- 1Department of Biology, Texas A&M University, College Station, TX, United States
- 2Texas A&M Institute for Neuroscience (TAMIN), Texas A&M University, College Station, TX, United States
- 3Center for Biological Clocks Research, Texas A&M University, College Station, TX, United States
Behavior arises from coordinated brain-wide neural and glial networks, enabling organisms to perceive, interpret, and respond to stimuli. Astrocytes play an important role in shaping behavioral output, yet the underlying molecular mechanisms are not fully understood. Astrocytes respond to intrinsic and extrinsic cues with calcium (Ca2+) fluctuations, which are highly heterogeneous across spatio-temporal scales, contexts, and brain regions. This heterogeneity allows astrocytes to exert dynamic regulatory effects on neuronal function but has made it challenging to understand the precise mechanisms and pathways linking astrocytic Ca2+ to specific behavioral outcomes, and the functional relevance of these signals remains unclear. Here, we review recent literature uncovering roles for astrocytic Ca2+ signaling in a wide array of behaviors, including cognitive, homeostatic, and affective focusing on its physiological roles, and potential pathological implications. We specifically highlight how different types of astrocytic Ca2+ signals are linked to distinct behavioral outcomes and discuss limitations and unanswered questions that remain to be addressed.
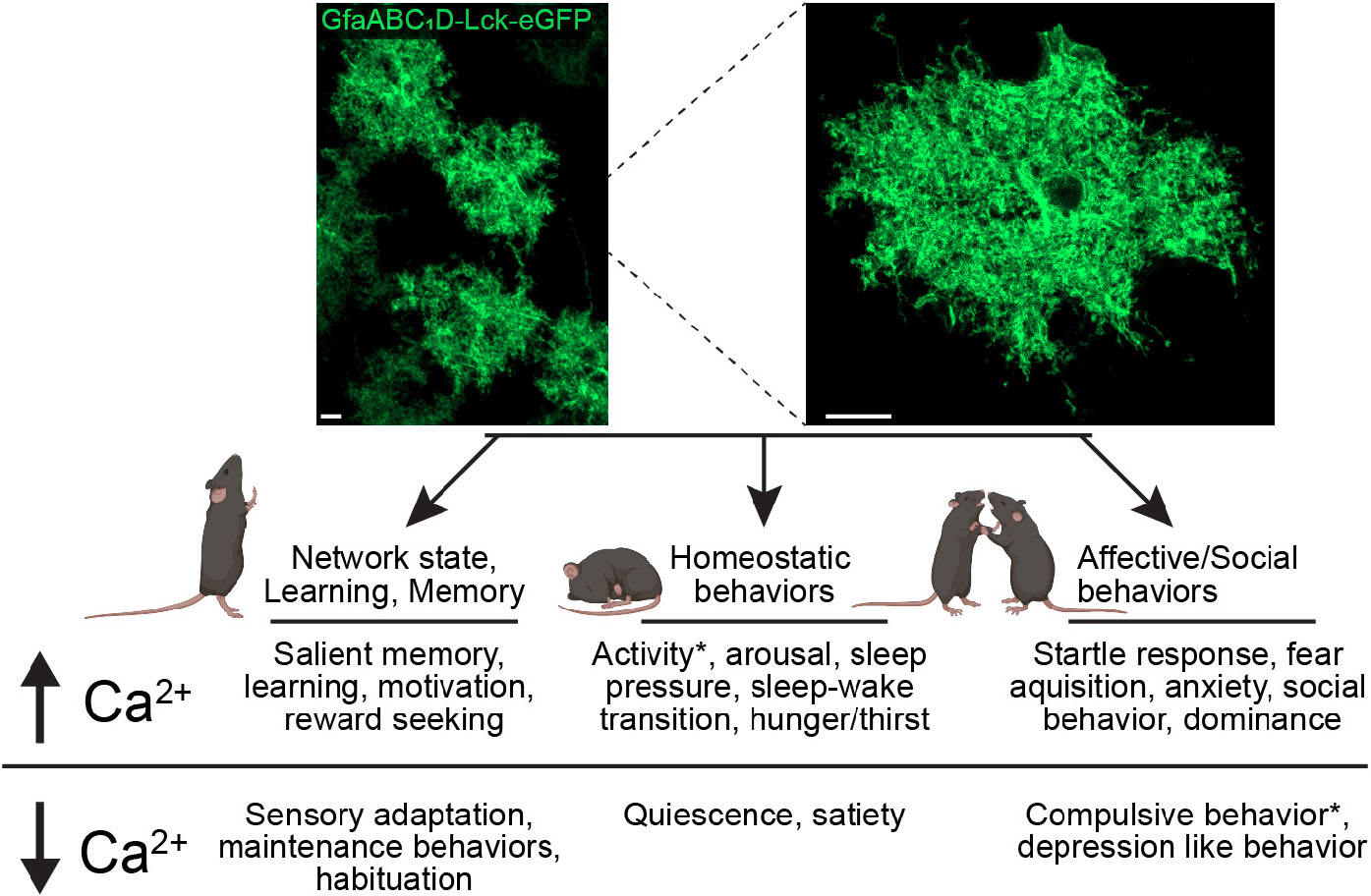
Graphical Abstract. Astrocytic Ca2+ regulates behavioral output. Confocal images of astrocyte network (top left) and single astrocyte (top right) from P14 mouse visual cortex expressing AAV5 GfaABC1D lck-eGFP, scale bar = 10 μm. Diagram (bottom) outlining differential behavioral outputs and corresponding changes in calcium levels. *denotes conditions where different effects were reported depending on brain region.
Introduction
Behavior emerges from the coordinated activity of brain-wide cellular networks including neurons and glia, which modulate an organism’s ability to perceive, interpret, and appropriately respond to environmental or intrinsic stimuli (McCormick et al., 2020; Wu Y. et al., 2024). It is now well established that astrocytes, a major type of glia, play key roles in shaping behavioral responses by regulating multiple aspects of neuronal function such as synaptic formation and function (Farhy-Tselnicker and Allen, 2018; Tan et al., 2021), plasticity (Ota et al., 2013; Wang et al., 2022), and circuit dynamics (Hirrlinger and Nimmerjahn, 2022; Oliveira and Araque, 2022). Tiling every region of the brain where they closely associate with the vasculature as well as hundreds of thousands of synapses in rodents (Bushong et al., 2002; Sofroniew, 2021; Hösli et al., 2022; Lorin et al., 2024) [and millions in humans (Oberheim et al., 2009; Oberheim et al., 2012)], astrocytes monitor the brain’s microenvironment and tune the responses of neurons and other glial cells to network activity and metabolic states (Jha et al., 2019; Nutma et al., 2020; Rueda-Carrasco et al., 2021; Xie et al., 2022; Molina-Gonzalez et al., 2023; Hu et al., 2024; Imrie et al., 2024). However, the specific cellular mechanisms linking astrocytic function and behavioral outputs are not fully understood.
Astrocytic signaling is primarily mediated by changes in calcium (Ca2+) levels [reviewed in Khakh and Deneen (2019), Goenaga et al. (2023), Ahrens et al. (2024), and Bai et al. (2024)] which are highly heterogeneous across multiple spatial and temporal scales (Srinivasan et al., 2015; Bindocci et al., 2017; Semyanov, 2019).
Microdomain Ca2+ transients occur within the fine astrocytic processes that contact synapses, allowing modulation of synaptic activity by influencing gliotransmitter release, neurotransmitter uptake and extracellular ion homeostasis with precise spatio-temporal control (Shigetomi et al., 2010; Shigetomi et al., 2013a; Agarwal et al., 2017; Ahmadpour et al., 2021; Lia et al., 2021; Denizot et al., 2022) (Figure 1). Astrocytes can also generate larger-scale somatic Ca2+ changes which are primarily mediated by the release of Ca2+ stored in the endoplasmic reticulum (ER) (Srinivasan et al., 2015; Stobart et al., 2018; Sherwood et al., 2021). These fluctuations can occur spontaneously as well as through extrinsic signaling via G protein coupled receptor (GPCR)-mediated inositol trisphosphate (IP3) pathway, which in astrocytes is predominantly mediated via the inositol trisphosphate 3 receptor type 2 (IP3R2). It was shown that unlike in neurons, activating both modulatory (Gαq) and inhibitory (Gαi)-coupled GPCRs in astrocytes can elicit stored Ca2+ release, demonstrating the complex nature of astrocytic Ca2+ dynamics (Kofuji and Araque, 2021b; Vaidyanathan et al., 2021; Denizot et al., 2022). Somatic Ca2+ fluctuations can further propagate as intracellular Ca2+ “waves” or “surges,” which can travel within the cell body and processes and extend to other astrocytes via gap junctions, thus facilitating communication within glial networks and coordinating activity across brain regions (Scemes and Giaume, 2006; Fujii et al., 2017).
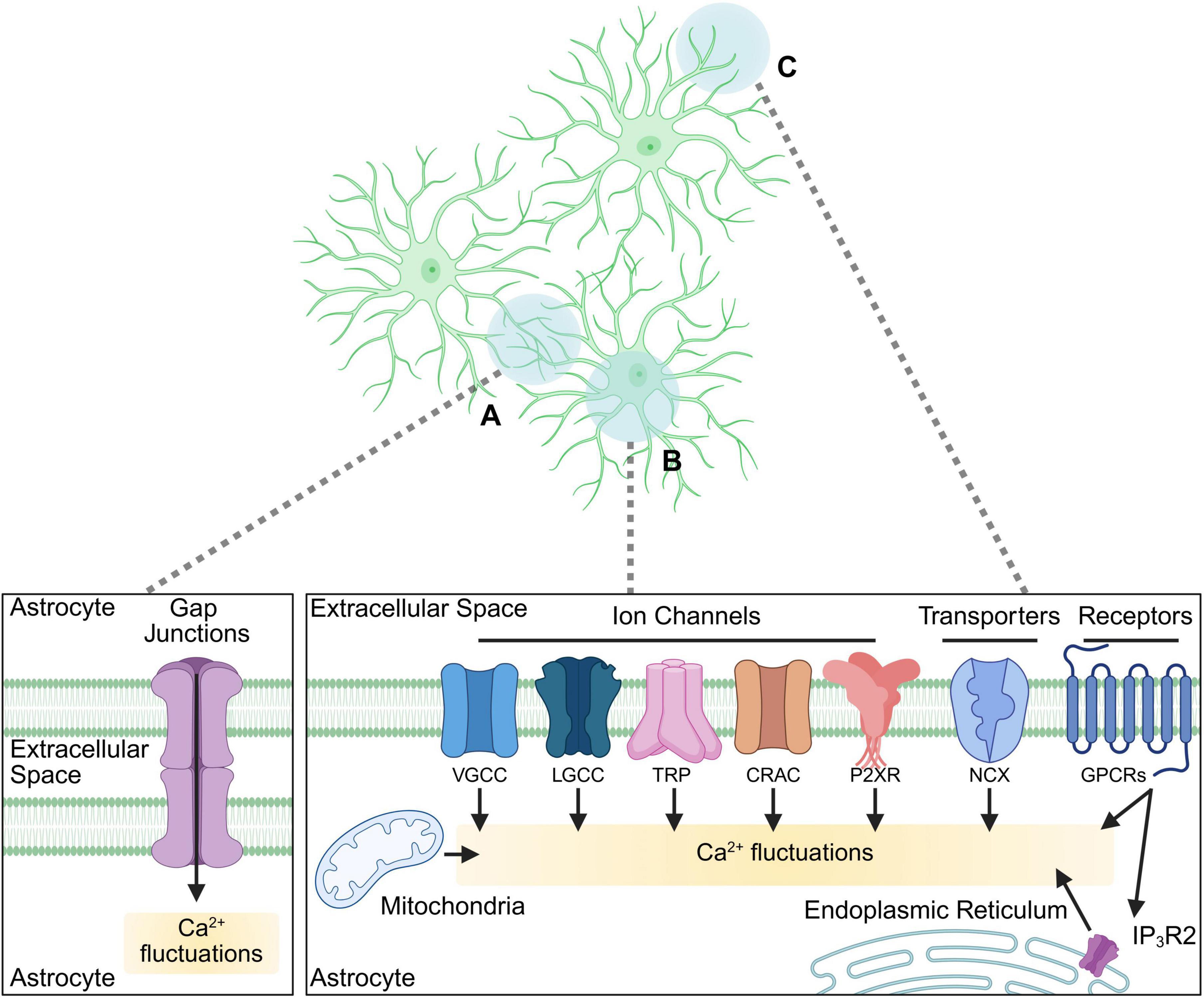
Figure 1. Cellular pathways involved in astrocytic Ca2+ signaling. A diagram of astrocytic cellular compartments (top panel) and the signaling pathways involved in Ca2+ dynamics (bottom panels) within them. (A) Astrocytic Ca2+ signals via gap junctions facilitate astrocytic network communication. (B, C) Diverse pathways mediated via receptors, transporters, channels, and cellular organelles activating Ca2+ fluctuations in soma and major processes (B), as well as in fine processes (C) such as those contacting synapses (synapses not depicted). VGCC, voltage-gated Ca2+ channels; LGCC, ligand-gated Ca2+ channels (including ionotropic receptors such as NMDAR); TRP, transient receptor potential channel; CRAC, Ca2+ release-activated Ca2+ channel; P2XR, purinergic receptor P2X; NCX, Na+-Ca2+ exchanger; GPCR, G protein coupled receptor; IP3R2, inositol trisphosphate receptor type 2. Biorender.
