- 1Division of Epigenetics, DKFZ-ZMBH Alliance, German Cancer Research Center, Heidelberg, Germany
- 2Merall Bioproducts GmbH, Heidelberg, Germany
- 3Fraunhofer IGB, Stuttgart, Germany
- 4Evonik (SEA) Pte Ltd, Asia Research Hub, Singapore, Singapore
- 5School of Applied Science, Republic Polytechnic, Singapore, Singapore
- 6Creavis, Evonik Operations GmbH, Hanau-Wolfgang, Germany
Biofloc technology is an innovative aquaculture approach that enhances water quality and reduces waste through the development of beneficial microbial communities. Marbled crayfish (Procambarus virginalis) are a newly discovered freshwater crayfish species that are distinguished by asexual reproduction, a genetically homogeneous all-female population structure and considerable robustness. These traits are attractive features for closed-system aquaculture production, which also mitigates the invasive risk associated with the species. Here we describe the establishment and characterization of biofloc culture for marbled crayfish. Juvenile crayfish raised in biofloc systems displayed robust growth with a six-fold weight increase over three months, comparable with other crustaceans under similar conditions. Metagenomic analysis revealed that biofloc communities consisted mostly of beneficial Gammaproteobacteria and Bacillariophyceae, with variations by environmental factors, such as temperature and light exposure. DNA methylation profiling identified systematic changes in response to biofloc environments, highlighting the potential of DNA methylation analysis for environmental biomarker identification. Finally, nutritional analysis showed that biofloc-grown crayfish produced meat with a highly favorable protein-to-fat ratio, while their shells retained considerable amounts of chitin, an important raw material for bioplastics production. Taken together, our study is the first to establish marbled crayfish biofloc culture and to characterize the interaction between the animals and the production system.
Introduction
Aquaculture is essential to meet the growing demand for sustainable protein sources (Naylor et al., 2021). Indoor farming plays a critical role by reducing the dependency on wild fisheries, thereby mitigating overharvesting of oceans while enabling sustainable food production in urban areas. However, advanced aquaculture systems like RAS (Recirculating Aquaculture Systems) require high resource input and produce waste, thus limiting their sustainability (Engle, 2023). Biofloc systems utilize microbial biotechnology to convert toxic nitrogen compounds into microbial protein that serves as supplementary feed for aquatic organisms. As such, they offer an attractive alternative by recycling potentially toxic nutrients through beneficial microbial communities, while reducing water usage, and improving system efficiency (Emerenciano et al., 2022).
Freshwater crayfish offer an interesting option for sustainable aquaculture due to their resilience and high feed conversion efficiency (FAO, 2020). Traditionally farmed in open ponds, they can also be raised in RAS systems, to mitigate environmental damages (FAO, 2020). Incorporating crayfish into aquaculture systems can be an effective strategy for decreasing greenhouse gas emissions, preventing water pollution, and enhancing economic returns compared to traditional farming methods (Naylor et al., 2021; Hu et al., 2022). Marbled crayfish (Procambarus virginalis) are a novel freshwater crayfish species that are characterized by a female-only population structure, asexual reproduction, clonality, rapid growth, high resilience and potential for zero-waste production (Tönges et al., 2021a). They provide high-quality meat and are a valuable source of chitin, a key biopolymer for various applications. In spite of their known invasiveness, they can therefore be considered an attractive aquaculture livestock, when produced in closed systems (Tönges et al., 2021a).
Epigenetic mechanisms are widely considered to function as an interface between animal livestock and their environment (Triantaphyllopoulos et al., 2016; Granada et al., 2017). Epigenetic alterations, such as DNA methylation changes in response to environmental factors like temperature, salinity, and acidification, play a crucial role in enhancing the resilience and adaptability of aquaculture species (Gavery and Roberts, 2017). DNA methylation is an epigenetic modification that regulates gene expression in response to several external cues (Carneiro and Lyko, 2020). In aquaculture, environmental factors may influence DNA methylation patterns (Zhang et al., 2017; Beemelmanns et al., 2021; Rasal et al., 2023). In this context, we have shown previously that marbled crayfish from different locations are characterized by distinct DNA methylation signatures (Tönges et al., 2021b). However, methodological limitations, such as high sequencing costs, have precluded more systematic analyses.
Freshwater crayfish biofloc culture has not been analyzed systematically yet. Using marbled crayfish as a model livestock, we performed growth trials to analyze animal growth performance and biomass yield. Furthermore, we characterized the microbial environment of biofloc cultures by metagenome sequencing. We also characterized the effect of the environment on the epigenome using a novel array-based platform for the analysis of DNA methylation patterns. Finally, we assessed the nutritional quality of biofloc-produced marbled crayfish meat and the chitin yield. Our results further establish marbled crayfish as an aquaculture species for sustainable, zero-waste aquaculture and circular economy approaches.
Materials and methods
Experimental design
This study describes the feasibility of marbled crayfish biofloc culture and uses comparisons with RAS culture for downstream analyses where necessary and appropriate. The RAS system was customized for marbled crayfish, featuring a vertical closed-loop design with continuous mechanical and biological filtration, an average water temperature of 21°C, and a 2.5% daily water exchange from a 300 l tank. Juveniles were housed communally in 45 × 60 cm containers with a 8 cm water column, while adults were kept individually in 15 × 60 cm compartments with a 6 cm water column. Animals were fed ad libitum (Nutramare Aquarium360 Krebs & Shrimps pellets) and maintained under a 12h/12h light-dark cycle.
Biofloc culture
Biofloc systems were maintained with aeration pipes to ensure water circulation and oxygenation. Water parameters, including dissolved oxygen, pH, temperature, and ammonia levels, were monitored daily (see Supplementary Table S1 for details) to maintain optimal conditions for biofloc development (Emerenciano et al., 2022). Total suspended solids (TSS) and settleable solids (SS) were measured weekly to track biofloc density, with target levels at 300 mg/l for TSS and 20 ml/l for SS. Nutrient supplementation was carried out by adding 2–5 g of shrimp feed (Nutramare Aquarium360 Krebs & Shrimps pellets) per 100 l tank, and a carbon source (e.g., sugar) to maintain a carbon-to-nitrogen (C:N) ratio of approximately 12:1. Regular adjustments of feeding rates and aeration were made based on water quality measurements and biofloc density to prevent nutrient imbalances and to maintain system stability (Supplementary Table S1).
Growth trial
100 juvenile marbled crayfish (mean weight=0.31 g, SD=0.13 g) were introduced into the biofloc culture system for each experimental replicate. The animals were housed under controlled aerated conditions, and feeding protocols (C:N ratio 12:1) were adjusted to ensure sufficient nutrition without overfeeding. Individual weights and total biomass were measured monthly using a precision scale. To minimize handling stress, measurements were performed within 15 min, and animals were returned to their systems immediately. The trial was conducted in three independent replicates, each lasting three months. After the trial, the total length and body weight of individual crayfish were recorded. Statistical analysis was used to assess correlations between total length and body weight, using GraphPad Prism9 software.
Metagenomic analysis of biofloc communities
50 ml samples were collected from 7 indoor cultures (water temperature 21°C) and 3 outdoor cultures (water temperature 28°C) into sterile tubes. Samples were immediately centrifuged, supernatants discarded, and pellets subjected to genomic DNA extraction using the Qiagen DNeasy PowerSoil DNA Extraction Kit. Libraries were prepared (LGC Genomics, Germany) for Prokaryota 16S and Eukaryota 18S ribosomal RNA sequencing using specific primers (U341F-U806R for 16S; TAReuk454FWD1-TAReukREV3mod for 18S). Sequencing was performed on the Illumina MiSeq platform (V3 chemistry, 300 bp paired-end reads), targeting 1 million reads per run.
