- Sezione INFN and Dipartimento di Fisica G. Occhialini, Universitá Milano Bicocca, Milano, Italy
Detection of photons with scintillating inorganic crystals in the high-energy range (>0.1 MeV) will be discussed, making a comparison with other available methods. Energy resolutions up to 2% at 662 keV and fast decay time of the order of 20 ns are within reach, with the introduction of Ce-doped crystals in place of alkali halide ones. Development is underway for the production of non-hygroscopic scintillating crystals, such as PrLuAg and Ce: GAAG. At the end of this review, examples of experimental devices based on scintillating inorganic crystals will be discussed. Practical hands-on experience is emphasized at the expense of a more comprehensive description of all available and possible options. Detectors’ construction details and consequences of the different choices will be discussed. Emphasis will be put on the
1 Introduction
Photons in the high-energy region (
X-rays were discovered approximately 130 years ago by W.C. Roentgen, by observing the glow on a phosphor screen (Roentgen, 1896). As direct registration of X-rays on a photographic plate is quite inefficient, a search for materials to convert X-rays into visible/ultraviolet (UV) light, to be detected later by a photographic plate, started immediately. Powders such as
Gamma-ray detectors in common use may be divided into three main categories:
• Gas-filled detectors
• Semiconductor crystal detectors
• Inorganic scintillating crystal detectors
The choice depends on the energy range of interest, the needed energy resolution, and the required detection efficiency. In addition, other requirements such as count rate performances, signal pulse shape, and cost may be of relevance.
In the high-energy region (
2 Detectors based on scintillating inorganic crystals
Common materials include sodium iodide (NaI(Tl)), bismuth germanate (BGO), lutetium-yttrium oxy orthosilicate (LYSO), lanthanum bromide (LaBr3:Ce), and many others. Their great importance is associated with their good energy and time resolution, their high counting rate capability (up to
Following are the main characteristics required for a scintillating crystal:
• High material density in the range of 3–7 g/cm3, with high atomic number of the major constituent, to allow high detection efficiency for gamma rays (high “stopping power”).
• Small decay time of the crystal’s fluorescent component providing fast signals, thus allowing high counting rates.
• High light yield, improving the photon statistics and thus the energy resolution.
• Small response nonlinearity, giving a small degradation in energy.
• Chemical stability and radiation hardness.
• Matching of the crystal peak emission wavelength with the photodetector peak response.
The first scintillating crystals used for gamma ray detection were alkali halide ones (such as NaI, NaCl, and NaBr) with thallium as the activator (Pohl, 1938). If the concentration of thallium is small (up to 0.1 molar percent), luminescence is in the near UV region; otherwise, if it is larger (from 0.1 to 5 molar percent), the emission extends to the visible region. A crystal, such as NaI(Tl), is a nonconducting crystal. This means there is a large energy gap between its filled valence band and its empty conduction band. Energetic electrons generated by a gamma interaction with the NaI material will lose their kinetic energy by producing electron–hole pairs. The recombination of these pairs may result in light emission through radiative transitions or energy release as lattice vibrations. The inclusion of thallium at a
In a simple phosphor, the number
where
with
After some preliminary studies on phosphors such as naphthalene (Kallman, 1947), anthracene (Bell, 1948), and calcium tungstate (Moon, 1948), the use of NaI(Tl) crystals for gamma detection was introduced in seminal papers by Hofstader (1948), Hofstader (1949) in the late 40s. They were the crystals of election for many years, having a good photon yield and a reasonable energy resolution even with a long signal decay time (
NaI(Tl) typically converts approximately 11% of the incident gamma energy into photons, with an average energy of 3.0 eV per photon. For a 1 MeV photon, approximately
2.1 Available crystals
A selection of scintillating crystals in current use for gamma ray detection is shown in Table 1, with their main properties.
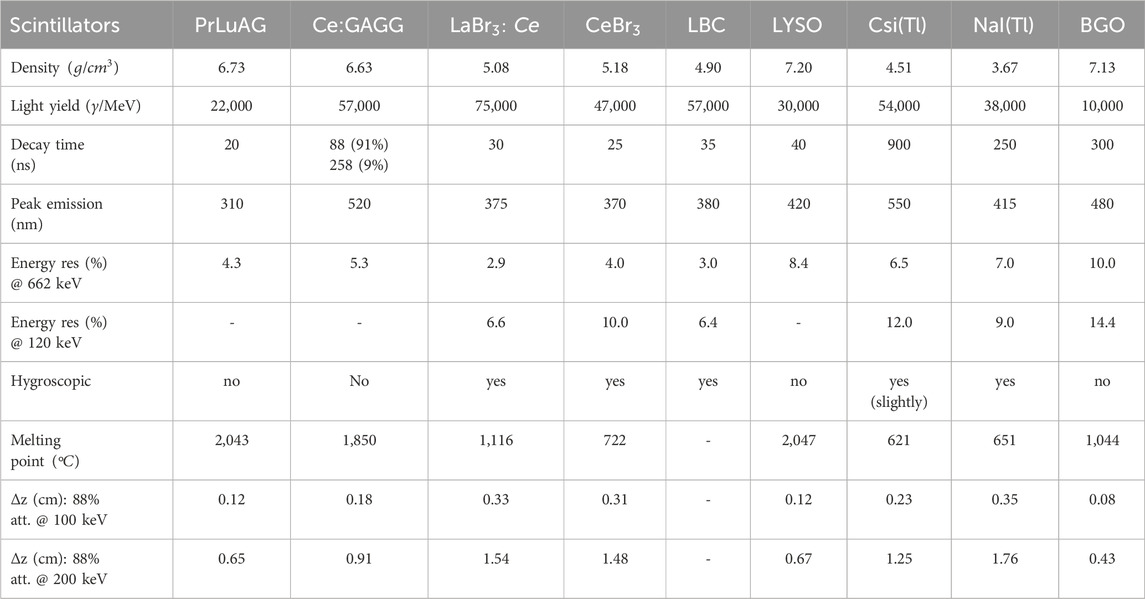
Table 1. Main characteristics of the crystals commonly used for X-ray detection. Typical energy resolutions (FWHM in
Crystals are made from compounds, with a melting point in the typical range 700°C–2,000°C. They can be grown using melt-based methods such as the ones from Bridgman or Czochralski. These methods are suitable for growing large-volume crystals (Brice, 1986).
The first relevant distinction is between hygroscopic crystals, where an encapsulation is needed, and non-hygroscopic ones. Whereas PrLuAG crystals (Drozdowski et al., 2008) and Ce: GAAG crystals (Yeom et al., 2013) are non-hygroscopic and thus do not need encapsulation, the more conventional
NaI(Tl) crystals have been recently superseded by more performant Ce-doped crystals such as
LaBr3: Ce is the crystal of choice for X-ray spectroscopy due to its high light output (
All inorganic crystals based on lanthanum or lutetium suffer from an intrinsic activity, due to the presence of either the 176Lu isotope in naturally occurring lutetium1 or 138La, which emits conversion electrons and
CeBr3 scintillating crystals (Quarati et al., 2007; Fraile, 2013; Ackerman, 2015) offer an alternative to NaI(Tl) for high-resolution gamma ray spectroscopy. With FWHM energy resolution similar to the one of
The new LBC crystals have similar properties to
For a more complete review of the available scintillating inorganic crystals, the reader may refer to the study by Nikl (2006) and Yanagida (2018).
Photon detectors in the high energy range (
2.2 Assembly of crystal-based detectors
Crystals are commonly made in cylindrical or cubic shapes. Usually, only one surface of the scintillator is designed to provide the light output, whereas the others are coated with diffusive or reflecting materials2. This introduces the problem of matching the terminal face or the optical window of the crystal with the surface of the readout device. If SiPM arrays are used, nearly circular sizes may be obtained only by a custom mounting, as done, for example, in the FATIMA experiment (Pascu et al., 2025), using
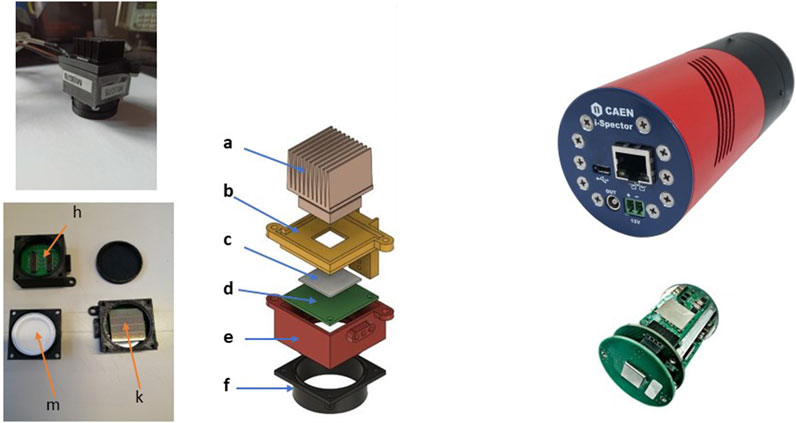
Figure 1. Left panel. Top-left: image of a complete 1″ X-ray detector for the FAMU experiment at RAL. Bottom-left: images of some details of the crystal holder: H) with the printed circuit board (PCB) inside, K) with mounted SiPM array, and M) with crystal inside. Right: exploded view of a 1″ detector. From top to bottom: (a) heat dissipator, (b) detector base, (c) gap filler, (d) PCB, (e) PCB holder, and (f) crystal holder. Right panel. Top-right: image of the i-Spector detector. Bottom-right: exploded inside view of one i-Spector detector. Crystals of different types and sizes may be provided (courtesy of CAEN srl).
