- 1Department of Chemistry, Maseno University, Maseno, Kenya
- 2Department of Chemistry, Faculty of Sciences, University of Douala, Douala, Cameroon
- 3Department of Physical Sciences, Kaimosi Friends University, Kaimosi, Kenya
The potent of watermelon based adsorbent for the elimination of various pollutants from water was reviewed. This study shows that watermelon-based adsorbents offer a cost effective and environmentally friendly adsorbent for the removal of many pollutants including dyes, heavy metals and nutrients. The main functional groups responsible for the binding properties of raw watermelon rind are the hydroxyl (–OH), carboxyl (–COOH), carbonyl (-C=O-) and amines (-NH2). Equilibrium adsorption data largely followed the Langmuir isotherm, whereas the kinetic data for both dyes and heavy metals mostly fitted to the pseudo-second order kinetic model. This notwithstanding, very few studies reported the use of natural wastewaters to gain insight into the potential for real world application. Again, the review noted an overreliance on the isotherms, kinetic and thermodynamic data in predicting the mechanism of adsorption. Nonetheless, watermelon rind based adsorbents offer an effective removal means for many pollutants, calling for further exploration, with future research likely to focus on overcoming the aforementioned limitations.
1 Introduction
The access to clean and consumable water remains an interminable global challenge. According to the UN water development report of 2024, about 2.2 billion people world over lack access to safe drinking water, a situation exacerbated by population growth and increasing industrialization (UNESCO, 2024). Water pollution is one the causes of shortage of safe drinking water. It is estimated that up to 20% of pollutants such as dyes and heavy metals among other pollutants are disposed directly into wastewaters (Gupta, 2009; Kyzas and Kostoglou, 2014). When pollutants exceed tolerance limits in water, they may cause detrimental health complications in humans. Amongst the various methods employed in wastewater treatment is adsorption. This is because of its simplicity, relatively high removal efficiencies and ready availability of required resources (Crini et al., 2019). Conventional adsorbents such as coal-based activated carbon have been widely used due to their microporous structure and high surface area. However, their usage is hindered by high operation costs, difficulty in recovery from aqueous systems and regeneration losses (Sankaran et al., 2020). These has necessitated the search for eco-friendly and sustainable adsorbent materials with wide scale applicability such as bioadsorbents.
Biosorption technology has proved to be a suitable alternative as a sustainable wastewater treatment approach due to the abundantly available variety of biomass. Apart from possessing relatively high efficiency, bioadsorbents are cost effective, ecofriendly and biodegradable adsorbents derived from agricultural wastes, microbial biomass and biopolymers. Agricultural wastes such as rice husks (Tome et al., 2023), coconut shell (Babel and Kurniawan, 2004; Song et al., 2013), banana peels (Dilekoglu, 2016), sugarcane bagasse (Shikuku and Jemutai-Kimosop, 2020) and watermelon rinds (Wanja et al., 2016) have been used as adsorbents for various pollutants including heavy metals and organic pollutants, majorly dyes. The application of agricultural waste presents the dual functionality of agricultural waste management and elimination of water pollutants.
The estimated global production of watermelon fruit in the year 2023 was 99.7 million tons, led by China at 60.4 million tons and followed by Turkiye at 3.5 million tons (Gorgulu and Gorgulu, 2025). About 33% of the watermelon fruit is the rind, which is discarded to the environment as solid food waste without further treatment and may cause environmental pollution. The rind has been put into use as a bio-fertilizer (Erugo et al., 2022), animal feeds (Ekloh and Yafetto, 2024) and food ingredients (Gorgulu and Gorgulu, 2025). Efforts have also been made towards high value utilization of this carbon rich material. As a source of carbon and nitrogen skeleton, WMR has been used to develop low cost catalysts (Jiang et al., 2022), super capacitor electrodes (Omar et al., 2022), and as adsorbent materials for wastewater remediation (Taib et al., 2021).
Watermelon rind is comprised of high cellulose and lignin content offering a plethora of functional groups including hydroxyl (-OH), amines (-NH2) and carboxylic (-COOH) groups (Wu et al., 2012; Chen et al., 2017; Masoudian et al., 2019; El-Shafie et al., 2021). Furthermore, some textural properties of watermelon rind, particularly its high porosity, enhances its adsorptive characteristics making it a potential adsorbent in wastewater treatment. In addition, watermelon rind is an ecofriendly material owing to its biodegradability, rationalizing its selection for use as an adsorbent. However, in its unmodified form WMR has limited adsorptive capabilities due to low specific surface areas (Dai et al., 2018; Santoso et al., 2020). Chemical and physical modifications have therefore, been employed to enhance the adsorption capacities of pristine WMR adsorbents but this involves some inherent costs and may also introduce toxic chemical additives posing a threat to the environment. Studies, therefore should be focused on overcoming these limitations through the development of green synthesis processes and biodegradable adsorbents.
In addition, watermelon rind based adsorbents compared to similar biomass based adsorbents such as orange peel, banana peels, pomelo peel and dragon fruit peel for the removal of both heavy metals and dyes shows remarkable adsorption capabilities (Table 1). Furthermore, watermelon rind based adsorbents show better removal efficiency for cationic dyes such as methylene blue, compared to anionic dyes and heavy metal cations. This has been attributed to the significant amount of negatively charged functional groups on the surface of raw watermelon rind, sustaining electrostatic interaction with cationic dye molecules. The results further suggest that watermelon rind in its raw form is an effective material for the removal of many pollutants including toxic heavy metals (Table 2) and organic pollutants such as dyes.
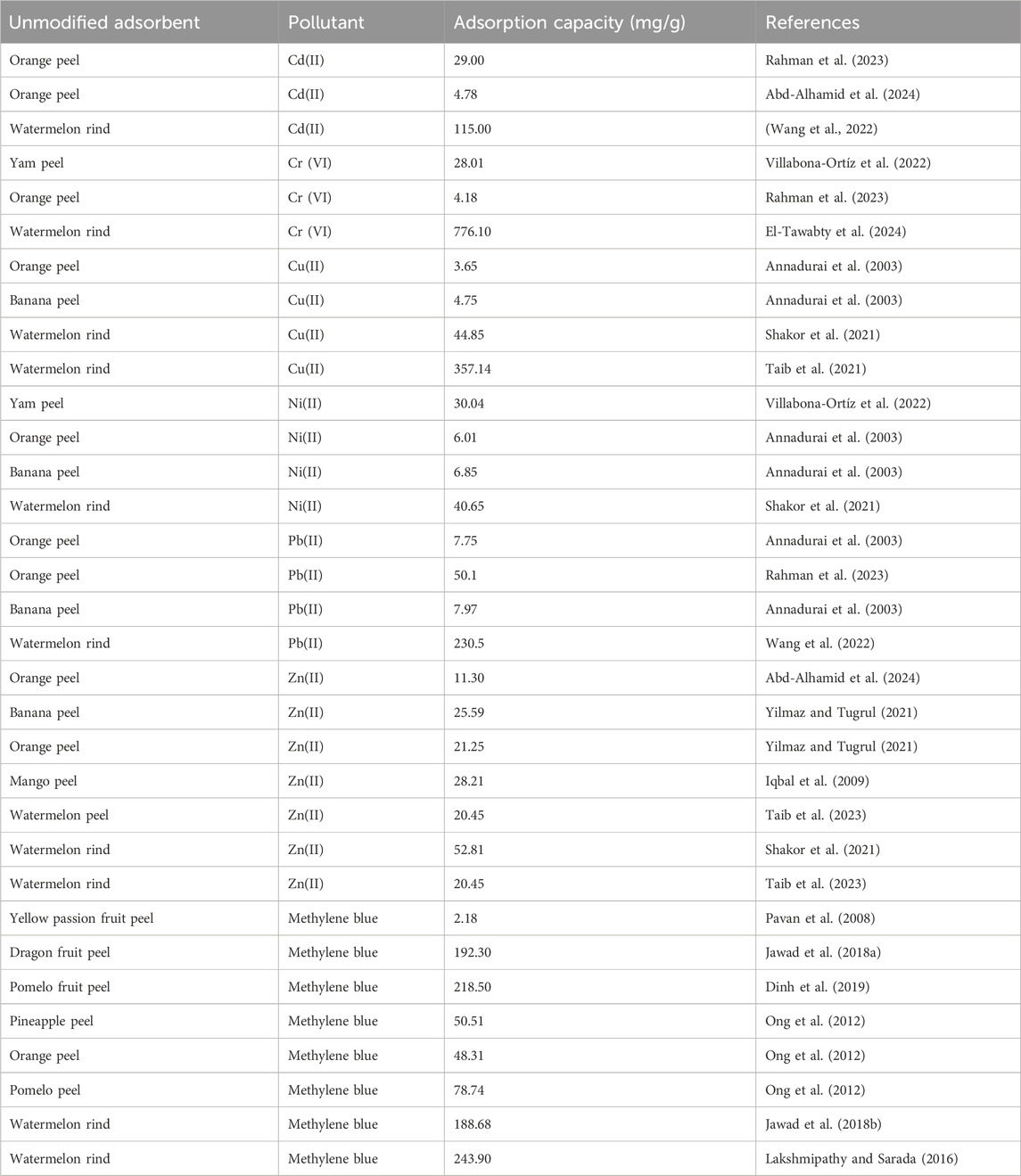
Table 1. A comparison of the adsorptive performance of watermelon rind and similar biomass based adsorbents for the removal of heavy metal ions and dyes.
A summary of the number of publications (Figure 1) shows that the study of watermelon rind based adsorbents has been on the increase over the past decade. This comprehensive review therefore examines the application of watermelon rind-based adsorbents for the adsorption of pollutants from aqueous systems. The biosorption mechanism of WMR and modification of watermelon rind-based adsorbents, application of the adsorbents for the removal of heavy metal ions and dyes, and the associated adsorption conditions, regeneration and reusability of the adsorbents shall be discussed. An overview of environmental and economic feasibility, challenges and limitations and the future perspectives is also provided.
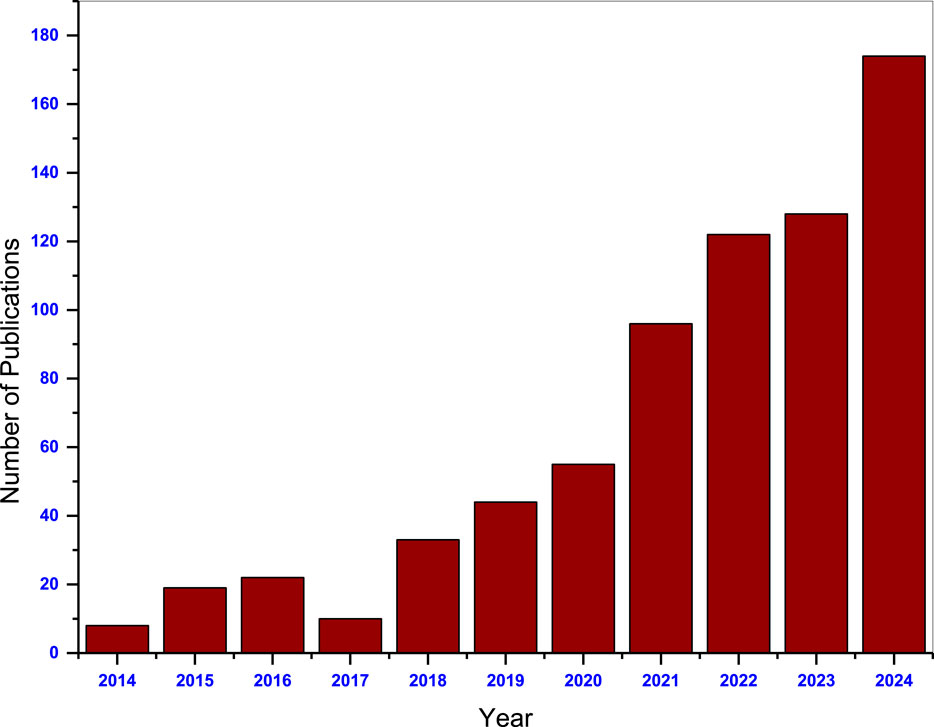
Figure 1. Records of the number of publications in indexed journals containing the key words “watermelon peel” and “adsorbents” between 2014 and 2024. (Source: ScienceDirect). Searched on 10 December 2024.
2 Biosorption mechanism
The mechanism of pollutant uptake is dependent on both the nature of the adsorbate and the surface chemistry of the adsorbent. The mechanism of adsorption can either be a physical process (physisorption), a chemical process (chemisorption) or a combination of both processes (Dąbrowski, 2001). Physisorption often involve weak van der Waal’s forces of attraction, low adsorption energies, generally reversible and is multilayer in nature. On the other hand, chemisorption involves the formation of strong ionic, covalent or hydrogen bonds, is generally irreversible and is a monolayer formation. Adsorption isotherms, kinetics and thermodynamics have been used to predict the mechanism of adsorption of a number of pollutants onto adsorbents. Adsorption normally occurs through four stages. First, the pollutant molecules are transferred to the bulk surface of the adsorbent. Secondly, the pollutant diffuses through the boundary layer to the surface of the adsorbent. The pollutant molecules then move to the pores of the adsorbent. And finally, adsorption occurs on the active sites on the adsorbent (Nethaji et al., 2013).
As a biomass adsorbent, raw watermelon rind inherently contains favorable structural properties that presents numerous benefits over the modified adsorbents. Raw watermelon rind based adsorbents are green, non-toxic and low cost adsorbents used to remove both organic and inorganic pollutants (El-Shafie et al., 2021). Watermelon rind is rich in carbohydrates, phenolic compounds and fatty acids. The carbohydrates are primarily composed of cellulose and hemicellulose. In addition, watermelon rinds also contain protein and pectin which have been found useful in the adsorption of various pollutants (Chen et al., 2017; Li H. et al., 2019; Masoudian et al., 2019; El-Shafie et al., 2021). Cellulose, protein and pectin are rich in the hydroxyl, amine and carboxylic functional groups, respectively. These functional groups are able to bind pollutants such as heavy metal cations through ion exchange or complexation, physisorption (Shakor et al., 2021; Taib et al., 2021; Taib et al., 2023) and organic pollutants such as dyes through electrostatic attractions, hydrogen bonding, π-π interactions and physisorption (Mort et al., 2008; Jawad et al., 2018b; Jawad et al., 2019; Santoso et al., 2020; Aljeboree et al., 2022). Watermelon rind-based adsorbents display diverse adsorption mechanisms largely dependent on the nature of the pollutant. For heavy metal cations such as Cd(II), Cr(VI), Cu(II), Ni(II), Pb(II) and Zn(II), the adsorption mechanism is majorly by ion exchange (Wang et al., 2022). Watermelon rind is inherently rich in metal cations such as K, Na, Mg, Ca and Fe that act as ion exchange sites for most of the heavy metals (Lakshmipathy and Sarada, 2013). This explains the considerably high adsorption capacities of the heavy metals on the unmodified watermelon rind based adsorbents. Figure 2 illustrates the adsorption mechanisms of WMR based adsorbents.
The commonly used analytical techniques for determining these functional groups include FTIR, BET surface area analysis, SEM and XRD. These tools are used to determine the chemical, physical and structural properties of adsorbents, and provide insight on the possible adsorption mechanisms involved in the adsorption process and thus explain their adsorptive properties. In this section, we review the common adsorption mechanisms for unmodified watermelon rind based adsorbents are discussed in literature.
Batch adsorption studies to investigate the potential of unmodified rind to effectively adsorb Ni(II), Cu(II) and Zn(II) ions from synthetic wastewater showed an impressive maximum adsorption capacities in the range 40.65, 44.85 and 52.81 mgg-1 for the three cations, respectively (Shakor et al., 2021). The study attributed the cations uptake to the presence of–OH and–COOH functional groups as identified by FTIR functional group analysis. The difference in the loading capacities for the three cations can be attributed to differences in their ionic radii, hydration energies and different complexations. This is consistent with reports of other studies (Kanungo et al., 2004; Dhaouadi et al., 2021), with minor variations resulting from solution pH and the nature of functional groups in the adsorbent. On the contrary, the adsorption rate as indicated by the adsorption rate constant (k2) values was in the order Ni(II)>Cu(II)>Zn(II). This is because the smaller Ni(II) ions diffuse faster into the pores of the adsorbent compared to the relatively larger ions of Cu(II) and Zn(II).
