- 1European Organization for Nuclear Research (CERN), Geneve, Switzerland
- 2Instituto Galego de Física de Altas Enerxías (IGFAE), Universidade de Santiago de Compostela, Santiago de Compostela, Spain
- 3University of Bonn, Bonn, Germany
- 4European Spallation Source (ESS ERIC), Lund, Sweden
Optical readout of micro-pattern gaseous detectors relies on recording scintillation light emitted during electron avalanche multiplication with imaging sensors of high-granularity pixelated readout. It can be used in applications such as optical Time Projection Chambers for track reconstruction, low material budget beam monitoring or radiography, to name but a few. A good match between the scintillation light emission spectra and the spectral sensitivity of the recording devices is required to achieve high signal-to-noise ratios and ensure optimal acquisition parameters. Experimental requirements for operation at low or high pressures may have an impact on the scintillation spectra of gases commonly used for optical readout. We investigate the pressure dependence of scintillation light emission spectra of primary and secondary scintillation in the range of 1,000 mbar down to 25 mbar, in the wavelength range of 200 nm–800 nm. Primary scintillation spectra for different CF4-based mixtures are observed to be independent of pressure in the investigated range. A strong variation in the ratio of ultraviolet (UV) emission to visible (VIS) emission bands is observed as a function of pressure for secondary scintillation of
1 Introduction
The optical readout of gaseous radiation detectors allows to use state-of-the-art imaging sensors to acquire high-granularity images representing incident radiation. MicroPattern Gaseous Detector (MPGD) technologies including Gaseous Electron Multipliers (GEMs) and MicroMesh Gaseous Structures (Micromegas) can be used as amplification structures producing significant scintillation light that is recorded with solid state imaging sensors like CCD and CMOS devices or fast photon detectors including Photomuliplier Tubes (PMTs) or Silicon Photomultipliers (SiPMs) with time resolutions on the order of nanoseconds. Advantages of optical readout include the high pixel count of modern cameras which allows for high spatial resolution imaging, the flexibility to tune magnification and guide light with suitable optical elements including lenses and mirrors, insensitivity to electronic noise and decoupling between readout and amplification structures. On the other hand, the low frame rate on the order of hundreds of Hz to kHz of most imaging sensors limits optical readout with CCD and CMOS sensors to an integrated imaging approach or makes it suitable only for low event rate environments. This limitation is gradually being overcome by the development of novel high-speed CMOS sensors and cameras based on Timepix ASICs. To be compatible with the spectral sensitivity of most commercially available cameras, optical readout is predominantly performed with gas mixtures containing carbon tetra-fluoride (
2 Experimental setup
Spectroscopic measurements of primary and secondary scintillation were performed in two setups: a gas volume with a collimating lens for primary scintillation and a GEM-based TPC for secondary scintillation.
Primary scintillation was recorded by collecting light emitted in a 10 cm thick gas volume with a collimating lens. X-rays from a Cu target X-ray generator operated at 40 kV with 38 mA filament current were used to irradiate the gas volume with high flux. The volume was pumped to base pressures below
The secondary-scintillation spectroscopy setup is shown in Figure 1. A single 10 cm × 10 cm glass GEM is placed in a TPC configuration with a 10 cm diameter, 10 cm-long field shaper and a mesh cathode. The glass GEM features 160 μm–180 µm diameter holes at a pitch of 280 um in a 570 µm-thick photo-etchable glass substrate (Takahashi et al., 2013) and was chosen for operating stably in a wide range of pressures due to the high aspect ratio of its holes which suppresses photon feedback geometrically.
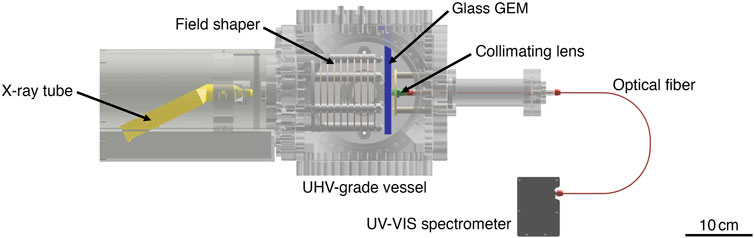
Figure 1. Spectroscopy setup for secondary scintillation measurements: a single glass GEM is used as electron amplification structure in a TPC-like detector setup contained in a UHV-grade gas volume. An X-ray tube is irradiating the chamber through a thin foil window and a mesh cathode from the left. A collimating lens placed below the GEM is collecting scintillation light into an optical fiber. Light is guided through a feedthrough to the UV-VIS spectrometer located outside of the detector volume.