Instead, an innovative off-the-shelf solution is the i-Spector detector from CAEN, as shown in the right panel of Figure 1. It is a fully integrated tube designed to replace existing systems based on PMTs. It includes an SiPM array, an amplifier stage, an integrated power supply for biasing of SiPM, and temperature drift correction of SiPM gain.
2.2.1 Optical coupling between scintillating crystals and photodetectors
When the bottom face of a crystal has a similar or equal area to the photodetector’s one, a simple coupling, based on optical glue or optical cement, may be used3. If, instead, there is a large mismatch in the two areas, a light guide is utilized. Such light guides are made of optical quality plexiglass, lucite, or perspex and work on the principle of internal reflection. Light is “guided” from one end to the other via internal reflections between the external walls of the light guide. For this scope, the external walls are polished. As the given flux of light at the input can never be concentrated into a smaller cross-sectional area at the output (Garwin, 1970), other methods, such as Winston cones (Winston, 1970), have to be used, to maximize the collection of incoming rays. A Winston cone is an off-axis parabola of revolution designed to maximize the collection of incoming rays within some field of view. Winston cones are non-imaging light concentrators intended to funnel all wavelengths passing through the entrance aperture out through the exit aperture. They maximize the collection of incoming rays by allowing off-axis rays to make multiple bounces before passing out of the exit aperture.
2.3 Readout techniques
The output signal from scintillating inorganic crystals may be read by different photodetectors such as photomultipliers (PMTs), silicon photomultipliers (SiPMs), silicon avalanche photodiodes (Si-APD), and silicon drift detectors (SDDs). The advantages and disadvantages of the different solutions will be discussed in the following. Their main characteristics are presented in Table 2, where QE is the peak quantum efficiency and
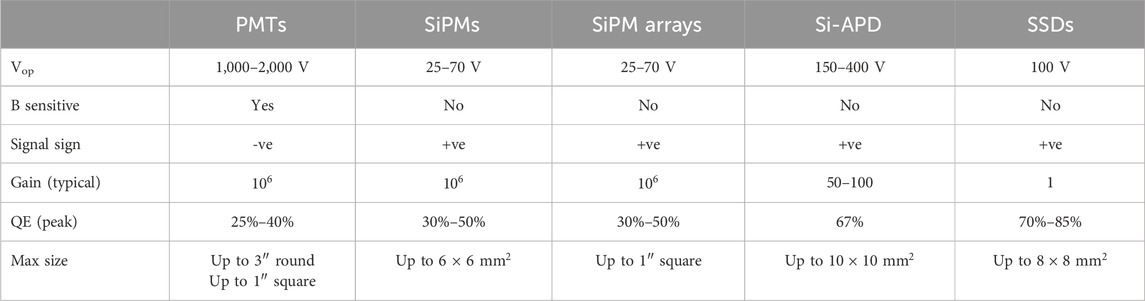
Table 2. Main characteristics of used photodetectors for crystal readout. Typical values are reported.
2.3.1 PMT-based readout
A PMT has been the conventional choice for many years for crystal readout. The main characteristics of some PMTs from Hamamatsu Photonics or Photonis, which are currently in use, are shown in Table 3. When a good energy resolution is needed, PMTs with higher photocathode QE have to be used. Hamamatsu has recently introduced an Ultra BiAlkali (UBA) photocathode (QE
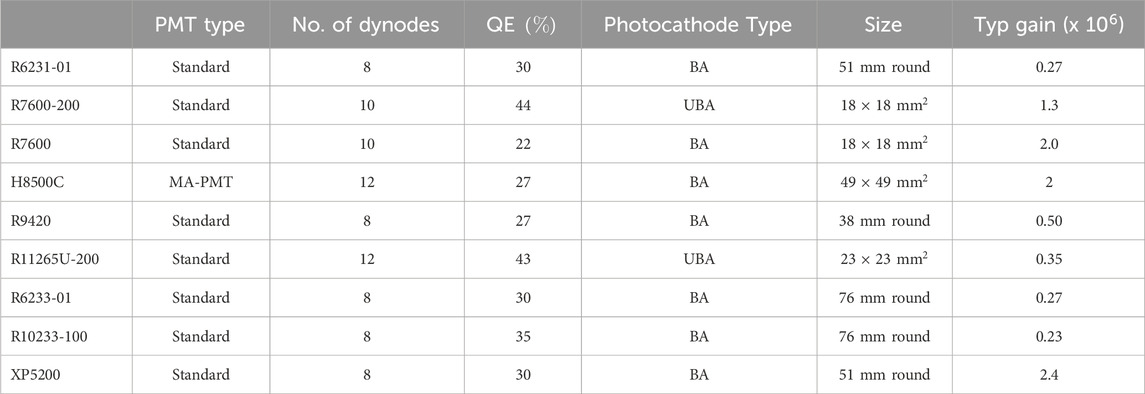
Table 3. Main characteristics of some PMTs from Hamamatsu or Photonis that are in common use for crystal readout.
The use of high photon yield crystals coupled to high-efficiency photocathodes may produce very high peak currents with the dynodes’ current saturation, producing nonlinear effects. To deal with these effects, one may reduce the number of dynodes or use a tapered voltage divider. In this last design, the voltage gradient is enhanced in either the first and/or last few stages. The output linearity is thus improved. Another way to increase the linearity is to use transistors or Zener diodes instead of resistors in the last few stages of the divider. This active divider ensures a good linearity up to an output current of
Examples of LaBr3:Ce detectors with a PMT readout are reported in several studies (Pani et al., 2008; Omer et al., 2013; Cinti et al., 2013; Gandolfo et al., 2023; Baldazzi et al., 2017; Chewpraditke and Moszynski, 2011; Quarati et al., 2007; Swiderski et al., 2015; Giaz et al., 2014; Giaz et al., 2013). Their main characteristics are shown in Table 4.
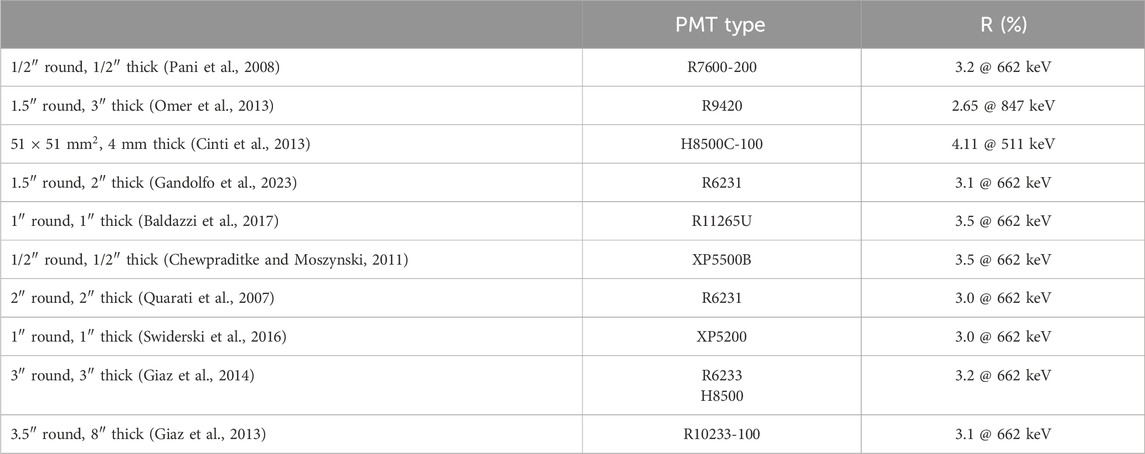
Table 4. Main characteristics of some LaBr3:Ce detectors with PMT readout. R is the FWHM energy resolution.
Whereas
2.3.2 SiPM-based readout
Silicon photomultipliers (SiPMs) are a valuable alternative to conventional photomultipliers (PMTs) for the readout of scintillation detectors4. As readout devices, they have high reliability, low sensitivity to external magnetic fields, and can operate at voltages significantly lower than the ones used for PMTs. Using a SiPM array for the readout of an inorganic scintillating crystal, it is possible to obtain energy resolutions comparable to what is obtained with PMTs. However, SIPMs have a relevant problem: in addition to an increased noise level, their gain drifts significantly as a function of temperature. This feature prevents their use in conditions with a changing temperature, such as homeland security and military applications, unless an ad hoc correction, either offline or online, is implemented.