Reads were processed following standard protocols, including adapter trimming, contaminant screening, and short-length read removal using Kraken2 (v2.1.3) (Lu et al., 2022) and the following parameters: k=23, ktrim=r, mink=11, hdist=1, tpe, tbo, ftm=5, qtrim=rl, trimq=20, and minlen=100. The resulting high-quality reads were then classified taxonomically using Kraken2 against preconstructed reference databases, including RefSeq for archaea, bacteria, viruses, fungi, and eukaryotic pathogens (https://benlangmead.github.io/aws-indexes/k2). Contaminant reads, including human and mammalian sequences, were identified using Kraken2 classification against reference databases containing human and UniVec Core sequences. Reads assigned to these taxa were removed before further analysis to minimize non-target contributions to the dataset. Sequencing statistics, including raw, filtered, and classified reads, are detailed in Supplementary Table S2. Follow-up analyses were done using R (v4.2.0). Taxonomic classifications were refined, and only reads classified at the domain or class levels were included. Relative abundances for each taxonomic group were calculated as the proportion of classified reads. The taxonomic composition was grouped into five categories (Archaea, Bacteria, Eukaryota, Virus, and Unclassified).
DNA methylation profiling
Abdominal muscle tissues were collected from crayfish raised in biofloc and RAS systems for DNA methylation analysis. We chose abdominal muscle as the tissue for DNA isolation it can be easily dissected and because DNA methylation patterns are tissue-invariant in marbled crayfish (Gatzmann et al., 2018). Genomic DNA was isolated using the Blood & Cell Culture DNA Kit (Qiagen). Methylation profiling was performed using a custom Illumina Infinium methylation array (Pidsley et al., 2016) with 6,127 methylation probes specifically targeting CpG sites in the marbled crayfish genome that had shown high methylation variation in previous analyses (Venkatesh et al., 2023). The raw methylation array data were processed using SeSAMe (Zhou et al., 2018) with standard settings for custom arrays and an array-specific manifest file.
To enable probe masking on the methylation arrays, 5 published abdominal muscle WGBS datasets from wild populations (Venkatesh et al., 2023) were pooled to increase the coverage. CpGs corresponding to array probes were filtered based on chromosomal coordinates. Weighted methylation ratio averages for each probe CpG were calculated, with coverage serving as the weight. CpGs with a pooled coverage below a threshold of 30 were excluded. Methylation differences between array and WGBS were calculated by subtracting the mean methylation ratio of all thirteen array samples for each probe from the corresponding pooled WGBS methylation ratio. Subsequently, the top 15% of probes with the largest methylation differences, both negative or positive, were excluded, resulting in 4,797 probes for further analysis.
Principal component analysis (PCA) was performed using the prcomp() function in R. Differentially methylated probes between the biofloc and clearwater groups were identified using unpaired t-tests. The top 500 CpGs with the lowest p-values were selected to generate heatmaps visualizing methylation ratios across sample groups. Heatmaps were generated using the heatmap.2 function from the gplots package.
Nutritional analysis of crayfish meat
The protein and fat content of marbled crayfish meat were analyzed by Bilacon GmbH (Berlin, Germany). Protein quantification was performed using the titrimetric method (DIN EN ISO 937:1978), while total fat was measured using the gravimetric method (DIN EN ISO 1444:1996).
Chitin biosynthesis analysis
For mRNA expression analysis, cuticle, gills, and muscle of crayfish were dissected for RNA extraction using TRIzol. cDNA synthesis was performed using the QuantiTec Reverse Transcription Kit (Qiagen, Germany). Quantitative PCR (qPCR) was performed to assess the expression of genes involved in chitin biosynthesis and degradation (primer sequences are provided in Supplementary Table S3) using the MESA Green qPCR Mastermix Plus for SYBR Assay (Eurogentec, Belgium). Gene expression was normalized to TATA-binding protein (TBP) by the 2-ΔΔCt method (Livak and Schmittgen, 2001). Fold-changes were calculated relative to RAS-raised crayfish and plotted using GraphPad Prism9.
Chitin content
The chitin content was determined from the difference between acid detergent fiber (ADF) and acid detergent lignin (ADL). The procedure was carried out according to DIN ISO 13906:2008. The ADF method was carried out first, followed by the ADL method. The chitin content was determined from the difference between acid detergent fiber (ADF) and acid detergent lignin (ADL) (Hahn et al., 2018).
Data access
Sequencing data have been deposited in the NCBI BioSample database under accession numbers SAMN46425236, SAMN46425237, SAMN46425238, SAMN46425239, SAMN46425240, SAMN46425241, SAMN46425242, SAMN46425243, SAMN46425244, and SAMN46425245 (Supplementary Table S2).
Results
To evaluate the capacity of marbled crayfish to grow in biofloc culture, we designed a culture system consisting of 120 x 80 x 20 cm plastic chutes and aeration pipes. Each chute was filled with 100 l of tap water to create a 12 cm water column suitable for benthic organisms. Biofloc growth was initiated by introducing 10 adult crayfish from a RAS culture (Tönges et al., 2021a) into the system. After 5 weeks, we noticed increasing turbidity of the water and confirmed the presence of flocculated organic matter and microbial communities through microscopic inspection. After a biofloc concentration of total suspended solids (TSS) of 30 mg/l and settleable solids (SS) of 20 ml/l was reached, the adult animals were removed from the system and regular testing was initiated to maintain stable water parameters (see Methods for details).
In the next step, 100 juvenile marbled crayfish were placed in a chute and their growth was monitored at monthly intervals. This analysis was performed in three independent replicates. The results showed a >6-fold increase in mean animal weight after 3 months (Figure 1A). To account for the known cannibalism among the animals, we also calculated the total biomass increase over the trial period, which revealed a >3-fold increase after 3 months (Figure 1B). At the end of the trial, many animals had reached a utilizable (see below) pre-adolescent stage with a total length of approximately 5 cm and a weight of approximately 4 g (Figure 1C). The relationship between body weight and total length showed a defined exponential correlation (rho=0.93, Figure 1D), which might aid the implementation of automated monitoring systems that infer animal weight based on measured length.