In another study, raw watermelon rind was prepared and used for the adsorption of synthetic Cd (II) ions from solution (Wang et al., 2022). The results showed an impressive adsorption capacity of 115.0 mgg-1 as predicted by the Langmuir isotherm, at neutral pH, underscoring the significance of agricultural waste in practical application as a pollutant remedy, with no chemical modification required. The mechanism of uptake of the cations, as predicted from the studies of the ionic strength, adsorption isotherms and zeta potential was reported to be 41% governed by electrostatic interactions. The study estimated that 83% of the cation adsorption resulted from ion exchange. Regeneration tests indicated that 0.1 M HNO3, had the highest potential to regenerate the spent adsorbent at 94.1% after one sorption-desorption cycle.
Unmodified watermelon rind has also been used in the removal of Cu(II) ions from its synthetic solution (Taib et al., 2021). In this study, functional group analysis showed the presence of -OH stretching vibrations of cellulose, pectin and lignin of watermelon. Bands typical of C=O vibrations were also detected and attributed to carboxylic acid or ester from pectin. This adsorbent was reported to have a very high adsorption capacity for Cu(II) ions at 357.14 mgg-1 equivalent to 79.5% removal. This study proposed watermelon as a non-hazardous agro-waste and an inexpensive adsorbent for the removal of the cations. The mechanism of adsorption, as reported by the study, was accomplished by deprotonation of hydroxyl or carboxyl functional groups followed by metal chelation.
Taib et al. (2023) also prepared an unmodified low cost adsorbent from watermelon rind for the removal of Zn(II) ions from its synthetic solution. The study reported a gradual increase in the adsorption of the cations with increasing pH up to pH 5. At low pH (pH < 3), a low percentage removal of Zn(II) ions was recorded at 70.6%, compared to 92.1% removal at pH 5. This observation was attributed to the high concentration of hydrogen ions at low pH, which compete with the Zn(II) ions for the active adsorption sites. The proposed mechanism for this adsorption was electrostatic attractions between the Zn(II) ions and carboxyl and hydroxyl groups, as well as formation of strong complexes between Zn(II) ions and amine groups through the nitrogen atom. The Langmuir isotherm best described the equilibrium data with qmax = 20.45 mgg-1. The adsorption kinetics were best described by the pseudo-second order (PSO) kinetic model. XRD diffractograms before and after the adsorption showed either disappearance, decreasing or shifting of peaks. The intensity and sharpness of the peak indicated that watermelon rind was semi-crystalline with peaks at 2θ = 22.6°, 38.4°, 44.7°, 65.0°, and 78.2°, whereas the Zn(II) ion-loaded adsorbent was essentially amorphous. This change was ascribed to the electrostatic interaction between the Zn(II) ions and the functional groups on the surface of the adsorbent.
An unmodified watermelon rind was activated for use in the adsorption of rare earth metal ions, Ce(III) and Pr(III) from aqueous solution (Devanathan et al., 2022). This was preceded by optimization of batch variables before equilibrium studies. The analysis reveals that the process is a physisorption and that the adsorption of both ions was spontaneous and exothermic. Implying that the process is feasible, widely applicable and environmentally friendly in respect to energy requirement. The adsorption capacity of the rind for Ce(III) and Pr(III) was reported as 56.8 and 55.3 mgg-1, respectively. It was observed that the adsorption capacity of the biosorbent increased with increasing pH due to decreased hydrogen ion concentration limiting competition with metal cations. The optimum pH was found to be 5 after which the adsorption of the cations diminished. The contact time was investigated by varying the adsorbent-adsorbate interaction time between 5 and 120 min. Rapid adsorption was observed within the first 5 min of the adsorption process. This can be attributed to the availability of fresh active adsorption sites. The adsorption rate gradually increased followed by pseudo-equilibration after 30 min, following the exhaustion of the active adsorption sites on the adsorbent. The effect of adsorbent dosage on the adsorption of Ce(III) and Pr(III) ions onto the indicated that 65% of the cations were removed at low adsorbent dosage. The results portray the adsorbent as a prolific means of Ce(III) and Pr(III) removal because of its multiple functional groups that can electrostatically bind metal cations (Lou et al., 2018). The Langmuir isotherm best described the adsorption data in the exclusion of these cations. A number of eluents such as 0.01 M HCl, 0.01 M CH3COOH, 0.01 M NaOH and distilled water were used to regenerate the spent adsorbents for further adsorption of Ce(III) and Pr(III) cations. The study reported that a ∼100% recovery using 0.01 M HCl underscoring both the recoverability of the adsorbent and the prolific nature of the eluent in doing this.
Aljeboree and co-workers prepared a watermelon rind based adsorbent by pre-treatment without any chemical or physical modification, for the removal of methylene blue from a synthetic solution of the dye (Aljeboree et al., 2022). The functional group analysis of the adsorbent before and after use for the adsorption of methylene blue dye showed variations in peak intensity which could be attributed to a physical interaction of the adsorbent with the dye. Worth noting, no new peak was formed, further corroborating the position that the adsorption onto the biosorbent was a physisorption. The analysis of surface morphology of the adsorbent before and after the adsorption of methylene blue dye reported agglomeration of the active sites on the surface of the adsorbent, an indication of the interaction of the adsorbent and the dye particles. The morphology of the adsorbent’s surface was studied without any chemical treatment making it more environmentally friendly (Sartape et al., 2017). The study initial dye concentration, varied between 1–20 mgL-1 indicated that the adsorption capacity of the adsorbent increased with increased initial concentration of the dye. On the contrary, the removal efficiency declined with increased initial concentration. This was attributed to the fact that the available active sites on the adsorbent, being invariable, became fewer and fewer with increased concentration of the dye (Xiong et al., 2017; ALjeboree et al., 2019). The influence of adsorbent dosage on both removal efficiency of the dye and the adsorption capacity of the adsorbent varied in the range 0.02–0.20 gL-1 showed that removal efficiency increased linearly with increase in adsorbent dosage, attributable to increased adsorption sites. However, the adsorption capacity diminished with increasing adsorbent dosage, attributable to agglomeration of the active adsorption sites (Hadi et al., 2014; Jasim and Aljeboree, 2021; Onyango et al., 2024). The regeneration of the spent watermelon rind based adsorbent after use was also investigated. A 100 mL of eluent (0.1 M NaOH, 0.1 M HCl, 0.1 M acetic acid or distilled water) was used at 25°C for 60 min. The study reported that 0.1 M HCl as the best eluent in terms of desorption efficiency.
In a study to investigate the removal of cationic methylene blue (MB) dye using powdered WMR, Jawad and group observed that dye-adsorbent interaction was through electrostatic attractions (Jawad et al., 2018a). FTIR analysis indicated that deprotonation of–COOH and–OH groups provided the binding sites for the positively charged MB dye molecules.
For cationic dyes like malachite green (MG) and methylene blue (MB), the mechanism of adsorption may involve electrostatic interactions, hydrogen bonding, n-π interactions and π-π interactions, contributing to the overall adsorption of these compounds (Tran et al., 2017). Electrostatic interactions occur due to functional group density of hydroxyl, carboxylic, carbonyl and amine functional groups. At high pH values, these functional groups get deprotonated rendering them negatively charged favoring the electrostatic interactions between them and the cationic dye molecules. For this reason, electrostatic mechanism is highly pH dependent.
The review reveals that adsorption of cationic dyes by watermelon rind is predominant over the uptake of heavy metals. The uptake of heavy metals is slowed by the presence of competing ions such as Mg, Zn, K, Ca and Fe inherently present in watermelon rind. Furthermore, the functional groups rich watermelon rind favors the electrostatic interactions between the deprotonated functional groups and the cationic dye molecules. Worth noting, the electrostatic interaction between dye molecules and the functional groups is highly pH dependent and can be enhanced by adjusting pH to favour deprotonation of the functional groups, or by alkali activation.
The adsorption of anionic dyes such as Congo Red and Methyl Orange mainly follows electrostatic interactions under low pH conditions. Under such conditions, the–COOH, -OH and–NH2 functional groups get protonated favouring electrostatic interactions with anionic dye molecules. The mechanism of adsorption of anionic Congo Red dye onto raw watermelon rind adsorbents (Hussain et al., 2022). At pH 4-5, the anionic dye molecules were electrostatically attracted to the protonated functional groups resulting in increased adsorption capacity. Other mechanisms of dye adsorption proposed included π-π interactions and hydrogen bonding.
In conclusion, adsorption isotherms, kinetics and thermodynamics have been used to predict the mechanism of adsorption of pollutants. Studies of effect of parameters such as pH, and the regeneration of the spent adsorbents, have also provided additional insight into the mechanism of pollutant uptake. A number of studies that have been reviewed herein also reported characterization of the adsorbents before and after pollutant uptake. Such techniques are useful in identification of the functional groups involved in the adsorption, and confirms chemical states and bonding interfaces. However, computational simulation studies such as density functional theory (DFT) have scarcely been used. Worth noting, many studies have used these methods in isolation, indicating that the results of such determinations can only be predictive. For a comprehensive understanding of the mechanism of uptake of pollutants, and to obtain a clear order of interaction between the watermelon rind-based adsorbents and the pollutants, a combination of the aforementioned methods can be applied in conjunction with computational simulations.
3 Modification of watermelon rind based adsorbents
Generally, unmodified biomaterials have lower adsorption capacities for pollutants compared to the modified forms (Supanchaiyamat et al., 2019; Santoso et al., 2020). Consequently, studies have reported modification of these biomaterials via physical or chemical activation, or a combination of both, prior to their use in adsorption. The incorporation of other materials with adsorptive capabilities to form composite/hybrid biomaterials has also been studied for the removal of pollutants from aqueous solutions.
Physical modification of bio-adsorbents is generally considered simple and inexpensive compared to chemical methods (Lee and Choi, 2021). Physical methods of activation of pre-treated watermelon rind may be achieved by thermal treatments at temperatures between 300°C–800°C in an oven to produce a biochar. This method is commonly referred to as carbonization. The biochar may further be steam-activated to improve the surface properties of the adsorbent, such as its porosity and functional group density.
Shukla and co-authors synthesized a watermelon rind based biochar by slow pyrolysis at 550°C, under anoxic conditions, for the removal of methylene blue dye from water (Shukla et al., 2024). The adsorption data was best described by the Langmuir isotherm (R2 > 0.99) with a maximum adsorption capacity of 115.61 mgg-1. The pseudo-second order kinetic model best described the adsorption kinetics. The uptake of methylene blue was reported to have proceeded through hydrogen bonding, electrostatic interactions and aromatic interactions. The results of the study suggest that thermal treatment ameliorates the textural properties of watermelon rind, making it a better adsorbent. Thermal treatment has also been known to improve structural stability and enhance functional groups. However, thermal treatment is an energy intensive process often requiring up to 800°C. In addition, providing anoxic conditions presents additional operational costs in addition to the high cost of the equipment.
Chemical modification involves controlled use of chemicals such as acids, bases and salts to activate the pre-treated watermelon adsorbent. Particularly, sulphuric acid activation has been reported in many studies to be efficient mainly for the adsorption of cationic substances (Li J. et al., 2019; Santoso et al., 2020). Chemical processes such as oxidation and surface functionalization have also been applied.
Lignocellulose dried watermelon rind activated with H2SO4 was used in the removal of methylene blue dye from aqueous solution (Lee and Choi, 2021). The activation resulted in enriched formation of–SO3H, –COOH and–OH functional groups which in turn enhanced the adsorption of methylene blue dye by electrostatic interactions, with a maximum adsorption capacity of 334.45 mgg-1 at pH seven reported. The pH point of zero charge of the adsorbent was adjusted from 6.3 to 4.1 to favour the adsorption of cationic methylene blue dye. The adsorption kinetics and isotherms were best described by the pseudo-second order kinetic model implying chemisorption, and the Langmuir isotherm suggesting adsorption onto homogenous sites with no lateral interaction between adsorbed molecules, respectively. BET analysis indicated a colossal increase, up to 75 orders of magnitude, in the specific surface area of the adsorbent after sulfonation.
In another study, a sulphuric acid activated watermelon peel based biochar was prepared and used in the adsorption of Cu(II) ions from synthetic wastewater with a 78.33% removal efficiency reported (El-Nemr et al., 2022), corresponding to a maximum adsorption capacity of 151.52 mgg-1. The synthesized biochar from watermelon peel (Melon-B) was first modified through Ozonation (Melon-BO) and subsequently by dissolution in NH4OH (Melon-BO-NH2) and triethylenetetramine (Melon-BO-TETA). TGA analysis was conducted on the adsorbents to determine the difference in their thermal behaviours resulting from their structural differences. All samples showed weight loss before 100°C attributable to volatilization of water molecules. Melon-B and Melon-BO showed a second weight loss at temperatures above 100°C attributable to decomposition of oxygen-based functional groups. For Melon-BO-NH2 and Melon-BO-TETA, a second weight loss occurred at temperature above 180°C signifying the decomposition of amines. At temperatures above 450°C the thermograms became similar as a result of decomposition of carbon in the biochar structure. In the end, the total weight loss of the samples as recorded were watermelon peel (87.38%), Melon-B (85.5%), Melon-BO-TETA (51.18%), Melon-BO (49.35%) and Melon-BO-NH2 (47.20%) reflecting the stability of the oxidized and amine modified samples compared to raw watermelon peel and Melon-BO.
Chaitra and co-workers also used sulphuric acid modified watermelon rinds as a low cost adsorbent for the removal of methylene blue dye from synthetic wastewater (Chaitra et al., 2022). Under the investigated environmental conditions, watermelon rind showed a clearance efficiency of 97%–99%. The authors reported a steady decline (99.7%–40.7%) in the removal efficiency of methylene blue dye from aqueous solution using the sulphuric acid modified watermelon rind when the initial concentration was varied between 20–160 mgL-1. Such a phenomenon is attributable to the need to first overcome the boundary layer effect in cases where the dye concentration is higher, and often requiring a longer contact time before equilibration is attained (Yunusa et al., 2021). The authors further investigated and reported on the effect of pH on the sequestration of methylene blue dye using the WMR based adsorbents. The solution pH was varied between pH 2–10. The study reported a marginal increase in the removal efficiency with increased pH, within the range of 56.2%–59.4%. The nominal increase in the removal efficiency with increased pH can be attributed to less competition between the hydrogen ions and the cationic methylene blue dye for the active adsorption sites at the elevated pH levels. The authors recommended pH 9 as ideal for further research but did not report on the effect, if any, of the sulphate ions introduced during the activation of the adsorbent. The authors also varied the contact time between 30–300 min. The dye removal efficiency increased with increasing contact time, followed by a pseudo equilibration after 180 min. The effect of adsorbent dosage on the adsorption of methylene blue indicated an increased removal efficiency (55.97%–99.58%) with increase in the mass of the adsorbent. This was attributed to the availability of more active adsorption sites that enhanced the uptake of the dye.
Alkalis have also been used to activate watermelon rind based adsorbents. In a particular study, watermelon rind activated with 0.1 M NaOH was used in the adsorption of Zn(II), Cu(II) and Fe(III) from synthetic wastewater (Low et al., 2023). The adsorption of Fe(III) and Cu(II) fitted well with the Langmuir monolayer isotherm while that of Zn(II) fitted with the multilayer Freundlich isotherm. Kinetic studies indicated that the adsorption of the three cations was a chemisorption process, following the pseudo-second order kinetic model. Alkali treatment has been known to increase the adsorption capacity by increasing cellulose content and reducing lignin, and instead forming–OH groups which increase the specific surface area of the adsorbent (Kumar and Sharma, 2017; Shimizu et al., 2020). However, the growing concerns on the use of alkali activators with respect to environmental safety and cost implications cannot be disregarded.
