- 1Laboratory of Entomology and Phytopathology, International Center for Agricultural Research in the Dry Areas (ICARDA), Rabat, Morocco
- 2AgroBioSciences Program, College of Agriculture and Environmental Sciences, Mohammed VI Polytechnic University, Ben Guerir, Morocco
- 3Plant and Microbial Biotechnology Center, Moroccan Foundation for Advanced Science, Innovation and Research (MAScIR), Mohammed VI Polytechnic University, Ben Guerir, Morocco
Pulse crops, including beans, peas, chickpeas, and lentils, are vital sources of protein, fiber, and essential nutrients worldwide. They serve not only as staple foods but also as key components of sustainable agricultural practices, contributing to soil fertility through nitrogen fixation and enhancing overall productivity. However, pulse crops face numerous abiotic and biotic stresses mainly insect pest attack and pathogen invasion, which pose significant threats to pulse crops, impacting both production and food security. To overcome these challenges, plants have evolved diverse defense mechanisms, including the emission of specific volatile organic compounds (VOCs). These volatiles play crucial roles in plant communication, protection, and real-time health status indication. Monitoring VOCs offers a promising approach for early detection of pest infestations or pathogen infections, enabling the grower to take early action and decide on the proper control measure to minimize losses. The identification of plant-emitted VOCs requires robust and sensitive analytical techniques such as gas chromatography and mass spectrometry, which are the mainly used techniques for in pulse crops studies. However, traditional methods have limitations, prompting the need for advanced, portable, and real-time detection alternatives, such as gas-sensing technologies. This paper provides a comprehensive review of VOC measuring methods, including extraction, separation, and analytical techniques, focusing on their application in pulse crops. Recent advancements in gas-sensing technologies are also discussed, highlighting their potential in enhancing crop protection and agricultural sustainability.
1 Introduction
Pulse crops including beans, peas, chickpeas, and lentils play a crucial role in global agriculture and nutrition, serving as a vital source of protein, fiber, and essential nutrients for millions worldwide (Semba et al., 2021). Pulse crops hold significant importance, not only as a staple food but also as a key component of sustainable agricultural practices. These crops contribute to soil fertility through nitrogen fixation, aiding in the cultivation of other crops and enhancing overall agricultural productivity (Yigezu et al., 2019). However, in many regions of the world, pulse crops encounter a range of abiotic and biotic stresses, posing substantial challenges to their productivity and quality. In Morocco, pulse crops show high yield instability due to several factors such as water scarcity, soil degradation and particularly susceptibility to pathogen and insect pest attack, which pose significant threats to production (Sabraoui et al., 2019; Ait Taadaouit et al., 2021, Ait Taadaouit et al., 2022).
The most prominent diseases affecting pulse crops in Morocco are Ascochyta blight, Fusarium wilt, and rust disease (Merkuz et al., 2011; Hossain et al., 2013; Houasli et al., 2020). Additionally, a variety of insect pests including leaf miners, pod borer, aphids, and beetles pose serious threats by causing extensive damage to both plants and stored grains, rendering them unsuitable for consumption or sale (Kumar et al., 2019). The surveillance and effective management of these biotic stresses, which detrimentally impact crop production and ecosystems worldwide, are crucial for enhancing crop yields and ensuring food security for the growing global population. Specifically, early detection of insect or pathogen presence before visible symptoms appear, is invaluable for implementing appropriate management strategies to control their spread and minimize losses (Das et al., 2008).
Plants deploy a broad range of defense mechanisms for combatting pathogen invasions and attacks by herbivorous insects. One such strategy involves the emission of specific volatile organic compounds (VOCs) (Maffei et al., 2007; Dicke and Loreto, 2010; Mutyambai et al., 2016; Castro et al., 2017), comprising various chemical groups including monoterpenes, sesquiterpenes, alcohols, aldehydes, aromatic compounds, esters, furans, hydrocarbons, and ketones (Pichersky and Gershenzon, 2002; Kramer and Abraham, 2012; Schenkel et al., 2015; Delory et al., 2016; Kihika et al., 2017; Kindlovits et al., 2018; Murungi et al., 2018; Xie et al., 2022).
The emission of VOCs in pulse crops occurs naturally within leaves, flowers, seeds, and roots (Mumm and Hilker, 2006; Loreto and Schnitzler, 2010; Fineschi and Loreto, 2012), but it intensified under various stressors like high temperature (Centritto et al., 2011; Fares et al., 2011), water scarcity (Holopainen and Gershenzon, 2010; Brilli et al., 2013) or attacks by herbivores or pathogens, which serves as a defense mechanism for these plants (López et al., 2011). VOCs of pulse crops are mainly produced from the oxidation of unsaturated free fatty acids and the degradation of amino acids during seed development, harvesting, and storage (Vincenti et al., 2019). A diverse range of chemical classes, such as aromatic hydrocarbons, aldehydes, alkanes, alkenes, alcohols, ketones, acids, esters, pyrazines, terpenes, furans, and lactones, have been identified in pulse crops (Roland et al., 2017).
Among these, aldehydes are the predominant chemical class found in pulse crops, except for certain crops like common beans (black beans, pinto beans, and dark red kidney beans), which exhibit a notable presence of styrene, an aromatic hydrocarbon. Also, Ketones represent an intermediate class of volatile compounds. Although aromatic hydrocarbons are generally present in low proportions across all pulse crops except for styrene. Similarly, esters are typically a minor component of pulse volatiles, except in stored faba beans. Additionally, alcohols emerge as the most abundant class, reflecting significant diversity. Terpenes present in pulse crops are composed of monoterpenes (C10) and sesquiterpenes (C15), with most originating from the degradation of carotenoids. Currently, the degradation of free fatty acids is the only identified source for furan synthesis, with no furans detected in chickpeas (Oomah and Liang, 2007; Burdock, 2010; Azarnia et al., 2011; Karolkowski et al., 2021).