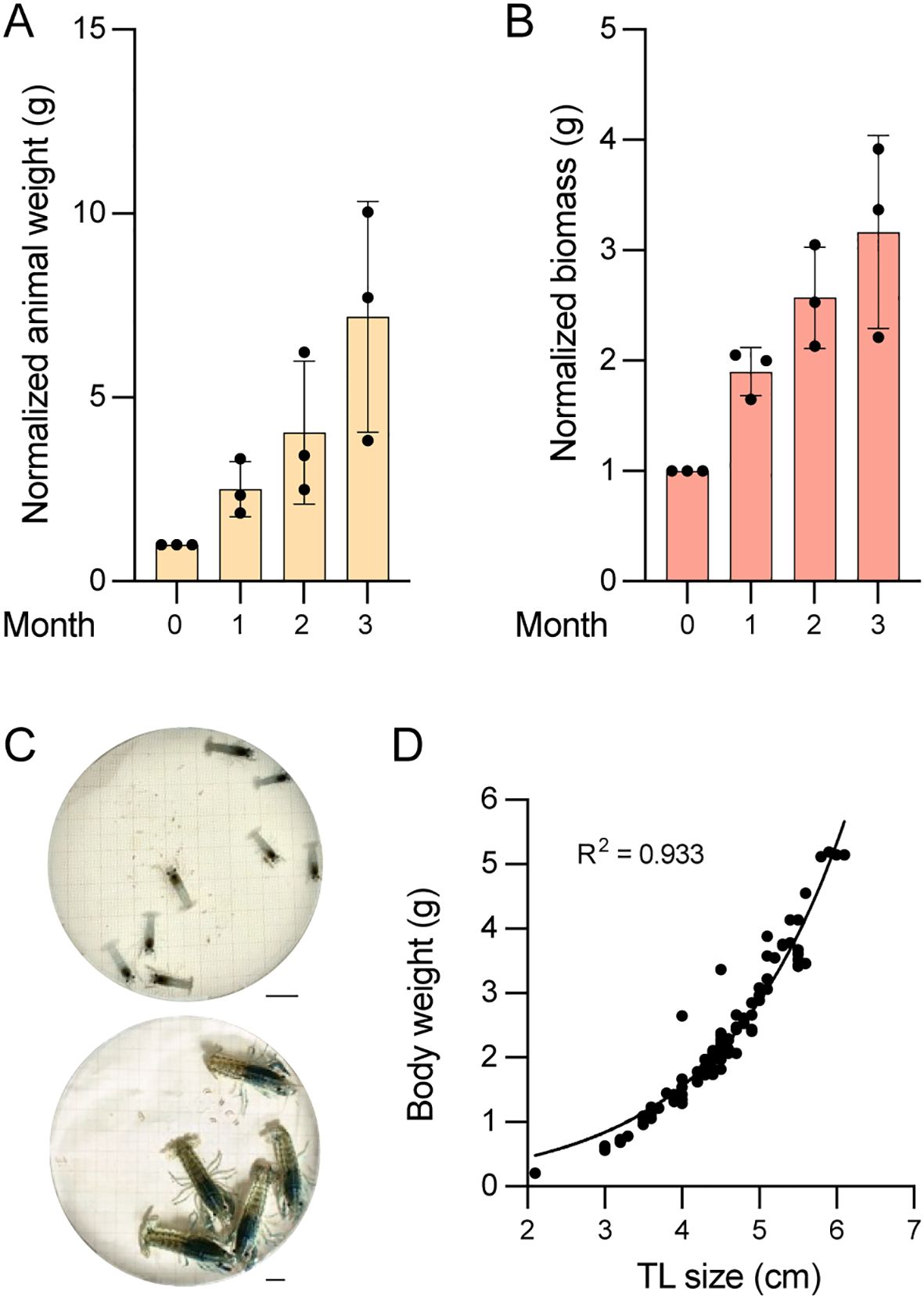
Figure 1. Growth of marbled crayfish in biofloc culture. (A) Normalized mean weight of marbled crayfish during the three-month trial period. (B) Normalized total biomass of marbled crayfish in the biofloc culture system. Error bars indicate standard deviations from three independent replicates. (C) Representative images of juvenile marbled crayfish at the start of the trial (top) and after three months of growth in biofloc culture (bottom). Scale bar: 1 cm. (D) Exponential correlation between total length and body weight of marbled crayfish, suggesting the possibility to use length measurements for automated weight monitoring.
To characterize the composition of our marbled crayfish biofloc, we carried out metagenome analysis. Samples were collected from 7 indoor biofloc tanks with a controlled water temperature of 21°C and 3 outdoor biofloc tanks with an average water temperature of 28°C. DNA was purified and then subjected to massively parallel sequencing of 16S and 18S amplified libraries. Sequence alignment revealed that the vast majority (99.9%) of classifiable sequences was from bacterial and eukaryotic genomes, respectively (Figure 2A). Further analysis revealed a strong prevalence of Gammaproteobacteria and Bacillariophyceae (diatoms) (Figure 2B), which are known as key communities of shrimp biofloc (do Nascimento Ferreira et al., 2024; Rajeev et al., 2024). We also detected additional communities such as Cryptophyceae, which have been described in other biofloc cultures, but typically at lower abundance (Padeniya et al., 2022). We also found a prominent increase of eukaryotic organisms, mainly microalgae such as Bacillariophyceae and Cryptophyceae, and a corresponding reduction in bacterial organisms in biofloc samples from outdoor tanks, consistent with their higher water temperature and sunlight exposure (Figure 2C). Taken together, these findings provide a first characterization of marbled crayfish biofloc communities.
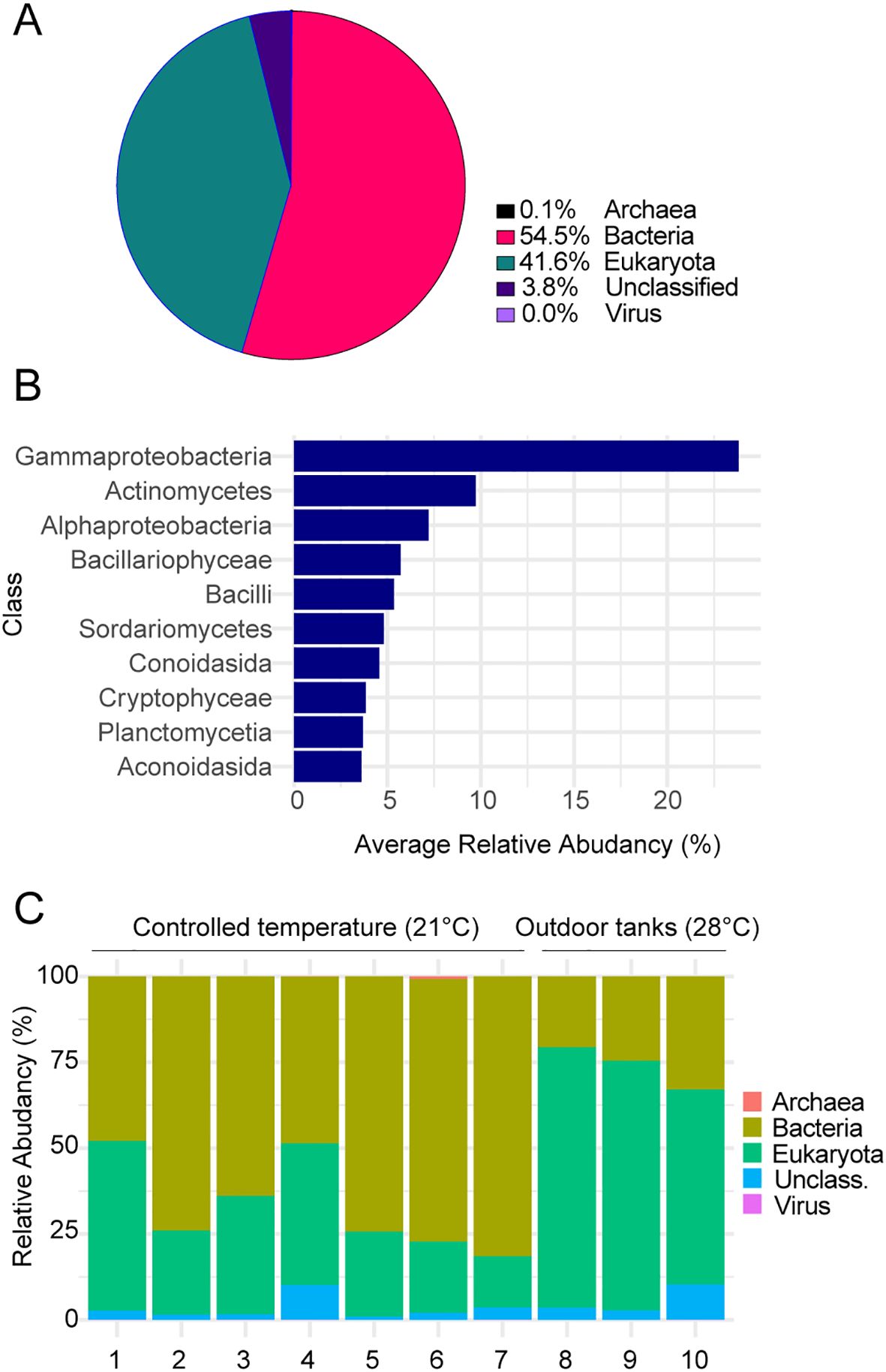
Figure 2. Metagenomic analysis of biofloc communities. (A) Pie chart showing the average taxonomic composition of biofloc samples across all analyzed samples, categorized into five main groups. (B) Bar plot showing the top 10 most abundant classes. (C) Stacked bar chart comparing the relative abundances of major groups (Archaea, Bacteria, Eukaryota, Virus, and Unclassified) between multiple independent samples from controlled-temperature indoor tanks (21°C) and outdoor tanks (28°C).