The sources of astrocytic cytosolic Ca2+ depend on the type of signaling initiated in the cell (Figure 1). While the ER serves as a primary reservoir for somatic fluctuations (Okubo, 2020) in response to GPCR activation, it is also shown to contribute to microdomain signals (Lia et al., 2021; Denizot et al., 2022). Further, the mitochondria, which interact with the ER, play a dual role by both buffering intracellular Ca2+ levels and regulating its release, thereby shaping the amplitude and duration of Ca2+ transients (Agarwal et al., 2017; MacVicar and Ko, 2017; Serrat et al., 2022). Extracellular Ca2+ influx also contributes significantly to astrocytic activity, occurring through multiple pathways including transient receptor potential (TRP) channels (Shigetomi et al., 2013b; Verkhratsky et al., 2014; Bosson et al., 2017), voltage-gated Ca2+ channels (VGCCs) (Cheli et al., 2016; Zamora et al., 2020), purinergic P2X receptors (Ahmadpour et al., 2021), transporters (such as Na+-Ca2+ exchanger, NCX) (Rose et al., 2020), store-operated Ca2+ entry (SOCE) mechanisms (Toth et al., 2019) (such as Ca2+ Release-Activated Ca2+ (CRAC) channels), and activation of Ca2+ permeable ionotropic receptors (or ligand-gated channels, LGCCs), such as N-methyl-D-aspartate receptors (NMDARs) which, in addition to neurons, are also expressed by astrocytes [reviewed in Imrie et al. (2024)]. How these signals intersect to produce cellular responses is not well understood, but the dynamic relationship between these sources and the cellular pathways they activate allows astrocytes to integrate diverse signals and regulate neuronal activity with high versatility.
The development of tools allowing for the visualization, quantification, and manipulation of astrocytic Ca2+ signals has been central to understanding their physiological relevance. Visualization of astrocytic Ca2+ signals is largely executed through imaging of fluorescent dyes or genetically encoded Ca2+ indicators (GECIs) such as green or red fluorescent protein conjugated calmodulin-M13 peptides (G/RCaMP) (Lohr et al., 2021). These indicators are used across multiple experimental models including cultured cells (Ryu et al., 2024), ex vivo slices (Srinivasan et al., 2015), or in vivo in head-fixed or freely behaving animals (Qin et al., 2020; Gau et al., 2024). Additionally, astrocytic Ca2+ signals can be manipulated to assess upstream effectors and downstream consequences. These include reductions via chelators (Sasaki et al., 2014), genetic removal of IP3R2 (Srinivasan et al., 2015), or via Ca2+ extrusion pumps such as CalEx (Yu et al., 2018), or activation through stimulation of GPCRs (Kofuji and Araque, 2021b), including chemogenetic stimulation of designer receptors exclusively activated by designer drugs (DREADDS) (Roth, 2016; Lee et al., 2023). Several analysis tools to decode astrocytic Ca2+ signals have been utilized including GECIquant (Sharmila, 2019) and Astrocyte Quantitative Analysis (AQuA) (Wang et al., 2019), providing high resolution signal detection and quantification. While these methods provide robust representations of Ca2+ events, their physiological relevance should be carefully considered, as Ca2+ buffering by indicators, insufficient labeling (such as lack of signal in the fine processes), or off target effects (such as gliosis due to overexpression of modified fluorescent proteins) are a possibility (Semyanov et al., 2020). Furthermore, when manipulating astrocytic Ca2+ signaling, discerning whether the effects accurately represent endogenous physiological activity is a challenge. For example, recent work using in vivo imaging of mouse cortex has shown that activation of Gq-DREADDs strongly yet transiently increases astrocytic Ca2+, followed by persistent suppression of Ca2+ signals (Vaidyanathan et al., 2021). For detailed reviews on this topic see: (Khakh and McCarthy, 2015; Shigetomi et al., 2016; Semyanov et al., 2020; Bai et al., 2024).
A large body of work in recent years using primarily mammalian models has strongly implicated astrocytic Ca2+ signaling in a wide range of central nervous system functions including behavioral output [for further reading see (Guerra-Gomes et al., 2018; Kofuji and Araque, 2021a; Lyon and Allen, 2022)], while disruptions in astrocytic Ca2+ homeostasis have been observed in neurological and psychiatric disorders in both rodent models and human tissue (Shah et al., 2022; Sobolczyk and Boczek, 2022; González-Arias et al., 2023), underscoring the importance of understanding these processes in both physiological and pathological contexts. Despite these prominent findings, the functional relevance of astrocytic Ca2+ signaling has been a controversial topic (Nizar et al., 2013; Takata et al., 2013; Bonder and McCarthy, 2014; Jego et al., 2014; Petravicz et al., 2014), and a comprehensive understanding of the mechanisms by which astrocytic Ca2+ signaling modulates behavior is lacking. In this review, we highlight recent advances in our understanding of how astrocytic Ca2+ signaling contributes to behavioral output focusing on findings which characterize astrocytic Ca2+ dynamics underlying various cognitive and emotional processes and neural circuit function. By integrating results from cellular, systems, and behavioral studies, we provide a comprehensive perspective on the role of astrocytic Ca2+ signaling in brain function and subsequent behavioral responses.
Astrocytic Ca2+ signaling regulates cortical network states, learning, and memory
Cortical network states
Cortical network states define how populations of brain cells interact and process information (Buonomano and Maass, 2009; Wu M. et al., 2024). In this context, “states” refers to distinct activity patterns which facilitate different kinds of behaviors and can be measured by recording local field potentials to quantify oscillatory dynamics (Colgin, 2011; Liu et al., 2022; Chen et al., 2024). For instance, deep sleep is characterized by delta oscillations at 0.5–4 Hz (Kim et al., 2019), while sensory processing, attention, and working memory are characterized by gamma oscillations at 30–100 Hz (Buzsáki and Wang, 2012). Astrocytic Ca2+ signaling diversely regulates cortical state maintenance and transitions, underlying multiple modes of behavioral output. Studies pairing in vivo Ca2+ imaging using genetically encoded Ca2+ indicators (GECIs) with behavioral tracking or electrophysiology show that cortical astrocyte Ca2+ fluctuations are critical for cortical network state switching, which drives initiation and cessation of behaviors including sleep, arousal, feeding, and exploration (Poskanzer and Yuste, 2016; Reitman et al., 2023; Gau et al., 2024). It was shown that Ca2+ signals within the fine astrocytic processes underlie a switch to a slow-oscillation dominated state which is critical for behavioral regulation in both quiescent and active states (Anand et al., 2024), and which is associated with enhanced extracellular glutamate levels (Poskanzer and Yuste, 2016). Interestingly, astrocytic Ca2+ signals appear to be suppressed during habitual or familiar behaviors, but increase during unexpected behaviors, such as exploration of novel stimuli. Notably, these astrocytic Ca2+ responses decline with repeated exposure, suggesting an adaptive mechanism for encoding contextual behavioral salience changes (Gau et al., 2024).
State dependent cortical astrocyte Ca2+ signals are encoded by the distinct actions of specific neurotransmitters and neuromodulators. Studies leveraging in vivo astrocytic Ca2+ imaging in combination with neurotransmitter uncaging show that brief neurotransmitter input leads to long lasting network-wide astrocyte Ca2+ changes, which may serve as a mechanism for prolonged neuronal network activity integration. Glutamate and GABA uncaging both induced prolonged and spatially extensive astrocyte Ca2+ activity, with glutamate preferentially increasing propagative Ca2+ waves, which appear to modulate information flow within astrocytic networks (Cahill et al., 2024). Importantly, in these studies, astrocytic Ca2+ responses were context dependent, with baseline propagative activity inversely correlated with responsiveness to neurotransmitter input. Additionally, norepinephrine (NE) is shown to drive astrocyte Ca2+ transients involved in cortical synchronization, important for transitions from quiescent to active states and behavioral timing (Reitman et al., 2023; Gau et al., 2024), while acetylcholine (ACh) modulates cortical astrocyte Ca2+ transient amplitude during novel experience (Gau et al., 2024).
Sensory perception
Network states allow organisms to make sense of environmental stimuli in a contextually relevant manner, supporting a role for astrocytic Ca2+ activity in sensory perception and processing. Indeed, recent work using Ca2+ imaging and electrocorticography (ECoG) in mice shows that astrocytes in the primary somatosensory cortex exhibit stimulus dependent Ca2+ elevations in response to sensory stimulation, which temporally correlate with neural network activity, specifically gamma oscillations, linked to sensory processing and cortical excitability. In mice lacking the ER receptor IP3R2 and subsequent store-released Ca2+ signaling in astrocytes, gamma oscillation steady state is increased and its temporal decline during sensory stimulation is diminished. Further, manipulation of astrocytic Ca2+ levels using a chemogenetic approach with Gαq-coupled designer receptors exclusively activated by designer drugs (Gq-DREADDs) reduced cortical gamma frequency responses to sensory stimulation, suggesting that astrocytic Ca2+ plays an important role in modulating sensory-evoked gamma activity by regulating its upper limits (Lines et al., 2020). Notably, since DREADD mediated activation of astrocytic Gαq-coupled pathways is pancellular, it may mask the contributions of compartmentalized astrocytic Ca2+ signals in this context. Astrocytic microdomain Ca2+ transients are also implied in cortical responses to sensory stimulation. Virally mediated knock-down of astrocytic NMDARs, activation of VGCCs, and metabotropic signaling caused neural network desynchronization and impaired adaptation to whisker stimulation in the mouse barrel cortex (Ahmadpour et al., 2024).