The ‘top’ electrode of the glass GEM (cathode side) was grounded and the bottom electrode (anode side) was powered with a high-voltage supply. Current collected at the bottom electrode was recorded from the power supply and used for normalising the secondary scintillation spectra. A drift field of 50 V/cm was applied between the cathode and the top electrode of the glass GEM. All avalanche electrons were collected on the bottom electrode of the glass GEM.
X-rays from a generator (Amptek Mini-X) were introduced through a thin Al foil window at the cathode side, with a diameter of 1 cm. Spectra were acquired with 20 kV X-ray voltage and 200 µm filament current resulting in primary currents on the scale of 1–10 nA, depending on gas composition and pressure. A lower X-ray flux was required for secondary scintillation measurements compared to the primary scintillation measurements due to the gain of the amplification stage.
A fiber-coupled CCD spectrometer (Ocean Optics FLAME S UV-VIS) with a 200 µm entrance slit was used. Light was coupled with a 5 mm diameter UV-VIS collimating lens and two optical fibers connected with a fiber feedthrough, one vacuum-compatible fiber inside the gas volume and a standard multi-mode fiber outside the gas volume.
For primary and secondary scintillation measurements, spectra were recorded with an exposure time of 30 s and multiple spectra were averaged for each data point. Background spectra were recorded without X-ray irradiation and with the detector powered off. Multiple background spectrum acquisitions were averaged and subtracted from the data.
For primary and secondary scintillation measurements, spectra were corrected for the spectral response and wavelength-dependent transmission of setup elements including the collimating lens, optical fibers and the fiber feedthrough. A UV-VIS-NIR calibration light source (Ocean Optics DH-3plus-CAL) was used to acquire calibration files by introducing light in the spectroscopy setup and separately comparing acquired spectra of deuterium and halogen lamps to nominal lamp output spectra.
3 Scintillation spectra
Scintillation spectra for pure
3.1 Primary scintillation
A comparison of primary scintillation spectra of
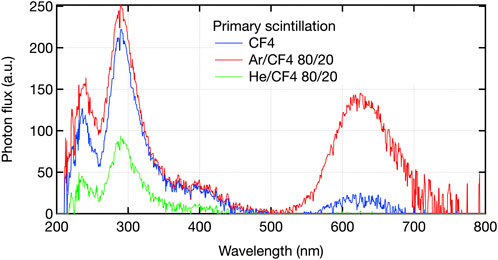
Figure 2. Primary scintillation spectra of
The UV and VIS emission bands of
The primary scintillation spectra of
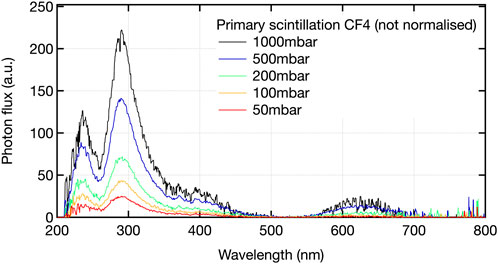
Figure 3. Primary scintillation spectra of pure
The primary scintillation spectra of an Ar/
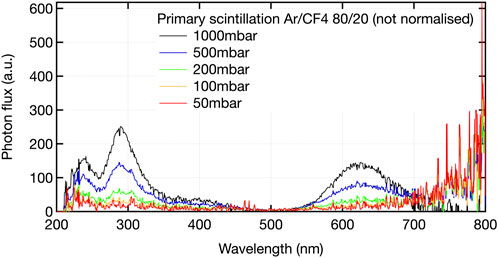
Figure 4. Primary scintillation spectra of Ar/
Primary scintillation spectra of an He/
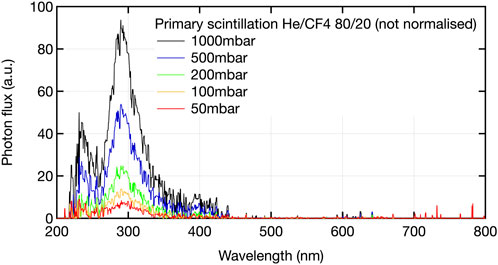
Figure 5. Primary scintillation spectra of He/
The absence of strong pressure dependencies of the spectral shapes in the range corresponding to the
3.2 Secondary scintillation
Secondary scintillation spectra were recorded with a glass GEM in a TPC setup as shown in Figure 1. Spectra are normalised in this case to the total collected current after electron multiplication. The detector was operated at voltages where some saturation of the gain was observed, hinting at possible space-charge effects. The effect of the operation in a regime with some loss of linearity was examined for different drift field strengths from 50 V/cm to 100 V/cm, for GEM gain values from several 10s–1000s and for varying X-ray flux. Operating with different parameters, the normalisation of recorded spectra by collected secondary current exhibited variations of up to 10% while the overall features of the spectra and the relative amplitude of different emission bands and features were not affected.