In a previous study, we have shown that marbled crayfish from different locations are characterized by distinct DNA methylation signatures (Tönges et al., 2021b). We therefore isolated genomic DNA from animals that were raised either in biofloc or in RAS culture to analyze DNA methylation profiles. Methylation patterns were analyzed by array-based profiling of approx. 5,000 variably methylated sites in the marbled crayfish genome (see Material and Methods for details). Principal component analysis of methylation patterns clearly separated the biofloc and RAS samples into two distinct groups (Figure 3A), suggesting the presence of environment-specific methylation signatures. Further data analysis also showed that the 500 most significantly differentially methylated sites were sufficient for separating biofloc from RAS samples in hierarchical clustering analyses (Figure 3B). These findings confirm previous observations about context-dependent methylation changes in marbled crayfish (Tönges et al., 2021b) and expand them to biofloc culture.
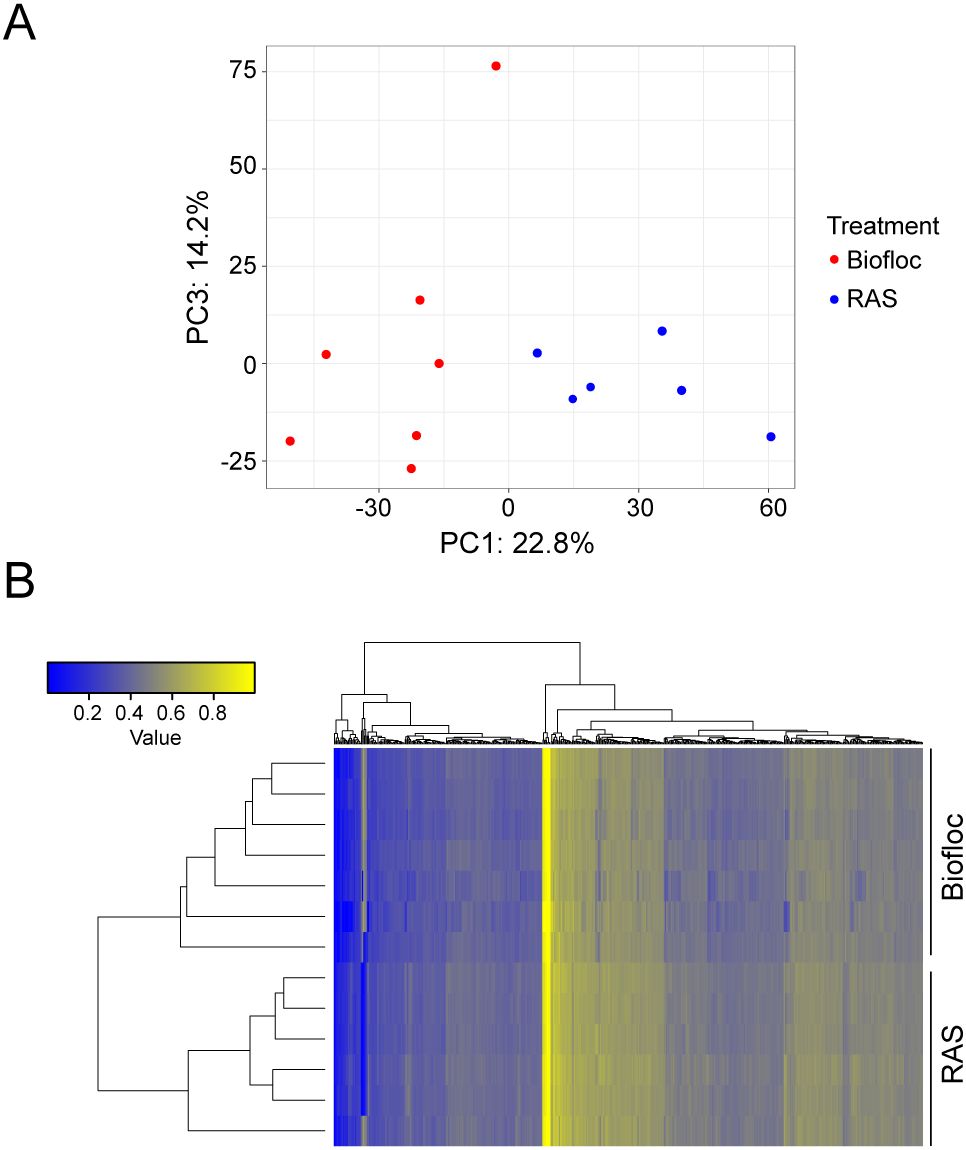
Figure 3. DNA methylation analysis of marbled crayfish in biofloc and RAS systems. (A) Principal component analysis (PCA) based on the methylation levels of 4,474 CpG sites. Biofloc (red) and RAS (blue) samples form distinct clusters, suggesting clear separation of methylation patterns between the two culture systems. (B) Hierarchical clustering of methylation ratios for the top 500 most significantly differentially methylated CpGs. Biofloc and RAS samples again show unambiguous clustering. Colors represent normalized methylation ratios, with yellow indicating high (beta-value = 1) and blue indicating low (beta-value = 0) methylation levels.
In further experiments we analyzed the effect of biofloc culture on marbled crayfish meat yield and quality. To this end, animals (n=37) were randomly collected after 3 months of growth in biofloc culture, and subsequently weighed and processed to obtain abdominal muscles, which were again weighed. The resulting meat pieces ranged broadly from 0.3-0.8 g (Figure 4A), with a mean meat recovery rate of 18% (meat weight relative to total weight), which was largely independent of the animal size (Figure 4B). Nutritional analysis revealed that biofloc-cultivated marbled crayfish produce lean meat, characterized by high protein and low fat levels (Figure 4C), with a correspondingly high protein-to-fat ratio (Figure 4D). These results provide first insight into the nutritional value of marbled crayfish meat, which is characterized by a visual appearance and texture that is similar to shrimp meat (Figure 4E).