Learning
Dynamic modulation of neural responses to relevant stimuli underlies the acquisition of behavior, which can be innate or learned (Shahaf and Marom, 2001; Aizenberg et al., 2015; Inácio et al., 2025). Encoding of reward drives learning through changes in synaptic plasticity brought about by signaling molecules that transmit information regarding expected and elicited outcomes (Ding et al., 2022). Recent evidence provides a framework for the role of astrocytic Ca2+ signaling in these processes. Using the AstroLight tool, which employs a light-sensitive transcriptional switch that only activates gene expression in the presence of high intracellular Ca2+ and blue light application, it was shown that astrocytes in the nucleus accumbens (NAc) form ensembles that mediate cue-motivated behaviors in mice. Though AstroLight is a powerful tool for identifying contextually respondent astrocytes, the manipulation of broad populations of astrocytes using opsins may lead to cellular activity changes that deviate from physiological norms. Fiber photometry and Ca2+ imaging showed progressive recruitment of astrocytic Ca2+ activity in the NAc during cue–reward learning. Optogenetic and chemogenetic modulation of these ensembles is sufficient to modulate behavior, demonstrating that astrocytes integrate motivational information through Ca2+ signaling to contribute to decision making processes (Serra et al., 2025). These findings are consistent with evidence in the globus pallidus externus, where astrocytic Ca2+ is shown to gradually reduce as habit formation progresses (Kang et al., 2023), while in the hippocampus, CA1 astrocytes chronically imaged in vivo were shown to gradually “ramp up” Ca2+ activity during reward seeking in a previously learned location (Doron et al., 2022). In the NAc these effects are diversely mediated by unique glutamatergic circuits, displaying input region-specific astrocytic Ca2+ responses: inputs from the medial prefrontal cortex (mPFC) trigger high levels of Ca2+ activity in astrocytes of the NAc core and shell, amygdala inputs enhance astrocytic Ca2+ signals in the dorsal NAc, while ventral hippocampus inputs broadly activate astrocytic networks (Serra et al., 2022).
Memory
Memory is essential for learning, and the neural and glial mechanisms underlying these processes are closely interrelated, mutually driving behavioral output shaped by experience. Accumulated evidence suggests an important role for astrocytic Ca2+ in multiple aspects of memory function including formation, consolidation, and retrieval (Huang et al., 2020; Escalada et al., 2024). In vivo Ca2+ imaging studies in mice show that hippocampal CA1 astrocytes integrate information from salient past events such that Ca2+ signals from distal astrocytic processes are followed by Ca2+ changes in the soma, generating specific patterns of networked Ca2+ activity dependent on arousal state and past Ca2+ signaling events (Rupprecht et al., 2024). This also has important implications for neuronal plasticity necessary for memory formation and allocation, which are enhanced by optogenetic or chemogenetic Gq-DREADD induced astrocytic Ca2+ changes which facilitate NMDAR-dependent long-term potentiation in CA1 (Adamsky et al., 2018; Suthard et al., 2023a). These effects may in part be mediated by the activity of astrocytic α4-nAChRs, which drive Ca2+ transients that regulate the co-agonist supply for NMDARs, strengthening temporal association memory, an effect that was diminished by attenuation of astrocytic Ca2+ (Ma et al., 2023). Recent work also reveals an important role for Gαi-GPCR-mediated astrocytic Ca2+ changes in CA1, which impair remote but not recent memory when chemogenetically modulated during learning. This manipulation also affected neuronal activity in the anterior cingulate cortex through the disruption of CA3 to CA1 communication, indicating an astrocytic role in circuit-specific regulation of memory (Kol et al., 2020).
This was also observed in the basolateral amygdala, where astrocytic Ca2+ extrusion by virally mediated expression of the CalEx pump impaired context dependent memory recall (Sun et al., 2024). The molecular mechanisms underlying astrocytic Ca2+ signaling in memory are still largely unknown, but work leveraging electrophysiological recording in mouse brain slices has uncovered that store-operated Ca2+ release-activated Ca2+ (CRAC) channels comprised of Orai1 and STIM1 are necessary for the development of sustained and oscillatory Ca2+ signals in response to GPCR stimulation, and subsequent release of ATP in CA1 (Toth et al., 2019). Additionally, rescuing STIM1 expression enhanced long-term plasticity in Alzheimer’s disease (AD) models in female mice, which display decreased astrocytic Ca2+ activity associated with store-released Ca2+ dysfunction (Lia et al., 2023).
To summarize, astrocytic Ca2+ signaling is emerging as a central regulator in cortical network state maintenance, sensory perception, learning, and memory, which are all critical components in the acquisition and elicitation of behavioral output. Through neurotransmitter-specific responses and regionally distinct signaling mechanisms, astrocytes adaptively encode environmental stimuli and behavioral salience via dynamic changes in Ca2+ fluctuations, reinforcing their importance in experience-dependent plasticity. Though recent work has made great progress in identifying the specific effects of upregulation or abrogation of astrocytic Ca2+ signaling in these functions, studies investigating the specific molecular mechanisms and subcellular pathways that are activated in response to astrocytic Ca2+ manipulation are lacking. Expanded investigations focusing on the interactions between CRAC mediated Ca2+ entry and other Ca2+ sources in vivo will be important for identifying how these pathways contribute to behavior. Ultimately, studies describing the context, temporal, and circuit dependent mechanisms by which astrocytic Ca2+ signaling mediates cortical state, learning, and memory will be critical for determining how astrocytes regulate behavioral output at its earliest stages.
Astrocyte Ca2+ signaling regulates homeostatic behaviors
Homeostatic behaviors such as sleep-wake cycles and food intake are essential for survival, allowing organisms to maintain stable internal conditions despite changes in their environment. These behaviors originate when conditions deviating from physiological ranges are detected and integrated by both neuronal and glial networks to generate appropriate responses (Simard and Nedergaard, 2004; Lam, 2010; Rosenberg and Rao, 2021; Ahn et al., 2022). Through their extensive interactions with neuronal synapses and CNS vasculature, astrocytic Ca2+ changes tune these behavioral outputs in a contextually relevant manner (Parpura and Verkhratsky, 2012; Murphy-Royal et al., 2017; Lee et al., 2021; Tewari et al., 2024).
Circadian rhythmicity
Circadian rhythmicity is fundamental to the maintenance of homeostatic functions in most animals (de Assis and Oster, 2021; Mortimer et al., 2025). Astrocytes express genes encoding the molecular clock and show robust circadian rhythmicity (Barca Mayo et al., 2019; Womac et al., 2009; Brancaccio et al., 2017; Ruben and Hogenesch, 2017; Whalley, 2017; McCauley et al., 2020; Coomans et al., 2021; Hastings et al., 2023; Ryu et al., 2024). In mammals, core circadian output is generated within the suprachiasmatic nucleus (SCN) of the hypothalamus, where astrocytic Ca2+ activity is anti-phase to neuronal Ca2+ activity, as shown by long term imaging of ex vivo organotypic mouse brain slices expressing virally delivered GECIs. This anti-phasic oscillatory pattern was observed in both the soma and microdomains, with the latter showing particularly robust signals in the dorsal SCN, implying that Ca2+ activity in astrocytic processes has important functional relevance in this region. Indeed, this study identified that the enhanced astrocytic Ca2+ signaling corresponds with astrocytic glutamate release which suppresses neural activity though increased GABAergic tone, mediated by astrocytic NMDARs in the dorsal SCN (Brancaccio et al., 2017).
Astrocytic Ca2+ activity also shows circadian rhythmicity in vivo. Leveraging long range fiberscope imaging in behaving mice, it was shown that cortical astrocytes exhibit robust somatic Ca2+ fluctuations corresponding with the animal’s activity, with higher frequency and amplitude during active periods than during quiescence (Gau et al., 2024). These oscillations in somatic Ca2+ signals may be driven by circadian changes in expression of the glial ER receptor IP3R2. Recent evidence in primary cultured cortical astrocytes demonstrates that rhythmic expression of heat shock factor-1 regulated protein (HERP) regulates the degradation of IP3Rs in a circadian manner. IP3R2 expression was found to be anti-phase to HERP expression, and ATP induced somatic Ca2+ transients, which are normally higher during subjective night (corresponding to active periods for rodent astrocytes), lost rhythmicity in Herp knockdown astrocytes (Ryu et al., 2024). HERP mediated IP3R2 Ca2+ signaling was also linked to the rhythmic phosphorylation of connexin 43, which is shown to reduce gap junction conductance (Solan and Lampe, 2014; Nimlamool et al., 2015), thereby modulating Ca2+ signaling across astrocyte networks.
Sleep/wake
During sleep/wake cycles, astrocytic Ca2+ signaling is heterogeneous across brain regions. Outside of the SCN, astrocytic Ca2+ fluctuations generally correlate with activity levels, decreasing/desynchronizing during sleep and amplifying/synchronizing during wakefulness (Bojarskaite et al., 2020; Ingiosi et al., 2020; Vaidyanathan et al., 2021; Peng et al., 2023; Gau et al., 2024; Péter and Héja, 2024; Ryu et al., 2024). Conversely, some brain regions such as the basolateral forebrain (BF) and brainstem exhibit increased astrocytic Ca2+ signaling during rapid eye movement (REM) sleep, characterized by high levels of neural activity, muscle atonia, and dreaming. Chemogenetic modulation of astrocytic Ca2+ using Gq-DREADDs generally reduced REM sleep, while differentially impacting brain activity in the delta frequency associated with non-REM sleep, reducing it in the BF and increasing it in the brainstem, suggesting that astrocytic Ca2+ dependent modulation of sleep/wake activity is both sleep state and brain region specific (Peng et al., 2023).
Recent work demonstrates that the arousal inducing effects of astrocytic Ca2+ are prominent at the network level, with global increases in intracellular astrocytic Ca2+ waves underlying the transitions from quiescent to active behavior, an effect which was strongly suppressed by inhibition of NE release from presynaptic terminals, suggesting an important role for this neuromodulator in elevating astrocytic Ca2+ during arousal (Gau et al., 2024). Consistently, live imaging of GECI expressing astrocytes shows that in the barrel cortex (BC), NE release from the locus coeruleus toggles a switch from small Ca2+ signals (observed in the quiescent BC during whisker stimulation) to large Ca2+ waves (observed in the awake BC during whisker stimulation) in the astrocytic processes (Wang et al., 2023). Interestingly, astrocytes in the BC also show large Ca2+ fluctuations in somata and processes underlying slow wave sleep to arousal, but not REM to arousal transitions (Bojarskaite et al., 2020). Combining Ca2+ imaging with local field potential recording in the BC showed that the small Ca2+ transients (characteristic of BC astrocytes during sleep) reduced EPSP amplitude, suppressing sensory transmission (Wang et al., 2023) and providing a potential mechanism for the role of astrocytic Ca2+ signaling in sleep modulation in this region. Duality in astrocytic Ca2+ signals is also observed in drosophila, where Ca2+ increases in somas and processes mediated by the astrocyte specific temperature sensitive cation channel dTrpA1 resulted in two unique phenotypes: a fast elevation in sleep which occurred at night, and delayed but persistent increase in sleep during the day supporting that in flies, astrocytic Ca2+ signaling encodes sleep pressure (Blum et al., 2021; Srinivasan, 2021). Taken together these findings suggest that astrocytic Ca2+ fluctuations are multimodal and intimately involved with state transitions between sleep and arousal.
Astrocytic Ca2+ activity is also implied in sleep architecture, which refers to the structured organization and progression of sleep stages across a sleep period (Younes et al., 2022). In studies combining Ca2+ imaging in behaving, head-fixed mice with electrocorticography (ECoG), it was shown that inhibition of ER released astrocytic Ca2+ through deletion of IP3R2 causes slow wave sleep to become fragmented, corresponding with reduced ECoG delta power (Bojarskaite et al., 2020), in agreement with studies showing that high frequency somatic astrocytic Ca2 + oscillations in the delta (and theta) frequency are critical for modulating slow wave sleep (Péter and Héja, 2024). The sleep phenotypes resulting from IP3R2 KO are likely linked to an inability to respond to both Gαq and Gαi-coupled GPCR-mediated signaling pathways, which are shown to regulate sleep duration and depth, respectively (Vaidyanathan et al., 2021).