A comparison of the secondary scintillation spectra of
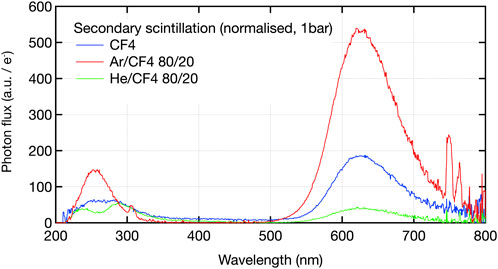
Figure 6. Secondary scintillation spectra of
Secondary scintillation spectra in pure
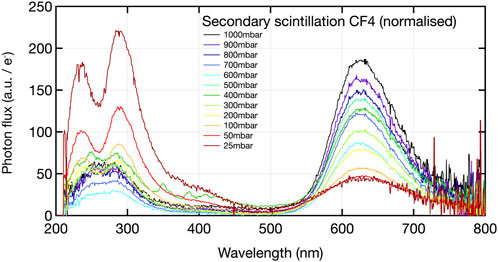
Figure 7. Secondary scintillation spectra of
The above observations can be correlated with the fact that avalanche multiplication at low pressure takes place at higher values of the pressure-reduced electric field, implying higher energy of the electrons within the avalanche. The higher electron energy needed to produce
The observed modifications, specifically the decrease of visible light emission yield, have important implications for detectors operating at low pressures such as the optical TPC used in the MIGDAL experiment operating at 50 torr of pure
In Ar/
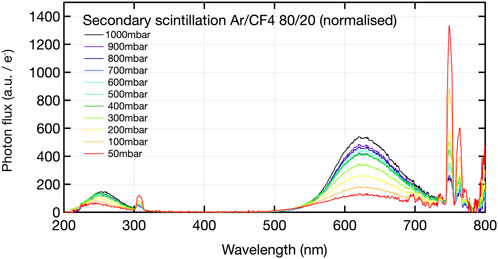
Figure 8. Secondary scintillation spectra of Ar/
The overall scintillation strength in the
Finally it must be noted that a narrow emission peak around 310 nm is observed for all Ar/
Secondary scintillation spectra of He/
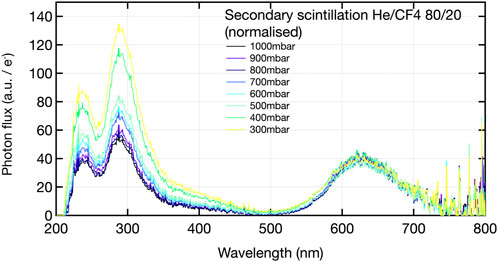
Figure 9. Secondary scintillation spectra of He/
In contrast to the primary scintillation spectra of these gas mixtures as shown in Figure 5, the visible scintillation band of
The trends of the VIS and UV fractions of the total light intensity in
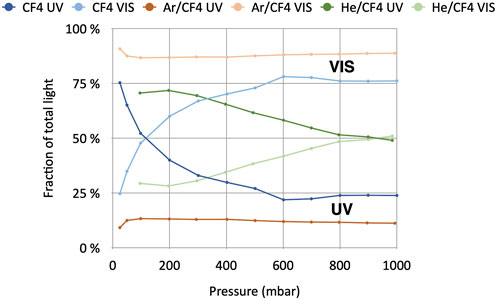
Figure 10. Fraction of UV and VIS emission components of the secondary scintillation spectra of
3.3 Secondary scintillation with negative ion drift
In addition to the electron-drift gas mixtures such as
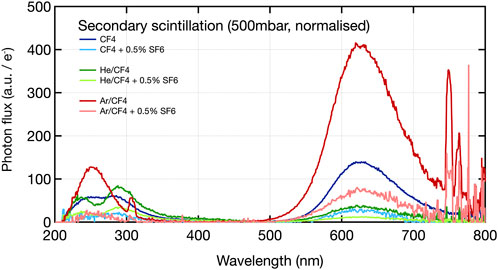
Figure 11. Secondary scintillation spectra of
For all mixtures, the same features are visible in the secondary scintillation spectra with and without
Further and more systematic studies of the impact of the admixture of negative-ion gases to scintillating gas mixtures will be investigated, including the effect of varying pressures and electric field. This is of great relevance in view of the potential of optically read out negative ion TPCs, owing to the decreased diffusion and low drift velocities potentially enabling high-resolution 3D track reconstruction.