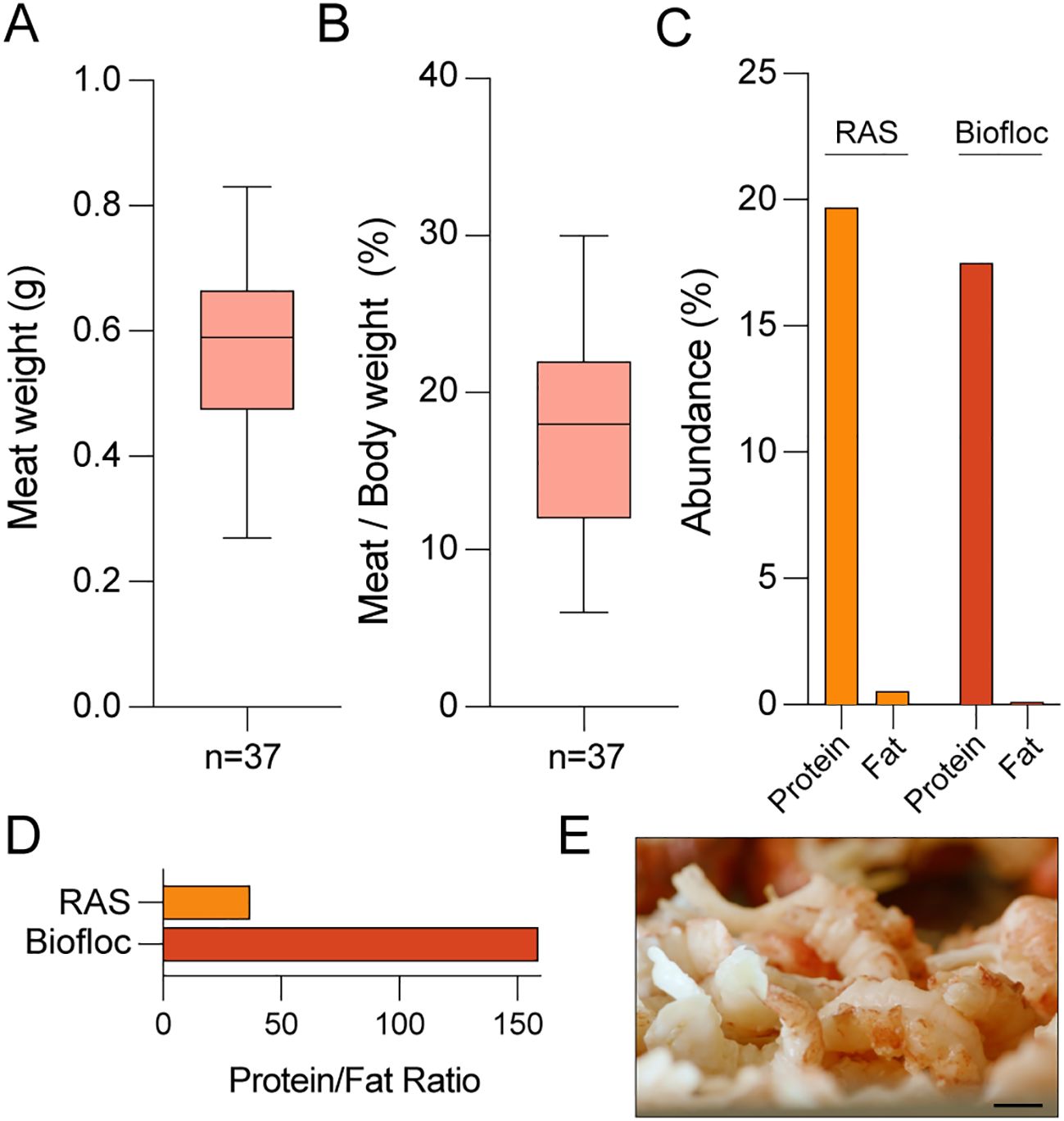
Figure 4. Analysis of biofloc-produced marbled crayfish meat. (A) Boxplot showing individual abdominal muscle weights from biofloc-raised crayfish (n=37). Boxes indicate 25% and 75% percentiles, respectively, whiskers indicate minimum and maximum, and horizontal lines denote medians. (B) Boxplot of meat recovery rates (% of body weight) for crayfish in biofloc culture. Boxes indicate 25% and 75% percentiles, respectively, whiskers indicate minimum and maximum, and horizontal lines denote medians. (C) Comparison of protein and fat content (%) in meat from biofloc and RAS systems, respectively. (D) Protein-to-fat ratio of crayfish meat from biofloc and RAS systems. (E) Photograph of marbled crayfish meat, showing a shrimp-like visual appearance. Scale bar: 1 cm.
As marbled crayfish represent a novel source of chitin (Tönges et al., 2021a), we also analyzed the effect of biofloc culture on the expression of key genes in the chitin metabolic pathway (Figure 5A). To this end, RNA was isolated from 3 different tissues (cuticle, gills, muscle) and gene expression was analyzed by qPCR. The results showed a moderate, but consistent downregulation of many of the analyzed genes in animals from biofloc culture (Figure 5B). This trend applied to both chitin biosynthesis genes and the chitin degrading genes, such as chitinase (CHT) and N-acetylglucosaminidase (NGase, Figure 5B). Our results thus suggest a reduced chitin turnover in biofloc culture, which is likely due to the growth of the animals in a controlled environment. To directly analyze chitin content in marbled crayfish shells, we used fiber analysis (see Material and Methods for details). The results showed only a moderate decrease in chitin content in shells from biofloc animals when compared to RAS controls (Figure 5C).
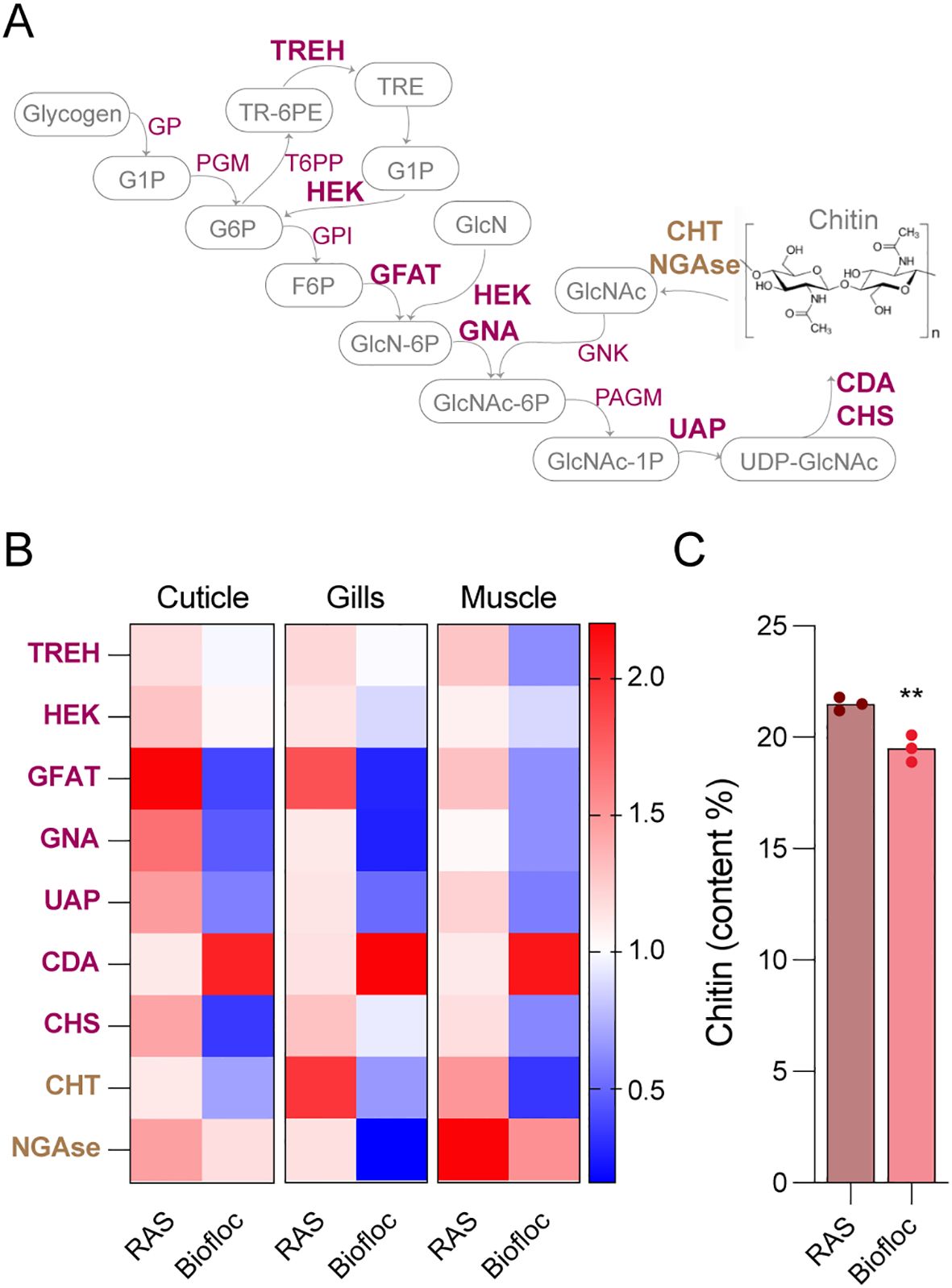
Figure 5. Analysis of marbled crayfish chitin biosynthesis. (A) Schematic representation of the chitin metabolic pathway, showing genes involved in synthesis (pink) and degradation (brown). Genes that were analyzed in (B) are shown in bold. Biosynthesis genes include TREH (Trehalase), GP (Glycogen Phosphorylase), PGM (Phosphoglucomutase), T6PP (Trehalose-6-phosphate Phosphatase), HEK (Hexokinase), GPI (Glucose-6-phosphate Isomerase), GFAT (Glutamine-fructose-6-phosphate Amidotransferase), GNA (Glucosamine-6-phosphate N-acetyltransferase), GNK (Glucosamine Kinase), PAGM (Phosphoacetylglucosamine Mutase), UAP (UDP-N-acetylglucosamine Pyrophosphorylase), and CHS (Chitin Synthase). Degradation genes include CHT (Chitinase) and NGase (N-acetylglucosaminidase). (B) Heatmap comparing relative mRNA expression levels of selected chitin biosynthesis genes in biofloc and RAS culture (n = 4 per group) across three different tissues. Expression values were normalized to TBP (TATA-binding protein), with blue color indicating downregulation and red color indicating upregulation. (C) Chitin content (% of dry shell weight) in crayfish shells from biofloc and RAS cultures. Data are presented as mean ± standard deviation, asterisks indicate statistical significance (**p < 0.01, unpaired t-test).
Discussion
Marbled crayfish represent a promising new livestock for sustainable closed-system aquaculture (Tönges et al., 2021a). Our study establishes the suitability of marbled crayfish for aquaculture in biofloc technology (BFT), which has significant potential for further reducing the economic and ecological costs of marbled crayfish aquaculture. More specifically, our results show robust growth of the animals in a BFT system, with a cultivation time of three months to harvest size. Additionally, we described the microbial composition of the biofloc, which is an important factor for the preservation of water quality and animal welfare. Marbled crayfish raised in the BFT system showed distinct DNA methylation profiles, suggesting epigenetic adaptation to the biofloc environment. The animals maintained high meat quality and chitin yield, which establishes BFT as a viable and resource-efficient alternative for marbled crayfish aquaculture.