Nutrient intake
Additional homeostatic behaviors modulated by astrocytic Ca2+ dynamics include feeding and drinking. During these behaviors under ad libitum conditions, cortical astrocytic Ca2+ transients are suppressed (Gau et al., 2024). However, after starvation or water restriction, astrocytic Ca2+ signals increase in response to food or water, and to a greater extent when visual or olfactory cues are presented to deprived mice while food is inaccessible. These data suggest that cortical astrocyte activity drives these behaviors and is flexible to neuromodulation dependent on internal motivation state (Gau et al., 2024). Indeed, in the murine arcuate nucleus (ARC) of the hypothalamus, which plays a central role in feeding behaviors (Zhang et al., 2019), chemogenetic Gq-DREADD manipulation of astrocytes induced robust somatic Ca2+ signaling driving food intake through increased activation of orexigenic AgRP/NPY neurons, which inhibit satiety promoting neurons (Chen et al., 2016; Zhang et al., 2019). These findings correspond with reports showing that astrocytes modulate feeding behavior through the regulation of extracellular adenosine levels, which is coupled to astrocytic intracellular Ca2+ levels (Yang et al., 2015). Further, hypothalamic astrocytes downstream of ARC nucleus show robust leptin receptor expression which induces somatic Ca2+ signals upon stimulation in mice. Leptin, a hormone produced by adipocytes, has a prominent role in satiety behavior, with increased levels leading to leptin resistance in obesity and metabolic syndromes (Engin, 2017; Balland et al., 2019; Zhang et al., 2019). Astrocytic leptin receptors and the related Ca2+ transients are increased in mice subject to diet induced obesity (DIO), suggesting a role for astrocytic Ca2+ in obesity linked metabolic disruption (Hsuchou et al., 2009). Accordingly, a recent report demonstrates that DIO in mice increases the frequency and amplitude of Ca2+ signals in astrocytes in the paraventricular nucleus (PVN), ARC, and dorsomedial nucleus of the hypothalamus (DMH), without affecting astrocytic Ca2+ mobilization in the ventromedial nucleus of the hypothalamus (VMH). Additionally, chemogenetic manipulation of these Ca2+ signals had aggravating (via Gq-DREADD) or alleviating (via Gi-DREADD) effects on metabolic condition in mice subject to DIO (Herrera Moro Chao et al., 2022).
Taken together, these findings provide critical insight into the role of astrocytic Ca2+ signaling in the modulation of homeostatic behaviors. Astrocytes regulate these processes through Ca2+ fluctuations at the network level and in multiple subcellular compartments in a heterogeneous manner dependent on the brain region and physiological context. Given its central role in arousal, particularly in sleep-wake transitions characterized by enhanced astrocytic Ca2+ signaling, NE is emerging as a key component in these processes, potentially mediated by astrocytic α1-adrenergic receptors. However, the specific interactions between neuronal NE release and astrocytic Ca2+ dynamics remain unclear, calling for further targeted investigations, especially with respect to spatially and functionally distinct astrocytic Ca2+ signals across brain regions. Furthermore, very little is known about the roles of astrocytic Ca2+ in other survival behaviors such as defensive responses, or how astrocytic Ca2+ integrates with metabolic signals, such as leptin and adenosine. Uncovering these roles may provide insights into how disruptions in relevant pathways contribute to disorders of sleep, metabolism, and circadian misalignment.
Astrocytic Ca2+ signaling regulates affective and social behaviors
Fear
Affective behaviors such as fear and anxiety, are fundamental to organismal responses to environmental stimuli (Raber et al., 2019; Mendl and Paul, 2020), and astrocytic Ca2+ signaling has been widely identified as a major component in mediating these behaviors. In vivo Ca2+ imaging during air-puff evoked startle demonstrated robust, global astrocytic Ca2+ responses in the cortex which consisted of a fast α1-adrenoceptor dependent spike in somatic Ca2+, and a phasic Ca2+ response with both early and late components within the astrocytic processes that was unaffected by α1-adrenoceptor blockade. In IP3R2 KO mice, somatic signals and early responses within the astrocytic processes were abrogated, but the late component was still readily identifiable, underscoring the complexity of compartmentalized astrocyte activation in response to relevant stimuli (Srinivasan et al., 2015). This air-puff-evoked startle response could be attenuated through expression of the GPCR signaling inhibitor, iβARK (Nagai et al., 2021). Astrocytic Ca2+ transients also mediate startle responses in zebrafish, with Ca2+ propagating bidirectionally from astrocytes in the rostral spinal cord through gap junctions in glial networks in a glutamate dependent manner, requiring adrenergic signaling for propagation in the hindbrain (Orts-Del’Immagine et al., 2022).
Neural mechanisms underlying startle responses are tightly interrelated with processes governing the more complex acquisition of fear, which engages numerous brain regions responsible for threat detection, stimulus integration, and contextual memory (de Haan et al., 2018; Zheng and Schmid, 2023). Recent work implies a prominent role for astrocytic Ca2+ signals in the modulation of these effects in the basolateral amygdala (BLA). During foot shock, BLA astrocytic Ca2+ signals were significantly elevated relative to non-shocked controls, indicating their role in encoding stimulus salience. Fascinatingly, different stages of fear acquisition appear to engage potentially distinct populations of astrocytes with unique Ca2+ kinetics (Suthard et al., 2023b). In the medial subdivision of the central amygdala (CeM), astrocytes respond to both endogenous endocannabinoid or exogenous Gq-DREADDs stimulation with robust Ca2+ transients, which enhanced inhibitory signaling at lateral central amygdala to CeM synapses, dampening excitatory signaling at BLA-CeM synapses and reducing fear expression in a delayed fear conditioning paradigm (Martin-Fernandez et al., 2017). Together these results demonstrate that context, population, and synapse specific astrocytic Ca2+ signals are highly diverse across different components of fear behavior.
Anxiety
While startle and fear represent acute responses to aversive stimuli, anxiety is characterized by a prolonged anticipation of and often disproportionate response to potential danger (Duval et al., 2015). Extruding Ca2+ from NaC astrocytes by overexpression of the CalEx pump led to pronounced reductions in anxiety behavior, increasing exploratory behaviors in mice. However, this manipulation also increased compulsive behaviors, such as perseverative responses in five choice serial reaction time task and enhanced hedonia in sucrose preference test (Peyton et al., 2025). These findings underscore a potential role for astrocytic Ca2+ not only in anxiety, but at the intersection between anxiogenesis and compulsive hedonic behaviors, which is a major factor underlying addiction (Koob, 2008). The hippocampus is also strongly implicated in the development of anxiety like behaviors, and recent evidence demonstrates an important role for astrocytic Ca2+ signaling in these processes. In vivo Ca2+ imaging in head-fixed mice revealed robust increases in astrocytic Ca2+ during the anxiogenic phase of a virtual reality paradigm, while mice in non-anxiogenic phases had minimal Ca2+ elevations. Interestingly while most astrocytes within the field of view responded to the anxiogenic phase, a smaller fraction of astrocytes responded specifically to the non-anxiogenic phase, suggesting heterogeneously respondent hippocampal astrocyte populations, and reinforcing the notion that astrocytes encode behavioral salience (Cho et al., 2022). Others show that anxiety-linked astrocytic Ca2+ increases are specifically abundant in the ventral hippocampus during anxiogenic behaviors, and conditional knockout of astrocytic IP3R2 was anxiolytic, implying a role for store-released Ca2+ in anxiety modulation. In these studies, it was also shown that chemogenetic manipulation of astrocytic Ca2+ signaling increased anxiogenic conditions through the enhanced release of glutamate, which contributes to stress susceptibility through neuronal NMDAR stimulation which could be ameliorated with specific NMDAR antagonists (Li et al., 2024). These effects may be modulated by metabotropic glutamate receptor (mGluR) signaling. MGluR5 induces robust IP3 mediated Ca2+ release in astrocytes, and its specific knockdown is associated with reduced inhibitory synaptic inputs in CA1 which correspond with increased anxiety-like behaviors (Li et al., 2023). In mice with chronically activated Gq-DREADDs, astrocytic Ca2+ signals in the ventral CA1 and anxiety-like behaviors were increased, but only in three month old mice, while six month old mice exhibited no change relative to controls (Suthard et al., 2023a). These findings highlight the importance of considering developmental timepoint, however, effects may also be due to compensatory astrocytic mechanisms, as chronic Gq-DREADD stimulation would also generate chronic Ca2+ depletion.
Depression
Persistent anxiety, among other factors, can contribute to the development of depression (Ross et al., 2017), an affective behavior characterized by prolonged emotional dysregulation and reduced motivation and pleasure. Given the findings linking aberrant astrocytic Ca2+ signaling to anxiety, it is unsurprising that it is also implied in depressive phenotypes. Reducing astrocytic Ca2+ via CalEx pump extrusion during a critical period in mouse development (postnatal week 2–3) led to synaptic hyperexcitation and depressive like behaviors in adults, including anti-social behaviors and prolonged immobility in tail suspension and forced swim tests which could be rescued through Gq-DREADD stimulation (Luo et al., 2023). Further, in chronically corticosterone treated juvenile mice, mPFC astrocytic Ca2+ fluctuations were aberrant at baseline and reduced during social and exploration behaviors relative to untreated controls, and serotonin (5-HT) evoked astrocytic Ca2+ signals were diminished (González-Arias et al., 2023). These age specific findings introduce important questions regarding the developmental nature of astrocytic Ca2+ signals, and how disruptions at specific timepoints may contribute to long term consequences.
Social behavior
Astrocytic Ca2+ signaling is also implicated in social behaviors, contributing to social interactions and disorders characterized by their dysregulation. Recent evidence shows that social dominance behaviors are modulated by these signals, with astrocytic Ca2+ increasing in the mPFC during assertive and resistant behaviors between male mice, with higher amplitude responses recorded in dominant mice compared to subordinates. The study also identified that these behaviors were mediated by astrocytic release of glutamate and ATP, which regulate cortical excitation/inhibition (E/I) balance (Noh et al., 2023). This in part may be modulated by store-released Ca2+ signaling, as IP3R2 KO mice exhibited a delay in the assertion of dominance behaviors relative to wild type controls, with no effect on competitive outcome, suggesting the involvement of other pathways in these behaviors (Guillot de Suduiraut et al., 2021). Moreover, astrocytic Ca2+ signaling is implied in the social behavioral deficits observed in autism spectrum disorders (ASD). Transplantation of ASD derived human astrocytes into mouse brains induced deficits including repetitive compulsive behaviors (perseverative digging) and attenuated fear memory. The ASD derived astrocytes elicited aberrant, exaggerated Ca2+ signals relative to wild type astrocytes, implying that increased Ca2+ and subsequent gliotransmitter release underlies ASD like behavioral dysfunction in mice (Allen et al., 2022). IP3R2 mediated Ca2+ transients are linked to ASD like behaviors in mice, with IP3R2 KO mice exhibiting antisocial behavior in a place preference test and increased repetitive behaviors including perseverative digging as well as compulsive-like grooming behaviors. These behavioral deficits, as well as GABAergic neurotransmission, which was abrogated by IP3R2 KO, could be rescued by treatment with ATP or ATPyS, a gliotransmitter which is reduced in IP3R2 KO mice, implying a potential mechanism for astrocytic store-released Ca2+ signals in ASD related behavioral pathology (Wang et al., 2021). In agreement with these results, repetitive grooming behaviors were also observed following CalEx pump-mediated extrusion of astrocytic Ca2+ which disrupted striatal microcircuits, suggesting the involvement of astrocytic Ca2+ in this brain region in ASD pathology (Yu et al., 2018).