Simultaneous application of physical and chemical treatment is also a common method of preparing WMR-based adsorbents. In a study to investigate the removal of acid yellow 11 (AY11) dye using modified biochar derived from watermelon, El Nemr and co-workers (El Nemr et al., 2020) synthesized four adsorbents, first by sulphuric acid activation of pretreated WMR to obtain Melon B biochar formed from both carbonization and sulfonation. The biochar was then oxidized in water using ozone as the oxidizing agent to form Melon-BO adsorbent. Ozonation has been known to increase the oxygen containing functional groups such as–OH and–COOH. Melon-BO was further modified by separately boiling it in 25% triethylenetetramine (TETA) and NH4OH for 2 h to form Melon-BO-TETA and Melon-BO-NH2, respectively. The study observed that TETA and NH4OH strongly influence the physicochemical properties of the biochar which ultimately improved the uptake of AY11 dye by the adsorbent. Melon-BO-NH2 showed increased surface area and improved adsorption attributable to the specific surface area. Furthermore, SEM micrographs showed that sulphuric acid treatment of watermelon peels did not alter the morphology of the raw peel. SEM images of Melon-BO-NH2 and Melon-BO-TETA showed wider porousity responsible for the increased specific surface area whereas Melon-B and Melon-BO had low porousity which is in consistence with the BET surface area analyses. Amine modification led to the formation of new functional groups. The FTIR spectra of Melon BO-NH2, and Melon-BO-TETA biochars had new peaks in the range 1,354–1,372 cm–1. This band was attributed to the stretching vibration of–N=C=O group.
Hybrid biomaterials containing WMR, other biomaterials and nanoparticles have also been studied and show improved adsorption capacities compared to those of unmodified WMR. Mesoporous watermelon rind based adsorbent functionalized with chitosan was prepared and used in the removal of Cu(II) ions from aqueous solution (Bamisaye et al., 2024). The FTIR spectra of the composite adsorbent showed the existence of carbonyl, hydroxyl and amine groups while SEM micrographs showed a porous substance. Furthermore, the FTIR spectra of watermelon rind and watermelon rind functionalized with chitosan showed significant differences. Several peak shifts were noted, with concomitant change in intensities. For instance, a peak associated with aromatic C-H in-plane bend shifted from 1140.80 cm-1 in watermelon rind (WMR) to 1010.40 cm-1 in the chitosan modified composite (WMR-CH), a total shift of 130.40 cm-1. Such shifts are obviously attributed to modifications as a results of chitosan functionalization, consistent with other studies (Lam et al., 2017; Bamisaye et al., 2023). Figure 3 shows the comparison between the FTIR spectra of the raw watermelon rind (WMR) and watermelon rind functionalised with chitosan (WMR-CH). The optimum uptake of the cation (96.5%) occurred at pH 6 with an adsorbent dosage of 0.45 g. The kinetic data was best described by the pseudo-first order kinetic model (R2 = 0.9800) and the thermodynamic parameters pointed to an endothermic and spontaneous process. Based on the coefficient of determination (R2) and lowest error values, the Langmuir-Freundlich isotherm best described the adsorption of Cu(II) ions onto the chitosan functionalized watermelon rind based adsorbent. This implies that the adsorption took place on a heterogeneous surface resulting in physicochemical interactions and complex mechanisms (Egbedina et al., 2023).
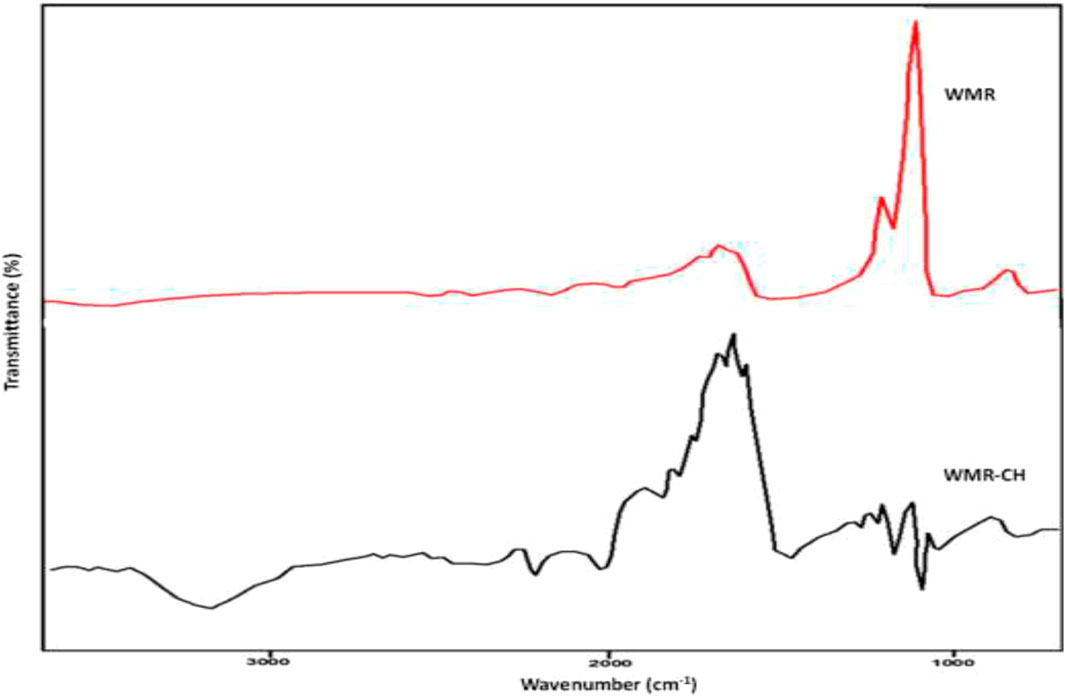
Figure 3. FTIR spectra of WMR and WMR-CH (Bamisaye et al., 2024).
Other than in enhancing the adsorptive properties of watermelon rind based adsorbents, biopolymers such as chitosan are eco-friendly and cost effective activators and additives which have proven versatile in removal of a number of pollutants. Such biopolymers will in future offer a more prolific alternative to conventional chemical activators such as mineral acids.
In another study, a composite adsorbent was synthesized using magnetite nanoparticles and watermelon rind for the adsorption of Pb(II) ions (Adebowale et al., 2020). In this study, watermelon rind was used as a composite to stabilize the magnetite nanoparticles often resulting in agglomeration (Lasheen et al., 2016). Magnetite nanoparticles have the ability to react with various functional groups, making the polyfunctional watermelon rind ideal for composite formation. The adsorbent was synthesized by mixing FeSO4 and FeCl3 in the ratio 3:2 followed by addition of deionized water and 2.5 M NaOH solution forming a solution of Fe3O4 to which the rind was added to form the composite. The adsorption capacity of this composite for Pb(II) ions was found to be 138.8 mgg-1. FTIR bands characteristic of O-H and C-H stretching vibrations attributable to organic compounds including phenolic and lactonic groups were linked to the watermelon component. Therefore, other than providing stability for the magnetite nanoparticles, it is conceivable that the functional groups originally present in the watermelon rind may have enhanced the uptake of the metal ions.
Despite the concomitant increase in the adsorptive properties of watermelon rind based adsorbents as a result of the enhanced textural and chemical properties arising from modifications, these processes present inherent challenges including detrimental impacts to the environment. Furthermore, carbonization is an energy intensive process hindering the up-scalability of the use of these adsorbents. Future studies must therefore focus on green and sustainable techniques including the use of biopolymers and enzymes. These studies will inevitably also focus on incorporating nanotechnology to develop more prolific adsorbents.
4 Application watermelon rind based adsorbents in the removal of heavy metal ions from aqueous solutions
Shakor et al. (2021) prepared an unmodified watermelon rind based adsorbent for the remotion of Ni (II), Cu(II) and Zn(II) ions. FTIR analysis reported peaks typical of functional groups capable of interacting with metalic cations. The observed peaks included O-H stretching vibrations associated with intramolecular hydrogen bonds of cellulose (3425 cm-1), C-H stretching vibrations of methoxy and methyl groups (2920 cm-1), C=O stretching vibrations of esters and carboxylic acids (1735 cm-1) and stretching vibrations associated with alcohols and carboxylic acids (1300–1000 cm-1). A shift in peak positions associated with–OH and–COOH functional groups after the adsorption was attributed to co-ordination with metal cations. These functional groups were reported to act as proton donors in their interaction with the cations. The study also reported on the effect of pH on the adsorption of the cations while varying the pH between pH 2-8, and while keeping other experimental conditions constant (initial concentration of 50 mgL-1, 0.1 g adsorbent dose, 250 rpm agitation speed and 60 min contact time). The authors reported a linear increase in the removal efficiency of the cations with increasing pH. This phenomenon was attributed to, on one hand, the formation of metal hydroxides due to the abundance of OH− ions at high pH, and on the other hand, competition between the cations and hydrogen ions at low pH. The study optimized the pH at 6 with the reported removal efficiencies of 62.6, 62.6% and 70.0% for Ni(II), Cu(II) and Zn(II) ions respectively. Furthermore, the effect of adsorbent dosage on the adsorption of the cations was conducted while keeping the other experimental conditions constant (initial concentration of 50 mgL-1, pH 6, 250 rpm agitation speed, 60 min contact time at 303 K). An increased uptake of the cations was reported with increasing adsorbent dosage. This was attributed to increased surface area for adsorption provided by increased available active sites.
In a separate study, watermelon rind activated with 15% HNO3 was prepared for the sequestration of Zn(II) ions from synthetic wastewater (Altowayti et al., 2021). Nitric acid has been reported to enhance porosity and specific surface area of adsorbents (Yahya et al., 2015). EDX analysis of the adsorbent showed the presence of mesoporous silica and a protein. The study concluded that under optimal conditions, dried watermelon rind activated with HNO3 has the potential to extract Zn(II) ions from the synthetic wastewater with a maximum adsorption capacity of 53.92 mgg-1 reported.
In another study, a watermelon based adsorbent (WMBA) was prepared first by carbonization in a muffle furnace at 500°C–700 °C for a total of 3 h followed by activation in 0.6 M solution of NaOH (Okam et al., 2022). The adsorbent was used in the removal of Cr(II) from textile effluent with a 97.8% removal efficiency for the metal ions. FTIR analysis showed a number of functional groups on the adsorbent before adsorption. These included O-H stretching of H2O (3395cm-1), asymmetric stretching of methylene H-H (2935 cm-1), pyridine C≡N stretching (1592 cm-1), N=N stretching of azo compound (1402 cm-1), aliphatic C-N stretching (1804 cm-1) and P=S stretching (683 cm-1). After adsorption, a shift of some of these bands confirmed the participation of these functional groups in the adsorption of metal ions onto the adsorbent. Morphology of a watermelon based adsorbent before adsorption of the ions also showed a highly porous surface structure capable of enhancing the adsorption of metal ions. In addition, some physical changes were observed after the adsorption indicating lump-like deposits of shiny particles typical of chromium metal. XRD analysis showed a largely amorphous material characterized by the marginal sharp peaks. This is an advantageous property of adsorbents in exclusion of pollutants especially metal ions (Othman et al., 2014). Mineralogical studies revealed the presence of mica, kaolinite and quartz. XRF analysis characterized the biosorbent before and after the adsorption. An impressive loading capacity of 24.4% Cr(II) ions was reported after adsorption. The results also highlighted the significance of silica and alumina in the adsorption process, with the availability of these minerals decreasing significantly after adsorption. For instance, the percentage composition of silica before and after the adsorption was 83.7% and 1.18% respectively.
Watermelon rind activated using ZnCl2 and carbonized using a microwave at 800 W microwave power showed potential for use in the sequestration of zinc(II) ions from aqueous solution (Yilmaz and Tugrul, 2021). The maximum adsorption capacity was reported at 14.76 mgg-1 corresponding to a 36.9% removal efficiency. FTIR functional group analysis demonstrated O-H stretching vibration of the hydrogen bonded carboxylic acid, phenols and alcohols with another band corresponding to the C=O of carboxylic acid, acetate, ketones and aldehydes detected. The functional groups have the potential to bind cations through the transfer of electrons to form complexes with ions such as Zn(II) ions in their solution (Meseldzija et al., 2019; Safari et al., 2019; Shakya and Agarwal, 2019; Yusuf et al., 2020).
In another study, thermally treated (at 500 °C) watermelon rind showed the best adsorption efficiency attributable to improved porosity and surface area (Popoola et al., 2022). The study investigated the efficiency of a thermally activated watermelon rind for the adsorption of Pb(II), Zn(II), Fe(II) and Cu(II) ions from oil well produced water. The adsorbent was pretreated then calcined at 681.10°C for 2.61 h using a muffle furnace. The activated carbon material exhibited porous-graphite crystalline structure, presence of active functional groups and an ultrahigh specific surface area of 1249.7 m2/g ideal for adsorption. The authors characterized the watermelon peel derived adsorbents by FTIR before and after adsorption of the heavy metal ions. The pre-adsorption analysis of the adsorbent revealed the presence of functional groups capable of enhancing the adsorption of the heavy metals. The identified functional groups include O-H groups in cellulose, C=C stretching of cyclic alkenes, C-H bending of alkanes, C-O stretching of aliphatic ether and C=C bending of alkenes (Guo and Rockstraw, 2006). A pseudo-disappearance of some of these adsorption bands after adsorption was attributable to the disappearance of some functional groups hitherto in the adsorbent as a result of interaction with the metal cations. The study further demonstrated that the temperature applied in thermal treatment is important in determining the specific surface area of the adsorbent. An ultrahigh specific surface area was obtained by calcination at 681.1°C, increasing the potential of the adsorbent to remove the cations in the oil well produced water (Yuliusman et al., 2020). The average pore diameter indicated that the adsorbent was microporous (Bibaj et al., 2019). SEM images showed a rough surface, evenly distributed pore formation with consolidated particles linked to massive dehydration during calcination. The closure of the pores observed following interaction with the heavy metal in the water was linked with the transfer of heavy metal cations from the water to the pores of the adsorbent. XRD analysis showed strong diffraction peaks at 2θ = 18° and 20°, indicating the existence of graphite crystalline phase on the wall of the adsorbent that makes it more porous. Again, better layer alignment as a result of increased crystalline structure could be inferred from these peaks (Yang and Lua, 2006). The bulk oxide determination by XRF revealed the presence of SiO2 (64.81%) and Al2O3 (8.33%). The presence of silica and alumina in such significant quantities is ideal for the fixation of heavy metal cations due to electrostatic interactions with silanol and aluminol groups.
In more recent studies, watermelon peels were carbonized at 300°C in a muffle furnace followed by chemical activation using 1M H2SO4 (Archibong et al., 2024). The adsorbent was used to remove Cu(II) and Cd(II) ions from synthetic wastewater. The study reported a removal efficiency of over 98.64% for both metals over the studied initial concentration range (50–140 mgL-1).
SEM images obtained from untreated watermelon rind based adsorbent compared to that of watermelon rind treated with citric acid (Letechipia et al., 2023) showed significant differences in the shape of the pores (Figure 4). Citric acid is a known chelating agent that reacts to form a ring, thereby adjusting the structure of the compounds and incorporating new fuctional groups (Bhakta and Ali, 2020).
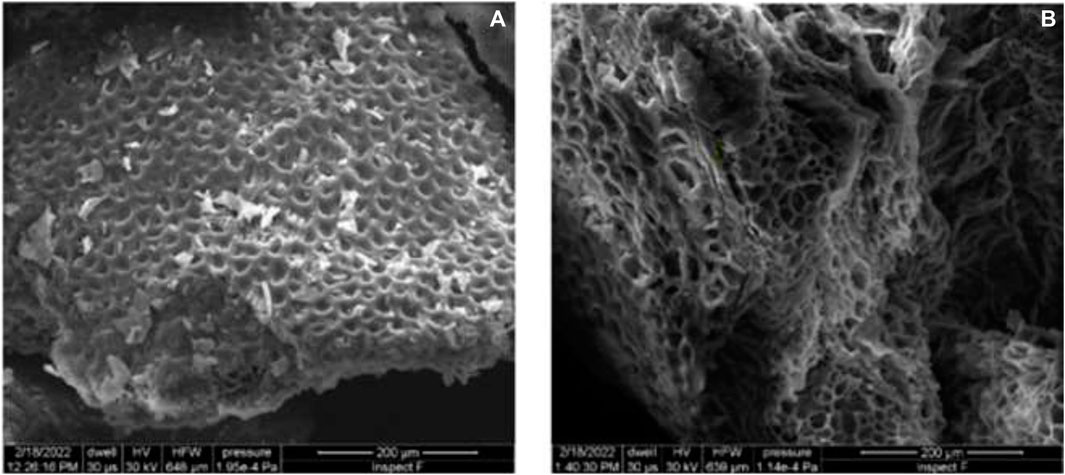
Figure 4. SEM images (A) Natural biosorbent (480X) and (B) biosorbent with treatment (480X) (Letechipia et al., 2023).