Relative to shrimp (L. vannamei) and other crustaceans raised in biofloc under controlled conditions (Aparna et al., 2024; Manan et al., 2024), growth rates of biofloc-raised marbled crayfish appeared comparable. Even though juvenile crayfish are known to show cannibalistic behavior, the total biomass of the culture increased substantially. This may be explained by the increased water turbidity and food availability in biofloc, which reduces hostile encounters between the animals. The natural monosex population structure of marbled crayfish (Scholtz et al., 2003; Gutekunst et al., 2018) is likely to further contribute to suppressing cannibalism and aggressive behavior (Sagi and Aflalo, 2005; Aflalo et al., 2006; Ironside et al., 2018).
Microbial communities play a critical role in maintaining water quality in aquaculture systems. Our metagenomic analysis showed that the biofloc system supported a diverse microbial community dominated by Gammaproteobacteria and Bacillariophyceae. In other BFT systems, such as for shrimp and fish, these groups are well-known for their contributions to nutrient cycling, water quality preservation, and biofloc stability (Lunda et al., 2020). We also observed that the abundance of diatoms (Bacillariophyceae) and algae (Cryptophyceae) was increased in outdoor tanks (28°C). The higher temperature and increased sunlight exposure in outdoor tanks likely accounts for these differences by promoting photosynthetic activity and the proliferation of microalgae, which thrive in warmer conditions and high light intensities. Elevated temperatures can also suppress bacterial growth or decrease bacterial community diversity in the presence of rapidly growing algae (Padeniya et al., 2022).
Changes in DNA methylation are increasingly recognized as biomarkers of environmental adaptation (Norouzitallab et al., 2019; Whelan et al., 2023). Our methylation analysis was carried out with a custom-made marbled crayfish methylation array that is based on the widely used Illumina Infinium Methylation array platform (Pidsley et al., 2016). The results showed distinct methylation profiles between marbled crayfish raised in biofloc and in RAS, consistent with observations in salmon, oysters and fish aquaculture (Zhang et al., 2017; Beemelmanns et al., 2021; Rasal et al., 2023), where environmental factors including diet, temperature, water quality, and microbiome composition influence epigenetic regulation (Gavery and Roberts, 2017; Liu et al., 2022). Our findings therefore contribute to the growing evidence that epigenetic mechanisms play a significant role in the organismal adaptation to aquaculture systems. This role may be particularly important in marbled crayfish, since it could compensate for the absence of genetic diversity in this clonal species. The extended use of array-based methylation analysis should provide further insight into the specific pathways underlying these adaptations and thus contribute to the optimization of marbled crayfish BFT in the future.
Marbled crayfish raised in biofloc produced lean meat, according to our nutritional data. This characteristic as well as its size and texture support marbled crayfish meat production as a sustainable alternative to shrimp fisheries that are struggling to align with the UN Sustainability Developmental goals, like the North Sea shrimp (Steenbergen et al., 2017). Biofloc-based production of marbled crayfish strongly reduces water consumption and energy expenditures, while minimizing toxicity from ammonia accumulation and producing edible microbial aggregates (Rajeev et al., 2023). These characteristics allow a close alignment with sustainability goals, especially for areas with inadequate infrastructure or scarce freshwater supplies (Raza et al., 2024).
Our study also revealed a general downregulation of genes involved in both chitin synthesis and degradation in animals grown in biofloc. This was associated with a modest reduction of total chitin content compared to RAS-grown animals. A possible explanation for this observation is the difference between the feeding strategies of the two systems. While the RAS environment is characterized by excess feed availability, the biofloc environment is a characterized by a more controlled diet to balance microbial growth and nutrient cycling (Raza et al., 2024). Nevertheless, the biofloc cultures supported robust crayfish growth and chitin production, which further illustrates their potential for sustainable aquaculture.