Thus, astrocytic Ca2+ signaling plays a crucial role in modulating affective and social behaviors, influencing processes like startle response, fear acquisition, anxiety regulation, and social dominance. However, unanswered questions remain regarding specific facets of astrocytic Ca2+ in these processes, for instance: how does gap junction mediated networked Ca2+ activity contribute to the development and maintenance of fear linked behaviors? What are the long-term effects of Ca2+ dysregulation in affective disorders, and are they limited to specific subsets of astrocytes? As transplanted human ASD astrocytes induced pathological phenotypes in rodents, can this approach be leveraged to investigate other disorders? Future studies considering the heterogeneity of astrocytes that regulate these behaviors, such as regional subpopulations, molecular signatures, specific gliotransmitter release, and different Ca2+ signaling pathways will be imperative to the identification of precise regulatory mechanisms involved in these processes. Importantly, because many of the neural pathways involved and phenotypes observed overlap between affective and social behaviors, studies characterizing their outcomes should be attentive to how astrocytic Ca2+ dynamics differ across these distinct behavioral responses.
Conclusion and perspectives
There is mounting evidence that astrocytic Ca2+ signaling is a prominent and fundamental regulator of neural processing, influencing a wide range of behaviors from sensory perception and learning to affective and social interactions. The dynamic and regionally heterogeneous nature of astrocytic Ca2+ fluctuations emphasize the importance of their role in the contextually specific encoding of critical determinants of behavior, including stimulus salience and transitions between activity states. While significant progress has been made in characterizing the diverse modes of Ca2+ activity correlating with behavioral outputs, questions remain regarding the molecular mechanisms underlying these effects as well as the relationships between astrocytic Ca2+ signaling and other neuromodulatory systems in the CNS that drive behavioral output. Investigating the role of neurotransmitters such as NE which is emerging as a major modulator of astrocytic Ca2+ activity, will be necessary to discern the distinct circuit dependent roles of astrocytic Ca2+ signaling in behavioral regulation. Additionally, attention to the different sources and types of Ca2+ signals, and the underlying mechanisms that induce or suppress them, will be imperative to understanding how astrocytes leverage Ca2+ to process information. Ascertaining which of these signals lead to gliotransmitter release, and in which contexts, will improve our understanding of how astrocytic Ca2+ modulates neuronal activity. Further, interactions with other glial cells in the behavioral context are largely unexplored. Future research leveraging high resolution imaging, genetic manipulations, and circuit level analyses will be critical to understanding the roles of astrocytic Ca2+ signals at the subcellular, single cell, and global network level. Such investigations will provide deeper insight into the role of astrocytic Ca2+ signaling in fundamental neurobiological processes involved in behavior and identify novel therapeutic targets for behavioral disorders.
Author contributions
GI: Conceptualization, Investigation, Writing – review and editing, Writing – original draft. IF-T: Supervision, Writing – review and editing, Conceptualization, Investigation, Writing – original draft, Data curation, Resources, Visualization, Funding acquisition.
Funding
The authors declare that financial support was received for the research and/or publication of this article. This work was funded by NIH R01NS133047 to IF-T.
Acknowledgments
Some figures components were designed using BioRender. In graphical abstract image credit GI, Farhy lab. We apologize to those whose work was not cited due to our oversight or to space considerations.
Conflict of interest
The authors declare that the research was conducted in the absence of any commercial or financial relationships that could be construed as a potential conflict of interest.
Generative AI statement
The authors declare that no Generative AI was used in the creation of this manuscript.
Publisher’s note
All claims expressed in this article are solely those of the authors and do not necessarily represent those of their affiliated organizations, or those of the publisher, the editors and the reviewers. Any product that may be evaluated in this article, or claim that may be made by its manufacturer, is not guaranteed or endorsed by the publisher.
References
Adamsky, A., Kol, A., Kreisel, T., Doron, A., Ozeri-Engelhard, N., Melcer, T., et al. (2018). Astrocytic activation generates de novo neuronal potentiation and memory enhancement. Cell 174, 59–71.e14. doi: 10.1016/j.cell.2018.05.002
Agarwal, A., Wu, P., Hughes, E., Fukaya, M., Tischfield, M., Langseth, A., et al. (2017). Transient opening of the mitochondrial permeability transition pore induces microdomain calcium transients in astrocyte processes. Neuron 93, 587–605.e7. doi: 10.1016/j.neuron.2016.12.034
Ahmadpour, N., Kantroo, M., and Stobart, J. (2021). Extracellular calcium influx pathways in astrocyte calcium microdomain physiology. Biomolecules 11:1467. doi: 10.3390/biom11101467
Ahmadpour, N., Kantroo, M., Stobart, M., Meza-Resillas, J., Shabanipour, S., Parra-Nuñez, J., et al. (2024). Cortical astrocyte N-methyl-D-aspartate receptors influence whisker barrel activity and sensory discrimination in mice. Nat. Commun. 15:1571. doi: 10.1038/s41467-024-45989-3
Ahn, B., Kim, M., and Kim, S. (2022). Brain circuits for promoting homeostatic and non-homeostatic appetites. Exp. Mol. Med. 54, 349–357. doi: 10.1038/s12276-022-00758-4
Ahrens, M., Khakh, B., and Poskanzer, K. (2024). Astrocyte calcium signaling. Cold Spring Harb. Perspect. Biol. 16:a041353. doi: 10.1101/cshperspect.a041353
Aizenberg, M., Mwilambwe-Tshilobo, L., Briguglio, J., Natan, R., and Geffen, M. (2015). Bidirectional regulation of innate and learned behaviors that rely on frequency discrimination by cortical inhibitory neurons. PLoS Biol. 13:e1002308. doi: 10.1371/journal.pbio.1002308
Allen, M., Huang, B., Notaras, M., Lodhi, A., Barrio-Alonso, E., Lituma, P., et al. (2022). Astrocytes derived from ASD individuals alter behavior and destabilize neuronal activity through aberrant Ca2+ signaling. Mol. Psychiatry 27, 2470–2484. doi: 10.1038/s41380-022-01486-x
Anand, S., Sogukpinar, F., and Monosov, I. (2024). Arousal effects on oscillatory dynamics in the non-human primate brain. Cereb. Cortex 34:bhae473. doi: 10.1093/cercor/bhae473
Bai, Y., Zhou, Z., Han, B., Xiang, X., Huang, W., and Yao, H. (2024). Revisiting astrocytic calcium signaling in the brain. Fundam. Res. 4, 1365–1374. doi: 10.1016/j.fmre.2023.11.021
Balland, E., Chen, W., Dodd, G., Conductier, G., Coppari, R., Tiganis, T., et al. (2019). Leptin signaling in the arcuate nucleus reduces insulin’s capacity to suppress hepatic glucose production in obese mice. Cell Rep 26, 346–355.e3. doi: 10.1016/j.celrep.2018.12.061
Barca Mayo, O., Berdondini, L., and De Pietri Tonelli, D. (2019). Astrocytes and circadian rhythms: An emerging astrocyte-neuron synergy in the timekeeping system. Methods Mol. Biol. 1938, 131–154. doi: 10.1007/978-1-4939-9068-9_10
Bindocci, E., Savtchouk, I., Liaudet, N., Becker, D., Carriero, G., and Volterra, A. (2017). Three-dimensional Ca2+ imaging advances understanding of astrocyte biology. Science 356:eaai8185. doi: 10.1126/science.aai8185
Blum, I., Keleş, M., Baz, E., Han, E., Park, K., Luu, S., et al. (2021). Astroglial calcium signaling encodes sleep need in Drosophila. Curr. Biol. 31, 150–162.e7. doi: 10.1016/j.cub.2020.10.012
Bojarskaite, L., Bjørnstad, D., Pettersen, K., Cunen, C., Hermansen, G., Åbjørsbråten, K., et al. (2020). Astrocytic Ca2+ signaling is reduced during sleep and is involved in the regulation of slow wave sleep. Nat. Commun. 11:3240. doi: 10.1038/s41467-020-17062-2
Bonder, D., and McCarthy, K. (2014). Astrocytic Gq-GPCR-linked IP3R-dependent Ca2+ signaling does not mediate neurovascular coupling in mouse visual cortex in vivo. J. Neurosci. 34, 13139–13150. doi: 10.1523/JNEUROSCI.2591-14.2014
Bosson, A., Paumier, A., Boisseau, S., Jacquier-Sarlin, M., Buisson, A., and Albrieux, M. (2017). TRPA1 channels promote astrocytic Ca2+ hyperactivity and synaptic dysfunction mediated by oligomeric forms of amyloid-β peptide. Mol. Neurodegener. 12:53. doi: 10.1186/s13024-017-0194-8
Brancaccio, M., Patton, A., Chesham, J., Maywood, E., and Hastings, M. (2017). Astrocytes control circadian timekeeping in the suprachiasmatic nucleus via glutamatergic signaling. Neuron 93, 1420–1435.e5. doi: 10.1016/j.neuron.2017.02.030
Buonomano, D., and Maass, W. (2009). State-dependent computations: Spatiotemporal processing in cortical networks. Nat. Rev. Neurosci. 10, 113–125. doi: 10.1038/nrn2558
Bushong, E., Martone, M., Jones, Y., and Ellisman, M. (2002). Protoplasmic astrocytes in CA1 stratum radiatum occupy separate anatomical domains. J. Neurosci. 22, 183–192. doi: 10.1523/JNEUROSCI.22-01-00183.2002
Buzsáki, G., and Wang, X. (2012). Mechanisms of gamma oscillations. Annu. Rev. Neurosci. 35, 203–225. doi: 10.1146/annurev-neuro-062111-150444
Cahill, M., Collard, M., Tse, V., Reitman, M., Etchenique, R., Kirst, C., et al. (2024). Network-level encoding of local neurotransmitters in cortical astrocytes. Nature 629, 146–153. doi: 10.1038/s41586-024-07311-5
Cheli, V., Santiago González, D., Smith, J., Spreuer, V., Murphy, G., and Paez, P. M. (2016). L-type voltage-operated calcium channels contribute to astrocyte activation In vitro. Glia 64, 1396–1415. doi: 10.1002/glia.23013
Chen, C., Altafi, M., Corbu, M., Trenk, A., van den Munkhof, H., Weineck, K., et al. (2024). The dynamic state of a prefrontal-hypothalamic-midbrain circuit commands behavioral transitions. Nat. Neurosci. 27, 952–963. doi: 10.1038/s41593-024-01598-3