5 Application watermelon rind based adsorbents in the removal of dyes from aqueous solutions
Hussain and co-workers demonstrated the dominance of chemically activated watermelon rind over raw watermelon for the adsorption of Congo Red (CR) dye (Hussain et al., 2022). In this study, the two adsorbents, an un-activated peels of watermelon (PWM) showed an adsorption capacity of 2.23 mgg-1 for CR dye whereas citric acid activated watermelon (CPWM) was almost four orders of magnitude better, at 8.3 mgg-1. The authors attributed the increased adsorption capacity to the evolution of additional adsorption sites as evidenced by SEM and FTIR characterization. Citric acid is an eco-friendly activator since it is non-toxic, and is biodegradable. It therefore offers a potent alternative to mineral acids such as sulphuric acid, which are both corrosive and hazardous. The authors analyzed both PWM and CPWM before and after use using FTIR. The results indicate that unused PWM had functional groups responsible for electrostatic interactions between the dye molecules and the adsorbents (Zhao et al., 2020). These functional groups include OH of alcohols (3775–3600 cm-1) (Åkerholm et al., 2004), OH of COOH groups (3300 and 1720cm-1) and C=O of–COOH (1685cm-1) (Sawant and Dhabe, 2022). In the modified adsorbent, the wavenumbers 3860–3720 cm-1 correspond to chemical interactions of citric acid molecules with OH groups through chelation on the interfaces of CPWM. Strong peaks at 1705 cm-1 showed carbonyl groups of COOH on the interface of CPWM. Such groups are highly polar and improve interactions with the polar dye molecules, as evidenced by the FTIR spectra of PWM and CPWM after dye adsorption. The study also reported morphological changes arising from chemical treatment of watermelon using citric acid. In this study, SEM micrographs showed the transformation of peels of watermelon (PWM) originally having imbedded depression and thick spiral clusters to flannel, fluffy and flossy citric acid treated PWM, with increased adsorption sites effective for dye removal. After adsorption of the dye, SEM micrographs showed further morphological changes on CPWM which showed a threadlike, highly wavy, fibroid and stringy morphology, signifying interaction between the dye and the adsorbent. The solution temperature was varied in the range of 10°C–80°C. The optimized adsorption temperature was found to be 40°C for PWM and 30°C for CPWM corresponding to 75.5% and 95.0% removal, respectively. The decline in removal efficiency recorded beyond, for example, 40°C for CPWM was attributed to disintegration of the structure of lignocellulosic biomass of the adsorbent (Bachmann et al., 2022). Hussain and co-workers further investigated the thermodynamic properties of the adsorption process, classifying it an exothermic process based on the negative ΔH values. The authors also used 0.1 M NaOH to desorb CR dye from watermelon rind based adsorbents with a 75% efficiency. Low pH favoured the protonation of the surface of watermelon based adsorbents favouring the electrostatic interaction with CR dye, an anionic dye (Nizam et al., 2022; Isik et al., 2024). The initial solution pH was varied between 1–10 mgL-1 with optimum results obtained at pH four corresponding to 82% removal for raw peels of watermelon and 95% removal corresponding to citric acid activated watermelon peel. The pH point of zero charge for both adsorbents was determined by the drift method and reported to be ∼4.00. Therefore, beyond pH 4, the adsorbent performance declined due to increased deprotonation of -COOH sites which provided anionic binding sites enhancing repulsive response of the anionic dye molecules. Furthermore, the contact time was varied between 5–60 min with optimum removal attained after 30 min (84.3%) for the raw adsorbent and 25 min (95%) for the chemically modified adsorbent. This was attributable to increased adsorption sites on the surface of the chemically modified adsorbent, as confirmed by the physicochemical characterization of the two adsorbents, before and after use. The adsorbent dosage of PWM and CPWM adsorbents was varied between 0.2–2.0 g. The removal efficiency for CR dye, as reported in this study, increased to 71.4% for PWM and 95.0% for CPWM, up to an optimum adsorbent dosage of 0.8 g, after which the efficiency declined. The decline in the removal efficiency beyond 0.8 g dosage was attributed to a rapid establishment of equilibrium between the dye and the surface of each adsorbent. Again, the authors varied the agitation rate from 25–200 rpm, with a 25 rpm step, while holding other adsorption conditions constant. The study optimized the removal of the dye at 125 rpm corresponding to 73.5% on PWM and 97.2% on CPWM, after which the adsorption efficiency diminished. The increased adsorption between 25–125 rpm is attributed to increased number of collisions with dye molecules, whereas the decreased performance was attributed to the formation of a thin layer of the dye on the surface of the adsorbent from the bulk solution, hindering the access to the adsorption sites (Magaji et al., 2020). The Langmuir isotherm best described the adsorption data, with larger KL values for chemically modified adsorbent (0.42 Lmg-1) compared to 0.27 Lmg-1 of the unmodified adsorbent. The low values recorded (KL<0.5) is indicative of weak adsorptive forces typical of physisorption. The chemically modified adsorbent therefore portrayed a higher adsorptive energy than the unmodified one showing that stronger forces of interaction were in play. This was attributed to the availability of additional–COOH moieties. Based on the residual between the model predicted and experimental values, the study best described the kinetic data using the PSO kinetic model. The coefficients of determination (R2) values were the same, and close to unity (R2 = 0.99). However, pseudo-second order kinetic model hypothesizes that the rate limiting step in an adsorption is chemisorption (Saad et al., 2022) contrary to the projection by the adsorption isotherms which pointed to a physical process.
El-Shafie et al. investigated the adsorptive performance of thermally modified watermelon rind for the sequestration of acridine orange dye (El-Shafie et al., 2021). Thermal treatment at 500°C improved the BET specific surface area from 2.66–5.03 m2/g with concomitant increase in its porousity, and subsequently, the adsorption efficiency. The specific surface area (SSA) of an adsorbent is a quintessential physical property that variously affect the performance of an adsorbent. The BET surface areas (m2/g), pore volumes (cm3/g) and pore radius (Å) of the various watermelon rind based adsorbents are summarized in Table 3.
FTIR spectra of raw watermelon (RWM) and thermally treated watermelon at 250°C and 500°C (TTWM250 and TTWM500) for the adsorption of acridine orange were studied. For RWM, a broad peak was observed at 3300 cm−1 characteristic of the O-H group of physical and crystalline water. The same peak was observed in TTWM250 and TTWM500 with progressively lower intensity, attributed to thermal treatment. Another peak was observed at 2916 cm−1 in RWM, corresponding to the C–H symmetric and asymmetric stretching vibrations with progressively low intensities in TTWM250 and TTWM500. The obtained data confirm the existence of different functional groups on the surface of RWM, with a decline in intensity with increased activation temperature. These functional groups have significant effects on the adsorptive properties. Some differences were also noted in the spectra of TTWM500 before and after adsorption, and that of acridine orange. These changes include variations in intensities, shifts in peak positions, evolution of new peaks and complete disappearance of others. For example, spectrum of acridine orange shows a band at 2928 cm−1 which could be attributed to the C-H stretching. This band almost disappear in the spectrum after adsorption. A sharp peak at 1497 cm−1 which is characteristic of the aliphatic C-N stretching coupled to the aromatic C=C vibrations is absent in the spectrum of TTWM500 after adsorption. A small peak was noted at 1501 cm−1 confirming the existence of AOH+ on the surface of the adsorbent. All these findings confirm an interaction between the NH+ group of the acridine orange and the surface of the adsorbent. The study also reported some morphological variation as a result of thermal treatment of watermelon based adsorbents. The untreated adsorbent showed a smooth homogenous surface with no pores formed. In contrast, SEM micrographs of the adsorbents treated at 250°C and 500 °C showed improved porousity attributed to the conversion of the biomass to carbonaceous material. The authors attributed total weight loss after thermal analysis of untreated watermelon adsorbent (RWM) to loss of physically adsorbed water (25°C–130°C), decomposition of organic matter and formation of thermally stable carbonaceous materials (150°C–800°C) (Chaudhari and Singhal, 2015). Freundlich isotherm was applied to best describe the adsorption of acridine orange onto thermally modified watermelon rind, suggesting the occurrence of multilayer distribution of the acridine orange onto a heterogeneous surface of the adsorbent, with an interaction among the dye molecules. This confirms electrostatic interactions with dye molecules. The D-R isotherm’s free energy value suggested chemisorption at low concentrations and a physisorption at higher concentrations of the dye. PSO best described the adsorption of Acridine orange onto thermally modified watermelon rind with the intra particle diffusion (IPD) parameters suggesting that intra-particle diffusion was not the only rate limiting step in the uptake of the dye. An indication that boundary layer thickness played a major role in the initial stages of the adsorption. The sorption/desorption studies using various eluents (0.1 M H2SO4, H2O, 0.1 M HNO3 and 0.1 M NaOH) through six cycles showed a highest desorption efficiency of 75.9% in the sixth cycle with around 87% regeneration efficiency with 0.1M NaOH.
Watermelon rind activated using phosphoric acid followed by carbonization in a muffle furnace at 700°C was investigated for the removal of cationic malachite green dye and anionic active black dye from synthetic solution (Wei et al., 2023). The equilibrium adsorption data for malachite green followed the Langmuir isotherm, with a maximum adsorption capacity of 182.28 mgg-1. Figure 5 shows the schematic diagram for the synthesis of this adsorbent.
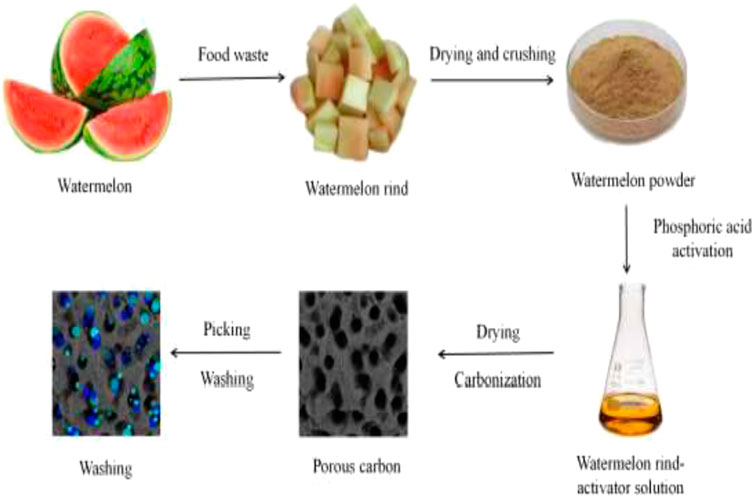
Figure 5. Phosphorus doped watermelon rind to porous carbonaceous material (Wei et al., 2023).
The effect of initial concentration of a phosphoric acid activated watermelon rind adsorbent on the sequestration of acid yellow 11 was investigated (El Nemr et al., 2020). The study reported that using an adsorbent dosage 2.0 gL-1, pH 1, 3 h contact time and at room temperature (25°C ± 2°C) and while varying dye concentration between 75–200 mgL-1, the dye percentage removal decreased from 96%–77% with increasing initial concentration. The study attributed this phenomenon to the saturation of adsorption sites on the adsorbent at low concentrations. The adsorption pH was varied from 1–10. The highest adsorption efficiency (96%–99%) was obtained at pH one and diminished with increasing pH. The authors related the pH dependent adsorption process with the functional groups of the modified biochars, particularly the hydroxyl, carboxyl and amino groups as identified by FTIR. At low pH, these functional groups get protonated rendering them attractive to the anionic acid yellow 11 dye. Furthermore, the authors investigated the effect of contact time (10–180 min) on the removal of acid yellow dye using the modified biochar derived from watermelon peel. At an initial concentration of 75 mgL-1 and pH 1, a 75% dye removal was recorded within the first 15 min of the adsorption using a 2 gL-1 adsorbent dosage. The study reported equilibration at 3 h with a corresponding removal efficiency of ∼90%. Such a long equilibration time was attributed to the slow pore diffusion of the solute ions in to the bulk of the adsorbent. The effect of adsorbent dosage on the sequestration of the dye was also investigated. The study reported that at pH 1, 3 h contact time and at room temperature (25°C ± 2°C) and while varying adsorbent dosage between 2.0–6.0 gL-1, a 100% dye removal was achieved at the 6.0 gL-1 adsorbent dosage at an initial dye concentration of 100 mgL-1. The study ascribed this situation to increased availability of active adsorption sites for dye binding. The adsorption of selected dyes using some WMR-based adsorbents, adsorption conditions and the fitting adsorption isotherms and kinetic models are summarized in Table 4.
6 Influence of adsorption conditions
Various environmental conditions influence the uptake of pollutants from their aqueous solutions. Such conditions include solution temperature, initial concentration of the pollutant, solution pH, contact time, ionic strength, adsorbent dosage and agitation speed. One of the key objectives of adsorption studies is the optimization of these adsorptive parameters for industrial scale application (Ngulube et al., 2017; Mashkoor and Nasar, 2020).
The study of the effect of temperature on the adsorption is important as it defines the thermodynamic properties of the process, that is whether the process is exothermic or endothermic, or whether the process is spontaneous or non-spontaneous. If the equilibrium adsorption capacity of an adsorbent increases linearly with temperature, then the process is said to be endothermic, and vice versa. For endothermic processes, increased kinetic energy enhances the collision of the pollutant with the surface of the adsorbent, whereas in exothermic processes, increased kinetic energy weakens the adsorptive forces holding the adsorbate onto the adsorbent. (Ofomaja and Ho, 2007; Prasanthi et al., 2016). Considering similar pollutants from the reviewed studies, it is concluded that there was inconsistency in the direction of the enthalpy changes (ΔH). For instance, the adsorption of Cu(II) ions by watermelon rind based adsorbents as reported in some studies was an exothermic processes (Lakshmipathy and Sarada, 2016; Shakor et al., 2021) whereas other studies demonstrated that it was an endothermic processes (Banerjee et al., 2012; Bamisaye et al., 2024). This contradiction can be attributed to the fact that adsorption thermodynamics are also influenced by other factors other than the identity of the pollutant and the nature of the adsorbent. These factors include the pretreatment of the adsorbent, functionalization and the experimental conditions. Three thermodynamic functions, that is, enthalpy (ΔH), Gibb’s free energy (ΔG) and entropy (ΔS) have been widely used to describe the adsorption thermodynamics. Negative values of ΔH point to an exothermic process, negative values of ΔG shows that the adsorption process is both spontaneous and feasible while negative values of ΔS denote decreased disorder during the uptake of the adsorbent. Table 5 provides thermodynamic data recorded in literature for the adsorption of some pollutants by various watermelon rind-based adsorbents. From the reviewed studies, it is clear that the adsorption of the pollutants onto watermelon rind based adsorbents is a spontaneous process given the negative values of ΔG. This is a significant observation useful in the applicability of the adsorbent and the feasibility of the adsorption process. It implies that the adsorption process can occur without input of external energy affirming its environmental friendliness.
