transcription factors in adipocytes upregulated the expression of lipase genes, HSL, and adipose triglyceride lipase (ATGL) and inhibited the expression of stearoyl-coenzyme A desaturase 1 (SCD1), acetyl-CoA carboxylase (ACC) encoded by ACACA gene, and sterol regulatory element-binding protein 1 (SREBP-1c), consequently inhibiting fatty acid synthesis and increasing lipolysis (Shijun et al., 2020). The transcription factors E2F1, C/EBPβ, PLAG1, and SMAD3 are the main transcriptional regulators of PLIN1 and the joint action of these genes plays an important role in bovine adipogenesis and lipogenesis (Shijun et al., 2020). The presence of this gene in the positively enriched module for early-weaned animals suggests an increased ability to store lipids, as this gene plays a crucial role in protecting lipid droplets from lipases, thereby promoting greater fat retention in adipose tissue (Maurizi et al., 2018).
Similarly, other genes that play a broad role in lipid metabolism in cattle, such as SCD1, ACACA, and FASN, were also found in module 2 and enriched in the PPAR and Insulin signaling pathway. Sterol regulatory element binding protein-1c (SREB-1c) encoded by the sterol regulatory element-binding transcription factor 1 (SREBF1) gene and PPARγ are reported as the main transcription factors involved in lipid metabolism in beef cattle (Ladeira et al., 2016). SCD1 encodes stearoyl-Coenzyme A-desaturase 1 (SCD), an enzyme that converts saturated to unsaturated fatty acids. Its expression has been shown to be regulated by SREBPF1 (Bénédicte et al., 2006; Sampath and Ntambi, 2006). Within this context, changes in the expression of SREBF1 have been shown to alter the synthesis of SCD, leading to differences in the fatty acid composition of animal adipose tissue (Sampath and Ntambi, 2006). ACACA and FASN are also controlled by SREBP-1c and are the main enzymes involved in the de novo synthesis of fatty acids (Ladeira et al., 2016). Although SREBF1 is not present within module 2, it is regulated by PLIN1 as demonstrated by Shijun et al., 2020, and yet SREBF1 is associated with the regulation of SCD1, ACACA and FASN.
The cell death inducing DFFA like effector C (CIDEC) gene, identified as a hub gene in module 2, is also involved in lipid metabolism. Kim et al. (2008) demonstrated that the transcriptional activity of CIDEC is induced by PPARγ2 during adipocyte differentiation. The product of CIDEC is a lipid droplet-associated protein that colocalizes with PLIN1 and that is involved the suppression of lipolysis, enhancement of droplet size, and accumulation of triglycerides (Puri et al., 2007). Also identified as a hub gene in module 2, retinol binding protein 4 (RBP4) encodes a circulating protein (RBP4 gene) that acts as a transporter of retinol (vitamin A) from the liver to peripheral organs (Liu et al., 2019). However, its function could be much broader since it has been proposed that RBP4 can bind and transport fatty acids (Perduca et al., 2018). Furthermore, RBP4 has been associated with intramuscular fat deposition in Longissimus muscle of cattle (De Jager et al., 2013). Analyzing taurine and Zebu breeds, the authors identified RBP4 among a set of genes involved in the lipid metabolism of intramuscular adipocytes and demonstrated a positive correlation between the expression of the gene and intramuscular fat deposition.
These genes are directly related to lipogenesis and adipogenesis, metabolic characteristics that are greatly influenced by feeds with higher energy levels, indicating a possible association of these modules with the supplementation provided to early weaned calves. This becomes more evident by the fact that hub genes with similar functions were not detected in sets involving non-supplemented calves.
The results indicate that early weaning combined with concentrate supplementation significantly impacts the expression of genes involved in lipid metabolism. These changes are associated with increased adipogenesis, lipogenesis, and de novo fatty acid synthesis, along with reduced lipolysis. The regulation of these genes and pathways in response to early weaning and concentrate supplementation may enhance intramuscular fat deposition and muscle growth, leading to improved marbling and fat accumulation in Nellore cattle, which is essential for enhancing beef quality.