These secondary metabolites typically possess small molecular masses (on average below 300 Da), low boiling points, and high vapor pressure (vaporizing at 0.01 kPa at a temperature of approximately 20°C) (Morath et al., 2012) allowing them to diffuse through the atmosphere, soils, and liquids, and acting over short and long distances (Maffei et al., 2011; Effmert et al., 2012; Schmidt et al., 2015; Tyc et al., 2017). These unique proprieties enabled them to ensure interactions between the organism and its environment, including plant–plant and plant–insect or pathogen interactions (Ruther and Kleier, 2005; Schulz and Dickschat, 2007; Choudhary et al., 2008; Das et al., 2012; Allmann et al., 2013; Junker and Tholl, 2013; Piechulla et al., 2017). By emitting specific blends of VOCs, plants can deter herbivores, attract beneficial organisms for pest control (Kessler and Baldwin, 2001; Ichiki et al., 2008; Dicke, 2009; Ode, 2013; Santos and Cabot, 2015; Singh et al., 2021), inhibit pathogen growth, and communicate with neighboring plants to collectively activate defense responses (Baldwin et al., 2002; Effah et al., 2019; Cellini et al., 2021). The significance of VOCs in plant communication underscores their potential for enhancing crop protection. Moreover, VOCs emitted by plants serve as indicators of their real-time physiological health status, providing bio-information that could facilitate rapid non-invasive disease diagnosis (Ficke et al., 2021). Furthermore, the composition of VOCs varies according to the type of damage, such as pathogen infection and herbivore feeding, with some VOCs existing as potent aromatic gases, albeit most occurring at extremely low concentrations (Cui et al., 2018).
Apart from their capacity to impact pathogen development, insect pest feeding habits, and host selection (Webster et al., 2008; Bruno et al., 2018), VOCs emitted by legume plants infected with viruses can also manipulate the virus vectors’ behavior to enhance their transmission and spread. But this contract could be managed by exploiting the attractive compounds for insect trapping (Reisenman et al., 2016; Wyatt, 2018).
Consequently, monitoring these VOCs could serve as an effective indicator of insect pest infestations or pathogen infections (Piesik et al., 2011). The detection and analysis of VOCs have emerged as crucial components of modern agricultural research and plant protection strategies. Today, scientists have developed various analytical techniques for VOC analysis, usually relying on gas chromatography and soft chemical ionization mass spectrometry. These technological advancements address key challenges associated with accurately sampling VOCs, overcoming the inherent reactivity of certain volatile compounds that makes them difficult to detect directly, as well as coping with their high sensitivity and low concentrations (Dudareva et al., 2006; Qualley and Dudareva, 2009; Materic et al., 2015; Tholl et al., 2021).
While traditional methods of VOC detection offer various advantages and a wide range of applications, they often come with drawbacks such as high cost, bulky equipment, and the need for specialized expertise and training. Therefore, inexpensive, portable, and user-friendly alternatives are required. One such approach involves the use of different gas-sensing technologies to detect VOCs released by plants under stress (Tisch and Haick, 2010; Liu et al., 2012; Fang and Ramasamy, 2015). Although these methods often meet requirements, they also possess their own set of advantages and disadvantages. Further research is necessary to overcome these limitations, particularly concerning the dynamic nature of VOCs, environmental factors, and to better understand the feasibility of deploying these methods under field conditions (Cui et al., 2018).
This paper provides a comprehensive review of the essential steps involved in VOC profiling and quantification including extraction, separation and analytical techniques. It provides a description and comparison of the most prevalent techniques currently employed for measuring volatiles emitted from plants and microorganisms, with a specific focus on their application in pulse crops. Furthermore, recent advancements in gas-sensing technologies are discussed to highlight their relevance and potential contributions to the field.
2 Methods of volatile organic compound extraction
A fundamental and primary step in plant volatile analysis is the headspace collection, which is a simple, non-invasive, transportable, and inexpensive method. The term ‘headspace’ refers to the gaseous phase surrounding the plant, within which VOCs are dispersed. This sampling process is typically coupled with the preconcentration of VOCs found in the air, often present at trace levels, to meet the detection limits of commonly utilized analytical instruments (Jansen et al., 2011).
Plant volatile sampling methods are classified into two main categories: static headspace sampling and dynamic headspace sampling (Table 1).