To read large-area crystals instead of a single SiPM (max area
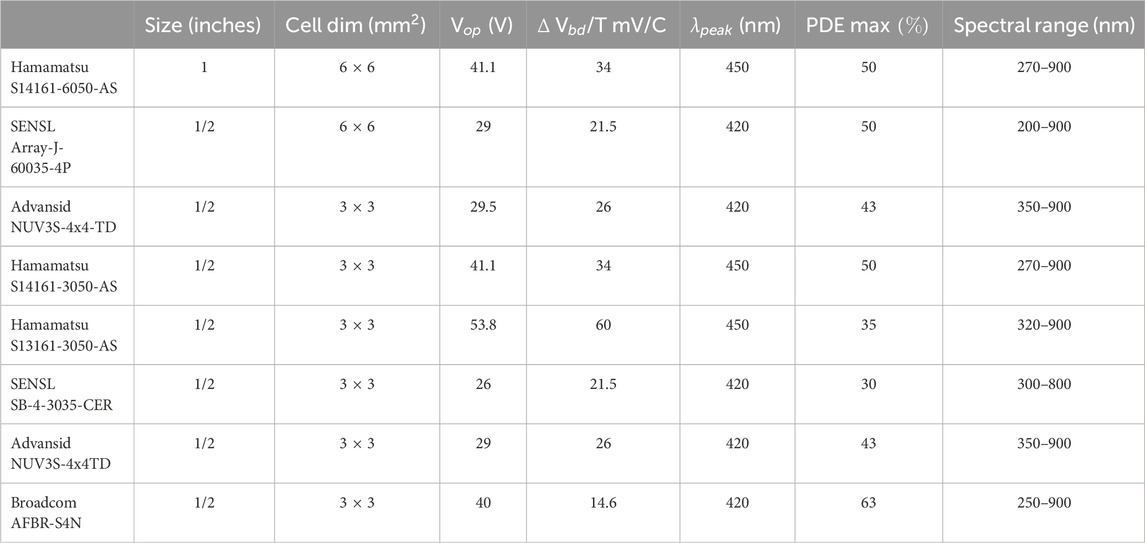
Table 5. Main characteristics of common SiPM 1/2“ and 1” arrays. Data are from producers’ datasheets.
The photon detection efficiency (PDE) of SiPM arrays at different light wavelengths depends also on the type of window used: epoxy or silicone. A more fragile silicone window is used to increase the response at approximately 380 nm (near UV) to match the light emission of
In the case when the signals from the SiPM cells in the readout of an SiPM array are summed up, the different cells may be powered using different schemes (“ganging”). The type of “ganging” used has a relevant influence on the shaping time of the signal (especially the fall time).
A simple processing scheme, based on a flash analog to digital converter (FADC), digitizes the input signal, producing charge, amplitude, and timing informations. This scheme compares well with the one used in a standard spectroscopic chain where the input signal is shaped by a spectroscopic amplifier5 going to a multi-channel analyzer (MCA). In the following section, results with different ganging schemes will be shown, going from standard parallel ganging to hybrid ganging and, finally, to a custom 4–1 scheme developed by Nuclear Instruments.
Examples of LaBr3:Ce detectors with an SiPM array readout are reported in several studies (Divita et al., 2022; Poleshchuck et al., 2021; Bonesini et al., 2023b; Bonesini et al., 2023a; Cozzi et al., 2017; He et al., 2023). Their main characteristics are shown in Table 6.
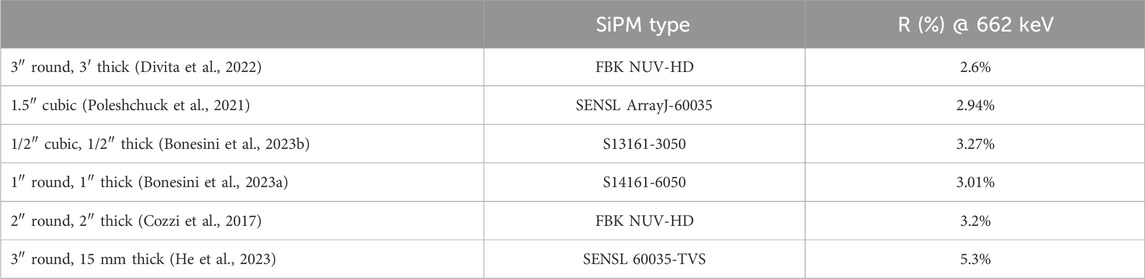
Table 6. Main characteristics of some
2.4.2.1 Correction of SiPM gain drift with temperature
The breakdown voltage
where
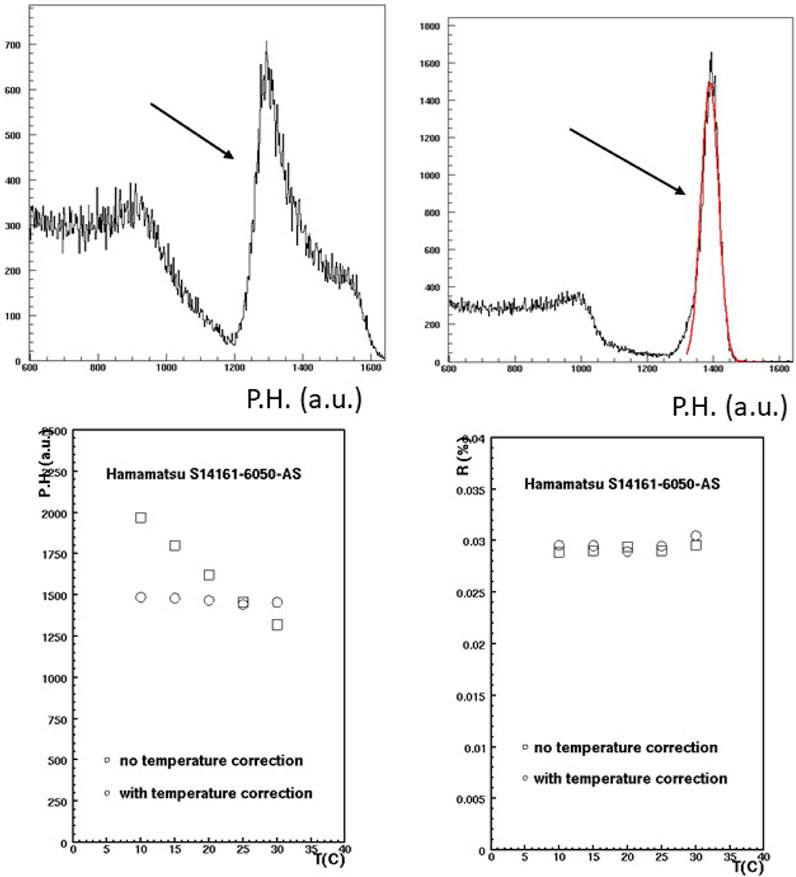
Figure 2. Top panel. Top-left: 137Cs spectra recorded by a
The SiPM gain drift may be corrected by measuring the temperature of the SiPM array and making either an online or an offline correction. In addition to custom solutions, as implemented in previous studies (Kaplan, 2009; Eigen, 2019; Shim et al., 2021; Divita et al., 2022), electronic circuits for SiPM biasing and corrections with temperature are commercially available, as is the A7585 chip from CAEN. In the FAMU experiment at RAL, a solution based on the custom assembly of these chips in an 8-channel NIM module was developed. Temperature T is measured on the back side of the SiPM arrays via Analog Devices TMP37 thermistors to correct the operating voltage online (see Bonesini et al., 2016, and Bonesini et al., 2022a for more details). As shown in Figure 2, the effect on the detector response (pulse height (P.H.) of the 137Cs photopeak in a.u.) between 10°C and 30°C is reduced from 41
2.4.2.2 SiPM ganging schemes
A single SiPM within an SiPM array can be interconnected in various configurations depending on specific requirements, such as speed, signal-to-noise ratio (S/N), and granularity. The top panel of Figure 3 illustrates the different possible conventional configurations.