Interestingly, despite reduced chitin turnover, extraction and quantification of chitin from BFT-derived crayfish shells showed only a moderate decrease compared to RAS controls. These findings strongly support the notion that biofloc environments maintain conditions for robust exoskeleton development. From a sustainability perspective, crustacean shells represent an underutilized biomass resource abundant in chitin, a valuable biopolymer with applications in bioplastics manufacturing, biomedicine, and agriculture (Park and Kim, 2010; Younes and Rinaudo, 2015; Tönges et al., 2021a). Efficient chitin extraction from BFT-cultivated crayfish could significantly enhance the environmental and economic value of the marbled crayfish production systems and thus constitutes a key element of a circular economy framework.
Data availability statement
The datasets presented in this study can be found in online repositories. The names of the repository/repositories and accession number(s) can be found in the article/Supplementary Material.
Ethics statement
The animal study was approved by DKFZ Institutional Animal Welfare Committee. The study was conducted in accordance with the local legislation and institutional requirements.
Author contributions
VC: Conceptualization, Formal analysis, Investigation, Methodology, Visualization, Writing – original draft, Writing – review & editing. IS: Investigation, Methodology, Writing – review & editing. JD-L: Formal analysis, Investigation, Writing – review & editing. EB: Formal analysis, Investigation, Writing – review & editing. TH: Formal analysis, Investigation, Writing – review & editing. JH: Investigation, Formal analysis, Writing – review & editing. GV: Methodology, Writing – review & editing. SN: Methodology, Writing – review & editing. SR: Methodology, Writing – review & editing. YN: Investigation, Resources, Writing – review & editing. FB: Resources, Supervision, Writing – review & editing. SZ: Funding acquisition, Supervision, Writing – review & editing. FL: Conceptualization, Funding acquisition, Project administration, Resources, Supervision, Writing – original draft, Writing – review & editing.
Funding
The author(s) declare that financial support was received for the research and/or publication of this article. This work was supported by a grant from the Federal Ministry of Education and Research (BMBF) within the framework “Bioeconomy International” (project ChitoCray, FKZ 031B1277A) to SZ and FL.
Acknowledgments
We thank Paul Schmitzberger, Georg Schmitzberger, and Andreas Werlberger and Steven Fong for stimulating discussions. We also thank Julie Beaucamp and Sina Lyko-Tönges for technical support.
Conflict of interest
Author VC was employed by the company Merall Bioproducts GmbH. Authors SN and SR were employed by the company Evonik SEA Pte Ltd. Author FB was employed by the company Evonik Operations GmbH.
The remaining authors declare that the research was conducted in the absence of any commercial or financial relationships that could be construed as a potential conflict of interest.
Generative AI statement
The author(s) declare that Generative AI was used in the creation of this manuscript. Grammarly and DeepL were used for English language correction without altering the authors’ original scientific content.
Publisher’s note
All claims expressed in this article are solely those of the authors and do not necessarily represent those of their affiliated organizations, or those of the publisher, the editors and the reviewers. Any product that may be evaluated in this article, or claim that may be made by its manufacturer, is not guaranteed or endorsed by the publisher.
Supplementary material
The Supplementary Material for this article can be found online at: https://www.frontiersin.org/articles/10.3389/faquc.2025.1580560/full#supplementary-material
References
Aflalo E. D., Hoang T. T. T., Nguyen V. H., Lam Q., Nguyen D. M., Trinh Q. S., et al. (2006). A novel two-step procedure for mass production of all-male populations of the giant freshwater prawn Macrobrachium rosenbergii. Aquaculture 256, 468–478. doi: 10.1016/j.aquaculture.2006.01.035
Aparna Y., Banafsha S. H., Reddy M. S. (2024). Growth performance of shrimp Litopenaeus vannamei under different carbon: Nitrogen (C/N) ratios of Bioflocs system. Int. J. Fisheries. Aquat. Stud. 12, 06–16. doi: 10.22271/fish.2024.v12.i2a.2905
Beemelmanns A., Ribas L., Anastasiadi D., Moraleda-Prados J., Zanuzzo F. S., Rise M. L., et al. (2021). DNA methylation dynamics in atlantic salmon (Salmo salar) challenged with high temperature and moderate hypoxia. Front. Marine. Sci. 7. doi: 10.3389/fmars.2020.604878
Carneiro V. C., Lyko F. (2020). Rapid epigenetic adaptation in animals and its role in invasiveness. Integr. Comp. Biol. 60, 267–274. doi: 10.1093/icb/icaa023
do Nascimento Ferreira J., Gagliardi T. R., Vieira F. N., Martins C. P., Rosa R. D., Perazzolo L. M. (2024). Assessing the impact of chemoautotrophic and heterotrophic biofloc cultivation systems on the immunity and intestinal bacteriome of shrimp. Aquacult. Int. 32, 4647–4663. doi: 10.1007/s10499-024-01394-7
Emerenciano M. G. C., Rombenso A. N., Vieira F., Martins M. A., Coman G. J., Truong H. H., et al. (2022). Intensification of penaeid shrimp culture: an applied review of advances in production systems, nutrition and breeding. Animals 12, 12030236. doi: 10.3390/ani12030236
Engle C. R. (2023). The economics of recirculating aquaculture systems. J. World Aquacult. Soc. 54, 782–785. doi: 10.1111/jwas.13004
FAO (2020). The state of world fisheries and aquaculture 2020. Sustainabil. Action 2020, 1–244. doi: 10.4060/ca9229en
Gatzmann F., Falckenhayn C., Gutekunstaucieri J., Hanna K., Raddatz G., Carneiro V. C., et al. (2018). The methylome of the marbled crayfish links gene body methylation to stable expression of poorly accessible genes. Epigenetics Chromatin 11, 57. doi: 10.1186/s13072-018-0229-6
Gavery M. R., Roberts S. B. (2017). Epigenetic considerations in aquaculture. PeerJ 5, e4147. doi: 10.7717/peerj.4147
Granada L., Lemos M. F. L., Cabral H. N., Bossier P., Novais S. C. (2017). Epigenetics in aquaculture – the last frontier. Rev. Aquacult. 10, 994–1013. doi: 10.1111/raq.12219
Gutekunst J., Andriantsoa R., Falckenhayn C., Hanna K., Stein W., Rasamy J., et al. (2018). Clonal genome evolution and rapid invasive spread of the marbled crayfish. Nat. Ecol. Evol. 2, 567–573. doi: 10.1038/s41559-018-0467-9
Hahn T., Roth A., Febel E., Fijalkowska M., Schmitt E., Arsiwalla T., et al. (2018). New methods for high-accuracy insect chitin measurement. J. Sci. Food Agric. 98, 5069–5073. doi: 10.1002/jsfa.9044
Hu Z., Gu C., Maucieri C., Shi F., Zhao Y., Feng C., et al. (2022). Crayfish–fish aquaculture ponds exert reduced climatic impacts and higher economic benefits than traditional wheat–rice paddy cultivation. Agriculture 12, 12040515. doi: 10.3390/agriculture12040515