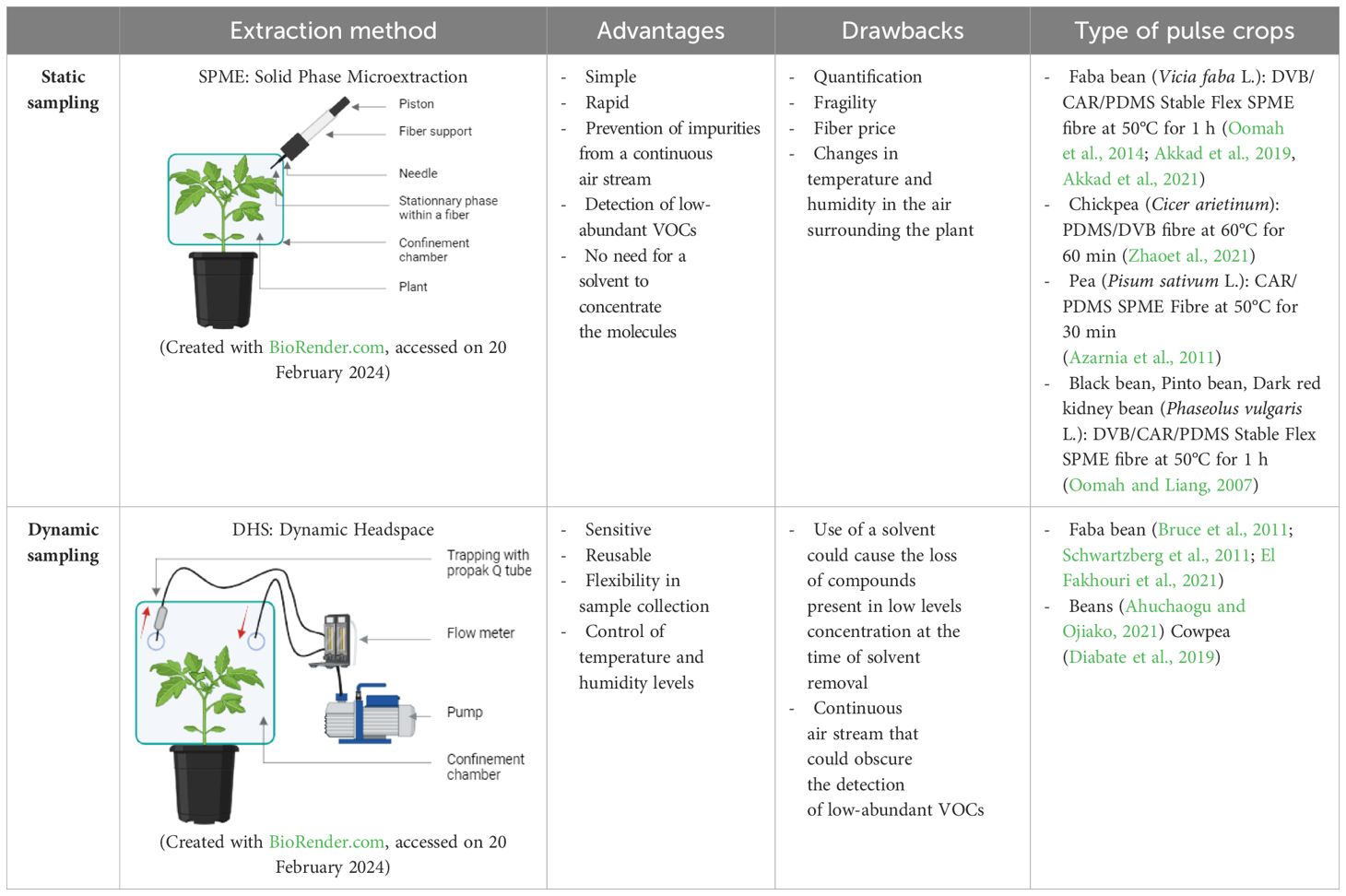
Table 1 Summary of advantages and disadvantages of different sampling techniques and the type of pulse crops and adsorbent phases used in different studies.
The choice of sampling method depends on factors such as the research objectives, environmental conditions, available resources, and desired level of temporal resolution in VOC data collection (Stierlin, 2020; MacDougall et al., 2022).
2.1 Static method
During passive sampling, VOCs emitted by the plant diffuse freely from the sampled environment to a collection medium. These molecules are captured on an adsorbent support positioned in close proximity to the plant, with the trapping of VOCs mainly relying on mass diffusion processes (Stierlin, 2020).
Passive or static sampling techniques involve the adsorption and subsequent thermal desorption of compounds from an inert fiber coated with various adsorbents of differing polarity and thickness, tailored to the type and concentration of the targeted compounds (Tholl et al., 2021).
These adsorbent phases are composed of diverse polymers like polydimethylsiloxane (PDMS), polyacrylate (PA), or polyethylene glycol (referred to as CW or carbowax), as well as porous polymers such as divinylbenzene (DVB) or carboxen (CAR) (Jansen et al., 2011). Among them polydimethylsiloxane (PDMS), divinylbenzene (DVB) and carboxen (CAR) are more used for pulse crops’ volatile extraction (Murat et al., 2012) (Table 1).
A significant advancement in static headspace sampling is the development of solid phase microextraction (SPME), a technique that enables rapid and straightforward collection of volatiles with detection limits reaching the parts per billion by volume (ppbv) range. SPME stands out for its mobility and the consolidation of collection, concentration, and introduction of VOCs into a single stage, which significantly reducing preparation time and enhancing sensitivity compared to other extraction methods (Vas and Vékey, 2004; Papet et al., 2010; Zhang and Li, 2010; Vangoethem, 2017; Rering et al., 2020) (Figure 1).