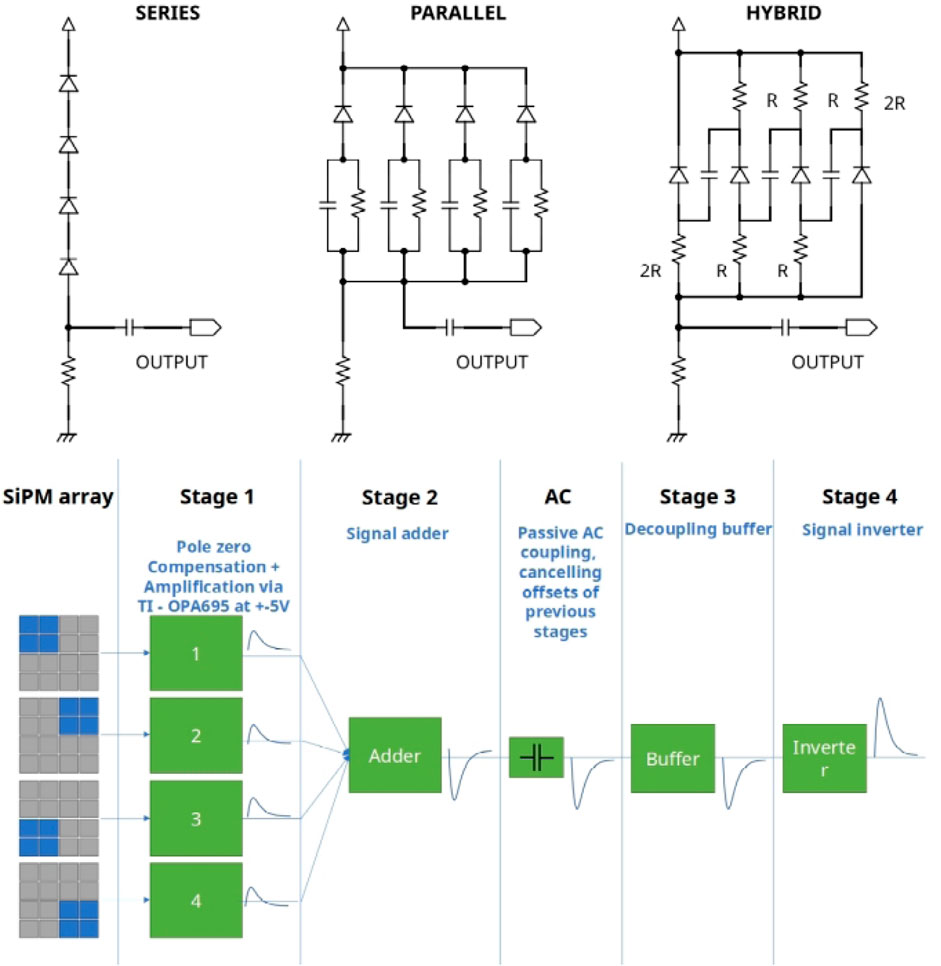
Figure 3. Top panel: layout of different ganging schemes for SiPMs: series ganging, parallel ganging, and hybrid ganging, from the study by Bonesini et al. (2023b). Bottom panel: schematic behavior of the various stages of the Nuclear Instruments 4-1 PCB circuit.
In parallel ganging, the increased capacitance results in slower rise times and longer fall times. Additionally, SiPMs with the same operating voltage
Hybrid ganging combines both series and parallel connections: the SiPMs are connected in series for the signal and in parallel for the bias, with decoupling capacitors placed between them. This configuration, which was originally developed for the MEG II upgrade (Ogawa, 2016), uses a common bias voltage for all the SiPMs.
Taking into account the shape of the produced signal waveforms with the different ganging schemes, the pulse height is nearly equivalent with either a series or a hybrid ganging, whereas it is smaller with a parallel ganging. Instead, time constants are bigger with parallel ganging and shorter with either series or hybrid ganging.
The 4-1 Nuclear Instruments circuit is based on the idea of dividing 1″ square SiPM arrays into four sub-arrays to reduce the capacitance of the single elements, dealing with smaller detectors. In a single sub-array, the ganging is still parallel. As shown in the bottom panel of Figure 3, in the initial stage (stage 1), the signal from each sub-array has a pole-zero compensation stage, followed by an amplification stage via Texas Instruments OPA695 amplifiers. Signals are then added in stage 2. The following stages realize an AC coupling (to cancel offsets) and invert the output signal. For construction details, refer to the studies by Bonesini et al. (2023a) and Bonesini et al. (2023b). Timing and energy resolution results for a typical 1”
Studies are underway to further reduce the fall time of the 4-1 Nuclear Instruments circuit solution. An additional factor of two is expected.
2.3.3 Alternatives readout schemes: Si-APD or silicon drift detectors
Innovative readout schemes for crystals, based on Si-APD or silicon drift detectors (SSDs), have been recently proposed, but their use is still quite limited.
SSDs were invented in 1964 by Gatti and Rehak (1984). They have a lower noise and thus a better energy resolution in principle, as compared to PMTs, smaller mass, and lower power consumption, thus well fitting space applications. Being additionally sensitive to visible light, they may be used for the readout of scintillating inorganic crystals. As an example, in the study by Gangemi et al. (2016), a
The good QE (
Using smaller
2.4 Electronic processing chains
To process the analog signal from a crystal detector, different front-end schemes may be used. In the most simple case, a spectroscopy shaping amplifier is used. The amplifier is then followed by an MCA. If the analog signal is sizeable, it may instead be fed directly into a FADC channel, as shown in the study by Bonesini et al. (2016). For segmented crystal detectors, many engineered processing chips are available, starting from the SPIROC application-specific integrated circuit (ASIC) developed by the OMEGA group in 2007 (Bouchet et al., 2007). With a large dynamic range and variable gain adjustment, it digitized the input information via a 12-bit Wilkinson ADC with a conversion time of
3 Performances of scintilating crystal-based detectors
Performances of a crystal-based detector involve FWHM energy resolution, linearity of the response vs. impinging X-ray energies, and signal timing properties as main properties. Detection efficiency, mainly connected with crystal thickness and density, also has to be considered. These properties depend on the crystal type and the chosen readout scheme. The energy resolution may be written as follows:
where
where
where QE is the quantum efficiency of the photocathode, FF is the filling-factor giving the ratio of the photodetector’s active area to the total area, and THR is the probability of electrons and holes to start the Geiger breakdown. The threshold THR depends on the applied voltage.
Detectors’ linearity and FWHM energy resolution may be studied in a laboratory with calibrated radioactive sources, such as 137Cs, or with X-ray machines.
The linearity and the energy resolution for three common inorganic scintillating crystal detectors with an SiPM array readout are shown in Figure 4. The resolution of LBC is slightly better at low energies with respect to the one of
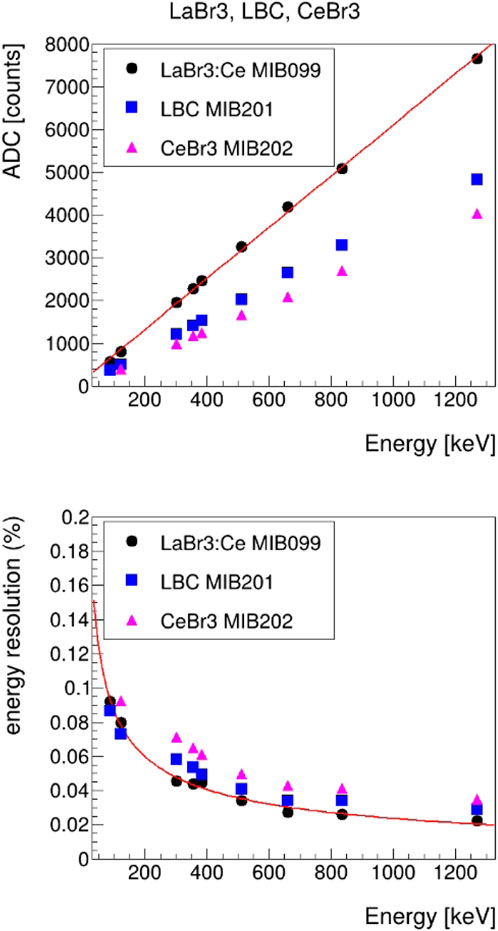
Figure 4. Top panel: linearity for typical 1″ LaBr3:Ce, CeBr3, and LBC crystals read by a Hamamtsu S14161-6050-AS SiPM array. Bottom panel: FWHM energy resolution vs. energy for the same detectors. Energy in keV refers to the incoming photon. Data were obtained in a laboratory with a readout based on a CAEN V1730 fast FADC. Statistical errors are not reported, being smaller than the symbols’ size.
The obtained energy resolution sometimes reduces the effectiveness of the application. This is also due to the limited light collection efficiency. As an example, in a BGO crystal,
A relevant issue for crystal-based detectors is their timing properties. They have a relevant impact on PET where adding TOF information enhances image-to-noise properties (Kuhn et al., 2006) or in experiments, such as FAMU, where a fast signal fall time may enhance the signal (prompt) background (delayed) X-ray separation (Bonesini et al., 2023a). In addition, a high-rate capability is a must in other experiments such as NUMEN (Cappuzzello et al., 2023). The light emission from an inorganic crystal normally follows an exponential decay law:
with
Timing issues are not a problem with a PMT-based readout, where a fast PMT adds a little to the scintillator decay time. In the study by Schaart et al. (2010), a
The use of larger crystals poses more severe problems due to the longer time-walk due to the increased variation of the photon path length with the interaction point (Moses, 2007). Without corrections, an increase of rise time up to
3.1 Comparison with other detector types
The main alternatives to scintillating inorganic crystals are semiconductor detectors. They are based on crystal materials with a few eV band gap. Their operation is based on the direct collection of the charge carriers produced in the intrinsic region of the detector by photon interaction, applying a suitable bias voltage. Their advantage is due to a much better energy resolution. The better intrinsic resolution is due to their small Fano factor and the much smaller ionization energy required: a factor ten times smaller than the one of scintillator detectors. As an example, at 1,173 keV, the energy FWHM resolution is
The drawback of the choice of the HPGe as the detector is the need of cooling the germanium crystal to reduce the intrinsic noise. At 20°C, a 1
Whereas semiconductor detectors, such as HPGe, have superior energy resolution, scintillating crystal-based detectors have better timing performances. Figure 5 from the study by Bonesini et al. (2019) compares typical muonic X-ray spectra taken in the FAMU experiment at RAL, with different types of detectors.