Data availability statement
The datasets presented in this article are not readily available because they are currently being used in ongoing, unpublished studies. Requests to access the datasets should be directed to Z3VpbGhlcm1lLmx1aXNAdW5lc3AuYnI=.
Ethics statement
The animal study protocol was approved by the Institutional Ethics Committee of the College of Veterinary and Animal Science of the University of São Paulo State, Brazil (protocol code 0190/2020 on 16 December 2020). The studies were conducted in accordance with the local legislation and institutional requirements. Written informed consent was obtained from the owners for the participation of their animals in this study.
Author contributions
GR: Data curation, Formal Analysis, Software, Writing – original draft, Writing – review & editing. GT: Formal Analysis, Software, Writing – original draft. MV: Writing – review & editing. RN: Data curation, Formal Analysis, Writing – original draft. PM: Formal Analysis, Software, Writing – original draft. JT: Investigation, Writing – original draft. RC: Resources, Supervision, Writing – original draft. PM: Supervision, Writing – original draft. WB: Resources, Writing – review & editing. LC: Visualization, Writing – review & editing. GP: Conceptualization, Funding acquisition, Methodology, Project administration, Software, Validation, Visualization, Writing – review & editing.
Funding
The author(s) declare that financial support was received for the research and/or publication of this article. This research was funded by São Paulo Research Foundation (FAPESP), grant number 2019/12851-1.
Acknowledgments
The authors thank the São Paulo Research Foundation, FAPESP for financial support.
Conflict of interest
The authors declare that the research was conducted in the absence of any commercial or financial relationships that could be construed as a potential conflict of interest.
Generative AI statement
The author(s) declare that no Generative AI was used in the creation of this manuscript.
Publisher’s note
All claims expressed in this article are solely those of the authors and do not necessarily represent those of their affiliated organizations, or those of the publisher, the editors and the reviewers. Any product that may be evaluated in this article, or claim that may be made by its manufacturer, is not guaranteed or endorsed by the publisher.
Supplementary material
The Supplementary Material for this article can be found online at: https://www.frontiersin.org/articles/10.3389/fanim.2025.1533043/full#supplementary-material
References
Abbas W., Keel B. N., Kachman S. D., Fernando S. C., Wells J. E., Hales K. E., et al. (2020). Rumen epithelial transcriptome and microbiome profiles of rumen epithelium and contents of beef cattle with and without liver abscesses. J. Anim. Sci. 98. doi: 10.1093/jas/skaa359
Abe H., Morimatsu M., Nikami H., Miyashige T., Saito M. (1997). Molecular cloning and mRNA expression of the bovine insulin-responsive glucose transporter (GLUT4). J. Anim. Sci. 75, 182–188. doi: 10.2527/1997.751182x
Abitante G., Leme P. R., de Paula Carlis M. S., Ramírez-Zamudio G. D., Gomes B. I. P., de Andrade L. B., et al. (2024). Effects of early weaning on performance and carcass quality of Nellore young bulls. Animals 14, 779. doi: 10.3390/ani14050779
Alforma A. M. P., Pereira G. R., da Rocha M. K., Teixeira O. D. S., de Oliveira M. C. M., Lima J. A., et al. (2023). Influence of weaning management at 30, 75 and 180 days of age on non-esterified fatty acids and reproductive performance in beef cows. J. Anim. Physiol. Anim. Nutr. 107, 407–417. doi: 10.1111/jpn.13736
Ameer F., Scandiuzzi L., Hasnain S., Kalbacher H., Zaidi N. (2014). De novo lipogenesis in health and disease. Metabolism 63, 895–902. doi: 10.1016/j.metabol.2014.04.003
Andrews S. (2010). FastQC: A quality control tool for high throughput sequence data. Available online at: http://www.bioinformatics.babraham.ac.uk/projects/fastqc/ (Accessed on November 02, 2024).