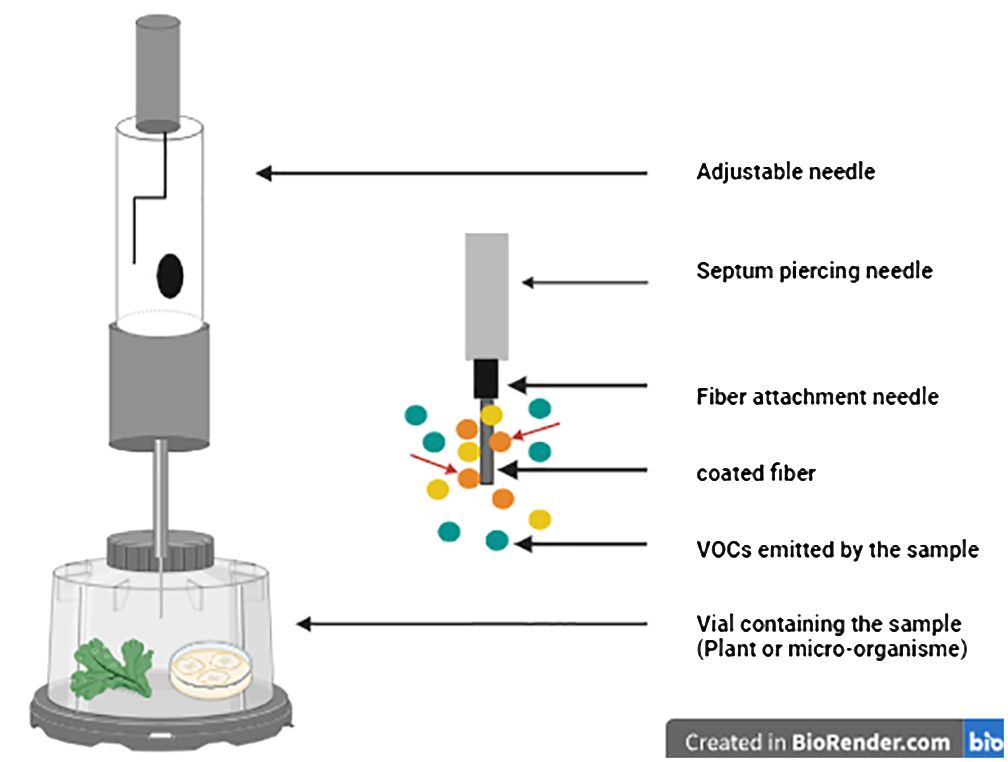
Figure 1 Setup of solid phase microextraction (SPME) device used for volatile compound extraction (Created with BioRender.com, accessed on 20 February 2024).
Due to its advantages, this technique is utilized in numerous research studies focusing on VOCs in pulse crops (Table 2).
2.2 Dynamic method
During dynamic sampling, VOCs are entrained by an actively pumped air stream, guiding the target compounds towards the trap via a packed cartridge. It enables the capture of a greater quantity of VOCs compared to static techniques (Stierlin, 2020).
In these methods, a continuous flow of a carrier gas is directed through the headspace container, allowing control over temperature and humidity levels (MacDougall et al., 2022).
Dynamic headspace sampling techniques are widely utilized in the analysis of pulse crops volatile compounds due to their efficiency and versatility. During this process, volatiles are trapped by adsorption onto a polymer within closed chambers featuring continuous air circulation. Then the trapped volatiles can be eluted from the adsorbent matrix using solvents or thermal desorption techniques for subsequent GC analysis (Tholl et al., 2006) (Figure 2).
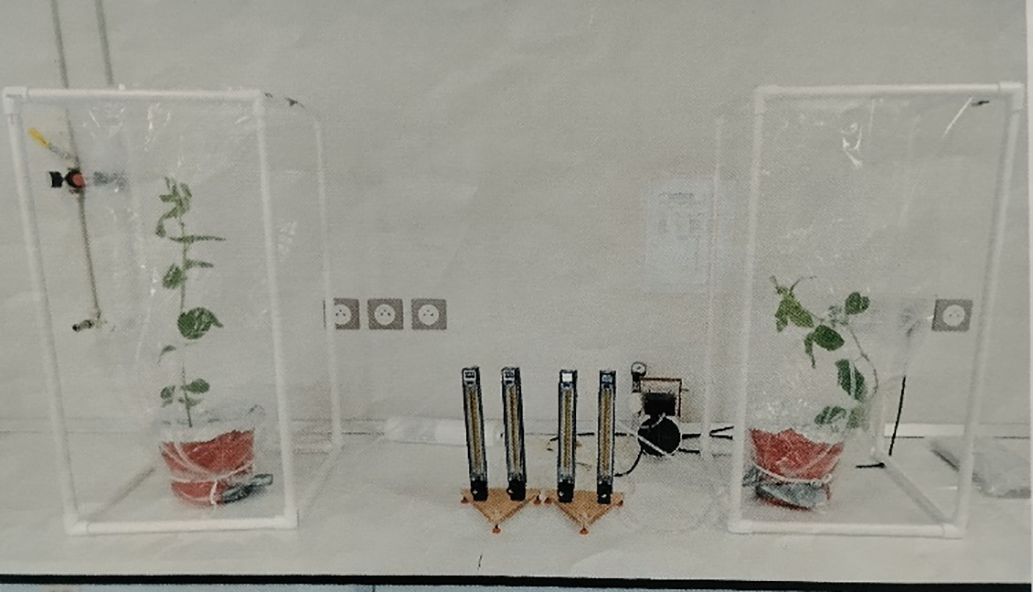
Figure 2 Dynamic headspace sampling process as described by El Fakhouri et al. (2021).
This sampling method is commonly employed for extracting VOCs from different pulse crops (Table 3).
3 Technique for VOC separation: gas chromatography
The characterization and quantification of individual substances within the VOC blend often necessitate separation prior to analysis. Gas chromatography (GC) stands out as the preferred method in most applications involves pulse crops (Jansen et al., 2011).
It is a prevalent laboratory analytical technique that has an excellent separation performance, and high sensitivity and selectivity. GC proves to be a well-established method suitable for both qualitative and quantitative analysis of plant foliar VOCs. In this technique, a carrier gas, typically an inert gas like helium that used in all pulse crop VOC analysis, serves as the mobile phase, while a layer of a polymer on an inert solid support presents the stationary phase, within a glass or metal column. The selection of column properties is critical as they significantly influence the separation of plant-emitted volatiles (Liu et al., 2012).