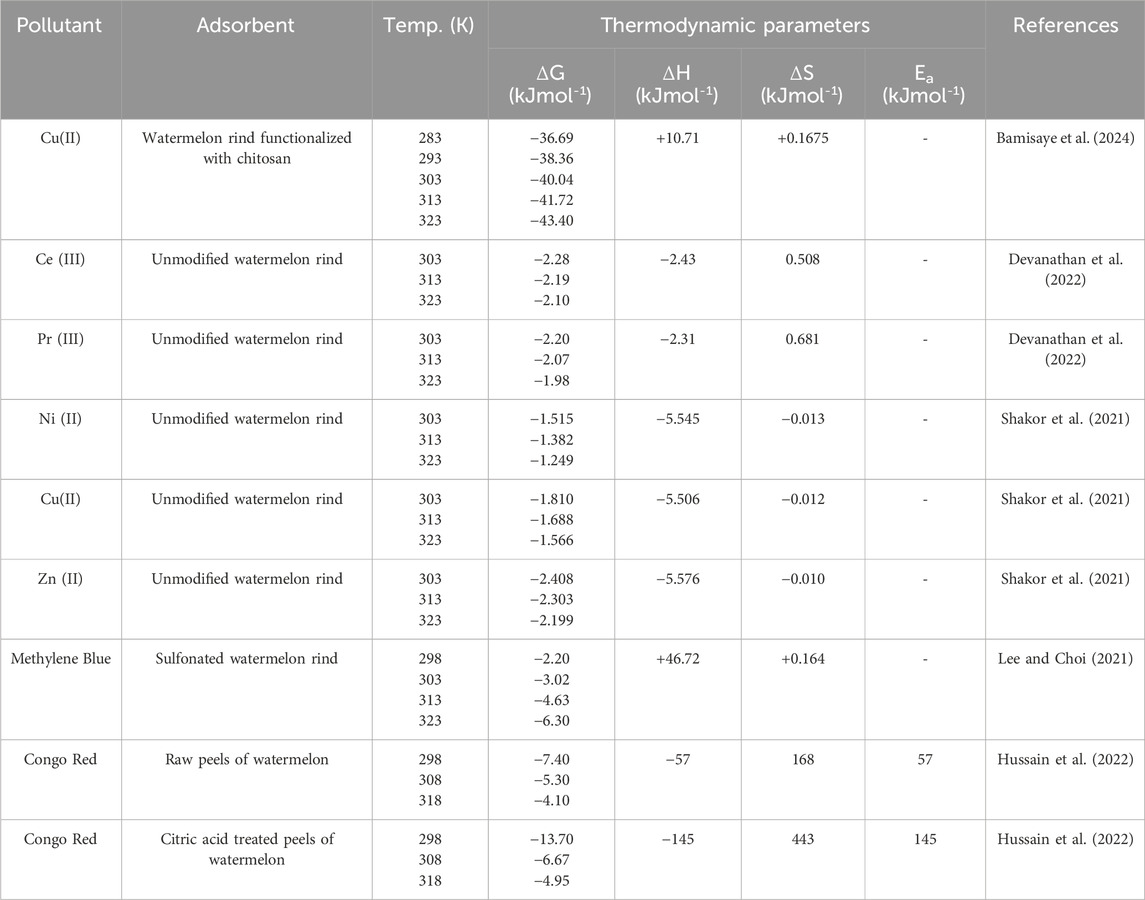
Table 5. A summary of the thermodynamic functions in the adsorption of various pollutants using watermelon rind based adsorbents.
While the removal efficiency has been reported to generally decrease with increased initial concentration and attributable to surface saturation of the adsorbent. The adsorption capacity, on the other hand, is reported to generally increse with increased initial concentration and is attributed to a higher concentration gradient driving more adsorbate to the surface of the adsorbent, a few studies have reported a deviancy from this phenomenon (Banerjee et al., 2012). Banerjee et al reported a decrease in the adsorption capacity of pollutants with increased initial concentration. The authors ascribed this to faster saturation of the adsorbent with increased concentration of Cu(II) ions, and that most of the Cu(II) adsorption therefore took place slowly inside the pores (Mohammad and Pa, 1997). The modeling of equilibrium adsorption data is the most common method used to determine the adsorption mechanism and provide insight into the performance of an adsorbent by predicting their maximum adsorption capacities. Isotherms provides information about the adsorbate distribution between the solid and liquid phases at equilibrium for different initial concentrations at constant temperature and pH (Molina-Calderón et al., 2022). Adsorption isotherms can be classified by the shapes of the isotherm graphs (L-shape, S-shape, H-shape and C-shape) (Limousin et al., 2007), and by the number of model parameters (Foo and Hameed, 2010; Al-Ghouti and Da’ana, 2020). However, both classifications provide little or no knowledge on the nature of the adsorption mechanism calling for an in-depth understanding of the physical meaning of each isotherm (Wang and Guo, 2020). Furthermore, adsorption isotherms do not provide critical adsorption information such as rate of adsorption and thermodynamics. Different isotherm models have been used to model equilibrium data. The Langmuir Isotherm (Langmuir, 1916) postulates a monolayer adsorption with no lateral interaction between the adsorbed molecules. Other than the Langmuir isotherm, the Freundlich model (Freundlich, 1906), the Sips isotherm (Sips, 1948) and the Temkin model (Temkin, 1940) have commonly been used to describe adsorption data. Based on the coefficient of determination (R2) values, the Langmuir isotherm predominantly described the adsorption of various pollutants onto the watermelon rind-based adsorbents. The Langmuir isotherm fits well with adsorbents with a homogeneous adsorption surface with specific binding sites for the pollutants. Watermelon is rich in functional groups which provide these binding sites.
The routes of pollutant uptake include physical uptake, electrostatic interactions and chemisorption. Chemisorption involves the formation of strong chemical bonds between the adsorbate and adsorbent and involve sharing or transfer of electrons. For this reason, chemisorbed substances are not easy to desorb (Allen and Koumanova 2005). However, in non-ideal conditions, both physisorption and chemisorption can occur simultaneously or alternatively (Dąbrowski, 2001). Again, in adsorption experiments, it is useful to know how well the experimental results fit with the various isotherm models. While the coefficient of determination (R2) is a useful tool in determining this relationship, it carries the risk of providing inadequate or incomplete information about the adsorption process. For example, it does not provide any information on the distribution of residual errors, and has the risk of overfitting adsorption data. From the reviewed studies, it is evident that there has been an over-reliance on this statistical tool, presenting the aforementioned limits. To overcome these limitations, more studies should embrace the use of other statistical tools including adjusted R2 and Chi-square. Furthermore, the analysis of residual errors should also be adopted in establishing best fitting models. Further, while batch adsorption is a cost effective and important technique in determining the feasibility of adsorption processes, and optimizing adsorption conditions, few studies have demonstrated an attempt to upscale the laboratory findings to multi-component or industrial scale situations, leaving a clear gap in their applicability. Furthermore, fixed bed systems have been reported to be more efficient in terms of higher adsorption capacities and removal efficiencies, while presenting more accurate thermodynamic and kinetic parameters, compared to batch adsorption (Malbenia et al., 2024). To overcome the limitations of batch processes, more studies need to explore column adsorption in the removal of various pollutants, especially using watermelon rind based adsorbents. The extrapolation of batch experimental results to continuous flow systems for practical application also require a careful examination and optimization of the hydraulic retention time (HRT) and flow rate. These two factors influence the removal rate and the adsorption capacity of contaminants in continuous flow systems. In a study to investigate the removal of crystal violet from aqueous solution using bone char, the highest adsorption capacity of 20.63 mgg-1 was recorded at the lowest flow rate reported at 15.4 ± 0.8 mL min-1. This was attributed to increased contact time between the adsorbent and the adsorbate at such low flow rates. To be able to relate such findings to real world scenarios, pilot scale continuous flow systems need to be designed and advanced by future studies. Such studies will optimize operational factors and conditions including HRT and flow rate, and ensure a well-researched transition from batch experiments to industrial scale continuous flow systems.
The effect of pH is a quintessential parameter for study as the solution pH affects the surface charge of an adsorbent, the degree of speciation and ionization and the structural stability of the adsorbate (Katal et al., 2011; Badiei et al., 2014). In general, the reviewed studies affirm that at low pH, due to the abundance of H+ ions, protonation of the–OH and–COOH among other functional groups occurs. This renders the active sites positively charged consequently favouring the electrostatic interaction with anionic pollutants. Conversely, at high pH, due to the abundance of OH− ions, the functional groups get deprotonated, rendering them negatively charged. This favours the electrostatic interaction between the deprotonated sites and cationic pollutants. Solution pH plays a major role on the performance of watermelon rind based adsorbents. As has been stated, watermelon rind based adsorbents perform differently depending on the nature of the adsorbate. Cationic dyes are majorly adsorbed by electrostatic interactions whereas metal ions are majorly adsorbed by complexation and ion exchange, even though an interplay of these processes has been suggested. The significant difference in the adsorbent’s adsorptive performance under different pH conditions points to the predominance of pH as an experimental condition, which further points to the predominance of electrostatic interactions over complexation and ion exchange, with respect to watermelon rind-based adsorbents.
A fast attainment of equilibrium time is an important feature of a good adsorbent as it lowers the operational costs of the adsorbent, ideal for industrial scale application (Istratie et al., 2019). This often translates to a more efficient and economical adsorption process since the absorbent resident time is reduced. The influence of contact time is therefore an important parameter to study, especially when designing a cost effective adsorbent. Adsorption kinetic models provide insight into the rate at which adsorption occurs. Some model parameters such as initial sorption rate, half-life and the sorption rate constant can be used to deduce the adsorption rate. Several adsorption kinetic models have been developed to describe adsorption reactions and diffusion of adsorbate particles into adsorbent active sites and pores. The Lagergren pseudo-first order (PFO) kinetic model (Ho and McKay, 1998), the pseudo-second order (PSO) kinetic model (Ho, 2006) and intra-particle diffusion (IPD) model (Weber and Morris, 1963) have commonly been used to describe kinetic data. Despite the quintessential information provided by the kinetic studies, the models cannot, however, predict vital adsorption information such as the adsorption capacities.
Studies have shown that under ideal circumstances, both physisorption and chemisorption can occur simultaneously (Dąbrowski, 2001). At an initial concentration of 30 mgL-1, a temperature of 303 K and at pH 5, Devanathan et al. compared the suitability of the PFO, PSO and the Elovich model in describing the kinetic data obtained in the adsorption of Ce (III) and Pr (III) using an unmodified watermelon rind based adsorbent (Devanathan et al., 2022). Based on the coefficient of determination (R2) values, the kinetic data was best described using the PSO kinetic model. A decrease in the loading capacity of the adsorbents for the cations was also observed to decrease with increased temperature. This was attributed to the weakening of the active adsorption sites that bind the metallic ions. Other than improving the adsorption capacity of the adsorbents, some chemical or physical methods of modification also improved certain adsorptive properties of the watermelon rind based adsorbents. Citric acid modification of a watermelon rind based adsorbent demonstrated increased rate constants, K2, from 0.038–0.071 g mg-1 min-1 suggesting the rate of uptake of Congo red dye increased two fold on modification (Hussain et al., 2022). Similar observations were also recorded in the removal of Acid Yellow 11 (El-Nemr et al., 2022). Based on the observations from the reviewed studies, non-linearized forms of the adsorption kinetic model equations have gained widespread and predominant use. The non-linearized equations are considered more accurate and realistic in interpretation of adsorption data and are more applicable to complex adsorption systems. However, the use of the coefficient of determination (R2) still remains a predominant, albeit inadequate, statistical tool in determining best fitting models. To improve the accuracy in selection of best fitting models, additional measures such as determination of residual errors is recommended. Some studies also depicted an apparent link between the specific surface area of the adsorbents and the rate of the adsorption process. An unmodified watermelon rind prepared for the adsorption of Acid Yellow 11 showed a BET specific surface area of 1.79 m2g-1 with an adsorption rate constant, K2, of 34.48 g mg−1. min−1. When the adsorbent was modified with 25% NH4OH, the specific surface area increased to 14.16 m2g-1 with a concomitant increase in the rate constant, K2, to 181.82 g mg−1. min−1. The rate of adsorption is therefore judged to be dependent on the specific surface area of the adsorbents. Future studies must therefore examine possible ways of ameliorating the specific surface areas of the watermelon rind based adsorbents, especially using environmentally benign modifiers.
The availability of competing ions in solution, especially in industrial effluents significantly affect the uptake of ionic pollutants by adsorbent (Han et al., 2007). The size of these competing ions is also important, as smaller ions are more mobile, and outcompete larger ions for the active sites on the adsorbent surface (Shee et al., 2014). Generally, when the electrostatic forces between an adsorbent and an adsorbate are attractive, an increase in ionic strength lowers the adsorption capacity (Yunusa et al., 2021). In a study to investigate the removal of Ni(II) and Co(II) from aqueous solution using watermelon rind based adsorbent (Lakshmipathy and Sarada, 2013), the effect of competing ions was investigated. Watermelon rind is known to be rich in ions of K, Na, Mg, Ca, Zn and Fe. These ions influenced the uptake of pollutants by blocking the active adsorption sites or by creating repulsive effect thereby minimizing the ion exchange capacity of the adsorbent. The study reported that a total cation content released by WMR was 2.102 mg g−1. The investigation also revealed that the presence of the metal ions in solution showed noticeable effect on adsorption rate for Ni(II) and Co(II) ions.
The adsorbent dosage is linkable to the cost of waste removal. Generally, it has been observed that the percentage removal of pollutants increases with increased adsorbent dosage as more adsorption sites are available for pollutant uptake, the contrary is observed with adsorption capacity. This has been attributed to agglomeration of the active sites on the adsorbent (Tome et al., 2023; Onyango et al., 2024). The reviewed studies are in consensus that increased adsorbent dosage improves the removal efficiency of pollutants while the adsorbents overall capacity for pollutant uptake is diminished. Therefore, in determining the amount of adsorbent to use for a particular process key considerations need to be made. Highly concentrated pollutants may require the application of higher quantities of the adsorbent. Similarly, pollutants that require complete removal such as highly toxic pollutants inevitably require a higher adsorbent dose. Other factors worth consideration are the cost of the adsorbent and its regeneration potential.
In the same way, a high agitation rate can improve adsorption of molecules onto adsorbents by reducing the boundary layer effect or by increasing the surface area of exposure. Conversely, an increased agitation rate can enhance desorption of physically adsorbed molecules. Optimization of the agitation rate is therefore as important operational parameter in adsorption studies as the aforementioned factors.
Whereas the aforementioned conditions have conventionally been used to optimize adsorption processes, an overreliance on their use is clearly implied in the reviewed studies. Contemporary techniques must therefore be adopted for improved efficiency and reliability of the interpretation of adsorption data. Machine learning (ML) is an efficient and adaptive technique capable of handling large, complex and multilinear data optimization, and has recently gained acceptance in optimization of adsorption studies. However, its application in watermelon rind-based adsorbents has not been reported leaving a clear gap in its applicability. Nonetheless, statistical optimization methods such as response surface methodology (RSM) have been used in enhancing the adsorption performance of watermelon rind-based adsorbents (Ahmad et al., 2017; El-Shafie et al., 2021). The use of modern technologies and statistical applications including artificial intelligence therefore need to be applied in optimizing adsorption of watermelon rind-based adsorbents. Again, whereas some studies have reported disparities in the equations used to calculate thermodynamic functions (Kumar and Sivanesan, 2005; Foo and Hameed, 2010), the authors did not obtain enough data to report on this aspect. This was mainly because nearly all the studies that reported the thermodynamic functions did not provide the equations used to determine these functions.
The adsorptive performance of watermelon rind-based adsorbents has been evaluated under diverse water quality conditions, including synthetic wastewater and natural water conditions. The outcome of these laboratory experiments is crucial in extrapolating laboratory findings to real world applications. Li and co-workers compared the removal efficiency of watermelon rind based adsorbents for thallium(I), a toxic metal, from synthetic wastewater and natural wastewater (Li H. et al., 2019). The study carefully compared the removal efficiencies under various conditions and reported a lower removal efficiency in natural wastewater (∼87% at maximum adsorbent dosage), compared to ∼99% removal from synthetic wastewater. This difference was attributed to the presence of co-existing metal ions effectively competing for the active adsorption sites on the adsorbents. In another study, watermelon rind was used as a coagulant in synthetic and real wastewater treatment (Muhammad et al., 2020). This study reported turbidity reduction in synthetic and real wastewater at 88% and 38% respectively. From the two studies, it is clear that real wastewater introduces challenges not presented by synthetic wastewaters. The presence of other contaminants in real wastewaters such as competing metal ions and organic matter reduce the efficiency of the watermelon rind based adsorbents by limiting the uptake of the target pollutants. For this reason, future studies must compare the efficacy of this class of adsorbents both for synthetic and industrial wastewaters to validate the laboratory findings. In the course of doing this, future studies must explore the possibility of designing pollutant selective adsorbents for industrial scale use.