4 Conclusion
Primary and secondary scintillation spectra of gas mixtures commonly used for optically read out MPGDs were investigated, in particular their variations with electric field, for sub-atmospheric pressures. While the shapes of the primary scintillation spectra are almost unaffected by pressure variations, significant spectral modifications were found for secondary scintillation spectra, most importantly altering the ratios of the UV/VIS scintillation, that is crucial for optical readout. The general trend observed, for both
In addition, the impact on the scintillation spectra of adding a small amount of
The presented study for
Data availability statement
The raw data supporting the conclusions of this article will be made available by the authors, without undue reservation.
Author contributions
FB: Conceptualization, Data curation, Formal Analysis, Investigation, Methodology, Supervision, Validation, Visualization, Writing – original draft, Writing – review and editing. PA: Conceptualization, Investigation, Writing – review and editing. KF: Conceptualization, Investigation, Writing – review and editing. DD: Conceptualization, Investigation, Supervision, Writing – review and editing. DJ: Conceptualization, Investigation, Writing – review and editing. SL: Conceptualization, Data curation, Investigation, Writing – review and editing. ML: Conceptualization, Investigation, Writing – review and editing. HM: Conceptualization, Writing – review and editing. EO: Conceptualization, Supervision, Writing – review and editing. GO: Writing – review and editing. DP: Writing – review and editing. LR: Conceptualization, Supervision, Writing – review and editing. FS: Conceptualization, Writing – review and editing. JS: Conceptualization, Writing – review and editing. LS: Conceptualization, Writing – review and editing. MV: Conceptualization, Writing – review and editing. RV: Conceptualization, Writing – review and editing.
Funding
The author(s) declare that no financial support was received for the research and/or publication of this article.
Conflict of interest
The authors declare that the research was conducted in the absence of any commercial or financial relationships that could be construed as a potential conflict of interest.
The author(s) declared that they were an editorial board member of Frontiers, at the time of submission. This had no impact on the peer review process and the final decision.
Generative AI statement
The author(s) declare that no Generative AI was used in the creation of this manuscript.
Publisher’s note
All claims expressed in this article are solely those of the authors and do not necessarily represent those of their affiliated organizations, or those of the publisher, the editors and the reviewers. Any product that may be evaluated in this article, or claim that may be made by its manufacturer, is not guaranteed or endorsed by the publisher.
References
Amaro, F., Baracchini, E., Benussi, L., Bianco, S., Borra, F., Capoccia, C., et al. (2024). Charge amplification in low pressure cf4:sf6:he mixtures with a multi-mesh thgem for directional dark matter searches. J. Instrum. 19, P06021. doi:10.1088/1748-0221/19/06/P06021
Amaro, F. D., Baracchini, E., Benussi, L., Bianco, S., Capoccia, C., Caponero, M., et al. (2022). The CYGNO experiment. Instruments 6, 6. doi:10.3390/instruments6010006
Amedo, P., González-Díaz, D., Brunbauer, F. M., Fernández-Posada, D. J., Oliveri, E., and Ropelewski, L. (2023). Observation of strong wavelength-shifting in the argon-tetrafluoromethane system. Front. Detect. Sci. Technol. 1. doi:10.3389/fdest.2023.1282854
Araújo, H. m., Balashov, S., Borg, J., Brunbauer, F., Cazzaniga, C., Frost, C., et al. (2023). The MIGDAL experiment: measuring a rare atomic process to aid the search for dark matter. Astropart. Phys. 151, 102853. doi:10.1016/j.astropartphys.2023.102853
Brunbauer, F. M., Garcia, F., Korkalainen, T., Lugstein, A., Lupberger, M., Oliveri, E., et al. (2018). Combined optical and electronic readout for event reconstruction in a GEM-based TPC. IEEE Trans. Nucl. Sci. 65, 913–918. doi:10.1109/TNS.2018.2800775