Arthington J. D., Kalmbacher R. S. (2003). Effect of early weaning on the performance of three-year-old, first-calf beef heifers and calves reared in the subtropics. J. Anim. Sci. 81, 1136–1141. doi: 10.2527/2003.8151136x
Ayres H., Ferreira R. M., Torres-Júnior J. R. D. S., Demétrio C. G. B., Sá Filho M. F. D., Gimenes L. U., et al. (2014). Inferences of body energy reserves on conception rate of suckled Zebu beef cows subjected to timed artificial insemination followed by natural mating. Theriogenology 82, 529–536. doi: 10.1016/j.theriogenology.2014.04.026
Baldwin R. L., McLeod K. R., Baumann R. G., Connor E. E. (2006). Influence of carbohydrate infusion on lipogenic enzyme and regulatory protein gene expression in growing beef steers. FASEB J. 20, LB84. doi: 10.1096/fasebj.20.5.LB84-a
Bénédicte R., Mullen A., Moloney F., Larondelle Y., Schneider Y. J., Roche H. M. (2006). Eicosapentaenoic acid and 3, 10 dithia stearic acid inhibit the desaturation of trans-vaccenic acid into cis-9, trans-11-conjugated linoleic acid through different pathways in Caco-2 and T84 cells. Br. J. Nutr. 95, 688–695. doi: 10.1079/BJN20061717
Berton M. P., de Lemos M. V. A., Stafuzza N. B., Simielli Fonseca L. F., Silva D. B. D. S., Peripolli E., et al. (2022). Integration analyses of structural variations and differential gene expression associated with beef fatty acid profile in Nellore cattle. Anim. Genet. 53, 570–582. doi: 10.1111/age.13242
Bionaz M., Chen S., Khan M. J., Loor J. J. (2013). Functional role of PPARs in ruminants: Potential targets for fine-tuning metabolism during growth and lactation. PPAR Res. 2013, 684159. doi: 10.1155/2013/684159
Brazilian Institute of Geography and Statistics (2023). PPM - Municipal Livestock Production, (2023 version). Available online at: https://biblioteca.ibge.gov.br/index.php/biblioteca-catalogo?view=detalhes&id=784 (Accessed on November 14, 2024).
Bunger M., van den Bosch H. M., van der Meijde J., Kersten S., Hooiveld G. J., Muller M. (2007). Genome-wide analysis of PPARα activation in murine small intestine. Physiol. Genomics 30, 192–204. doi: 10.1152/physiolgenomics.00198.2006
Cantalapiedra-Hijar G., Abo-Ismail M., Carstens G. E., Guan L. L., Hegarty R., Kenny D. A., et al. (2018). Biological determinants of between-animal variation in feed efficiency of growing beef cattle. Animal 12, s321–s335. doi: 10.1017/S1751731118001489
Cardozo L. E., Russo P. S., Gomes-Correia B., Araujo-Pereira M., Sepúlveda-Hermosilla G., Maracaja-Coutinho V., et al. (2019). webCEMiTool: co-expression modular analysis made easy. Front. Genet. 10, 146. doi: 10.3389/fgene.2019.00146
Chen E. Y., Tan C. M., Kou Y., Duan Q., Wang Z., Meirelles G. V., et al. (2013). Enrichr: interactive and collaborative HTML5 gene list enrichment analysis tool. BMC Bioinf. 14, 1–14. doi: 10.1186/1471-2105-14-128
Chen S., Zhou Y., Chen Y., Gu J. (2018). fastp: an ultra-fast all-in-one FASTQ preprocessor. Bioinformatics 34, i884–i890. doi: 10.1093/bioinformatics/bty560
Cheng C. W., Beech D. J., Wheatcroft S. B. (2020). Advantages of CEMiTool for gene co-expression analysis of RNA-seq data. Comput. Biol. Med. 125, 103975. doi: 10.1016/j.compbiomed.2020.103975
Cho Y. C., Jefcoate C. R. (2004). PPARγ1 synthesis and adipogenesis in C3H10T1/2 cells depends on S-phase progression, but does not require mitotic clonal expansion. J. Cell. Biochem. 91, 336–353. doi: 10.1002/jcb.10743