7 Regeneration and reusability
To obtain an efficient and cost-effective adsorption process, the adsorbent needs to be recyclable after numerous sorption and desorption cycles (Singh and Singhal, 2015). From the reviewed studies, it is evident that a number of eluents of diverse nature have been used to regenerate spent watermelon rind-based adsorbents. This predominantly included acids such as H2SO4, HNO3, HCl and CH3COOH, NaOH was the only alkaline eluent reported while water was the only neutral eluent as shown in Table 6. It is apparent that the most effective eluents for the recovery of the spent adsorbents were either acidic or alkaline. However, acidic and alkaline eluents present numerous environmental and economic concerns they can also be costly over repeated cycles. This has the potential to increase operational costs in large scale application. Secondly, the use of such eluents eventually produce highly acidic or alkaline wastewaters raising environmental concerns with regard to disposal of such wastewaters. To avert this situation, more financial resources will be required for the pre-discharge treatment of such wastewaters. Pre-discharge treatment generally increases the operational costs in large scale applications. Acidic eluents are corrosive and may increase the cost of maintenance of the equipment, further increasing costs of operation. The toxic nature of NO2 from HNO3 eluents present a health risk calling for strict safety protocols which may present additional costs. Finally, the organic nature of the components of watermelon rind imply increased risk of degradation when such chemical eluents are used. Considering the aforementioned limitations, future studies must focus on the development of eluents that are more versatile, less costly and more environmentally friendly. Organic acids such as citric acid could be explored. Citric acid has already been used as a regenerant with considerable success (Wang et al., 2022), but with potential for further investigations. Again, physical regeneration techniques such as thermal techniques could be investigated and biodegradable eluents could also be developed.
8 Environmental and techno-economic feasibility
The use of watermelon rind provides both an environmental and economic practicability. The availability of watermelon, a tropical seasonal fruit, is at the core of this viability. Watermelon waste is readily available and therefore an inexpensive potential adsorbent. Moreover, the rind, mostly considered an unusable waste is ordinarily disposed of in landfills as solid waste. Its use as an adsorbent in its raw form or in activated or functionalized forms offers a sustainable way of co-valorization by converting waste to resource. Furthermore, the preparation of watermelon based adsorbents is an inexpensive venture, especially using the unmodified peels, since this may involve basic steps such as washing and sun drying. This offers sustainable and cost effective alternative to synthetic adsorbents. Some forms of chemical activation such as the use of acids, mostly sulphuric acid, are also economically feasible, but may present environmental challenges, most commonly in post use disposal. However, many studies have demonstrated the potential to regenerate and reuse such adsorbents, countering, albeit minimally, any environmental challenges that may be presented. Finally, even though limited studies have ventured into industrial scale use, watermelon based adsorbents present potential for scale-up for use to remove pollutants beyond the laboratory setting. To conclusively address the question of economic feasibility, a cost-benefit analysis study needs to be conducted. A cost analysis of a chitosan functionalized watermelon rind-based adsorbent was analyzed in comparison with activated carbon (Bamisaye et al., 2024). The study reported that the activated carbon, considering all costs, was over three times more expensive than the watermelon rind-based adsorbent confirming that this adsorbent was significantly cheaper than activated carbon. This notwithstanding, no studies have reported a cost analysis of the watermelon rind-based adsorbent, leaving a clear gap in such knowledge.
9 Challenges, limitations and possible solutions
This paper reviewed studies in which watermelon rind was used as an adsorbent for the adsorption of a number of pollutants including toxic dyes and heavy metals. Despite the excellent results, the use of watermelon rinds as an adsorbent is not without limitations. The review herein highlights a few of these limitations, and the possible ways to overcome them.
First, despite the fact that unmodified watermelon based adsorbent is reported to be highly efficient in remotion of a number of pollutants, many studies still reported the use of physical or chemical modifications to make it a more versatile adsorbent. Such modifications come with numerous challenges. Carbonization, for instance, is an energy intensive process and therefore costly. Other chemicals required for modification, and as reported in literature, are either expensive, or toxic, raising concerns over their cost and environmental consciousness, especially in an industrial scale. To overcome this challenge, green and sustainable modification techniques could be adopted. Biodegradable biopolymers such as chitosan and starch can be used to functionalize the watermelon rind-based adsorbents. Again, low-energy carbonization techniques such as solar powered carbonization should be explored. Optimization of the adsorptive properties of raw watermelon rind using machine learning tools should also be investigated. Secondly, most studies reported adsorption results in synthetic wastewater. There exists a dearth of data on the application of watermelon-based adsorbents in real wastewater treatment, leading to gaps in the knowledge of their performance in industrial scale application or pilot-scale. Many studies herein reported the use of batch adsorption studies which has inherent limitations (Patel, 2022). Very few studies have reported the use of fixed-bed (column) processes which has more industrial relevance. The adoption of fixed-bed processes come with continuous-flow reactors that are more efficient in terms of cost, thus ore studies need to venture into this area. Additionally, there is an apparent overreliance on the isotherms and kinetic models to predict the mechanism of the adsorption. Few studies delved deep into the uneasy question of the mechanisms that controlled the adsorption of the studied pollutants on to the watermelon rind based adsorbents. The use of simulation and computational models could help effectively describe the nuances of the nature of interaction between the adsorbent and the adsorbate, with minimal need to rely solely on inferences from equilibrium data. Computational methods have hardly been used to determine the adsorption mechanism. The authors recommend a venture into computational methods such as density functional theory (DFT) or molecular dynamics (DM). Furthermore, the aforementioned methods could be amalgamated to obtain a more comprehensive understanding of the adsorption mechanism. Again, the studies reported the use of non-biodegradable and costly eluents in the regeneration of the spent adsorbents. Future studies should focus on more environmentally friendly and less costly alternative regenerants for the recovery of watermelon rind-based adsorbents. The use of citric acid as a regenerant could be further explored. Furthermore, it is important to conduct a cost analysis of the synthesized adsorbents to confirm the techno-economic feasibility. In addition to this, no studies have reported the life cycle analysis (LCA) and possible disposal of watermelon rind-based adsorbents. This has left a gap in the knowledge of the environmental effects of these adsorbents from synthesis to disposal. Such gaps will form prospective areas of research for future studies. Finally, the optimization of the adsorption process mainly relied on traditional methods of optimization using kinetic, thermodynamic and isotherms data in addition to optimization of adsorption conditions such as pH and regeneration studies. Few studies reported statistical optimization methods such as response surface methodology (RSM) while no study reported contemporary methods such as machine learning (ML). These techniques could provide a breakthrough in optimizing adsorption processes and provide a deeper understanding on the drivers of the adsorption processes. To this end, the application of watermelon rind based adsorbents presents a huge potential for use in removal of pollutants. If this is to be achieved exhaustively, the aforementioned areas must be addressed by future studies.
10 Future perspectives
As can be predicted from the growing trend in the use of watermelon rind-based adsorbents (Figure 1), the increasing use of this class of bio-adsorbents is self-evident. Furthered by the growing desire to a clean environment and the increasing need for clean water, the use of bio-adsorbents is going to be on the rise. Particularly, the development of green bio-adsorbents with minimal or no chemical modification is going to take the centre stage in development of adsorbents based on watermelon peel. The expansion of the application of the watermelon rind-based adsorbents to include sequestration of emerging contaminants and bacteriological pollutants will be a high potential area for exploration. Furthermore, future undertakings will witness a digression from traditional optimization techniques using isotherms and thermodynamics, and delve into contemporary statistical optimization methods such as RSM and the use of artificial intelligence including machine learning models. Such techniques will be efficient and less costly especially in industrial scale application. Additionally, a combination of kinetics, isotherms, thermodynamics, regeneration studies and effect of adsorption conditions with computational simulations using first principles will be required for comprehensive description of adsorption mechanisms. Again, future studies will predictably focus on the development of eluents that are more versatile, less costly and more environmentally friendly. The use of organic acids such as citric acids and physical regeneration techniques such as microwave irradiation could replace mineral acids predominantly in use currently. Future adsorption studies should be aligned to both the principles of green chemistry and circular economy. Pilot-scale studies in continuous flowing systems are critically needed to gain practical insight on the sustainability of the proposed technology.
11 Conclusion
Watermelon rind is an agricultural waste that is naturally available, cost-effective and eco-friendly. Compositionally, watermelon rind has no reported toxicity, but has an abundance of functional groups such as carbonyl, carboxyl, hydroxyl and amine groups which offer active sites for pollutant adsorption. For this reason, watermelon rind shows considerable adsorption capacities both for organic and inorganic pollutants. Furthermore, the preparation of raw watermelon rind is significantly easy making it a simple adsorbent easily applicable against a wide array of pollutants. This review shows that watermelon rind-based adsorbents are effective in the removal of many pollutants from water, a plausible cause for the increased publications on its use. The study reveals that the most common adsorbates examined are heavy metals and dyes. The study also reveals that the functional groups that pre-exist in watermelon, coupled with its high porosity and surface area, are important in the interaction of the adsorbent and the pollutants. Cationic dyes are predominantly adsorbed through electrostatic interactions while heavy metal ion uptake is mainly by complexation and ion exchange. Because of the functional group density, the adsorptive action of watermelon rind is predominantly against cationic dyes. The functional groups can be enhanced by chemical activation and functionalization. Furthermore, other physical properties such as specific surface area can be improved by carbonization. Watermelon rind is also techno-economically feasible because of its availability, low cost and simple preparation. The use of watermelon rind based adsorbents aligns with key waste management strategies (waste to resource) by converting agricultural waste to a treasured adsorbent. However, pilot-scale application is only possible following more techno-economic studies, life-cycle assessment, evaluation of sequestration potential for bacteriological contaminants, and use in real wastewater treatment.
Author contributions
CO: Investigation, Methodology, Writing – original draft. WN: Formal Analysis, Supervision, Writing – review and editing. GT: Writing – review and editing. VS: Conceptualization, Formal Analysis, Supervision, Writing – review and editing.
Funding
The author(s) declare that no financial support was received for the research and/or publication of this article.
Conflict of interest
The authors declare that the research was conducted in the absence of any commercial or financial relationships that could be construed as a potential conflict of interest.
Generative AI statement
The author(s) declare that no Generative AI was used in the creation of this manuscript.
Publisher’s note
All claims expressed in this article are solely those of the authors and do not necessarily represent those of their affiliated organizations, or those of the publisher, the editors and the reviewers. Any product that may be evaluated in this article, or claim that may be made by its manufacturer, is not guaranteed or endorsed by the publisher.
References
Abd-Alhamid, R. R., Youssef, W., Khalil, M., Mohamed, I., and Shiha, A. A. (2024). A dynamic study on removal some heavy elements using natural orange peels as low-cost subsequent adsorbent from agricultural waste. Zagazig J. Agric. Res. 51 (3), 493–504. doi:10.21608/zjar.2024.367204
Adebowale, K., Egbedina, A., and Shonde, B. (2020). Adsorption of lead ions on magnetically separable Fe3O4 watermelon composite. Appl. Water Sci. 10 (10), 225–233. doi:10.1007/s13201-020-01307-y
Ahmad, M. A., Ahmad, N., and Bello, O. S. (2017). Statistical optimization of adsorption process for removal of synthetic dye using watermelon rinds. J. Model. Earth Syst. Environ. 3 (25), 25–29. doi:10.1007/s40808-017-0269-0
Åkerholm, M., Hinterstoisser, B., and Salmén, L. (2004). Characterization of the crystalline structure of cellulose using static and dynamic FT-IR spectroscopy. Carbohydr. Res. 339 (3), 569–578. doi:10.1016/j.carres.2003.11.012
Al-Ghouti, M. A., and Da'ana, D. A. (2020). Guidelines for the use and interpretation of adsorption isotherm models: a review. J. Hazard. Mater. 393, 122383. doi:10.1016/j.jhazmat.2020.122383
Aljeboree, A., Gs, H., Aa, K., Mm, A., Ha, L., Amb, A.-D., et al. (2022). Highly adsorbent surface from watermelon peels: as non-conventional low-cost sorbent; Equilibrium and Recycle study. IOP Conf. Ser. Earth Environ. Sci. 1029, 012008. doi:10.1088/1755-1315/1029/1/012008
Aljeboree, A. M., Alshirifi, A. N., and Alkaim, A. F. (2019). Removal of Pharmaceutical Amoxicillin drug by using (CNT) decorated Clay/Fe 2 O 3 Micro/Nanocomposite as effective adsorbent: process optimization for ultrasound-assisted adsorption. Int. J. Pharm. Res. 11 (4), 80. doi:10.31838/ijpr/2019.11.04.012
Allen, S., and Koumanova, B. (2005). Decolourisation of waterwastewater using adsorption. J. Univ. Chem. Technol. Metallurgy 40 (3), 175–192.
Altowayti, W. a. H., Othman, N., Al-Gheethi, A., Dzahir, N. H. B. M., Asharuddin, S. M., Alshalif, A. F., et al. (2021). Adsorption of Zn2+ from synthetic wastewater using dried watermelon rind (D-WMR): an overview of nonlinear and linear regression and error analysis. Molecules 26 (20), 6176. doi:10.3390/molecules26206176
Annadurai, G., Juang, R. S., and Lee, D. J. (2003). Adsorption of heavy metals from water using banana and orange peels. Water Sci. Technol. 47 (1), 185–190. doi:10.2166/wst.2003.0049
Archibong, U. D., Ikpe, E. E., and Amienghemhen, O. D. (2024). Adsorption of copper and cadmium from wastewater using chemically activated watermelon peels as adsorbent. Chem. Sci. Int. J. 33 (1), 1–11. doi:10.9734/CSJI/2024/v33i1879
Babel, S., and Kurniawan, T. A. (2004). Cr(VI) removal from synthetic wastewater using coconut shell charcoal and commercial activated carbon modified with oxidizing agents and/or chitosan. Chemosphere 54 (7), 951–967. doi:10.1016/j.chemosphere.2003.10.001
Bachmann, S., Dávila, I., Calvete, T., and Féris, L.Technology (2022). Adsorption of Cr (VI) on lignocellulosic wastes adsorbents: an overview and further perspective. Int. J. Environ. Sci. 19 (12), 12727–12748. doi:10.1007/s13762-022-03928-z
Badiei, A., Mirahsani, A., Shahbazi, A., Younesi, H., and Alizadeh, M. (2014). Adsorptive removal of toxic dye from aqueous solution and real industrial effluent by tris (2-aminoethyl) amine functionalized nanoporous silica. Environ. Prog. Sustain. Energy 33 (4), 1242–1250. doi:10.1002/ep.11923
Bamisaye, A., Adesina, M. O., Alfred, M. O., Ige, A. R., Idowu, M. A., and Adegoke, K. A. (2023). Kinetic and mechanistic study of the biosorption of Copper(II) Ion from wastewater using porous Chitosan-Walnut shell composite. Chem. Data Collect. 45, 101024. doi:10.1016/j.cdc.2023.101024
Bamisaye, A., Adesina, M. O., Ige, A. R., Alli, Y. A., Adeniyi, O. M., Idowu, M. A., et al. (2024). Adsorption of Cu(II) ion from aqueous solution onto mesoporous chitosan-functionalized watermelon rind composite. Biomass Convers. Biorefinery. doi:10.1007/s13399-024-05959-7
Banerjee, K., Ramesh, S., Nidheesh, P., and Bharathi, K.Environment (2012). A novel agricultural waste adsorbent, watermelon shell for the removal of copper from aqueous solutions. Iran. J. energy 3 (2), 143–156. doi:10.5829/idosi.ijee.2012.03.02.0396
Bhakta, J. N., and Ali, M. M. (2020). in Arsenic water resources contamination: challenges and solutions. Editors A. Fares, and S. K. Singh (Cham: Springer International Publishing), 207–230.
Bibaj, E., Lysigaki, K., Nolan, J. W., Seyedsalehi, M., Deliyanni, E. A., Mitropoulos, A. C., et al. (2019). Activated carbons from banana peels for the removal of nickel ions. Int. J. Environ. Sci. Technol. 16 (2), 667–680. doi:10.1007/s13762-018-1676-0
Chaitra, G., Sumana, Y., and Praveen Kumar, G. (2022). Removal efficiency of methylene blue dye using chemically activated watermelon rinds as a low-cost adsorbent. Int. Res. J. Eng. Technol. (IRJET) 9 (9), 1157–1160.