Ironside J. E., Dalgleish S. T., Kelly S. J., Payne W. (2018). Sex or food? Effects of starvation, size and diet on sexual cannibalism in the amphipod crustacean Gammarus zaddachi. Aquat. Ecol. 53, 1–7. doi: 10.1007/s10452-018-9668-1
Liu Z., Zhou T., Gao D. (2022). Genetic and epigenetic regulation of growth, reproduction, disease resistance and stress responses in aquaculture. Front. Genet. 13. doi: 10.3389/fgene.2022.994471
Livak K. J., Schmittgen T. D. (2001). Analysis of relative gene expression data using real-time quantitative PCR and the 2(-Delta Delta C(T)) Method. Methods 25, 402–408. doi: 10.1006/meth.2001.1262
Lu J., Rincon N., Wood D. E., Breitwieser F. P., Pockrandt C., Langmead B., et al. (2022). Metagenome analysis using the Kraken software suite. Nat. Protoc. 17, 2815–2839. doi: 10.1038/s41596-022-00738-y
Lunda R., Roy K., Dvorak P., Kouba A., Mraz J. (2020). Recycling biofloc waste as novel protein source for crayfish with special reference to crayfish nutritional standards and growth trajectory. Sci. Rep. 10, 19607. doi: 10.1038/s41598-020-76692-0
Manan H., Kasan N. A., Ikhwanuddin M., Kamaruzzan A. S., Jalilah M., Fauzan F., et al. (2024). Biofloc technology in improving shellfish aquaculture production – A review. Ann. Anim. Sci. 24, 983–993. doi: 10.2478/aoas-2023-0093
Naylor R. L., Hardy R. W., Buschmann A. H., Bush S. R., Cao L., Klinger D. H., et al. (2021). A 20-year retrospective review of global aquaculture. Nature 591, 551–563. doi: 10.1038/s41586-021-03308-6
Norouzitallab P., Baruah K., Vanrompay D., Bossier P. (2019). Can epigenetics translate environmental cues into phenotypes? Sci. Total. Environ. 647, 1281–1293. doi: 10.1016/j.scitotenv.2018.08.063
Padeniya U., Davis D. A., Wells D. E., Bruce T. J. (2022). Microbial interactions, growth, and health of aquatic species in biofloc systems. Water 14, 4019. doi: 10.3390/w14244019
Park B. K., Kim M.-M. (2010). Applications of chitin and its derivatives in biological medicine. Int. J. Mol. Sci. 11, 5152–5164. doi: 10.3390/ijms11125152
Pidsley R., Zotenko E., Peters T. J., Lawrence M. G., Risbridger G. P., Molloy P., et al. (2016). Critical evaluation of the Illumina MethylationEPIC BeadChip microarray for whole-genome DNA methylation profiling. Genome Biol. 17, 208. doi: 10.1186/s13059-016-1066-1
Rajeev M., Jung I., Kang I., Cho J.-C., Poretsky R. (2024). Genome-centric metagenomics provides insights into the core microbial community and functional profiles of biofloc aquaculture. mSystems 9, e00782–e00724. doi: 10.1128/msystems.00782-24
Rajeev M., Jung I., Lim Y., Kim S., Kang I., Cho J.-C. (2023). Metagenome sequencing and recovery of 444 metagenome-assembled genomes from the biofloc aquaculture system. Sci. Data 10, 707. doi: 10.1038/s41597-023-02622-0
Rasal K. D., Mohapatra S., Kumar P. V., K S. R., Asgolkar P., Acharya A., et al. (2023). DNA methylation profiling of ovarian tissue of climbing perch (Anabas testudienus) in response to monocrotophos exposure. Marine. Biotechnol. 25, 1123–1135. doi: 10.1007/s10126-023-10264-x
Raza B., Zheng Z., Yang W. (2024). A review on biofloc system technology, history, types, and future economical perceptions in aquaculture. Animals 14, 1489. doi: 10.3390/ani14101489
Sagi A., Aflalo E. D. (2005). The androgenic gland and monosex culture of freshwater prawn Macrobrachium rosenbergii (De Man): a biotechnological perspective. Aquacult. Res. 36, 231–237. doi: 10.1111/j.1365-2109.2005.01238.x
Scholtz G., Braband A., Tolley L., Reimann A., Mittmann B., Lukhaup C., et al. (2003). Ecology: Parthenogenesis in an outsider crayfish. Nature 421, 806. doi: 10.1038/421806a
Steenbergen J., Trapman B. K., Steins N. A., Poos J. J., Schmidt J. (2017). The commons tragedy in the North Sea brown shrimp fishery: how horizontal institutional interactions inhibit a self-governance structure. ICES. J. Marine. Sci. 74, 2004–2011. doi: 10.1093/icesjms/fsx053
Tönges S., Masagounder K., Lenich F., Gutekunst J., Tönges M., Lohbeck J., et al. (2021a). Evaluating invasive marbled crayfish as a potential livestock for sustainable aquaculture. Front. Ecol. Evol. 9. doi: 10.3389/fevo.2021.651981
Tönges S., Venkatesh G., Andriantsoa R., Hanna K., Gatzmann F., Raddatz G., et al. (2021b). Location-dependent DNA methylation signatures in a clonal invasive crayfish. Front. Cell Dev. Biol. 9. doi: 10.3389/fcell.2021.794506
Triantaphyllopoulos K. A., Ikonomopoulos I., Bannister A. J. (2016). Epigenetics and inheritance of phenotype variation in livestock. Epigenet. Chromatin. 9, 31. doi: 10.1186/s13072-016-0081-5
Venkatesh G., Tonges S., Hanna K., Ng Y. L., Whelan R., Andriantsoa R., et al. (2023). Context-dependent DNA methylation signatures in animal livestock. Environ. Epigenet. 9, dvad001. doi: 10.1093/eep/dvad001
Whelan R., Tönges S., Böhl F., Lyko F. (2023). Epigenetic biomarkers for animal welfare monitoring. Front. Vet. Sci. 9. doi: 10.3389/fvets.2022.1107843
Younes I., Rinaudo M. (2015). Chitin and chitosan preparation from marine sources. Structure, properties and applications. Marine. Drugs 13, 1133–1174. doi: 10.3390/md13031133
Zhang X., Li Q., Kong L., Yu H. (2017). DNA methylation changes detected by methylation-sensitive amplified polymorphism in the Pacific oyster (Crassostrea gigas) in response to salinity stress. Genes Genomics 39, 1173–1181. doi: 10.1007/s13258-017-0583-y
Keywords: biofloc technology, sustainability, aquaculture, marbled crayfish, epigenetics, chitin
Citation: Coutinho Carneiro V, Schäfer I, Diaz-Larrosa JJ, Böhl E, Hahn T, Hempelmann J, Venkatesh G, Nagarajan S, Roy S, Ng YL, Böhl F, Zibek S and Lyko F (2025) Establishment and characterization of biofloc culture for marbled crayfish. Front. Aquac. 4:1580560. doi: 10.3389/faquc.2025.1580560
Received: 20 February 2025; Accepted: 14 April 2025;
Published: 08 May 2025.
Edited by:
Parisa Norouzitallab, Swedish University of Agricultural Sciences, SwedenReviewed by:
Margarita Smirnov, Ministry of Agriculture and Rural Development (Israel), IsraelJulie Ekasari, IPB University, Indonesia
Pande Gde Sasmita Julyantoro, Udayana University, Indonesia
Copyright © 2025 Coutinho Carneiro, Schäfer, Diaz-Larrosa, Böhl, Hahn, Hempelmann, Venkatesh, Nagarajan, Roy, Ng, Böhl, Zibek and Lyko. This is an open-access article distributed under the terms of the Creative Commons Attribution License (CC BY). The use, distribution or reproduction in other forums is permitted, provided the original author(s) and the copyright owner(s) are credited and that the original publication in this journal is cited, in accordance with accepted academic practice. No use, distribution or reproduction is permitted which does not comply with these terms.
*Correspondence: Vitor Coutinho Carneiro, di5jYXJuZWlyb0BtZXJhbGwtYmlvLmNvbQ==; Frank Lyko, Zi5seWtvQGRrZnouZGU=