The central component of a gas chromatograph is its column, situated in the oven of the instrument. Generally, there are two types of columns: packed and capillary. Although packed columns still find utility in certain applications, capillary columns are more prevalent in plant VOC research. The crucial consideration in column selection lies in its stationary phase. Nowadays, capillary columns are coated with various stationary phases, ranging from nonpolar to polar, depending on the functionality of the target compounds. Typically, polar columns are requisite for effective separation of polar VOCs, while nonpolar columns are suited for nonpolar VOCs. As a general rule for analyzing complex mixtures of foliar VOCs, initiating with a 5% phenyl-substituted (polar) column is recommended.
The standard length of a column employed in plant foliar VOC research ranges from 15 to 60 meters, depending on the compounds of interest. The gas chromatograph instrument consists of temperature-controlled oven, capable of rapid and reproducible temperature ramping from ambient to over 300°C. Additionally, the instrument houses a series of pressure control systems and facilitates the introduction of samples and interface with analytical detectors. Inside the oven is an open tubular column (30–60 m) containing a stationary-phase film that separates compounds based on their physicochemical properties. One end of the gas chromatograph column is connected to the inlet (usually an injector), and the other end (outlet) is linked to the detector.
Samples are introduced either through a heated injector or by direct desorption from the adsorbent, and then transported by the flow of carrier gas, usually helium, through the column. Each VOC interacts differently with the stationary phase of the column, resulting in differentially partitioned between the stationary phase and the mobile phase (helium) (Figure 3) (Tholl et al., 2006).
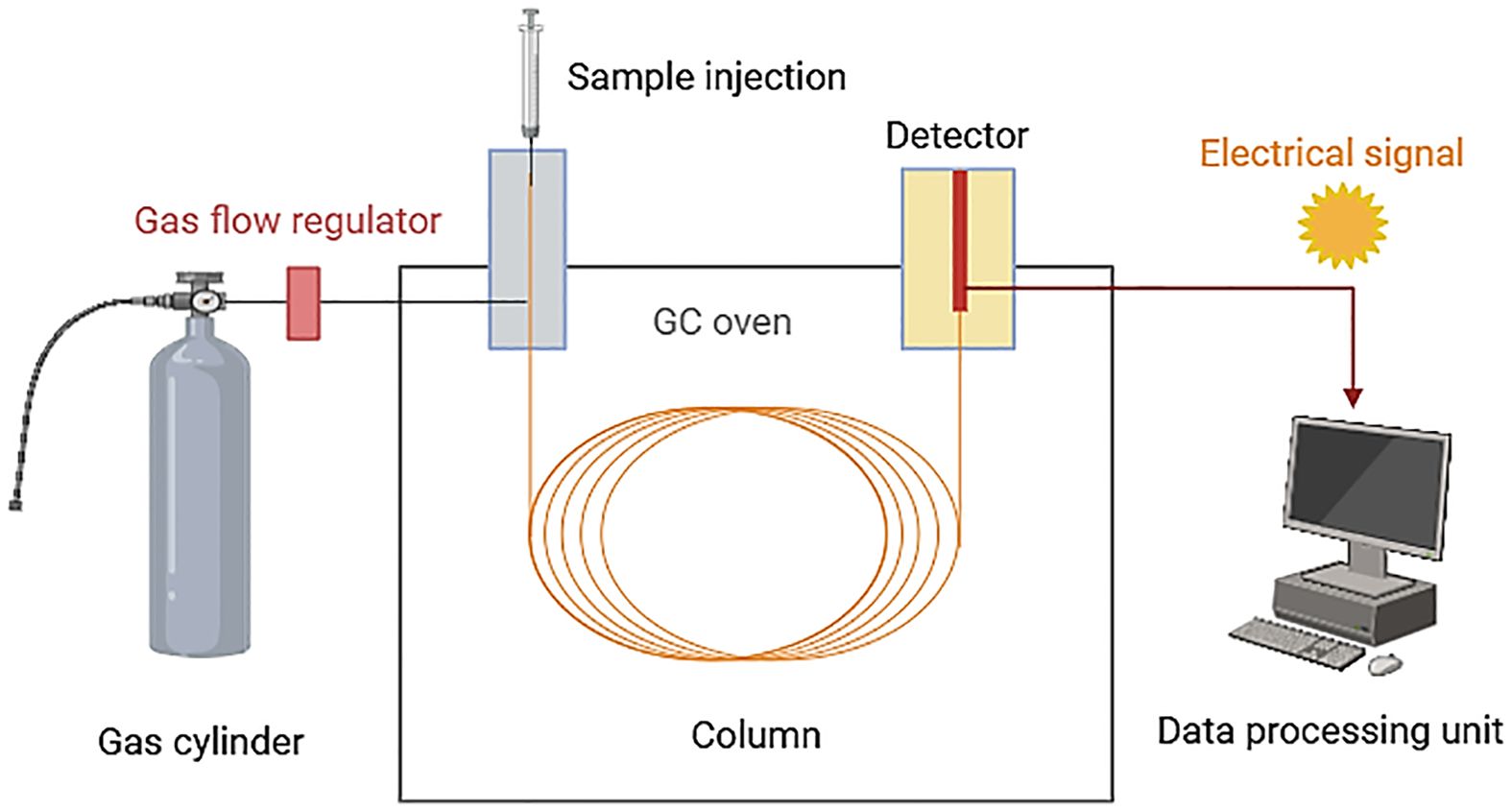
Figure 3 Gas chromatography (GC) technique overview (Created with BioRender.com, accessed on 03 June 2024).
An increase in temperature affects the partition coefficient, eventually causing the compound to fully transition into the mobile phase and be carried into the detector via a heated transfer line. Consequently, different VOCs elute from the column at different times (retention time). After exiting the column, VOCs can be identified and quantified using a mass spectrometer or another detector (Materic et al., 2015).