Da Silva A. G., Paulino M. F., Detmann E., Fernandes H. J., da Silva Amorim L., Ortega R. E. M., et al. (2017). Energetic-protein supplementation in the last 60 days of gestation improves performance of beef cows grazing tropical pastures. J. Anim. Sci. Biotechnol. 8, 1–9. doi: 10.1186/s40104-017-0209-x
De Jager N., Hudson N. J., Reverter A., Barnard R., Cafe L. M., Greenwood P. L., et al. (2013). Gene expression phenotypes for lipid metabolism and intramuscular fat in skeletal muscle of cattle. J. Anim. Sci. 91, 1112–1128. doi: 10.2527/jas.2012-5409
de Nadai Bonin M., Pedrosa V. B., e Silva S. D. L., Bünger L., Ross D., da Costa Gomes R., et al. (2021). Genetic parameters associated with meat quality of Nellore cattle at different anatomical points of longissimus: Brazilian standards. Meat Sci. 171, 108281. doi: 10.1016/j.meatsci.2020.108281
Dimitriadis G., Mitrou P., Lambadiari V., Maratou E., Raptis S. A. (2011). Insulin effects in muscle and adipose tissue. Diabetes Res. Clin. Pract. 93, S52–S59. doi: 10.1016/S0168-8227(11)70014-6
Dobin A., Davis C. A., Schlesinger F., Drenkow J., Zaleski C., Jha S., et al. (2013). STAR: ultrafast universal RNA-seq aligner. Bioinf. (Oxford England) 29, 15–21. doi: 10.1093/bioinformatics/bts635
Ewels P., Magnusson M., Lundin S., Käller M. (2016). MultiQC: summarize analysis results for multiple tools and samples in a single report. Bioinformatics 32, 3047–3048. doi: 10.1093/bioinformatics/btw354
Fernandes A. C., Reverter A., Keogh K., Alexandre P. A., Afonso J., Palhares J. C. P., et al. (2024). Transcriptional response to an alternative diet on liver, muscle, and rumen of beef cattle. Sci. Rep. 14, 13682. doi: 10.1038/s41598-024-63619-2
Fernyhough M. E., Okine E., Hausman G., Vierck J. L., Dodson M. V. (2007). PPARγ and GLUT-4 expression as developmental regulators/markers for preadipocyte differentiation into an adipocyte. Domest. Anim. Endocrinol. 33, 367–378. doi: 10.1016/j.domaniend.2007.05.001
Fluharty F. L., Loerch S. C., Turner T. B., Moeller S. J., Lowe G. D. (2000). Effects of weaning age and diet on growth and carcass characteristics in steers. J. Anim. Sci. 78, 1759–1767. doi: 10.2527/2000.7871759x
Graugnard D. E., Piantoni P., Bionaz M., Berger L. L., Faulkner D. B., Loor J. J. (2009). Adipogenic and energy metabolism gene networks in longissimus lumborum during rapid post-weaning growth in Angus and Angus × Simmental cattle fed high-starch or low-starch diets. BMC Genomics 10, 1–15. doi: 10.1186/1471-2164-10-142
Kersten S. (2014). Integrated physiology and systems biology of PPARα. Mol. Metab. 3, 354–371. doi: 10.1016/j.molmet.2014.02.002
Kim Y. J., Cho S. Y., Yun C. H., Moon Y. S., Lee T. R., Kim S. H. (2008). Transcriptional activation of Cidec by PPARγ2 in adipocyte. Biochem. Biophys. Res. Commun. 377, 297–302. doi: 10.1016/j.bbrc.2008.09.129
Ladeira M. M., Schoonmaker J. P., Gionbelli M. P., Dias J. C. O., Gionbelli T. R. S., Carvalho J. R. R., et al. (2016). Nutrigenomics and beef quality: A review about lipogenesis. Int. J. Mol. Sci. 17, 918. doi: 10.3390/ijms17060918
Lamb G. C., Dahlen C. R., Larson J. E., Marquezini G., Stevenson J. S. (2010). Control of the estrous cycle to improve fertility for fixed-time artificial insemination in beef cattle: a review. J. Anim. Sci. 88, E181–E192. doi: 10.2527/jas.2009-2349