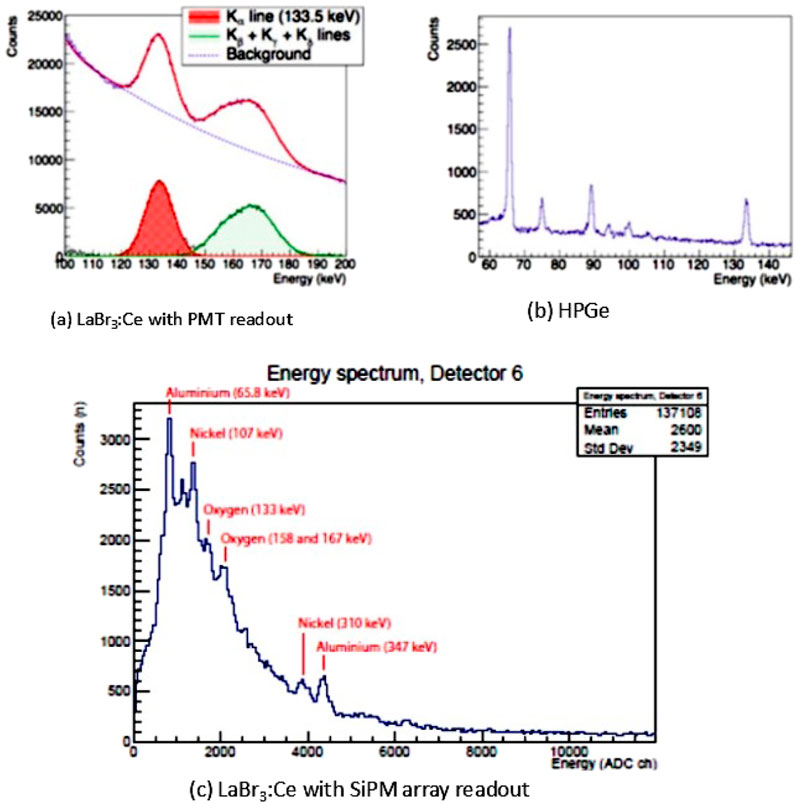
Figure 5. Muonic X-ray spectra recorded in the FAMU experiment at RAL using (a) 1″ LaBr3:Ce counters with PMT readout, where
The better energy resolution of the HPGe detectors, as compared to LaBr3:Ce either with a PMT or an SiPM array readout, is clear. However, the much longer signal fall time of HpGe compared to LaBr3:Ce has prompted the choice of these last detectors in the FAMU experiment to have a better separation between the signal (delayed X-rays) and background (prompt X-rays).
For nuclear spectroscopy at higher energy, CdTe and CdZnTe (CZT) have found increasing applications (Squillante and Entine, 1992; McConnel et al., 2000; Zambelli et al., 2020). Their main advantage is to operate at room temperature, with no need for cooling, and to have a high count-rate capability (up to
3.2 Future improvements
Future improvements are mainly connected to the development of non-hygroscopic crystals, the improvement of
As shown in the study by Yang et al. (2012), with a Sr or Ba co-doping, there is a light output improvement of
4 Examples of crystal-based gamma-ray detection systems
In the following, some gamma-ray detection systems based on scintillating crystals are discussed. They cover the field from fundamental physics: the FAMU experiment at RAL for the study of hyperfine spectroscopy of muonic hydrogen and the NUMEN experiment at INFN LNS, to study
For the FAMU experiment at RAL, a fast detector response (fall time
4.1 The FAMU apparatus
The FAMU (Fisica degli Atomi Muonici) experiment at RIKEN-RAL (Pizzolotto et al., 2020; Adamzack et al., 2018) aims at high-precision spectroscopic studies of muonic hydrogen. In particular, it aims to measure the hyperfine splitting
The signal X-ray detection (around 130 keV) is based on
The FAMU setup for the 2023–2024 data taking is based on one ORTEC GEM-S5020P4 HpGe for inter-calibrations and 34
• Six 1″ round and 1″ thick detectors are read by conventional PMTs (Baldazzi et al., 2017).
• Sixteen 1″ round and 0.5″ thick detectors are read by SiPM arrays (Bonesini et al., 2023a).
• Twelve 1/2″ cubic detectors are read by SiPM arrays (Bonesini et al., 2016).
In the 2024 data taking, the twelve 1/2″ detectors were replaced by 1″ round and 0.5″ thick detectors. An enlargement of the layout of the FAMU experimental setup in the region where X-ray detectors are placed is shown in Figure 6, where the 34
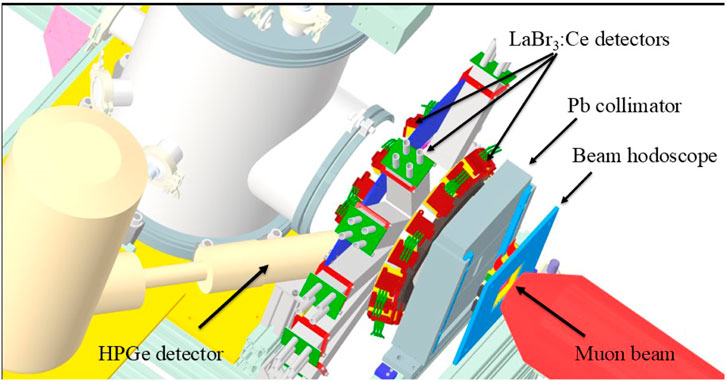
Figure 6. Enlargement of the FAMU experimental setup in the region of X-ray detectors. The three crowns of LaBr3:Ce detectors are shown, together with the HPGe detector.
The detectors with a PMT readout have a fully active divider and a custom digital pulse processor (DPP) based on 12-bit 500 M/s analog devices ADC, as explained in the study by Baldazzi et al. (2017).
Timing and FWHM energy resolution of the three types of detectors are shown in Table 8. FWHM energy resolutions at 137Cs and 57Co peaks are from laboratory measurements, whereas those at 142 keV (Ag peak) are from beam data at RAL with 55 Mev/c impinging muons. For comparison, the FWHM energy resolution at the 142 keV muonic silver peak is

Table 8. Average performances of FAMU
Rise time and fall time are 10–90
4.2 The G-NUMEN apparatus
The G-NUMEN
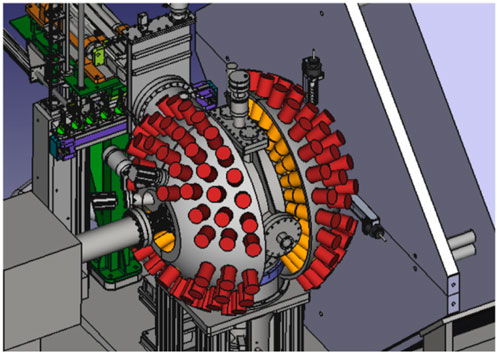
Figure 7. Image of the NUMEN experimental setup in the region of X-rays detectors, from the study by Cappuzzello et al. (2023).
4.3 The GECAM experiment
The Gravitational Wave High-Energy Electromagnetic Counterpart All-Sky Monitor (GECAM) is a Chinese Academy of Sciences project aiming at the detection of the high-energy counterparts of gravitational waves (Feng and Su, 2024). Scientific goals of the GECAM include the detection of gamma-ray bursts (GRBs), solar flares (SGLs), and fast radio bursts (FRBs). Approximately 300 GRBs have been detected by GECAM, including the brightest GDR of all time, that is, the GRB221009A. This GDR was also detected by many other instruments worldwide, but the GECAM was the only one not suffering from signal saturation and pulse pile-up.
The GeCAM includes four instruments, namely, GECAM A/B, GECAM C, and GECAM D, launched between December 2020 and March 2024, equipped with gamma-ray detectors (GDRs) mainly based on 3″ round and 15 mm thick
In-orbit gain variation of SiPMs due to temperature excursions was corrected, reducing nonuniformity from 17% to 0.6%. Due to the presence of background cosmic high-energy protons, an increase in the dark currents of SiPM
5 Conclusion
The field of high-energy X-ray and gamma-ray detectors based on inorganic scintillating crystals is in continuous evolution. The last years have seen a relevant progress in the development of new scintillating crystals. Energy resolutions up to 2% at 662 keV are within reach, and heavy and fast scintillators have been produced. The present development effort is mainly concentrated on the improvement of
However, a full understanding of the finite measured energy resolution of scintillating crystals (below the intrinsic one) has not yet been fully reached, even if there are hints that nonlinearity in the crystal’s response or nonuniformity may be the reason.
Author contributions
MB: conceptualization, funding acquisition, investigation, resources, supervision, validation, visualization, writing – original draft, and writing – review and editing.
Funding
The author(s) declare that financial support was received for the research and/or publication of this article. This research was funded by INFN Commissione 3 under the 2024 funding for the FAMU experiment at RAL.