GC stands out as the primary method employed for analyzing VOCs in various pulse crops. For example, volatiles emitted by broad bean, V. faba were analyzed quantitatively using a Hewlett-Packard 6890 GC equipped with a flame ionization detector (FID). The GC utilized an HP-1 bonded phase capillary column (30 m × 0.25 mm × 0.25 μm, J&W Scientific, Folsom, CA, USA), with Helium employed as the carrier gas at a linear flow rate of 36.3 cm/sec (Schwartzberg et al., 2011).
Additionally, according to Mhlanga et al. (2021), VOC emissions in two bean (Phaseolus vulgaris L.) cultivars were separated on a capillary GC column (ZB-5MSi, Thermo Scientific, United Kingdom). The injection volume (splitless) was 1 µl, with the injector temperature set at 200°C. Helium served as the carrier gas at a constant flow rate of 2.6 ml min−1 in an oven maintained at 30°C for 5 min and then programmed to increase at a rate of 15°C per minute until reaching 230°C.
Furthermore, Ajayi et al. (2015) employed gas chromatography (GC) to analyze volatile compounds produced by seeds of three legume cultivars: Ife-brown and black-eyed cowpeas (Vigna unguiculata L.) and soybean (Glycine max L.).
A quantity of 1 µL of each headspace volatile extract was injected into a Shimadzu GC17A equipped with a flame ionization detector (FID), and a capillary column HP-5MS5MS (0.25 mm i.d., 0.25 µm film thickness, Agilent Technologies Inc., Santa Clara, CA, USA). Helium served as the carrier gas at a flow rate of 1 mL/min. The GC oven was programmed as follows: an initial injection at 40°C held for 2 minutes, followed by an increase at a rate of 5°C/min until reaching 200°C. Both the injector and detector temperatures were set at 200°C.
Moreover, Bruce et al. (2011) conducted an analysis of VOCs emitted by V. faba cv. ‘Sutton dwarf’ flowers using a HP 6890 GC (Agilent, UK). The instrumentation included a cold on-column injector, an FID, and two types of capillary columns: a non-polar HP-1 bonded-phase fused-silica column (50 m × 0.32 mm i.d., film thickness 0.52 µm) and a polar DB-WAX column (30 m × 0.32 mm i.d., film thickness 0.82 µm). A 2 µL aliquot of the headspace sample was injected onto the capillary GC column. The oven temperature was initially held at 30°C for 1 minute, then programmed to increase at a rate of 5°C/min to 150°C, where it was maintained for 0.1 minutes, followed by a further increase at a rate of 10°C/min to 230°C. Hydrogen was used as the carrier gas.
4 Analytical techniques for volatile organic compounds
Gas chromatography (GC), when coupled with a detector, becomes indispensable for analyzing biological VOCs (Zhang and Li, 2010). Various types of detectors are utilized for identifying and/or quantifying individual VOCs within the sample. The mass spectrometer (MS) is the most employed detector for the identification of plant VOCs. More recently, electronic noses (E-noses) have emerged as promising tools for detecting plant-emitted VOCs in the air (Zhang and Li, 2010; Jansen et al., 2011; Materic et al., 2015). A crucial specification of any detector is its limit of detection (LOD), defined as the lowest quantity of a substance distinguishable from its absence within a stated confidence limit. The limit of quantification (LOQ) should also be considered when both detection and quantification of concentration are required. LODs and LOQs may be expressed in either absolute amounts (nanograms or picograms) or in relation to concentrations in the air.
The choice of the appropriate analytical technique in plant VOC research depends mainly on three factors: the research question, instrument availability, and budget (Materic et al., 2015). Additionally, other parameters are essential to consider such as accuracy, precision, limit of detection (LOD), selectivity, robustness, cost, and response or analysis time (MacDougall et al., 2022).
These considerations ensure the selection of the most suitable analytical approach for the research objectives and constraints (Table 4).
4.1 Offline techniques
Currently, gas chromatography-mass spectrometry (GC-MS) is one of the most widely employed techniques for VOC detection due to its powerful separation abilities and robust identification capabilities of MS. The highly sensitive detection of GC-MS makes it a valuable tool for both qualitative and quantitative analysis of VOCs emitted by plants and microorganisms under different ecological and biological conditions (Jansen et al., 2011).
VOC identification is facilitated by using mass spectra libraries such as Wiley and NIST MS databases, or by comparing retention times and spectra with those of known standards (Morath et al., 2012; Vangoethem, 2017). However, Gas Chromatography columns exhibit selectivity towards different chemical groups of VOCs and are unable to identify novel compounds, thus limiting their capability for total VOC estimation (Morath et al., 2012). Additionally, this technique can be very time-consuming and requires the use of heavy, bulky laboratory equipment, rendering it not suitable for field applications (Figure 4) (Tholl et al., 2006). Despite these limitations, the GC-MS technique has been widely employed in various research studies to analyze the VOC profiles of different pulse crops (Table 5).