Fanelli, F., Fracassi, F., and d’Agostino, R. (2008). Fluorination of polymers by means of He/CF4-fed atmospheric pressure glow dielectric barrier discharges. Plasma Process. Polym. 5, 424–432. doi:10.1002/ppap.200800012
Fraga, M., Fraga, F., Fetal, S., Margato, L., Marques, R., and Policarpo, A. (2003). The GEM scintillation in He–CF4, Ar–CF4, Ar–TEA and Xe–TEA mixtures. Nucl. Instrum. Methods Phys. Res. Sect. A Accel. Spectrom. Detect. Assoc. Equip. 504, 88–92. doi:10.1016/S0168-9002(03)00758-7
Ikeda, T., Shimada, T., Ishiura, H., Nakamura, K., Nakamura, T., and Miuchi, K. (2020). Development of a negative ion micro tpc detector with sf6 gas for the directional dark matter search. J. Instrum. 15, P07015. doi:10.1088/1748-0221/15/07/P07015
Lambert, I. R., Mason, S. M., Tuckett, R. P., and Hopkirk, A. (1988). Decay pathways of excited electronic states of Group IV tetrafluoro and tetrachloro molecular ions studied with synchrotron radiation. J. Chem. Phys. 89, 2683–2690. doi:10.1063/1.455019
Ligtenberg, C., van Beuzekom, M., Bilevych, Y., Desch, K., van der Graaf, H., Hartjes, F., et al. (2021). On the properties of a negative-ion tpc prototype with gridpix readout. Nucl. Instrum. Methods Phys. Res. Sect. A Accel. Spectrom. Detect. Assoc. Equip. 1014, 165706. doi:10.1016/j.nima.2021.165706
Martoff, C., Snowden-Ifft, D., Ohnuki, T., Spooner, N., and Lehner, M. (2000). Suppressing drift chamber diffusion without magnetic field. Nucl. Instrum. Methods Phys. Res. Sect. A Accel. Spectrom. Detect. Assoc. Equip. 440, 355–359. doi:10.1016/S0168-9002(99)00955-9
Mašláni, A., and Sember, V. (2014). Emission spectroscopy of OH radical in water-argon arc plasma jet. J. Spectrosc. 2014, 1–6. doi:10.1155/2014/952138
Morozov, A., Fraga, M., Pereira, L., Margato, L., Fetal, S., Guerard, B., et al. (2010). Photon yield for ultraviolet and visible emission from CF4 excited with -particles. Nucl. Instrum. Methods Phys. Res. Sect. B Beam Interact. Mater. Atoms 268, 1456–1459. doi:10.1016/j.nimb.2010.01.012
Morozov, A., Fraga, M., Pereira, L., Margato, L., Fetal, S., Guerard, B., et al. (2011). Effect of the electric field on the primary scintillation from CF4. Nucl. Instrum. Methods Phys. Res. Sect. A Accel. Spectrom. Detect. Assoc. Equip. 628, 360–363. doi:10.1016/j.nima.2010.07.001
Ohnuki, T., Snowden-Ifft, D. P., and Martoff, C. (2001). Measurement of carbon disulfide anion diffusion in a tpc. Nucl. Instrum. Methods Phys. Res. Sect. A Accel. Spectrom. Detect. Assoc. Equip. 463, 142–148. doi:10.1016/S0168-9002(01)00222-4
Phan, N., Lafler, R., Lauer, R., Lee, E., Loomba, D., Matthews, J., et al. (2017). The novel properties of sf6 for directional dark matter experiments. J. Instrum. 12, P02012. doi:10.1088/1748-0221/12/02/P02012
Keywords: gaseous detector, scintillation, optical readout, MPGD (Micropattern gaseous detector), CF4
Citation: Brunbauer FM, Amedo P, Flöthner KJ, Gonzalez Diaz D, Janssens D, Leardini S, Lisowska M, Müller H, Oliveri E, Orlandini G, Pfeiffer D, Ropelewski L, Sauli F, Samarati J, Scharenberg L, van Stenis M and Veenhof R (2025) Primary and secondary scintillation of CF4-based mixtures in low-pressure gaseous detectors. Front. Detect. Sci. Technol. 3:1561739. doi: 10.3389/fdest.2025.1561739
Received: 16 January 2025; Accepted: 10 April 2025;
Published: 22 April 2025.
Edited by:
Gabriele Croci, University of Milano-Bicocca, ItalyReviewed by:
Giorgio Dho, National Laboratory of Frascati (INFN), ItalyStefano Levorato, National Institute of Nuclear Physics of Trieste, Italy
Copyright © 2025 Brunbauer, Amedo, Flöthner, Gonzalez Diaz, Janssens, Leardini, Lisowska, Müller, Oliveri, Orlandini, Pfeiffer, Ropelewski, Sauli, Samarati, Scharenberg, van Stenis and Veenhof. This is an open-access article distributed under the terms of the Creative Commons Attribution License (CC BY). The use, distribution or reproduction in other forums is permitted, provided the original author(s) and the copyright owner(s) are credited and that the original publication in this journal is cited, in accordance with accepted academic practice. No use, distribution or reproduction is permitted which does not comply with these terms.
*Correspondence: F. M. Brunbauer, Zmxvcmlhbi5icnVuYmF1ZXJAY2Vybi5jaA==