Lemay D. G., Hwang D. H. (2006). Genome-wide identification of peroxisome proliferator response elements using integrated computational genomics. J. Lipid Res. 47, 1583–1587. doi: 10.1194/jlr.M500504-JLR200
Liao Y., Smyth G. K., Shi W. (2014). featureCounts: an efficient general purpose program for assigning sequence reads to genomic features. Bioinformatics 30, 923–930. doi: 10.1093/bioinformatics/btt656
Lim D., Kim N. K., Park H. S., Lee S. H., Cho Y. M., Oh S. J., et al. (2011). Identification of candidate genes related to bovine marbling using protein-protein interaction networks. Int. J. Biol. Sci. 7, 992. doi: 10.7150/ijbs.7.992
Liu Y., Albrecht E., Dannenberger D., Hammon H. M., Kuehn C., Sauerwein H., et al. (2019). Retinol binding protein 4 abundance in plasma and tissues is related to body fat deposition in cattle. Sci. Rep. 9, 8056. doi: 10.1038/s41598-019-44509-4
Londoño-Gil M., Cardona-Cifuentes D., Rodríguez J. D., Brunes L. C., Magnabosco C. U., Pereira A. S. C., et al. (2022). Heritability and genetic correlations between marbling in longissimus dorsi muscle and conventional economic traits in Nellore beef cattle. Trop. Anim. Health Production 54, 274. doi: 10.1007/s11250-022-03293-6
Maurizi G., Petäistö T., Maurizi A., Della Guardia L. (2018). Key-genes regulating the liposecretion process of mature adipocytes. J. Cell. Physiol. 233, 3784–3793. doi: 10.1002/jcp.v233.5
Meyer D. L., Kerley M. S., Walker E. L., Keisler D. H., Pierce V. L., Schmidt T. B., et al. (2005). Growth rate, body composition, and meat tenderness in early vs. traditionally weaned beef calves. J. Anim. Sci. 83, 2752–2761. doi: 10.2527/2005.83122752x
Moisá S. J., Shike D. W., Faulkner D. B., Meteer W. T., Keisler D., Loor J. J. (2014). Central role of the PPARγ gene network in coordinating beef cattle intramuscular adipogenesis in response to weaning age and nutrition. Gene Regul. Syst. Biol. 8, GRSB–S11782. doi: 10.4137/GRSB.S11782
Moorey S. E., Biase F. H. (2020). Beef heifer fertility: importance of management practices and technological advancements. J. Anim. Sci. Biotechnol. 11, 97. doi: 10.1186/s40104-020-00503-9
Moriel P., Johnson S. E., Vendramini J. M. B., McCann M. A., Gerrard D. E., Mercadante V. R. G., et al. (2014). Effects of calf weaning age and subsequent management systems on growth performance and carcass characteristics of beef steers. J. Anim. Sci. 92, 3598–3609. doi: 10.2527/jas.2014-7751
Myers S. E., Faulkner D. B., Ireland F. A., Berger L. L., Parrett D. F. (1999b). Production systems comparing early weaning to normal weaning with or without creep feeding for beef steers. J. Anim. Sci. 77, 300–310. doi: 10.2527/1999.772300x
Myers S. E., Faulkner D. B., Ireland F. A., Parrett D. F. (1999a). Comparison of three weaning ages on cow-calf performance and steer carcass traits. J. Anim. Sci. 77, 323–329. doi: 10.2527/1999.772323x
Nishimura T. K., da Silva A. G., Abitante G., Dahlen C. R., Goulart R. S., Zamudio G. D. R., et al. (2023). Effects of early weaning on the reproductive performance of suckled Nelore cows in the subsequent breeding season. J. Anim. Sci. 101. doi: 10.1093/jas/skad330