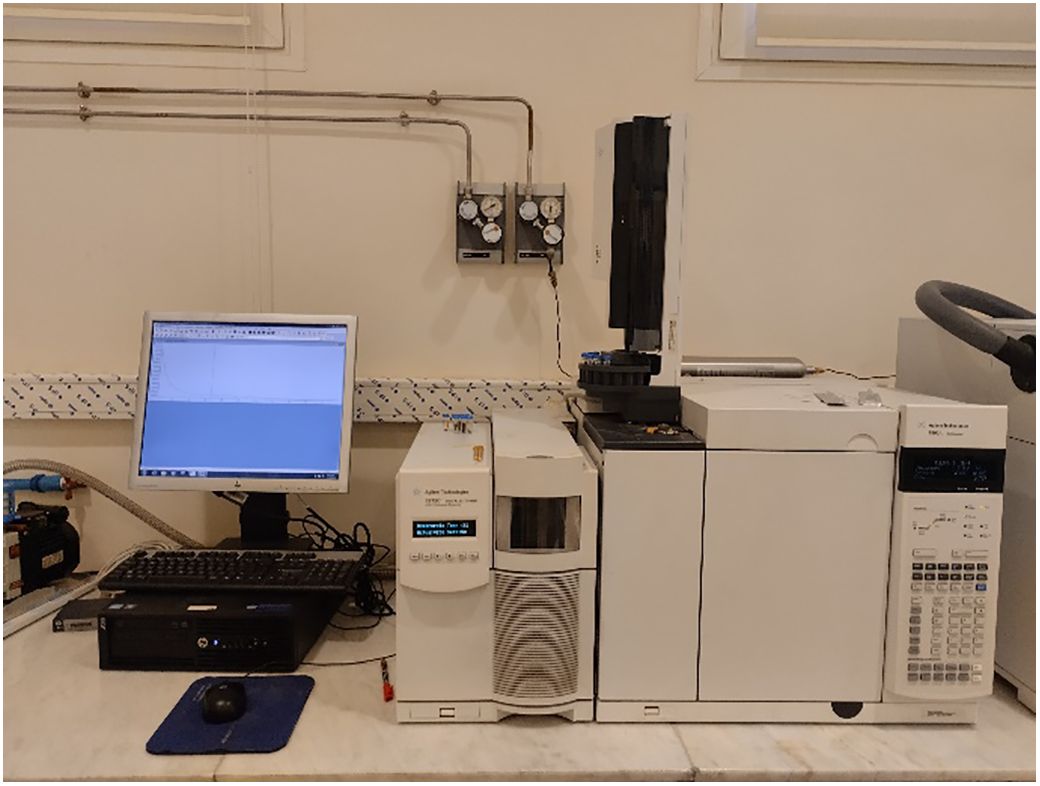
Figure 4 Gas chromatography coupled to mass spectrometry (GC-MS) instrumentation in laboratory setting.
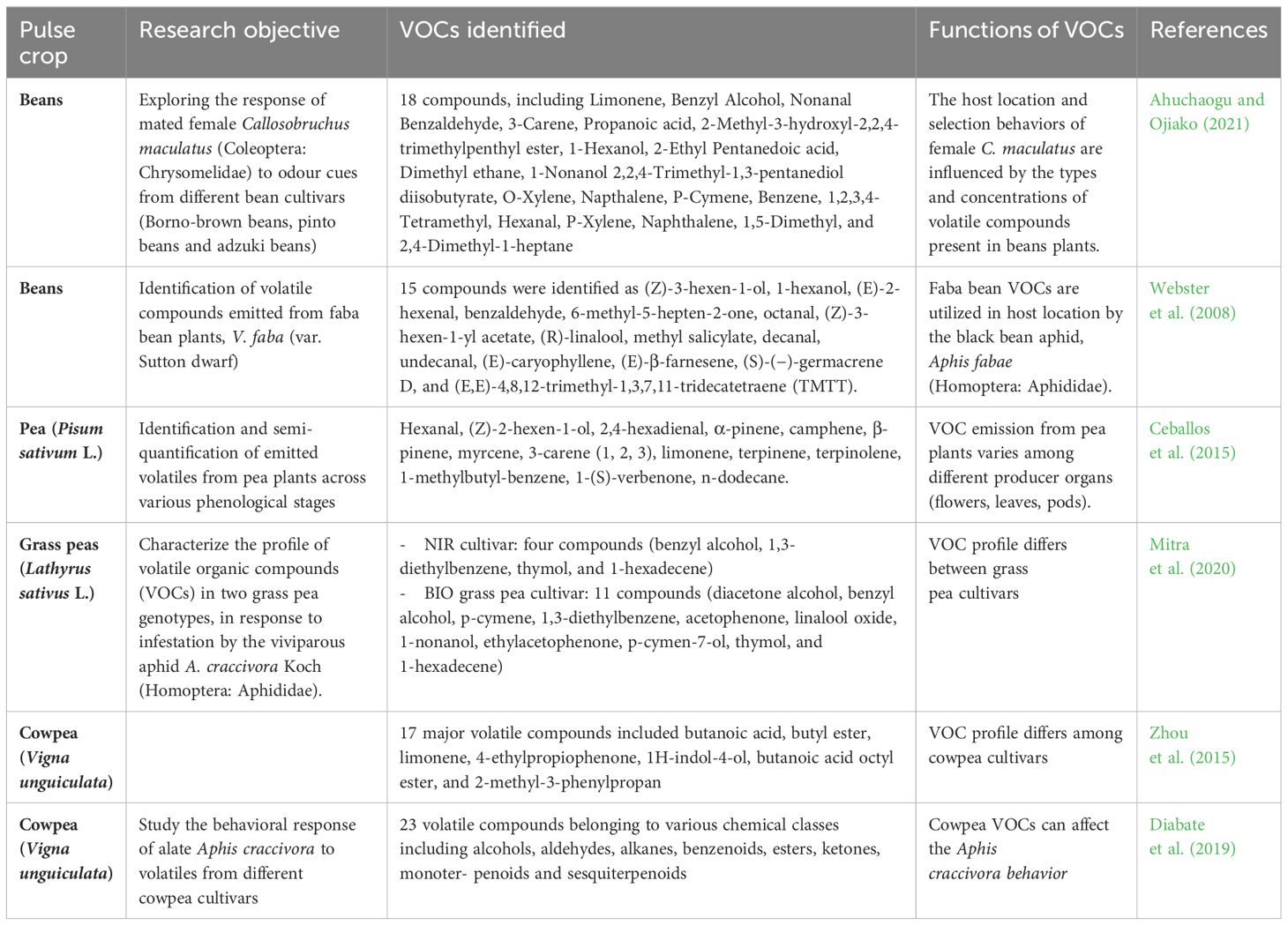
Table 5 Volatile organic compounds detected through GC-MS analysis in various research investigations of pulse crops.