Chen, N., Sugihara, H., Kim, J., Fu, Z., Barak, B., Sur, M., et al. (2016). Direct modulation of GFAP-expressing glia in the arcuate nucleus bi-directionally regulates feeding. Elife 5:e18716. doi: 10.7554/eLife.18716
Cho, W., Noh, K., Lee, B., Barcelon, E., Jun, S., Park, H., et al. (2022). Hippocampal astrocytes modulate anxiety-like behavior. Nat. Commun. 13:6536. doi: 10.1038/s41467-022-34201-z
Colgin, L. (2011). Oscillations and hippocampal-prefrontal synchrony. Curr. Opin. Neurobiol. 21, 467–474. doi: 10.1016/j.conb.2011.04.006
Coomans, C., Saaltink, D., Deboer, T., Tersteeg, M., Lanooij, S., Schneider, A., et al. (2021). Doublecortin-like expressing astrocytes of the suprachiasmatic nucleus are implicated in the biosynthesis of vasopressin and influences circadian rhythms. Glia 69, 2752–2766. doi: 10.1002/glia.24069
de Assis, L., and Oster, H. (2021). The circadian clock and metabolic homeostasis: Entangled networks. Cell Mol. Life Sci. 78, 4563–4587. doi: 10.1007/s00018-021-03800-2
de Haan, M., van Well, S., Visser, R., Scholte, H., van Wingen, G., and Kindt, M. (2018). The influence of acoustic startle probes on fear learning in humans. Sci. Rep. 8:14552. doi: 10.1038/s41598-018-32646-1
Denizot, A., Arizono, M., Nägerl, U., Berry, H., and De Schutter, E. (2022). Control of Ca2+ signals by astrocyte nanoscale morphology at tripartite synapses. Glia 70, 2378–2391. doi: 10.1002/glia.24258
Ding, Q., Zhu, J., and Yan, C. (2022). Encoding tasks moderated the reward effect on brain activity during memory retrieval. Sci. Rep. 12:8246. doi: 10.1038/s41598-022-12344-9
Doron, A., Rubin, A., Benmelech-Chovav, A., Benaim, N., Carmi, T., Refaeli, R., et al. (2022). Hippocampal astrocytes encode reward location. Nature 609, 772–778. doi: 10.1038/s41586-022-05146-6
Duval, E., Javanbakht, A., and Liberzon, I. (2015). Neural circuits in anxiety and stress disorders: A focused review. Ther. Clin. Risk Manag. 11, 115–126. doi: 10.2147/TCRM.S48528
Engin, A. (2017). Diet-induced obesity and the mechanism of leptin resistance. Adv. Exp. Med. Biol. 960, 381–397. doi: 10.1007/978-3-319-48382-5_16
Escalada, P., Ezkurdia, A., Ramírez, M., and Solas, M. (2024). Essential role of astrocytes in learning and memory. Int. J. Mol. Sci. 25:1899. doi: 10.3390/ijms25031899
Farhy-Tselnicker, I., and Allen, N. (2018). Astrocytes, neurons, synapses: A tripartite view on cortical circuit development. Neural Dev. 13:7. doi: 10.1186/s13064-018-0104-y
Fujii, Y., Maekawa, S., and Morita, M. (2017). Astrocyte calcium waves propagate proximally by gap junction and distally by extracellular diffusion of ATP released from volume-regulated anion channels. Sci. Rep. 7:13115. doi: 10.1038/s41598-017-13243-0
Gau, Y., Hsu, E., Cha, R., Pak, R., Looger, L., Kang, J., et al. (2024). Multicore fiber optic imaging reveals that astrocyte calcium activity in the mouse cerebral cortex is modulated by internal motivational state. Nat. Commun. 15:3039. doi: 10.1038/s41467-024-47345-x
Goenaga, J., Araque, A., Kofuji, P., and Herrera Moro Chao, D. (2023). Calcium signaling in astrocytes and gliotransmitter release. Front. Synaptic Neurosci. 15:1138577. doi: 10.3389/fnsyn.2023.1138577
González-Arias, C., Sánchez-Ruiz, A., Esparza, J., Sánchez-Puelles, C., Arancibia, L., Ramírez-Franco, J., et al. (2023). Dysfunctional serotonergic neuron-astrocyte signaling in depressive-like states. Mol. Psychiatry 28, 3856–3873. doi: 10.1038/s41380-023-02269-8
Guerra-Gomes, S., Sousa, N., Pinto, L., and Oliveira, J. (2018). Functional roles of astrocyte calcium elevations: From synapses to behavior. Front. Cell Neurosci. 11:427. doi: 10.3389/fncel.2017.00427
Guillot de Suduiraut, I., Grosse, J., Ramos-Fernández, E., Sandi, C., and Hollis, F. (2021). Astrocytic release of ATP through type 2 inositol 1,4,5-trisphosphate receptor calcium signaling and social dominance behavior in mice. Eur. J. Neurosci. 53, 2973–2985. doi: 10.1111/ejn.14892
Hastings, M., Brancaccio, M., Gonzalez-Aponte, M., and Herzog, E. (2023). Circadian rhythms and astrocytes: The good, the bad, and the ugly. Annu. Rev. Neurosci. 46, 123–143. doi: 10.1146/annurev-neuro-100322-112249
Herrera Moro Chao, D., Kirchner, M., Pham, C., Foppen, E., Denis, R., Castel, J., et al. (2022). Hypothalamic astrocytes control systemic glucose metabolism and energy balance. Cell Metab. 34, 1532–1547.e6. doi: 10.1016/j.cmet.2022.09.002
Hirrlinger, J., and Nimmerjahn, A. (2022). A perspective on astrocyte regulation of neural circuit function and animal behavior. Glia 70, 1554–1580. doi: 10.1002/glia.24168
Hösli, L., Zuend, M., Bredell, G., Zanker, H., Porto de Oliveira, C., Saab, A., et al. (2022). Direct vascular contact is a hallmark of cerebral astrocytes. Cell Rep. 39:110599. doi: 10.1016/j.celrep.2022.110599
Hsuchou, H., He, Y., Kastin, A., Tu, H., Markadakis, E., Rogers, R., et al. (2009). Obesity induces functional astrocytic leptin receptors in hypothalamus. Brain 132(Pt 4), 889–902. doi: 10.1093/brain/awp029
Hu, Q., Wu, X., Guo, C., Wang, T., Guo, H., Wang, J., et al. (2024). Astrocyte-neuron crosstalk through extracellular vesicle-shuttled miRNA-382-5p promotes traumatic brain injury. Exp. Mol. Med. 56, 2642–2658. doi: 10.1038/s12276-024-01355-3
Huang, A., Woo, J., Sardar, D., Lozzi, B., Bosquez Huerta, N., Lin, C., et al. (2020). Region-specific transcriptional control of astrocyte function oversees local circuit activities. Neuron 106, 992–1008.e9. doi: 10.1016/j.neuron.2020.03.025
Imrie, G., Gray, M., Raghuraman, V., and Farhy-Tselnicker, I. (2024). Gene expression at the tripartite synapse: bridging the gap between neurons and astrocytes. Adv. Neurobiol. 39, 95–136. doi: 10.1007/978-3-031-64839-7_5
Inácio, A., Lam, K., Zhao, Y., Pereira, F., Gerfen, C., and Lee, S. (2025). Brain-wide presynaptic networks of functionally distinct cortical neurons. Nature [Online ahead of print]. doi: 10.1038/s41586-025-08631-w
Ingiosi, A., Hayworth, C., Harvey, D., Singletary, K., Rempe, M., Wisor, J., et al. (2020). A role for astroglial calcium in mammalian sleep and sleep regulation. Curr. Biol. 30, 4373–4383.e7. doi: 10.1016/j.cub.2020.08.052
Jego, P., Pacheco-Torres, J., Araque, A., and Canals, S. (2014). Functional MRI in mice lacking IP3-dependent calcium signaling in astrocytes. J. Cereb. Blood Flow Metab. 34, 1599–1603. doi: 10.1038/jcbfm.2014.144
Jha, M., Jo, M., Kim, J., and Suk, K. (2019). Microglia-astrocyte crosstalk: An intimate molecular conversation. Neuroscientist 25, 227–240. doi: 10.1177/1073858418783959
Kang, S., Hong, S., Kang, S., Song, M., Yang, M., Essa, H., et al. (2023). Astrocyte activities in the external globus pallidus regulate action-selection strategies in reward-seeking behaviors. Sci. Adv. 9:eadh9239. doi: 10.1126/sciadv.adh9239
Khakh, B., and Deneen, B. (2019). The emerging nature of astrocyte diversity. Annu. Rev. Neurosci. 42, 187–207. doi: 10.1146/annurev-neuro-070918-050443
Khakh, B., and McCarthy, K. (2015). Astrocyte calcium signaling: From observations to functions and the challenges therein. Cold Spring Harb. Perspect. Biol. 7:a020404. doi: 10.1101/cshperspect.a020404
Kim, J., Gulati, T., and Ganguly, K. (2019). Competing roles of slow oscillations and delta waves in memory consolidation versus forgetting. Cell 179, 514–526.e13. doi: 10.1016/j.cell.2019.08.040
Kofuji, P., and Araque, A. (2021a). Astrocytes and behavior. Annu. Rev. Neurosci. 44, 49–67. doi: 10.1146/annurev-neuro-101920-112225
Kofuji, P., and Araque, A. G. - (2021b). Protein-coupled receptors in astrocyte-neuron communication. Neuroscience 456, 71–84. doi: 10.1016/j.neuroscience.2020.03.025
Kol, A., Adamsky, A., Groysman, M., Kreisel, T., London, M., and Goshen, I. (2020). Astrocytes contribute to remote memory formation by modulating hippocampal-cortical communication during learning. Nat. Neurosci. 23, 1229–1239. doi: 10.1038/s41593-020-0679-6
Koob, G. (2008). Hedonic homeostatic dysregulation as a driver of drug-seeking behavior. Drug Discov. Today Dis. Models 5, 207–215. doi: 10.1016/j.ddmod.2009.04.002
Lam, T. (2010). Neuronal regulation of homeostasis by nutrient sensing. Nat. Med. 16, 392–395. doi: 10.1038/nm0410-392
Lee, J., Kim, J., Noh, S., Lee, H., Lee, S., Mun, J., et al. (2021). Astrocytes phagocytose adult hippocampal synapses for circuit homeostasis. Nature 590, 612–617. doi: 10.1038/s41586-020-03060-3
Lee, S., Mak, A., and Verheijen, M. (2023). Comparative assessment of the effects of DREADDs and endogenously expressed GPCRs in hippocampal astrocytes on synaptic activity and memory. Front. Cell Neurosci. 17:1159756. doi: 10.3389/fncel.2023.1159756
Li, J., Jin, S., Hu, J., Xu, R., Xu, J., Li, Z., et al. (2024). Astrocytes in the ventral hippocampus bidirectionally regulate innate and stress-induced anxiety-like behaviors in male mice. Adv. Sci. 11:e2400354. doi: 10.1002/advs.202400354
Li, X., Du, Z., Xu, J., Liang, Z., Lin, S., Chen, H., et al. (2023). mGluR5 in hippocampal CA1 pyramidal neurons mediates stress-induced anxiety-like behavior. Neuropsychopharmacology 48, 1164–1174. doi: 10.1038/s41386-023-01548-w
Lia, A., Henriques, V., Zonta, M., Chiavegato, A., Carmignoto, G., Gómez-Gonzalo, M., et al. (2021). Calcium signals in astrocyte microdomains, a decade of great advances. Front. Cell Neurosci. 15:673433. doi: 10.3389/fncel.2021.673433
Lia, A., Sansevero, G., Chiavegato, A., Sbrissa, M., Pendin, D., Mariotti, L., et al. (2023). Rescue of astrocyte activity by the calcium sensor STIM1 restores long-term synaptic plasticity in female mice modelling Alzheimer’s disease. Nat. Commun. 14:1590. doi: 10.1038/s41467-023-37240-2
Lines, J., Martin, E. D., Kofuji, P., Aguilar, J., and Araque, A. (2020). Astrocytes modulate sensory-evoked neuronal network activity. Nat. Commun. 11:3689. doi: 10.1038/s41467-020-17536-3
Liu, T., Qi, C., Bai, W., Tian, X., and Zheng, X. (2022). Behavioral state-dependent oscillatory activity in prefrontal cortex induced by chronic social defeat stress. Front. Neurosci. 16:885432. doi: 10.3389/fnins.2022.885432
Lohr, C., Beiersdorfer, A., Fischer, T., Hirnet, D., Rotermund, N., Sauer, J., et al. (2021). Using genetically encoded calcium indicators to study astrocyte physiology: A field guide. Front. Cell Neurosci. 15:690147. doi: 10.3389/fncel.2021.690147