Acknowledgments
This review is the outcome of a 10-year-old research activity in the FAMU experiment at RAL, where a lot of the shown problems were tackled. The author would like to thank all their colleagues of the FAMU collaboration, in particular Andrea Vacchi, Roberto Bertoni,Ludovico Tortora, and Emiliano Mocchiutti for many interesting discussions and suggestions. In addition, they would like to thank Francesco Caponio and Andrea Abba from Nuclear Instruments srl for many interesting discussions on SiPM readout and M. Saviozzi from CAEN srl for a lot of help on electronics issues.
Conflict of interest
The author declares that the research was conducted in the absence of any commercial or financial relationships that could be construed as a potential conflict of interest.
Generative AI statement
The author(s) declare that no Generative AI was used in the creation of this manuscript.
Publisher’s note
All claims expressed in this article are solely those of the authors and do not necessarily represent those of their affiliated organizations, or those of the publisher, the editors and the reviewers. Any product that may be evaluated in this article, or claim that may be made by its manufacturer, is not guaranteed or endorsed by the publisher.
Footnotes
1
2For crystals emitting aproximately at 310 nm, as PrLuAg, it is difficult to find a proper optical diffuser. The choice in the study by Bonesini et al. (2016) was the Avian-B optical coating, based on
3Bicron BC600 (BC630) is a typical optical cement (grease) used in this case. BC630 has a refractive index of 1.465.
4A SiPM is a set of miniature avalanche photodiodes operating in Geiger mode, connected in parallel. Their outputs are connected to one common output. For a full discussion, see the studies by Buzhan et al. (2003) and Van Dam et al. (2010).
5The Ortec 672 NIM module is a well-known example.
6For good PMTs used in gamma spectroscopy,
7A preliminary study to asses if non-hygroscopic crystals, such as PrLuAg and Ce:GAAG, may be suitable was done, with a negative response, and is reported in the study by Bonesini et al. (2017).
References
Ackerman, U., Ackermann, U., Egger, W., Sperr, P., and Dollinger, G. (2015). Time and energy resolution measurements of BaF2, BC-418, LYSO and CeBr3 scintillators. Nucl. Instr. Meth. A786, 5–11. doi:10.1016/j.nima.2015.03.016
Adamczak, A., Bakalov, D., Stoychev, L., and Vacchi, A. (2012). Hyperfine spectroscopy of muonic hydrogen and the PSI lamb shift experiment. Nucl. Instrum. Methods Phys. Res. Sect. B Beam Interact. Mater. Atoms 281, 72–76. doi:10.1016/j.nimb.2012.04.001
Adamczak, A., Baccolo, G., Banfi, S., Bakalov, D., Baldazzi, G., Benocci, R., et al. (2018). The FAMU experiment at RIKEN-RAL to study the muon transfer rate from hydrogen to other gases. JINST 13, P12033. doi:10.1088/1748-0221/13/12/p12033
Ahmed, S., Blin, R., Callier, S., Cizel, S., Cizel, J. B., Conforti, S., de La Taille, C., et al. (2021). OMEGA SiPM readout ASICs. Nucl. Instr. Meth. A986, 164628. doi:10.1016/j.nima.2020.164628
Alekhin, M., Biner, D. A., Krämer, K. W., and Dorenbos, P. (2013). Improvement of LaBr3: 5 % Ce scintillation properties by Li, Na, Mg, Ca, Sr and Ba co-doping. J. Appl. Phys. 113, 224904. doi:10.1063/1.4810848
Antognini, A., Nez, F., Schuhmann, K., Amaro, F. D., Biraben, F., Cardoso, J. M. R., et al. (2013). Proton structure from the measurement of 2S-2P transition frequencies of muonic hydrogen. Science 339, 417–420. doi:10.1126/science.1230016
Bakalov, D., Milotti, E., Rizzo, C., Vacchi, A., and Zavattini, E. (1993). Experimental method to measure the hyperfine splitting of muomic hydrogen (μ-p)1S. Phys. Lett. A 172, 277–280. doi:10.1016/0375-9601(93)91021-v
Baldazzi, G., Vacchi, A., Labanti, C., Morgante, G., Fuschino, F., Rignanese, L., et al. (2017). The LaBr3:Ce based detection system for the FAMU experiment. JINST 12, C03067. doi:10.1088/1748-0221/12/03/c03067
Baruzzo, M., Suárez-Vargas, J. J., Stoychev, L. I., Cabrera, H., Gadedjisso-Tossou, K. S., Toci, G., et al. (2024). A mid-IR laser source for muonic hydrogen spectroscopy: the FAMU laser system. Opt. Laser Technol. 179, 111375. doi:10.1016/j.optlastec.2024.111375
Bell, P. (1948). The use of Anthracene as a scintillation counter. Phys. Rev. 73, 1405–1406. doi:10.1103/physrev.73.1405.2
Bonesini, M., Bertoni, R., Cervi, T., Clemenza, M., de Bari, A., Mazza, R., et al. (2016). “Laboratory tests for X-rays crystal detectors with SiPM array readout”. IEEE nuclear science symposium, medical imaging conference and room-temperature semiconductor detector workshop (NSS/MIC/RTSD), 1–5. doi:10.1109/NSSMIC.2016.8069925
Bonesini, M., Bertoni, R., Cervi, T., Clemenza, M., de Bar, A., Mazza, R., et al. (2017). Systematic study of innovative hygroscopic and non-hygroscopic crystals with SiPM readout. PoS(EPS-HEP2017), 777. doi:10.22323/1.314.0777
Bonesini, M. (2019). The FAMU experiment at RiKEN RAL. PoS(EPS-HEP2019), 132. doi:10.22323/1.364.0132
Bonesini, M., Benocci, R., Bertoni, R., Clemenza, M., Ghittori, D., Mazza, R., et al. (2020). Ce:LaBr3 crystals with sipm array readout and temperature control for the famu experiment at ral. JINST 15, C05065. doi:10.1088/1748-0221/15/05/c05065
Bonesini, M., Benocci, R., Bertoni, R., Menegolli, A., Prata, M., Rossella, M., et al. (2023a). Large area Labr3:Ce crystals read by SiPM arrays with improved timing and temperature gain drift control. Nucl. Instrum. Methods Phys. Res. Sect. A Accel. Spectrom. Detect. Assoc. Equip. 1046, 167677. doi:10.1016/j.nima.2022.167677
Bonesini, M., Benocci, R., Bertoni, R., Menegolli, A., Prata, M., Rossella, M., et al. (2023b). 1 inch LaBr3:Ce detectors, with temperature control and improved time resolution for low energy X-ray spectroscopy. PoS (EPS-HE2023), 547.
Bonesini, M., Bertoni, R., Abba, A., Caponio, F., Prata, M., and Rossella, M. (2023c). Improving the time resolution of large-area LaBr3:Ce detectors with SiPM array readout. Condens. Matter 8, 99. doi:10.3390/condmat8040099
Bouchel, M., Dulucq, F., Fleury, J., de La Taille, C., Martin-Chassard, G., Raux, L., et al. (2007). SPIROC (SiPM integrated read-out chip). IEEE NSS-MIC, 1857–1860. doi:10.1109/NSSMIC.2007.4436519
Burr, K., and Wang, G. (2007). Scintillation detection using 3 mm × 3 mm silicon photomultipliers. IEEE trans. Nucl. Sci. Conf. Record, 975–982.
Buzhan, P., Dolgoshein, B., Filatov, L., Ilyin, A., Kantzerov, V., Kaplin, V., et al. (2003). Silicon Photomultipliers and its possible applications. Nucl. Instr. Meth. A504, 48–52. doi:10.1016/S0168-9002(03)00749-6
Cappuzzello, F., Acosta, L., Agodi, C., Boztosun, I., Brischetto, G. A., Calabrese, S., et al. (2023). The NUMEN project: an update of the facility towards the future experimentals campaign. Front. Astron, Space Sci. 8, 668587. doi:10.3389/fspas.2021.668587
Carter, L. L., Cashwell, E. D., Everett, C. J, Forest, C. A., Schrandt, R. G, Taylor, W. M., et al. (1975). Monte Carlo development in Los Angeles LA-5903-MS.
Chen, G., and Belbot, M. (2005). Improving energy resolution of scintillation detectors. IEEE Nucl. Sci. Symposium Conf. Rec. 2005 1, 235–238. doi:10.1109/NSSMIC.2005.1596244
Chewpraditke, W., and Moszynski, M. (2011). Scintillation properties of Lu3 Al5 O12, Lu12 SiO5 and LaBr3 crystals activated with Cerium. Phys. Procedia 22, 218–226.