Novais F. J. D., Yu H., Cesar A. S. M., Momen M., Poleti M. D., Petry B., et al. (2022). Multi-omic data integration for the study of production, carcass, and meat quality traits in Nellore cattle. Front. Genet. 13, 948240. doi: 10.3389/fgene.2022.948240
Olefsky J. M., Saltiel A. R. (2000). PPARγ and the treatment of insulin resistance. Trends Endocrinol. Metab. 11, 362–368. doi: 10.1016/S1043-2760(00)00306-4
Pedro A. E., Torrecilhas J. A., Torres R. N. S., Ramírez-Zamudio G. D., Baldassini W. A., Chardulo L. A. L., et al. (2023). Early weaning possibly increases the activity of lipogenic and adipogenic pathways in intramuscular adipose tissue of Nellore calves. Metabolites 13, 1028. doi: 10.3390/metabo13091028
Perduca M., Nicolis S., Mannucci B., Galliano M., Monaco H. L. (2018). Human plasma retinol-binding protein (RBP4) is also a fatty acid-binding protein. Biochim. Biophys. Acta (BBA)-Molecular Cell Biol. Lipids 1863, 458–466. doi: 10.1016/j.bbalip.2018.01.010
Pereira-Flores M. E., Justino F., Rodrigues J. M., Boehringer D., Melo A. A. M., Cursi A. G., et al. (2023). Seasonal climate impact on Brazilian pasture (Brachiaria brizantha cv Marandu): growth rate, CO2 efflux, and irrigation strategies. Theor. Appl. Climatology 151, 651–666. doi: 10.1007/s00704-022-04295-y
Puri V., Konda S., Ranjit S., Aouadi M., Chawla A., Chouinard M., et al. (2007). Fat-specific protein 27, a novel lipid droplet protein that enhances triglyceride storage. J. Biol. Chem. 282, 34213–34218. doi: 10.1074/jbc.M707404200
Rasby R. (2007). Early weaning beef calves. Veterinary Clinics North America: Food Anim. Pract. 23, 29–40. doi: 10.1016/j.cvfa.2007.01.002
Rau A., Maugis-Rabusseau C. (2018). Transformation and model choice for RNA-seq co-expression analysis. Briefings Bioinf. 19, 425–436. doi: 10.1093/bib/bbw128
Raza S. H. A., Shijun L., Khan R., Schreurs N. M., Manzari Z., Abd El-Aziz A. H., et al. (2020). Polymorphism of the PLIN1 gene and its association with body measures and ultrasound carcass traits in Qinchuan beef cattle. Genome 63, 483–492. doi: 10.1139/gen-2019-0184
Reddy K. E., Jeong J., Lee S. D., Baek Y. C., Oh Y., Kim M., et al. (2017). Effect of different early weaning regimens for calves on adipogenic gene expression in Hanwoo loin at the fattening stage. Livestock Sci. 195, 87–98. doi: 10.1016/j.livsci.2016.11.014
Russo P. S., Ferreira G. R., Cardozo L. E., Bürger M. C., Arias-Carrasco R., Maruyama S. R., et al. (2018). CEMiTool: a Bioconductor package for performing comprehensive modular co-expression analyses. BMC Bioinf. 19, 1–13. doi: 10.1186/s12859-018-2053-1
Sampath H., Ntambi J. M. (2006). Stearoyl-coenzyme A desaturase 1, sterol regulatory element binding protein-1c and peroxisome proliferator-activated receptor-α: independent and interactive roles in the regulation of lipid metabolism. Curr. Opin. Clin. Nutr. Metab. Care 9, 84–88. doi: 10.1097/01.mco.0000214564.59815.af
Santana J. M.L., Pereira R. J., Bignardi A. B., Ayres D. R., Menezes G. D. O., Silva L. O. C., et al. (2016). Structure and genetic diversity of Brazilian Zebu cattle breeds assessed by pedigree analysis. Livestock Sci. 187, 6–15. doi: 10.1016/j.livsci.2016.02.002
Scheffler J. M., McCann M. A., Greiner S. P., Jiang H., Hanigan M. D., Bridges G. A., et al. (2014). Early metabolic imprinting events increase marbling scores in fed cattle. J. Anim. Sci. 92, 320–324. doi: 10.2527/jas.2012-6209