Chaudhari, S. A., and Singhal, R. S. (2015). Cutin from watermelon peels: a novel inducer for cutinase production and its physicochemical characterization. Int. J. Biol. Macromol. 79, 398–404. doi:10.1016/j.ijbiomac.2015.05.006
Chen, X., Lin, Q., He, R., Zhao, X., and Li, G. (2017). Hydrochar production from watermelon peel by hydrothermal carbonization. Bioresour. Technol. 241, 236–243. doi:10.1016/j.biortech.2017.04.012
Crini, G., Lichtfouse, E., Wilson, L. D., and Morin-Crini, N. (2019). Conventional and non-conventional adsorbents for wastewater treatment. Environ. Chem. Lett. 17 (1), 195–213. doi:10.1007/s10311-018-0786-8
Dąbrowski (2001). Adsorption—from theory to practice. Adv. Colloid Interface Sci. 93 (1-3), 135–224. doi:10.1016/S0001-8686(00)00082-8
Dai, Y., Sun, Q., Wang, W., Lu, L., Liu, M., Li, J., et al. (2018). Utilizations of agricultural waste as adsorbent for the removal of contaminants: a review. Chemosphere 211, 235–253. doi:10.1016/j.chemosphere.2018.06.179
Devanathan, R., Vivekanandan, B., Rengadurai, S., Balaji, G. L., and Lakshmipathy, R. (2022). Efficiency of Watermelon rind towards adsorption of Ce (III) and Pr (III) ions. Mater. Today Proc. 55, 253–258. doi:10.1016/j.matpr.2021.06.429
Dhaouadi, F., Sellaoui, L., Reynel-Ávila, H. E., Landín-Sandoval, V., Mendoza-Castillo, D. I., Jaime-Leal, J. E., et al. (2021). Adsorption mechanism of Zn(2+), Ni(2+), Cd(2+), and Cu(2+) ions by carbon-based adsorbents: interpretation of the adsorption isotherms via physical modelling. Environ. Sci. Pollut. Res. Int. 28 (24), 30943–30954. doi:10.1007/s11356-021-12832-x
Dilekoglu, M. F. (2016). Use of genetic algorithm optimization technique in the adsorption of phenol on banana and grapefruit peels. J. Chem. Soc. Pak. 38 (6), 1252–1262.
Dinh, V.-P., Le, H. M., Nguyen, V.-D., Dao, V.-A., Hung, N. Q., Tuyen, L. A., et al. (2019). Insight into the adsorption mechanisms of methylene blue and chromium (III) from aqueous solution onto pomelo fruit peel. RSC Adv. 9 (44), 25847–25860. doi:10.1039/C9RA04296B
Egbedina, A. O., Olu-Owolabi, B. I., and Adebowale, K. O. (2023). Batch and continuous fixed-bed adsorption of antibiotics from aqueous solution using stearic acid-activated carbon composite. Energy, Ecol. Environ. 8 (2), 129–140. doi:10.1007/s40974-023-00268-7
Ekloh, E., and Yafetto, L. (2024). Fermentation and valorization of watermelon (Citrullus lanatus) rind wastes into livestock feed using Aspergillus Niger and Mucor sp. Sci. Afr. 23, 020355–e2110. doi:10.1016/j.sciaf.2023.e02035
El Nemr, M. A., Abdelmonem, N. M., Ismail, I. M. A., Ragab, S., and El-Nemr, A. (2020). Removal of Acid Yellow 11 dye using a novel modified biochar derived from watermelon peels. Desalination Water Treat. 203, 403–431. doi:10.5004/dwt.2020.26207
El-Nemr, M. A., Yilmaz, M., Ragab, S., and El Nemr, A. (2022). Watermelon peels biochar-S for adsorption of Cu2+ from water. Desalination Water Treat. 261, 195–213. doi:10.5004/dwt.2022.28506
El-Shafie, A. S., Hassan, S. S., Akther, N., and El-Azazy, M. (2021). Watermelon rinds as cost-efficient adsorbent for acridine orange: a response surface methodological approach. Environ. Sci. Pollut. Res. 30, 71554–71573. doi:10.1007/s11356-021-13652-9
El-Tawabty, D. S., Abd El-Aleem, I. M., Ahmed, S. S., El-Hadary, A. E., and Ali, R. M. (2024). Hexavalent chromium adsorption by watermelon peels from tannery wastewater. Egypt. J. Chem. 67 (13), 241–250. doi:10.21608/ejchem.2024.257332.9027
Erugo, A. I., Sunday, K., and Olusesi, F. Y. (2022). Optimization of bio-fertilizer production from watermelon peels using response surface methodology. Chem. Eng. Industrial Chem. doi:10.26434/chemrxiv-2022-cttd7
Foo, K. Y., and Hameed, B. H. (2010). Insights into the modeling of adsorption isotherm systems. Chem. Eng. J. 156 (1), 2–10. doi:10.1016/j.cej.2009.09.013
Freundlich, H. M. F. (1906). Over the adsorption in solution. J. Phys. Chem. 57 (385471), 1100–1107.
Gorgulu, A., and Gorgulu, Y. F. (2025). Production of sustainable food ingredients from watermelon wastes using drum-drying. J. Food Process Eng. 48 (1), 1–12. doi:10.1111/jfpe.70040
Guo, Y., and Rockstraw, D. A. (2006). Physical and chemical properties of carbons synthesized from xylan, cellulose, and Kraft lignin by H3PO4 activation. Carbon 44 (8), 1464–1475. doi:10.1016/j.carbon.2005.12.002
Gupta, V., and Suhas, (2009). Application of low-cost adsorbents for dye removal–a review. J. Environ. Manag. 90 (8), 2313–2342. doi:10.1016/j.jenvman.2008.11.017
Hadi, Z. A., Aljeboree, A., and Alkaim, A. (2014). Adsorption of a cationic dye from aqueous solutions by using waste glass materials: isotherm and thermodynamic studies. Int. J. Chem. Sci. 12 (4), 1273–1288.
Han, R., Zou, W., Yu, W., Cheng, S., Wang, Y., and Shi, J. (2007). Biosorption of methylene blue from aqueous solution by fallen phoenix tree's leaves. J. Hazard. Mater. 141 (1), 156–162. doi:10.1016/j.jhazmat.2006.06.107
Ho, Y.-S. (2006). Review of second-order models for adsorption systems. J. Hazard. Mater. 136 (3), 681–689. doi:10.1016/j.jhazmat.2005.12.043
Ho, Y. S., and Mckay, G. (1998). Sorption of dye from aqueous solution by peat. Chem. Eng. J. 70 (2), 115–124. doi:10.1016/S0923-0467(98)00076-1
Hussain, M. S., Rehman, R., Imran, M., Dar, A., Akram, M., Al-Abbad, E. A., et al. (2022). Eco-friendly detoxification of Congo red dye from water by citric acid activated bioadsorbents consisting of watermelon and water chestnuts peels collected from indigenous resources. Adsorpt. Sci. and Technol. 2022, 9056288. doi:10.1155/2022/9056288
Iqbal, M., Asma, S., and And Kalim, I. (2009). Characterization of adsorptive capacity and investigation of mechanism of Cu2+, Ni2+ and Zn2+ adsorption on mango peel waste from constituted metal solution and genuine electroplating effluent. Sep. Sci. Technol. 44 (15), 3770–3791. doi:10.1080/01496390903182305
Isik, Z., Saleh, M., M’barek, I., Yabalak, E., Dizge, N., and Deepanraj, B. (2024). Investigation of the adsorption performance of cationic and anionic dyes using hydrochared waste human hair. Biomass Convers. Biorefinery 14 (3), 3715–3728. doi:10.1007/s13399-022-02582-2
Istratie, R., Stoia, M., Păcurariu, C., and Locovei, C. (2019). Single and simultaneous adsorption of methyl orange and phenol onto magnetic iron oxide/carbon nanocomposites. Arabian J. Chem. 12 (8), 3704–3722. doi:10.1016/j.arabjc.2015.12.012
Jasim, L. S., and Aljeboree, A. M. (2021). Removal of heavy metals by using chitosan/poly (acryl amide-acrylic acid) hydrogels: characterization and kinetic study. Neuro Quantology 19 (2), 31–37. doi:10.14704/nq.2021.19.2.nq21014
Jawad, A. H., Kadhum, A. M., and Ngoh, Y. S. (2018a). Applicability of dragon fruit (Hylocereus polyrhizus) peels as low-cost biosorbent for adsorption of methylene blue from aqueous solution: kinetics, equilibrium and thermodynamics studies. Desalination Water Treat. 109, 231–240. doi:10.5004/dwt.2018.21976
Jawad, A. H., Ngoh, Y., and Radzun, K. A. (2018b). Utilization of watermelon (Citrullus lanatus) rinds as a natural low-cost biosorbent for adsorption of methylene blue: kinetic, equilibrium and thermodynamic studies. J. Taibah Univ. Sci. 12 (4), 371–381. doi:10.1080/16583655.2018.1476206
Jawad, A. H., Razuan, R., Appaturi, J. N., and Wilson, L. D.Interfaces (2019). Adsorption and mechanism study for methylene blue dye removal with carbonized watermelon (Citrullus lanatus) rind prepared via one-step liquid phase H2SO4 activation. Surfaces 16, 76–84. doi:10.1016/j.surfin.2019.04.012
Jiang, J., Zhang, S., Li, S., Zeng, W., Li, F., and Wang, W. (2022). Magnetized manganese-doped watermelon rind biochar as a novel low-cost catalyst for improving oxygen reduction reaction in microbial fuel cells. Sci. Total Environ. 802, 149989. doi:10.1016/j.scitotenv.2021.149989
Kanungo, S. B., Tripathy, S. S., Mishra, S. K., Sahoo, B., and Rajeev, (2004). Adsorption of Co2+, Ni2+, Cu2+, and Zn2+ onto amorphous hydrous manganese dioxide from simple (1–1) electrolyte solutions. J. colloid interface Sci. 269 (1), 11–21. doi:10.1016/j.jcis.2003.07.002
Katal, R., Ghiass, M., and Esfandian, H. (2011). Application of nanometer size of polypyrrole as a suitable adsorbent for removal of Cr (VI). J. Vinyl Addit. Technol. 17 (3), 222–230. doi:10.1002/vnl.20287
Kumar, A. K., and Sharma, S. (2017). Recent updates on different methods of pretreatment of lignocellulosic feedstocks: a review. Bioresour. Bioprocess. 4 (1), 7. doi:10.1186/s40643-017-0137-9
Kumar, K. V., and Sivanesan, S. (2005). Comparison of linear and non-linear method in estimating the sorption isotherm parameters for safranin onto activated carbon. J. Hazard. Mater. 123 (1-3), 288–292. doi:10.1016/j.jhazmat.2005.03.040
Kyzas, G. Z., and Kostoglou, M. (2014). Green adsorbents for wastewaters: a critical review. Materials 7 (1), 333–364. doi:10.3390/ma7010333
Lakshmipathy, R., and Sarada, N. (2013). Application of watermelon rind as sorbent for removal of nickel and cobalt from aqueous solution. Int. J. Mineral Process. 122, 63–65. doi:10.1016/j.minpro.2013.03.002
Lakshmipathy, R., and Sarada, N. C. (2016). Methylene blue adsorption onto native watermelon rind: batch and fixed bed column studies. Desalination Water Treat. 57 (23), 10632–10645. doi:10.1080/19443994.2015.1040462
Lam, S. S., Liew, R. K., Wong, Y. M., Yek, P. N. Y., Ma, N. L., Lee, C. L., et al. (2017). Microwave-assisted pyrolysis with chemical activation, an innovative method to convert orange peel into activated carbon with improved properties as dye adsorbent. J. Clean. Prod. 162, 1376–1387. doi:10.1016/j.jclepro.2017.06.131
Langmuir, I. (1916). The constitution and fundamental properties of solids and liquids. Part I. Solids. J. Am. Chem. Soc. 38 (11), 2221–2295. doi:10.1021/ja02268a002
Lasheen, M., El-Sherif, I. Y., Sabry, D. Y., El-Wakeel, S., and El-Shahat, M. (2016). Adsorption of heavy metals from aqueous solution by magnetite nanoparticles and magnetite-kaolinite nanocomposite: equilibrium, isotherm and kinetic study. Desalination Water Treat. 57 (37), 17421–17429. doi:10.1080/19443994.2015.1085446
Lee, S.-Y., and Choi, H.-J. (2021). Efficient adsorption of methylene blue from aqueous solution by sulfuric acid activated watermelone rind (Citrullus lanatus). Appl. Chem. Eng. 32 (3), 348–356. doi:10.14478/ace.2021.1030
Letechipia, J. O., González-Trinidad, J., Júnez–Ferreira, H. E., Bautista–Capetillo, C., Robles Rovelo, C. O., and Contreras Rodríguez, A. R. (2023). Removal of arsenic from semiarid area groundwater using a biosorbent from watermelon peel waste. Heliyon 9 (2), e13251. doi:10.1016/j.heliyon.2023.e13251
Li, H., Xiong, J., Xiao, T., Long, J., Wang, Q., Li, K., et al. (2019a). Biochar derived from watermelon rinds as regenerable adsorbent for efficient removal of thallium(I) from wastewater. Process Saf. Environ. Prot. 127, 257–266. doi:10.1016/j.psep.2019.04.031
Li, J., Li, H., Yuan, Z., Fang, J., Chang, L., Zhang, H., et al. (2019b). Role of sulfonation in lignin-based material for adsorption removal of cationic dyes. Int. J. Biol. Macromol. 135, 1171–1181. doi:10.1016/j.ijbiomac.2019.06.024
Limousin, G., Gaudet, J.-P., Charlet, L., Szenknect, S., Barthes, V., and Krimissa, M. (2007). Sorption isotherms: a review on physical bases, modeling and measurement. Appl. Geochem. 22 (2), 249–275. doi:10.1016/j.apgeochem.2006.09.010
Lou, Z., Xing, S., Xiao, X., Shan, W., Xiong, Y., and Fan, Y. (2018). Selective adsorption of Re(VII) by chitosan modified with imidazolium-based ionic liquid. Hydrometallurgy 179, 141–148. doi:10.1016/j.hydromet.2018.05.025
Low, W., Kevin, S., and Lee, H. (2023). Adsorption of zinc, copper, and iron from synthetic wastewater using watermelon (Citrullus Lanatus), Mango (Mangifera Indica L) and rambutan peels (Nephelium Lappaceum L) as bio-sorbents. J. Eng. Sci. Technol. 18 (1), 386–405.
Magaji, B., Maigari, A. U., Abubakar, U. A., Sani, M. M., and Maigari, A. U. (2020). Batch adsorption of safranin dye from an aqueous solution of balanites aegyptiaca seed coats. Asian J. Phys. Chem. Sci. 8 (1), 48–54. doi:10.9734/AJOPACS/2020/v8i130109
Malbenia, J. M., Benettayeb, A., Belkacem, M., Ruvimbo Mitchel, C., Hadj Brahim, M., Benettayeb, I., et al. (2024). An overview on the key advantages and limitations of batch and dynamic modes of biosorption of metal ions. Chemosphere 357, 142051. doi:10.1016/j.chemosphere.2024.142051
Mashkoor, F., and Nasar, A. (2020). Magsorbents: potential candidates in wastewater treatment technology–A review on the removal of methylene blue dye. J. magnetism magnetic Mater. 500, 166408. doi:10.1016/j.jmmm.2020.166408
Masoudian, N., Rajabi, M., and Ghaedi, M. (2019). Titanium oxide nanoparticles loaded onto activated carbon prepared from bio-waste watermelon rind for the efficient ultrasonic-assisted adsorption of Congo red and phenol red dyes from wastewaters. Polyhedron 173, 114105–114109. doi:10.1016/j.poly.2019.114105
Meseldzija, S., Petrovic, J., Onjia, A., Volkov-Husovic, T., Nesic, A., and Vukelic, N. (2019). Utilization of agro-industrial waste for removal of copper ions from aqueous solutions and mining-wastewater. J. Industrial Eng. Chem. 75, 246–252. doi:10.1016/j.jiec.2019.03.031
Mohammad, A., and Pa, M. N. (1997). Physico-chemical adsorption treatments for minimization of heavy metal contents in water and wastewaters. J. Sci. industrial Res. 56 (9), 523–539.