Cinti, M., Pani, R., Pellegrini, R., Bennati, P., Orlandi, C., Fabbri, A., et al. (2013). Spectrometric performances of high quantum efficiency multi and single anode pmts coupled to LaBr3:Ce crystal. Nucl. Instrum. Methods Phys. Res. Sect. A Accel. Spectrom. Detect. Assoc. Equip. 724, 27–33. doi:10.1016/j.nima.2013.04.045
Cozzi, G., Buonanno, L., Busca, P., Carminati, M., Fiorini, C., Montagnani, G. L., et al. (2017). A SiPM-based detection module for 2 LaBr3:Ce readout for nuclear physics applications. 2017 IEEE nuclear science symposium and medical imaging conference NSS/MIC, 1–3. doi:10.1109/NSSMIC.2017.8532888
Dinu, N., Bazin, C, Chaumat, V, Cheikali, C, Para, A, Puill, V, Sylvia, C, and Vagnucci, JF (2010). “Temperature and bias voltage dependence of the MPPC detectors,“. IEEE Nuclear Science Symposuim & Medical Imaging Conference, 215–219. doi:10.1109/nssmic.2010.5873750
Di Vita, D., Buonanno, L., Canclini, F., Ticchi, G., Camera, F., Carminati, M., et al. (2022). A 144-SiPM 3 inches LaBr3 readout module for PMTs replacement in Gamma Spectroscopy. Nucl. Instr. Meth. A1040, 167179. doi:10.1016/j.nima.2022.167179
Dorenbos, P., de Haas, J., and van Eijk, C. (1995). Non-proportionality in the scintillation response and the energy resolution obtainable with scintillation crystals. IEEE Trans. Nucl. Sci. 42, 2190–2202. doi:10.1109/23.489415
Drozdowski, W., Dorenbos, P., de Haas, J. T. M., Drozdowska, R., Owens, A., Kamada, K., et al. (2008). Scintillation properties of praseodymium activated Lu3Al5O12 single crystals. IEEE Trans. Nucl. Sci., 2429. doi:10.1109/TNS.2008.2000845
Du, J., Yang, Y., Bai, X., Judenhofer, M. S., Berg, E., Di, K., et al. (2016). Characterization of large-area SiPM array for PET applications. IEEE Trans. Nucl. Sci. 63, 8–16. doi:10.1109/tns.2015.2499726
Eigen, G. (2019). Gain stabilization of SiPMs and afterpulsing. J.Phys.:Conf. Ser. 1162, 012013. doi:10.1088/1742-6596/1162/1/012013
Feng, P.-Y., and Su, X.-L. (2024). SiPM-based gamma-ray detectors of GECAM. Nucl. Instr. Meth. A1069, 169826. doi:10.1016/j.nima.2024.169826
Fraile, L. M., Mach, H., Vedia, V., Olaizola, B., Paziy, V., Picado, E., et al. (2013). Fast timing study of CeBr3 crystal: time resolution below 120ps at 60Co energies. Nucl. Instr. Meth. A701, 235–242. doi:10.1016/j.nima.2012.11.009
Gandolfo, E., Oliveira, J. R. B., Campajola, L., Pierroutsakou, D., Boiano, A., Agodi, C., et al. (2023). Response of G-NUMEN LaBr3:Ce detectors to high counting rates. Instruments 7, 28. doi:10.3390/instruments7030028
Gangemi, C. (2016). Characterization of a versatile sensitive detector for gamma ray astronomy. Master Thesis Bologna.
Gatti, E., and Rehak, P. (1984). Semiconductor drift chamber. an application of a novel charge transport scheme. Nucl. Instrum. Methods Phys. Res. 225, 608–614. doi:10.1016/0167-5087(84)90113-3
Giaz, A., Pellegri, L., Riboldi, S., Camera, F., Blasi, N., Boiano, C., et al. (2013). Characterization of large volume 3.5 x 8 LaBr3: Ce detectors. Nucl. Instr. Meth. A729, 910–921. doi:10.1016/j.nima.2013.07.084
Giaz, A., Blasi, N., Camera, F., Boiano, C., Brambilla, S., Million, B., et al. (2014). 3 x 3 LaBr3:Ce position sensitivity with multi-anode PMT readout,”. 2014 IEEE Nucl. Sci. Symposium Med. Imaging Conf. (NSS/MIC), 1–5. doi:10.1109/NSSMIC.2014.7431199
Gostojić, A., Tatischeff, V., Kiener, J., Hamadache, C., Peyré, J., Karkour, N., et al. (2016). Characterization of LaBr3:Ce and CeBr3 calorimeter modules for 3d imaging in gamma-ray astronomy. Nucl. Instr. Meth A832, 24. doi:10.1016/j.nima.2016.06.044
Grimm, O., Kim, G., Lee, M., Röser, U., Viertel, G., and von Gunten, H. (2003). A channel photomultiplier with a scintillator faceplate. Nucl. Instrum. Methods Phys. Res. Sect. A Accel. Spectrom. Detect. Assoc. Equip. 513, 644–646. doi:10.1016/j.nima.2003.07.034
Gu, F., Liu, Y., Sun, X., Xu, Y., Zhang, D., An, Z., et al. (2023). Achieving significant performance recovery of SiPM’s irradiation damage with in-situ current annealing. Nucl. Instrum. Methods Phys. Res. Sect. A Accel. Spectrom. Detect. Assoc. Equip. 1053, 168381. doi:10.1016/j.nima.2023.168381
He, J., An, Z. H., Peng, W. X., Li, X. Q., Xiong, S. L., Zhang, D. L., et al. (2023). Ground-based calibration and characterization of LaBr3-SiPM-based gamma-ray detector on GECAM satellite: 8-160 keV. Mon. Notices R. Astronomical Soc. 525, 3399–3412. doi:10.1093/mnras/stad2439
Hofstader, R. (1949). The detection of gamma-rays with thallium activated sodium iodide crystals. Phys. Rev. 796.
Hofstadter, R. (1948). Alkali halide scintillation counters. Phys. Rev. 74, 100–101. doi:10.1103/physrev.74.100
Hubbell, J., and Seltzer, S. (1996). X-ray mass attenuation coefficients. Available online at: https://www.nist.gov/pm/X-ray-mass-attenuation-coefficients.
Iyudin, A., Bogomolov, V. V., Svertilov, S. I., Yashin, I. V., Klassen, N. V., Shmurak, S. Z., et al. (2009). Peculiarities of intrinsic background in LaBr3: Ce and CeBr3 crystals. Instrum. Exp. Tech. 52, 774–781. doi:10.1134/s0020441209060037
Kaplan, A. (2009). Correction of SiPM temperature dependencies. Nucl. Instrum. Methods Phys. Res. Sect. A Accel. Spectrom. Detect. Assoc. Equip. 610, 114–117. doi:10.1016/j.nima.2009.05.137
Kim, C., Wang, G. C., and Dolinsky, S. (2009). Multi-pixel photon counters for TOF PET detector and its challenges. IEEE Trans. Nucl. Sci. 56, 2580–2585. doi:10.1109/tns.2009.2028075
Kuhn, A., Surti, S., Karp, J., Muehllehner, G., Newcomer, F., and VanBerg, R. (2006). Performance assessment of pixelated LaBr3 detector modules for TOF PET. IEEE Trans. Nucl. Sci. 53, 1090–1095. doi:10.1109/tns.2006.873708
Matsuzaki, T., Ishida, K., Nagamine, K., Watanabe, I., Eaton, G., and Williams, W. (2001). The RIKEN RAL pulsed muon facility. Nucl. Instrum. Methods Phys. Res. Sect. A Accel. Spectrom. Detect. Assoc. Equip. 465, 365–383. doi:10.1016/s0168-9002(01)00694-5
McConnell, M. L., Macri, J. R., Ryan, J. M., Larson, K. P., Hamel, L. A., Bernard, G., et al. (2000). Three-dimensional imaging and detection efficiency performance of orthogonal CZT strip detectors. Proc. SPIE 4141, 157–167. doi:10.1117/12.407576
Moon, R. (1948). Inorganic crystals for the detection of high energy particles and quanta. Phys. Rev. 73, 1210. doi:10.1103/physrev.73.1210
Moses, W., and Shah, K. S. (2005). Potential of RbGd2 Br7:Ce, LaBr3:Ce and LuI3:Ce in nuclear medical imaging. Nucl. Instr. Meth. 537, 317. doi:10.1016/j.nima.2004.08.034
Moses, W. (2007). Recent advances and future advances in time-of-flight PET. Nucl. Instrum. Methods Phys. Res. Sect. A Accel. Spectrom. Detect. Assoc. Equip. 580, 919–924. doi:10.1016/j.nima.2007.06.038
Moszyński, M., Zalipska, J., Balcerzyk, M., Kapusta, M., Mengesha, W., Valentine, J. D., et al. (2002). Intrinsic energy resolution of NaI (Tl). Nucl. Instr. Meth. A484, 259–269. doi:10.1016/S0168-9002(01)01964-7
Moszynski, M., Plettner, C., Nassalski, A., Szczesniak, T., Swiderski, L., Syntfeld-Kazuch, A., et al. (2009). “A comparative study with PMTs, avalanche photodiodes, photodiodes and PIN photodiodes in gamma spectroscopy with LaBr3 crystals,”, 56. IEEE Trans. Nucl. Sci., 1006–1011. doi:10.1109/tns.2008.2005110
Nikl, M., Nitsch, K., Polak,, K., Mikokova, E., Dafinei, I., et al. (1996). Slow components in the photoluminescence and scintillation decay of PbWO4 single crystals. Phys. Status Solidi b195, 311–323.