Lorin, C., Guiet, R., Chiaruttini, N., Ambrosini, G., Boci, E., Abdellah, M., et al. (2024). Structural and molecular characterization of astrocyte and vasculature connectivity in the mouse hippocampus and cortex. Glia 72, 2001–2021. doi: 10.1002/glia.24594
Luo, R., Zhou, B., Liao, P., Zuo, Y., and Jiang, R. (2023). Disrupting cortical astrocyte Ca2+ signaling in developing brain induces social deficits and depressive-like behaviors. Glia 71, 1592–1606. doi: 10.1002/glia.24358
Lyon, K., and Allen, N. (2022). From synapses to circuits, astrocytes regulate behavior. Front. Neural Circuits 15:786293. doi: 10.3389/fncir.2021.786293
Ma, W., Si, T., Wang, Z., Wen, P., Zhu, Z., Liu, Q., et al. (2023). Astrocytic α4-containing nAChR signaling in the hippocampus governs the formation of temporal association memory. Cell Rep. 42:112674. doi: 10.1016/j.celrep.2023.112674
MacVicar, B., and Ko, R. (2017). Mitochondrial calcium sparkles light up astrocytes. Dev. Cell 40, 327–328. doi: 10.1016/j.devcel.2017.02.013
Martin-Fernandez, M., Jamison, S., Robin, L., Zhao, Z., Martin, E. D., Aguilar, J., et al. (2017). Synapse-specific astrocyte gating of amygdala-related behavior. Nat. Neurosci. 20, 1540–1548. doi: 10.1038/nn.4649
McCauley, J., Petroccione, M., D’Brant, L., Todd, G., Affinnih, N., Wisnoski, J., et al. (2020). Circadian modulation of neurons and astrocytes controls synaptic plasticity in hippocampal area CA1. Cell Rep. 33:108255. doi: 10.1016/j.celrep.2020.108255
McCormick, D., Nestvogel, D., and He, B. (2020). Neuromodulation of brain state and behavior. Annu. Rev. Neurosci. 43, 391–415. doi: 10.1146/annurev-neuro-100219-105424
Mendl, M., and Paul, E. (2020). Animal affect and decision-making. Neurosci. Biobehav. Rev. 112, 144–163. doi: 10.1016/j.neubiorev.2020.01.025
Molina-Gonzalez, I., Holloway, R., Jiwaji, Z., Dando, O., Kent, S., Emelianova, K., et al. (2023). Astrocyte-oligodendrocyte interaction regulates central nervous system regeneration. Nat. Commun. 14:3372. doi: 10.1038/s41467-023-39046-8
Mortimer, T., Smith, J., Muñoz-Cánoves, P., and Benitah, S. (2025). Circadian clock communication during homeostasis and ageing. Nat. Rev. Mol. Cell Biol. 26, 314–331. doi: 10.1038/s41580-024-00802-3
Murphy-Royal, C., Dupuis, J., Groc, L., and Oliet, S. (2017). Astroglial glutamate transporters in the brain: Regulating neurotransmitter homeostasis and synaptic transmission. J. Neurosci. Res. 95, 2140–2151. doi: 10.1002/jnr.24029
Nagai, J., Bellafard, A., Qu, Z., Yu, X., Ollivier, M., Gangwani, M., et al. (2021). Specific and behaviorally consequential astrocyte Gq GPCR signaling attenuation in vivo with iβARK. Neuron 109, 2256–2274.e9. doi: 10.1016/j.neuron.2021.05.023
Nimlamool, W., Andrews, R., and Falk, M. (2015). Connexin43 phosphorylation by PKC and MAPK signals VEGF-mediated gap junction internalization. Mol. Biol. Cell 26, 2755–2768. doi: 10.1091/mbc.E14-06-1105
Nizar, K., Uhlirova, H., Tian, P., Saisan, P., Cheng, Q., Reznichenko, L., et al. (2013). In vivo stimulus-induced vasodilation occurs without IP3 receptor activation and may precede astrocytic calcium increase. J. Neurosci. 33, 8411–8422. doi: 10.1523/JNEUROSCI.3285-12.2013
Noh, K., Cho, W., Lee, B., Kim, D., Kim, Y., Park, K., et al. (2023). Cortical astrocytes modulate dominance behavior in male mice by regulating synaptic excitatory and inhibitory balance. Nat. Neurosci. 26, 1541–1554. doi: 10.1038/s41593-023-01406-4
Nutma, E., van Gent, D., Amor, S., and Peferoen, L. (2020). Astrocyte and oligodendrocyte cross-talk in the central nervous system. Cells 9:600. doi: 10.3390/cells9030600
Oberheim, N., Goldman, S., and Nedergaard, M. (2012). Heterogeneity of astrocytic form and function. Methods Mol. Biol. 814, 23–45. doi: 10.1007/978-1-61779-452-0_3
Oberheim, N., Takano, T., Han, X., He, W., Lin, J., Wang, F., et al. (2009). Uniquely hominid features of adult human astrocytes. J. Neurosci. 29, 3276–3287. doi: 10.1523/JNEUROSCI.4707-08.2009
Okubo, Y. (2020). Astrocytic Ca2+ signaling mediated by the endoplasmic reticulum in health and disease. J. Pharmacol. Sci. 144, 83–88. doi: 10.1016/j.jphs.2020.07.006
Oliveira, J., and Araque, A. (2022). Astrocyte regulation of neural circuit activity and network states. Glia 70, 1455–1466. doi: 10.1002/glia.24178
Orts-Del’Immagine, A., Dhanasekar, M., Lejeune, F., Roussel, J., and Wyart, C. (2022). A norepinephrine-dependent glial calcium wave travels in the spinal cord upon acoustovestibular stimuli. Glia 70, 491–507. doi: 10.1002/glia.24118
Ota, Y., Zanetti, A., and Hallock, R. (2013). The role of astrocytes in the regulation of synaptic plasticity and memory formation. Neural Plast. 2013:185463. doi: 10.1155/2013/185463
Parpura, V., and Verkhratsky, A. (2012). Homeostatic function of astrocytes: ca(2+) and Na(+) signalling. Transl. Neurosci. 3, 334–344. doi: 10.2478/s13380-012-0040-y
Peng, W., Liu, X., Ma, G., Wu, Z., Wang, Z., Fei, X., et al. (2023). Adenosine-independent regulation of the sleep-wake cycle by astrocyte activity. Cell Discov. 9:16. doi: 10.1038/s41421-022-00498-9
Péter, M., and Héja, L. (2024). High-frequency imaging reveals synchronised delta- and theta-band Ca2+ oscillations in the astrocytic soma in vivo. Int. J. Mol. Sci. 25:8911. doi: 10.3390/ijms25168911
Petravicz, J., Boyt, K., and McCarthy, K. (2014). Astrocyte IP3R2-dependent Ca(2+) signaling is not a major modulator of neuronal pathways governing behavior. Front. Behav. Neurosci. 8:384. doi: 10.3389/fnbeh.2014.00384
Peyton, L., Haroon, H., Umpierre, A., Essa, H., Bruce, R., Wu, L., et al. (2025). In vivo calcium extrusion from accumbal astrocytes reduces anxiety-like behaviors but increases compulsive-like responses and compulsive ethanol drinking in mice. Neuropharmacology 268:110320. doi: 10.1016/j.neuropharm.2025.110320
Poskanzer, K., and Yuste, R. (2016). Astrocytes regulate cortical state switching in vivo. Proc. Natl. Acad. Sci. U. S. A. 113, E2675–E2684. doi: 10.1073/pnas.1520759113
Qin, H., He, W., Yang, C., Li, J., Jian, T., Liang, S., et al. (2020). Monitoring astrocytic Ca2+ activity in freely behaving mice. Front. Cell Neurosci. 14:603095. doi: 10.3389/fncel.2020.603095
Raber, J., Arzy, S., Bertolus, J., Depue, B., Haas, H., Hofmann, S., et al. (2019). Current understanding of fear learning and memory in humans and animal models and the value of a linguistic approach for analyzing fear learning and memory in humans. Neurosci. Biobehav. Rev. 105, 136–177. doi: 10.1016/j.neubiorev.2019.03.015
Reitman, M., Tse, V., Mi, X., Willoughby, D., Peinado, A., Aivazidis, A., et al. (2023). Norepinephrine links astrocytic activity to regulation of cortical state. Nat. Neurosci. 26, 579–593. doi: 10.1038/s41593-023-01284-w
Rose, C., Ziemens, D., and Verkhratsky, A. (2020). On the special role of NCX in astrocytes: Translating Na+-transients into intracellular Ca2+ signals. Cell Calcium 86:102154. doi: 10.1016/j.ceca.2019.102154
Rosenberg, H., and Rao, M. (2021). Enteric glia in homeostasis and disease: From fundamental biology to human pathology. iScience 24:102863. doi: 10.1016/j.isci.2021.102863
Ross, R., Foster, S., and Ionescu, D. (2017). The role of chronic stress in anxious depression. Chronic Stress 1:2470547016689472. doi: 10.1177/2470547016689472
Ruben, M., and Hogenesch, J. (2017). Circadian rhythms: Move over neurons - astrocytes mediate SCN clock function. Curr. Biol. 27, R350–R352. doi: 10.1016/j.cub.2017.03.055
Rueda-Carrasco, J., Martin-Bermejo, M., Pereyra, G., Mateo, M., Borroto, A., Brosseron, F., et al. (2021). SFRP1 modulates astrocyte-to-microglia crosstalk in acute and chronic neuroinflammation. EMBO Rep. 22:e51696. doi: 10.15252/embr.202051696
Rupprecht, P., Duss, S., Becker, D., Lewis, C., Bohacek, J., and Helmchen, F. (2024). Centripetal integration of past events in hippocampal astrocytes regulated by locus coeruleus. Nat. Neurosci. 27, 927–939. doi: 10.1038/s41593-024-01612-8
Ryu, J., Shim, K., Roh, H., Park, M., Lee, J., and Kim, E. (2024). Circadian regulation of endoplasmic reticulum calcium response in cultured mouse astrocytes. Elife 13:R96357. doi: 10.7554/eLife.96357
Sasaki, T., Ishikawa, T., Abe, R., Nakayama, R., Asada, A., Matsuki, N., et al. (2014). Astrocyte calcium signalling orchestrates neuronal synchronization in organotypic hippocampal slices. J. Physiol. 592, 2771–2783. doi: 10.1113/jphysiol.2014.272864
Scemes, E., and Giaume, C. (2006). Astrocyte calcium waves: What they are and what they do. Glia 54, 716–725. doi: 10.1002/glia.20374
Semyanov, A. (2019). Spatiotemporal pattern of calcium activity in astrocytic network. Cell Calcium 78, 15–25. doi: 10.1016/j.ceca.2018.12.007
Semyanov, A., Henneberger, C., and Agarwal, A. (2020). Making sense of astrocytic calcium signals - from acquisition to interpretation. Nat. Rev. Neurosci. 21, 551–564. doi: 10.1038/s41583-020-0361-8
Serra, I., Esparza, J., Delgado, L., Martín-Monteagudo, C., Puigròs, M., Podlesniy, P., et al. (2022). Ca2+-modulated photoactivatable imaging reveals neuron-astrocyte glutamatergic circuitries within the nucleus accumbens. Nat. Commun. 13:5272. doi: 10.1038/s41467-022-33020-6
Serra, I., Martín-Monteagudo, C., Sánchez Romero, J., Quintanilla, J., Ganchala, D., Arevalo, M., et al. (2025). Astrocyte ensembles manipulated with AstroLight tune cue-motivated behavior. Nat. Neurosci. 28, 616–626. doi: 10.1038/s41593-025-01870-0
Serrat, R., Covelo, A., Kouskoff, V., Delcasso, S., Ruiz-Calvo, A., Chenouard, N., et al. (2022). Astroglial ER-mitochondria calcium transfer mediates endocannabinoid-dependent synaptic integration. Cell Rep. 41:111499. doi: 10.1016/j.celrep.2022.111499
Shah, D., Gsell, W., Wahis, J., Luckett, E., Jamoulle, T., Vermaercke, B., et al. (2022). Astrocyte calcium dysfunction causes early network hyperactivity in Alzheimer’s disease. Cell Rep. 40:111280. doi: 10.1016/j.celrep.2022.111280
Shahaf, G., and Marom, S. (2001). Learning in networks of cortical neurons. J. Neurosci. 21, 8782–8788. doi: 10.1523/JNEUROSCI.21-22-08782.2001
Sharmila, V. (2019). R. S. B. S. K. in Computational Glioscience (Springer Series in Computational Neuroscience ((NEUROSCI)). Cham: Springer.