Molina-Calderón, L., Basualto-Flores, C., Paredes-García, V., and Venegas-Yazigi, D. (2022). Advances of magnetic nanohydrometallurgy using superparamagnetic nanomaterials as rare earth ions adsorbents: a grand opportunity for sustainable rare earth recovery. Sep. Purif. Technol. 299, 121708. doi:10.1016/j.seppur.2022.121708
Mort, A., Zheng, Y., Qiu, F., Nimtz, M., and Bell-Eunice, G. (2008). Structure of xylogalacturonan fragments from watermelon cell-wall pectin. Endopolygalacturonase can accommodate a xylosyl residue on the galacturonic acid just following the hydrolysis site. Carbohydr. Res. 343 (7), 1212–1221. doi:10.1016/j.carres.2008.03.021
Muhammad, A., Rashidi, A. R., Roslan, A., and Buddin, M. M. H. S. (2020). Performance study of watermelon rind as coagulants for the wastewater treatment. J. Phys.: Conf. Ser. 1535 012053. doi:10.1088/1742-6596/1535/1/012053
Nethaji, S., Sivasamy, A., and Mandal, A. (2013). Adsorption isotherms, kinetics and mechanism for the adsorption of cationic and anionic dyes onto carbonaceous particles prepared from Juglans regia shell biomass. Int. J. Environ. Sci. Technol. 10, 231–242. doi:10.1007/s13762-012-0112-0
Ngulube, T., Gumbo, J. R., Masindi, V., and Maity, A. (2017). An update on synthetic dyes adsorption onto clay based minerals: a state-of-art review. J. Environ. Manag. 191, 35–57. doi:10.1016/j.jenvman.2016.12.031
Nizam, N. U. M., Hanafiah, M. M., Mahmoudi, E., Mohammad, A. W., and Oyekanmi, A. A. (2022). Effective adsorptive removal of dyes and heavy metal using graphene oxide based Pre-treated with NaOH/H2SO4 rubber seed shells synthetic graphite Precursor: equilibrium Isotherm, kinetics and thermodynamic studies. Sep. Purif. Technol. 289, 120730. doi:10.1016/j.seppur.2022.120730
Ofomaja, A. E., and Ho, Y.-S. (2007). Equilibrium sorption of anionic dye from aqueous solution by palm kernel fibre as sorbent. Dyes Pigments 74 (1), 60–66. doi:10.1016/j.dyepig.2006.01.014
Okam, E. C., Odo, G. I., Uduma, C. K., Ijioma, G. U., and Okolo, B. I. (2022). Biosorption of chromium (II) ion from textile effluent using watermelon shell-activated carbon. Path Sci. 8 (9), 1018–1029. doi:10.22178/pos.85-2
Omar, N., Abdullah, E. C., Numan, A., Mubarak, N. M., Khalid, M., Aid, S. R., et al. (2022). Facile synthesis of a binary composite from watermelon rind using response surface methodology for supercapacitor electrode material. J. Energy Storage 49, 104147. doi:10.1016/j.est.2022.104147
Ong, S. T., Keng, P. S., Ooi, S. T., Hung, Y. T., and Lee, S. L. (2012). Utilization of fruits peel as a sorbent for removal of methylene blue. Asian J. Chem. 24 (1), 398.
Onyango, C., Nyairo, W., Kwach, B., Shikuku, V., Sylvain, T., Tamaguelon, H. D., et al. (2024). Synthesis of pumice and medical waste incinerator fly ash based phosphate geopolymers for methylene blue dye adsorption: co-valorization, parameters and mechanism. Mater. Adv. 5 (21), 8546–8563. doi:10.1039/D4MA00779D
Othman, N., Kueh, Y., Azizul-Rahman, F., and Hamdan, R. (2014). Watermelon rind: a Potential Adsorbent for zinc removal. Appl. Mech. 680, 146–149. doi:10.4028/www.scientific.net/AMM.680.146
Patel, H. (2022). Comparison of batch and fixed bed column adsorption: a critical review. Int. J. Environ. Sci. Technol. 19 (10), 10409–10426. doi:10.1007/s13762-021-03492-y
Pavan, F. A., Mazzocato, A. C., and Gushikem, Y. (2008). Removal of methylene blue dye from aqueous solutions by adsorption using yellow passion fruit peel as adsorbent. Bioresour. Technol. 99 (8), 3162–3165. doi:10.1016/j.biortech.2007.05.067
Popoola, L. T., Yusuff, A. S., Adeyi, A. A., and Omotara, O. O. (2022). Adsorptive removal of heavy metals from oil well produced water using citrullus lanatus peel: characterization and optimization. South Afr. J. Chem. Eng. 39, 19–27. doi:10.1016/j.sajce.2021.11.001
Prasanthi, M. R., Jayasravanthi, M., and Nadh, R. V. (2016). Kinetic, thermodynamic and equilibrium studies on removal of hexavalent chromium from aqueous solutions using agro-waste biomaterials, casuarina equisetifolia L. and sorghum bicolor. Korean J. Chem. Eng. 33, 2374–2383. doi:10.1007/s11814-016-0078-6
Rahman, A., Yoshida, K., Islam, M. M., and Kobayashi, G. (2023). Investigation of efficient adsorption of toxic heavy metals (chromium, lead, cadmium) from aquatic environment using orange peel cellulose as adsorbent. Sustainability 15 (5), 4470. doi:10.3390/su15054470
Saad, H., El-Dien, F. a. N., El-Gamel, N. E. A., and Abo Dena, A. S. (2022). Azo-functionalized superparamagnetic Fe3O4 nanoparticles: an efficient adsorbent for the removal of bromocresol green from contaminated water. RSC Adv. 12 (39), 25487–25499. doi:10.1039/D2RA03476J
Safari, E., Rahemi, N., Kahforoushan, D., and Allahyari, S. (2019). Copper adsorptive removal from aqueous solution by orange peel residue carbon nanoparticles synthesized by combustion method using response surface methodology. J. Environ. Chem. Eng. 7 (1), 102847. doi:10.1016/j.jece.2018.102847
Sankaran, R., Show, P. L., Ooi, C.-W., Ling, T. C., Shu-Jen, C., Chen, S.-Y., et al. (2020). Feasibility assessment of removal of heavy metals and soluble microbial products from aqueous solutions using eggshell wastes. Clean Technol. Environ. Policy 22 (4), 773–786. doi:10.1007/s10098-019-01792-z
Santoso, E., Ediati, R., Kusumawati, Y., Bahruji, H., Sulistiono, D., and Prasetyoko, D. (2020). Review on recent advances of carbon based adsorbent for methylene blue removal from waste water. Mater. Today Chem. 16, 100233. doi:10.1016/j.mtchem.2019.100233
Sartape, A. S., Mandhare, A. M., Jadhav, V. V., Raut, P. D., Anuse, M. A., and Kolekar, S. S. (2017). Removal of malachite green dye from aqueous solution with adsorption technique using Limonia acidissima (wood apple) shell as low cost adsorbent. Arabian J. Chem. 10, S3229–S3238. doi:10.1016/j.arabjc.2013.12.019
Sawant, P., and Dhabe, A. S. (2022). FT-IR analysis of In-vivo and In-vitro stem, leaf and callus of Ceropegia bulbosa roxb. var. bulbosa. BIOINFOLET-A Q. J. Life Sci. 19 (1), 46–50.
Shakor, Z. M., Mahdi, H. H., Al-Sheikh, F., Alwan, G. M., and Al-Jadir, T. (2021). Ni, Cu, and Zn metal ions removal from synthetic wastewater using a watermelon rind (Catullus landaus). Mater. Today Proc. 42, 2502–2509. doi:10.1016/j.matpr.2020.12.570
Shakya, A., and Agarwal, T. (2019). Removal of Cr(VI) from water using pineapple peel derived biochars: adsorption potential and re-usability assessment. J. Mol. Liq. 293, 111497. doi:10.1016/j.molliq.2019.111497
Shee, A., Onyari, J. M., Wabomba, J. N., and Munga, D. (2014). Methylene blue adsorption onto coconut husks/polylactide blended films: equilibrium and kinetic studies. Chem. Material Res. 6, 28–38.
Shikuku, V. O., and Jemutai-Kimosop, S. (2020). Efficient removal of sulfamethoxazole onto sugarcane bagasse-derived biochar: two and three-parameter isotherms, kinetics and thermodynamics. South Afr. J. Chem. 73 (1), 111–119.
Shimizu, F. L., De Azevedo, G. O., Coelho, L. F., Pagnocca, F. C., and Brienzo, M. (2020). Minimum lignin and xylan removal to improve cellulose accessibility. BioEnergy Res. 13, 775–785. doi:10.1007/s12155-020-10120-z
Shukla, S., Khan, R., Srivastava, M. M., and Zahmatkesh, S. (2024). Valorization of waste watermelon rinds as a bio-adsorbent for efficient removal of methylene blue dye from aqueous solutions. Appl. Biochem. Biotechnol. 196 (5), 2534–2548. doi:10.1007/s12010-023-04448-3
Singh, T., and Singhal, R. (2015). Methyl Orange adsorption by reuse of a waste adsorbent poly (AAc/AM/SH)-MB superabsorbent hydrogel: matrix effects, adsorption thermodynamic and kinetics studies. Desalination Water Treat. 53 (7), 1942–1956. doi:10.1080/19443994.2013.859098
Sips, R. (1948). On the structure of a catalyst surface. J. Chem. Phys. 16 (5), 490–495. doi:10.1063/1.1746922
Song, C., Wu, S., Cheng, M., Tao, P., Shao, M., and Gao, G. (2013). Adsorption studies of coconut shell carbons prepared by KOH activation for removal of lead (II) from aqueous solutions. Sustainability 6 (1), 86–98. doi:10.3390/su6010086
Supanchaiyamat, N., Jetsrisuparb, K., Knijnenburg, J. T., Tsang, D. C., and Hunt, A. (2019). Lignin materials for adsorption: current trend, perspectives and opportunities. Bioresour. Technol. 272, 570–581. doi:10.1016/j.biortech.2018.09.139
Taib, N. I., Rosli, N. A., Rejab, M. M., Rosdi, N. a. F. M., Aziz, N. a. A., and Halim, S. N. A. (2023). Kinetic and equilibrium modeling of low-cost adsorbent of untreated watermelon peel for adsorption of zinc(II). Desalination Water Treat. 306, 122–130. doi:10.5004/dwt.2023.29831
Taib, N. I., Rosli, N. A., Saharrudin, N. I., Rozi, N. M., Kasdiehram, N. a. A., and Nazri, N. N. T. A. (2021). Kinetic, equilibrium, and thermodynamic studies of untreated watermelon peels for removal of copper (II) from aqueous solution. Desalination Water Treat. 227, 289–299. doi:10.5004/dwt.2021.27299
Temkin, M. (1940). Kinetics of ammonia synthesis on promoted iron catalysts. Acta physiochim. URSS 12, 327–356.
Tome, S., Shikuku, V., Tamaguelon, H. D., Akiri, S., Etoh, M. A., Rüscher, C., et al. (2023). Efficient sequestration of malachite green in aqueous solution by laterite-rice husk ash-based alkali-activated materials: parameters and mechanism. Environ. Sci. Pollut. Res. 30, 67263–67277. doi:10.1007/s11356-023-27138-3
Tran, H. N., You, S.-J., Nguyen, T. V., and Chao, H.-P. (2017). Insight into the adsorption mechanism of cationic dye onto biosorbents derived from agricultural wastes. Chem. Eng. Commun. 204 (9), 1020–1036. doi:10.1080/00986445.2017.1336090
Unesco (2024). Editor R. Connor Available online at: https://coilink.org/20.500.12592/3j9kkc5.
Villabona-Ortíz, A., Tejada-Tovar, C., and González-Delgado, Á. D. (2022). Elimination of chromium (VI) and nickel (II) ions in a packed column using oil palm bagasse and yam peels. Water 14 (8), 1240. doi:10.3390/w14081240
Wang, J., and Guo, X. (2020). Adsorption isotherm models: classification, physical meaning, application and solving method. Chemosphere 258, 127279. doi:10.1016/j.chemosphere.2020.127279
Wang, Q., Wang, Y., Tang, J., Yang, Z., Zhang, L., and Huang, X. (2022). New insights into the interactions between Pb(II) and fruit waste biosorbent. Chemosphere 303 (Pt 1), 135048. doi:10.1016/j.chemosphere.2022.135048
Wanja, N. E., Murungi, J., Ali, A. H., and Wanjau, R. (2016). Efficacy of adsorption of Cu (II), Pb (II) and Cd (II) Ions onto acid activated watermelon peels biomass from water. Int. J. Sci. Res. 5 (8), 671–679. doi:10.21275/ART2016929
Weber, W. J., and Morris, J. C. (1963). Kinetics of adsorption on carbon from solution. J. Sanit. Eng. Div. 89 (2), 31–59. doi:10.1061/JSEDAI.0000430
Wei, Y., Li, P., Yang, C., Li, X., Yi, D., and Wu, W. (2023). Preparation of porous carbon materials as adsorbent materials from phosphorus-doped watermelon rind. Water 15 (13), 2433. doi:10.3390/w15132433
Wu, W., Yang, M., Feng, Q., Mcgrouther, K., Wang, H., Lu, H., et al. (2012). Chemical characterization of rice straw-derived biochar for soil amendment. Biomass bioenergy Res. 47, 268–276. doi:10.1016/j.biombioe.2012.09.034
Xiong, W., Tong, J., Yang, Z., Zeng, G., Zhou, Y., Wang, D., et al. (2017). Adsorption of phosphate from aqueous solution using iron-zirconium modified activated carbon nanofiber: performance and mechanism. J. Colloid Interface Sci. 493, 17–23. doi:10.1016/j.jcis.2017.01.024
Yahya, M. A., Al-Qodah, Z., and Ngah, C. W. Z. (2015). Agricultural bio-waste materials as potential sustainable precursors used for activated carbon production: a review. Renew. Sustain. Energy Rev. 46, 218–235. doi:10.1016/j.rser.2015.02.051
Yang, T., and Lua, A. C. (2006). Textural and chemical properties of zinc chloride activated carbons prepared from pistachio-nut shells. Mater. Chem. Phys. 100 (2), 438–444. doi:10.1016/j.matchemphys.2006.01.039
Yilmaz, O., and Tugrul, N. (2021). Zinc adsorption from aqueous solution using lemon, orange, watermelon, melon, pineapple, and banana rinds. Water Pract. Technol. 17 (1), 318–328. doi:10.2166/wpt.2021.102
Yuliusman, A. M. P., Hanafi, A., and Nafisah, A. R. (2020). Activated carbon preparation from durian peel wastes using chemical and physical activation. AIP Conf. Proc. 2230 (1). doi:10.1063/5.0002348
Yunusa, U., Usman, B., and Ibrahim, M. B. (2021). Cationic dyes removal from wastewater by adsorptive method: a systematic in-depth review. Algerian J. Chem. Eng. AJCE 1 (2), 6–40.
Yusuf, I., Flagiello, F., Ward, N. I., Arellano-García, H., Avignone-Rossa, C., and Felipe-Sotelo, M. (2020). Valorisation of banana peels by hydrothermal carbonisation: potential use of the hydrochar and liquid by-product for water purification and energy conversion. Bioresour. Technol. Rep. 12, 100582. doi:10.1016/j.biteb.2020.100582
Keywords: watermelon waste, adsorption, synthesis, performance, mechanisms
Citation: Onyango C, Nyairo W, Tchieta GP and Shikuku VO (2025) Watermelon rind based adsorbents for the removal of water pollutants: a critical review. Front. Environ. Chem. 6:1568695. doi: 10.3389/fenvc.2025.1568695
Received: 30 January 2025; Accepted: 25 April 2025;
Published: 13 May 2025.
Edited by:
Dengjun Wang, University of Florida, United StatesReviewed by:
Jian Lu, Tongji University, ChinaXueyan Lyu, Nanjing University of Information Science and Technology, China
Copyright © 2025 Onyango, Nyairo, Tchieta and Shikuku. This is an open-access article distributed under the terms of the Creative Commons Attribution License (CC BY). The use, distribution or reproduction in other forums is permitted, provided the original author(s) and the copyright owner(s) are credited and that the original publication in this journal is cited, in accordance with accepted academic practice. No use, distribution or reproduction is permitted which does not comply with these terms.
*Correspondence: Victor O. Shikuku, dnNoaWt1a3VAa2FmdS5hYy5rZQ==