Schmidt T. B., Olson K. C. (2007). The effects of nutritional management on carcass merit of beef cattle and on sensory properties of beef. Veterinary Clinics North America: Food Anim. Pract. 23, 151–163. doi: 10.1016/j.cvfa.2006.11.004
Schoonmaker J. P., Cecava M. J., Faulkner D. B., Fluharty F. L., Zerby H. N., Loerch S. C. (2003). Effect of source of energy and rate of growth on performance, carcass characteristics, ruminal fermentation, and serum glucose and insulin of early-weaned steers. J. Anim. Sci. 81, 843–855. doi: 10.2527/2003.814843x
Shijun L., Khan R., Raza S. H. A., Jieyun H., Chugang M., Kaster N., et al. (2020). Function and characterization of the promoter region of perilipin 1 (PLIN1): Roles of E2F1, PLAG1, C/EBPβ, and SMAD3 in bovine adipocytes. Genomics 112, 2400–2409. doi: 10.1016/j.ygeno.2020.01.012
Sithyphone K., Yabe M., Horita H., Hayashi K., Fumita T., Shiotsuka Y., et al. (2011). Comparison of feeding systems: feed cost, palatability and environmental impact among hay-fattened beef, consistent grass-only-fed beef and conventional marbled beef in Wagyu (Japanese Black cattle). Anim. Sci. J. 82, 352–359. doi: 10.1111/j.1740-0929.2010.00836.x
Subramanian A., Tamayo P., Mootha V. K., Mukherjee S., Ebert B. L., Gillette M. A., et al. (2005). Gene set enrichment analysis: a knowledge-based approach for interpreting genome-wide expression profiles. Proc. Natl. Acad. Sci. 102, 15545–15550. doi: 10.1073/pnas.0506580102
Tyagi S., Gupta P., Saini A. S., Kaushal C., Sharma S. (2011). The peroxisome proliferator-activated receptor: A family of nuclear receptors role in various diseases. J. Advanced Pharm. Technol. Res. 2, 236–240. doi: 10.4103/2231-4040.90879
Keywords: adipose tissue, beef cattle, gene co-expression modules, hub genes, peroxisome proliferator-activated receptor gamma
Citation: Russo GH, Tinoco GLB, Vicari MR, Nogueira RS, Melo PLG, Torrecilhas JA, Curi R, Moriel P, Baldassini WA, Chardulo LAL and Pereira GL (2025) Gene co-expression network analysis reveals positive effects of concentrate supplementation on energy metabolism in early-weaned Nellore calves. Front. Anim. Sci. 6:1533043. doi: 10.3389/fanim.2025.1533043
Received: 22 November 2024; Accepted: 27 March 2025;
Published: 28 April 2025.
Edited by:
Gregorio Miguel Ferreira De Camargo, Federal University of Bahia (UFBA), BrazilReviewed by:
Fabiana Cristina Belchior De Sousa, Federal University of Piauí, BrazilImanuel Benu, University of Nusa Cendana, Indonesia
Copyright © 2025 Russo, Tinoco, Vicari, Nogueira, Melo, Torrecilhas, Curi, Moriel, Baldassini, Chardulo and Pereira. This is an open-access article distributed under the terms of the Creative Commons Attribution License (CC BY). The use, distribution or reproduction in other forums is permitted, provided the original author(s) and the copyright owner(s) are credited and that the original publication in this journal is cited, in accordance with accepted academic practice. No use, distribution or reproduction is permitted which does not comply with these terms.
*Correspondence: Gustavo Henrique Russo, Z3VzdGF2by5ydXNzb0B1bmVzcC5icg==; Welder Angelo Baldassini, dy5iYWxkYXNzaW5pQHVuZXNwLmJy; Guilherme Luis Pereira, Z3VpbGhlcm1lLmx1aXNAdW5lc3AuYnI=