Sherwood, M., Arizono, M., Panatier, A., Mikoshiba, K., and Oliet, S. (2021). Astrocytic IP3Rs: Beyond IP3R2. Front. Cell Neurosci. 15:695817. doi: 10.3389/fncel.2021.695817
Shigetomi, E., Bushong, E., Haustein, M., Tong, X., Jackson-Weaver, O., Kracun, S., et al. (2013a). Imaging calcium microdomains within entire astrocyte territories and endfeet with GCaMPs expressed using adeno-associated viruses. J. Gen. Physiol. 141, 633–647. doi: 10.1085/jgp.201210949
Shigetomi, E., Jackson-Weaver, O., Huckstepp, R., O’Dell, T., and Khakh, B. (2013b). TRPA1 channels are regulators of astrocyte basal calcium levels and long-term potentiation via constitutive D-serine release. J. Neurosci. 33, 10143–10153. doi: 10.1523/JNEUROSCI.5779-12.2013
Shigetomi, E., Kracun, S., Sofroniew, M., and Khakh, B. S. (2010). A genetically targeted optical sensor to monitor calcium signals in astrocyte processes. Nat. Neurosci. 13, 759–766. doi: 10.1038/nn.2557
Shigetomi, E., Patel, S., and Khakh, B. (2016). Probing the complexities of astrocyte calcium signaling. Trends Cell Biol. 26, 300–312. doi: 10.1016/j.tcb.2016.01.003
Simard, M., and Nedergaard, M. (2004). The neurobiology of glia in the context of water and ion homeostasis. Neuroscience 129, 877–896. doi: 10.1016/j.neuroscience.2004.09.053
Sobolczyk, M., and Boczek, T. (2022). Astrocytic calcium and cAMP in neurodegenerative diseases. Front. Cell Neurosci. 16:889939. doi: 10.3389/fncel.2022.889939
Sofroniew, M. (2021). HepaCAM shapes astrocyte territories, stabilizes gap-junction coupling, and influences neuronal excitability. Neuron 109, 2365–2367. doi: 10.1016/j.neuron.2021.07.010
Solan, J., and Lampe, P. (2014). Specific Cx43 phosphorylation events regulate gap junction turnover in vivo. FEBS Lett. 588, 1423–1429. doi: 10.1016/j.febslet.2014.01.049
Srinivasan, R. (2021). Calcium signals in astrocytes of the fly brain promote sleep. Cell Calcium 94:102341. doi: 10.1016/j.ceca.2020.102341
Srinivasan, R., Huang, B., Venugopal, S., Johnston, A., Chai, H., Zeng, H., et al. (2015). Ca(2+) signaling in astrocytes from Ip3r2(-/-) mice in brain slices and during startle responses in vivo. Nat. Neurosci. 18, 708–717. doi: 10.1038/nn.4001
Stobart, J., Ferrari, K., Barrett, M., Glück, C., Stobart, M., Zuend, M., et al. (2018). Cortical circuit activity evokes rapid astrocyte calcium signals on a similar timescale to neurons. Neuron 98, 726–735.e4. doi: 10.1016/j.neuron.2018.03.050
Sun, W., Liu, Z., Jiang, X., Chen, M., Dong, H., Liu, J., et al. (2024). Spatial transcriptomics reveal neuron-astrocyte synergy in long-term memory. Nature 627, 374–381. doi: 10.1038/s41586-023-07011-6
Suthard, R., Jellinger, A., Surets, M., Shpokayte, M., Pyo, A., Buzharsky, M., et al. (2023a). Chronic Gq activation of ventral hippocampal neurons and astrocytes differentially affects memory and behavior. Neurobiol. Aging 125, 9–31. doi: 10.1016/j.neurobiolaging.2023.01.007
Suthard, R., Senne, R., Buzharsky, M., Pyo, A., Dorst, K., Diep, A., et al. (2023b). Basolateral amygdala astrocytes are engaged by the acquisition and expression of a contextual fear memory. J. Neurosci. 43, 4997–5013. doi: 10.1523/JNEUROSCI.1775-22.2023
Takata, N., Nagai, T., Ozawa, K., Oe, Y., Mikoshiba, K., and Hirase, H. (2013). Cerebral blood flow modulation by Basal forebrain or whisker stimulation can occur independently of large cytosolic Ca2+ signaling in astrocytes. PLoS One 8:e66525. doi: 10.1371/journal.pone.0066525
Tan, C., Burrus Lane, C., and Eroglu, C. (2021). Role of astrocytes in synapse formation and maturation. Curr. Top. Dev. Biol. 142, 371–407. doi: 10.1016/bs.ctdb.2020.12.010
Tewari, B., Woo, A., Prim, C., Chaunsali, L., Patel, D., Kimbrough, I., et al. (2024). Astrocytes require perineuronal nets to maintain synaptic homeostasis in mice. Nat. Neurosci. 27, 1475–1488. doi: 10.1038/s41593-024-01714-3
Toth, A., Hori, K., Novakovic, M., Bernstein, N., Lambot, L., and Prakriya, M. (2019). CRAC channels regulate astrocyte Ca2+ signaling and gliotransmitter release to modulate hippocampal GABAergic transmission. Sci. Signal. 12:eaaw5450. doi: 10.1126/scisignal.aaw5450
Vaidyanathan, T., Collard, M., Yokoyama, S., Reitman, M., and Poskanzer, K. (2021). Cortical astrocytes independently regulate sleep depth and duration via separate GPCR pathways. Elife 10:e63329. doi: 10.7554/eLife.63329
Verkhratsky, A., Reyes, R., and Parpura, V. (2014). TRP channels coordinate ion signalling in astroglia. Rev. Physiol. Biochem. Pharmacol. 166, 1–22. doi: 10.1007/112_2013_15
Wang, F., Wang, W., Gu, S., Qi, D., Smith, N., Peng, W., et al. (2023). Distinct astrocytic modulatory roles in sensory transmission during sleep, wakefulness, and arousal states in freely moving mice. Nat. Commun. 14:2186. doi: 10.1038/s41467-023-37974-z
Wang, Q., Kong, Y., Wu, D., Liu, J., Jie, W., You, Q., et al. (2021). Impaired calcium signaling in astrocytes modulates autism spectrum disorder-like behaviors in mice. Nat. Commun. 12:3321. doi: 10.1038/s41467-021-23843-0
Wang, Y., DelRosso, N., Vaidyanathan, T., Cahill, M., Reitman, M., Pittolo, S., et al. (2019). Accurate quantification of astrocyte and neurotransmitter fluorescence dynamics for single-cell and population-level physiology. Nat. Neurosci. 22, 1936–1944. doi: 10.1038/s41593-019-0492-2
Wang, Y., Fu, A., and Ip, N. (2022). Instructive roles of astrocytes in hippocampal synaptic plasticity: Neuronal activity-dependent regulatory mechanisms. FEBS J. 289, 2202–2218. doi: 10.1111/febs.15878
Whalley, K. (2017). Circadian rhythms: Astrocytes keep time. Nat. Rev. Neurosci. 18:264. doi: 10.1038/nrn.2017.43
Womac, A., Burkeen, J., Neuendorff, N., Earnest, D., and Zoran, M. (2009). Circadian rhythms of extracellular ATP accumulation in suprachiasmatic nucleus cells and cultured astrocytes. Eur. J. Neurosci. 30, 869–876. doi: 10.1111/j.1460-9568.2009.06874.x
Wu, M., Kourdougli, N., and Portera-Cailliau, C. (2024). Network state transitions during cortical development. Nat. Rev. Neurosci. 25, 535–552. doi: 10.1038/s41583-024-00824-y
Wu, Y., Podvalny, E., Levinson, M., and He, B. (2024). Network mechanisms of ongoing brain activity’s influence on conscious visual perception. Nat. Commun. 15:5720. doi: 10.1038/s41467-024-50102-9
Xie, Y., Kuan, A., Wang, W., Herbert, Z., Mosto, O., Olukoya, O., et al. (2022). Astrocyte-neuron crosstalk through Hedgehog signaling mediates cortical synapse development. Cell Rep. 38:110416. doi: 10.1016/j.celrep.2022.110416
Yang, L., Qi, Y., and Yang, Y. (2015). Astrocytes control food intake by inhibiting AGRP neuron activity via adenosine A1 receptors. Cell Rep. 11, 798–807. doi: 10.1016/j.celrep.2015.04.002
Younes, M., Gerardy, B., Pack, A., Kuna, S., Castro-Diehl, C., and Redline, S. (2022). Sleep architecture based on sleep depth and propensity: Patterns in different demographics and sleep disorders and association with health outcomes. Sleep 45:zsac059. doi: 10.1093/sleep/zsac059
Yu, X., Taylor, A., Nagai, J., Golshani, P., Evans, C., Coppola, G., et al. (2018). Reducing astrocyte calcium signaling in vivo alters striatal microcircuits and causes repetitive behavior. Neuron 99, 1170–1187.e9. doi: 10.1016/j.neuron.2018.08.015
Zamora, N., Cheli, V., Santiago González, D., Wan, R., and Paez, P. (2020). Deletion of voltage-gated calcium channels in astrocytes during demyelination reduces brain inflammation and promotes myelin regeneration in mice. J. Neurosci. 40, 3332–3347. doi: 10.1523/JNEUROSCI.1644-19.2020
Zhang, L., Hernandez-Sanchez, D., and Herzog, H. (2019). Regulation of feeding-related behaviors by arcuate neuropeptide Y neurons. Endocrinology 160, 1411–1420. doi: 10.1210/en.2019-00056
Keywords: astrocyte, neuron, behavior, synapse, astrocytic Ca2+ signaling
Citation: Imrie G and Farhy-Tselnicker I (2025) Astrocyte regulation of behavioral outputs: the versatile roles of calcium. Front. Cell. Neurosci. 19:1606265. doi: 10.3389/fncel.2025.1606265
Received: 04 April 2025; Accepted: 25 April 2025;
Published: 15 May 2025.
Edited by:
Annamaria Lia, University of Padua, ItalyReviewed by:
Alessandro Di Spiezio, National Research Council (CNR), ItalyOlga Tiurikova, UCL Queen Square Institute of Neurology, United Kingdom
Copyright © 2025 Imrie and Farhy-Tselnicker. This is an open-access article distributed under the terms of the Creative Commons Attribution License (CC BY). The use, distribution or reproduction in other forums is permitted, provided the original author(s) and the copyright owner(s) are credited and that the original publication in this journal is cited, in accordance with accepted academic practice. No use, distribution or reproduction is permitted which does not comply with these terms.
*Correspondence: Gillian Imrie, Z3JpbXJpZUB0YW11LmVkdQ==; Isabella Farhy-Tselnicker, aWZhcmh5QGJpby50YW11LmVkdQ==