Nikl, M. (2006). Scintillation detectors for x-rays. Meas. Sci. Technol. 17, R37–R54. doi:10.1088/0957-0233/17/4/r01
Omer, M., Ohgaki, H., Negm, H., Daito, I., Hori, T., Kii, T., et al. (2013). “Performance of LaBr3(Ce) array detector system for non-destructive inspection of special nuclear material by using nuclear resonance fluorescence,” in IEEE Int. Conf. Technol. Homel. Secur. (HST), 671–676doi. doi:10.1109/THS.2013.6699084
Otte, A., Garcia, D., Nguyen, T., and Purushotham, D. (2017). Characterization of three high efficiency and blue sensitive silicon photomultipliers. Nucl. Instrum. Methods Phys. Res. Sect. A Accel. Spectrom. Detect. Assoc. Equip. 846, 106–125. doi:10.1016/j.nima.2016.09.053
Pani, R., Cinti, M., Scafe, R., Bennati, P., Pellegrini, R., Vittorini, F., et al. (2008). Gamma-ray spectroscopy with LaBr3:Ce scintillation crystal coupled to an ultra high quantum efficiency pmt. 2008 IEEE Nucl. Sci. Symposium Conf. Rec., 2462–2466. doi:10.1109/nssmic.2008.4774853
Pascu, S., Stoica, A., Neacşu, C., Bruce, A., Costache, C., Das, B., et al. (2025). New readout system of the FATIMA detectors based on silicon photomotipliers arrays. Nucl. Instr. Meth., A17001. doi:10.1016/j.nima.2024.170001
Pizzolotto, C., Adamczak, A., Bakalov, D., Baldazzi, G., Baruzzo, M., Benocci, R., et al. (2020). The FAMU experiment: muonic hydrogen high precision spectroscopy studies. Eur. Phys. J. A 56, 185. doi:10.1140/epja/s10050-020-00195-9
Pohl, R., Antognini, A., Nez, F., Amaro, F. D., Biraben, F., Cardoso, J. M. R., et al. (2010). The size of the proton. Nature 466, 213–216. doi:10.1038/nature09250
Pohl, R. (1938). Zusammen fassender bericht uber elektronenleitung und photochemische vorgang in alkalihalogeneid kristallen. Zeit. Phys. 39, 36.
Poleshchuck, O., Swartz, J. A., Arokiaraj, A., Ceruti, S., De Witte, H., Grinyer, G. F., et al. (2021). Performances tests of a LaBr3:Ce detector coupled to a SiPM array and the GET electronics for γ-ray spectroscopy in a strong magnetic field. Nucl. Istr. Meth. A987, 1648663. doi:10.1016/j.nima.2020.164863
Quarati, F., Bos, A. J. J., Brandenburg, S., Dathy, C., Dorenbos, P., Kraft, S., et al. (2007). X-ray and gamma-ray response of a 2 x 2 LaBr3: Ce scintillation detector. Nucl. Instr. Meth. A574, 115–120. doi:10.1016/j.nima.2007.01.161
Quarati, F., Dorenbos, P., van der Biezen, J., Owens, A., Selle, M., Parthier, L., et al. (2013). Scintillation and detection characteristics of high-sensitivity CeBr3 gamma-ray spectrometers. Nucl. Instrum. Methods Phys. Res. Sect. A Accel. Spectrom. Detect. Assoc. Equip. 729, 596–604. doi:10.1016/j.nima.2013.08.005
Roy, U., Camarda, G. S., Cui, Y., Gul, R., Yang, G., Zazvorka, J., et al. (2019). Evaluation of cdzntese as a high-quality gamma-ray spectroscopic material with better compositional homogeneity and reduced defects. Sci. Rep. 9, 7303. doi:10.1038/s41598-019-43778-3
Scafe, R., Pani, R., Pellegrini, R., Iurlaro, G., Montani, L., and Nerina Cinti, M. (2007). Si-APD readout for LaBr3:Ce scintillator. Nucl. Instrum. Methods Phys. Res. Sect. A Accel. Spectrom. Detect. Assoc. Equip. 571, 355–357. doi:10.1016/j.nima.2006.10.108
Schaart, D. R., Seifert, S., Vinke, R., van Dam, H. T., Dendooven, P., Löhner, H., et al. (2010). LaBr3:Ce and SiPMs for time-of-flight PET: achieving 100 ps coincidence resolving time. Phys. Med. Biol. 55, N179–N189. doi:10.1088/0031-9155/55/7/n02
Shah, K. S., Glodo, J., Klugerman, M., Moses, W. W., Derenzo, S. E., Weber, M. J., et al. (2002). LaBr3:Ce scintillators for gamma ray spectroscopy. LBNL-51793. Available online at: https://www.osti.gov/servlets/purl/894974
Shim, H. S., Park, H., and Lee, J. S. (2021). A temperature-dependent gain compensation technique for positron emission tomography detectors based on a silicon photomultiplier. Phys. Med. Biol. 66, 205015. doi:10.1088/1361-6560/ac2b81
Squillante, M., and Entine, G. (1992). New applications of CdTe nuclear detectors. Nucl. Instrum. Methods Phys. Res. Sect. A Accel. Spectrom. Detect. Assoc. Equip. 322, 569–574. doi:10.1016/0168-9002(92)91234-z
Swiderski, L., Gojska, A., Grodzicka, M., Korolczuk, S., Mianowski, S., Moszynski, M., et al. (2016). Scintillators for high temperature plasma diagnostics. Proc. 1st EPS Conf. Plasma Diagnostics — PoS(ECPD2015), 162. doi:10.22323/1.240.0162
Vacchi, A., Adamczak, A., Andreson, B., Bakalov, D., Battistoni, G., Bhattacharya, N., et al. (2012). “Measuring the size of the proton,” in SPIE NewsRoom. doi:10.1117/2.1201207.004274
van Dam, H., Seifert, S., Vinke, R., Dendooven, P., Lohner, H., Beekman, F. J., et al. (2010). A comprehensive model of the response of silicon photomultipliers. IEEE Trans. Nucl. Sci. 57, 2254–2266. doi:10.1109/tns.2010.2053048
van Loef, E., Dorenbos, P., van Eijk, C. W. E., Krämer, K., and Güdel, H. U. (2001). High resolution scintillator: Ce3+ Activated LaBr3:Ce. Appl. Phys. Lett. 79, 1573–1575. doi:10.1063/1.1385342
Winston, R. (1970). Light collection within the framework of geometrical optics*. J. Opt. Soc. Am. 60, 245–247. doi:10.1364/josa.60.000245
Workman, R. L., Burkert, V. D., Crede, V., Klempt, E., Thoma, U., Tiator, L., et al. (2022). Review of particle physics (PTEP 2022). doi:10.1093/ptep/ptac097
Yanagida, T. (2018). Inorganic scintillating materials and scintillation detectors. Proc. Jpn. Acad. Ser. B94, 75–97. doi:10.2183/pjab.94.007
Yang, K., Menge, P. R., Buzniak, J. J., and Ouspenski, V. (2012). Performance improvement of large Sr2+ and Ba2+ co-doped LaBr3:Ce scintillation crystals. NSS/MIC Symp. Anheim, U. S. A. doi:10.1109/NSSMIC.2012.6551133
Yeom, J., Yamamoto, S., Derenzo, S. E., Spanoudaki, V. C., Kamada, K., Endo, T., et al. (2013). First performance results of Ce:GAGG scintillation crystals with silicon photomultipliers. IEEE Trans. Nucl. Sci. 60, 988–992. doi:10.1109/tns.2012.2233497
Zambelli, N., Zanettini, S., Benassi, G., Bettati, A., and Zappettini, A. (2020). CdZnTe-Based X-ray spectrometer for absolute density determination. IEEE Trans. Nucl. Sci. 67, 2273–2277. doi:10.1109/tns.2020.2996272
Zemach, A. (1956). Proton structure and the hyperfine shift in hydrogen. Phys. Rev. 104, 1771–1781. doi:10.1103/physrev.104.1771
Keywords: gamma rays, muonic X-rays, SiPM, PMT, crystals, LaBr 3:Ce
Citation: Bonesini M (2025) Gamma-ray and high-energy X-ray detection with large-area scintillating crystals: A hands-on review. Front. Detect. Sci. Technol. 3:1551948. doi: 10.3389/fdest.2025.1551948
Received: 26 December 2024; Accepted: 31 March 2025;
Published: 02 May 2025.
Edited by:
Luca Moleri, Weizmann Institute of Science, IsraelReviewed by:
Andrea BRAMBILLA, Commissariat à l’Energie Atomique et aux Energies Alternatives (CEA), FranceDavid Vartsky, Weizmann Institute of Science, Israel
Copyright © 2025 Bonesini. This is an open-access article distributed under the terms of the Creative Commons Attribution License (CC BY). The use, distribution or reproduction in other forums is permitted, provided the original author(s) and the copyright owner(s) are credited and that the original publication in this journal is cited, in accordance with accepted academic practice. No use, distribution or reproduction is permitted which does not comply with these terms.
*Correspondence: Maurizio Bonesini, bWF1cml6aW8uYm9uZXNpbmlAbWliLmluZm4uaXQ=