In addition to conventional MS detectors, new MS technologies have been developed for analyzing biological VOCs, such as proton-transfer reaction MS (PTR-MS). These innovations play a crucial role in identifying unknown VOCs, contributing to a deeper understanding of biological processes (Zhang and Li, 2010).
4.2 Online techniques
Proton-transfer reaction mass spectrometry (PTR-MS) emerges as a non-invasive and highly sensitive method enabling the online detection of plant VOCs in real-time with high throughput (Blake et al., 2009). This powerful technique offers many advantages, including reduced sample preparation, very low detection limits, high selectivity and sensitivity, real-time VOC monitoring, and the ability to detect VOCs at low concentrations (parts per trillion volume – pptv) in air and gas samples (Tholl et al., 2006).
By integrating a compact high-resolution time-of-flight detector with the ion source, PTR-TOF-MS enables rapid and complete detection of VOCs with a time resolution of less than one second. This technology (PTR-TOF-MS) has proven successful in characterizing the VOC profiles of various biological entities, including plants, soils, bacteria, and fungi such as Fusarium spp., Muscodor albus, Tuber magnatum, and various Mortierella species.
Despite its strengths, PTR-Qi-TOF-MS has some limitations, notably in the identification of fragments from other molecules or compounds associated with parent molecules. Additionally, it lacks the capability to provide isomer-specific information in VOCs, such as the structural identity of isomers (Figure 5) (Gualtieri et al., 2022).
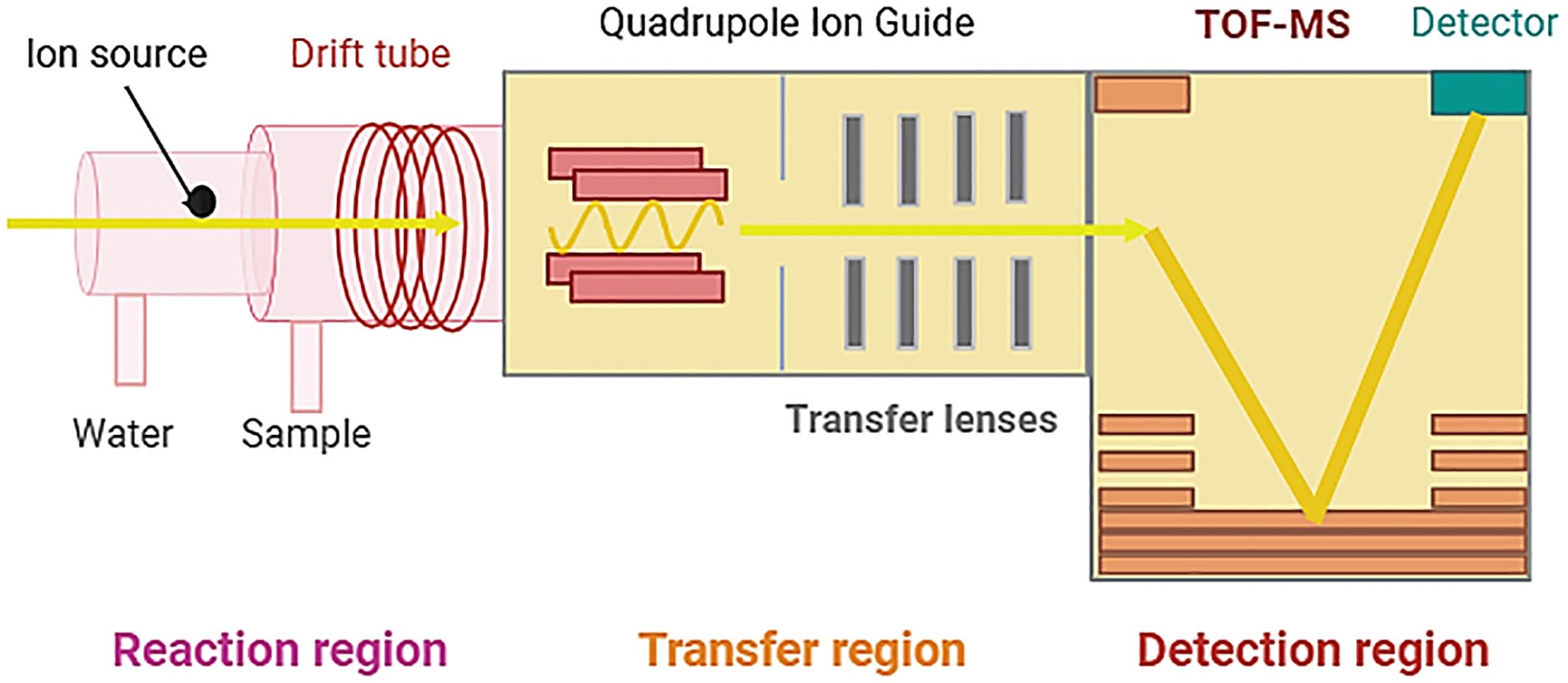
Figure 5 Proton-transfer reaction time-of-flight mass spectrometry (PTR-TOF-MS) (Created with BioRender.com, accessed on 03 June 2024) (Majchrzak et al., 2019).
Moreover, PTR-MS technique is the inability to differentiate molecules with the same molecular weight, as compounds are identified based on their molecular masses. Furthermore, without enhancement or gas chromatography (GC) separation, PTR-MS may not achieve the same sensitivity as offline GC methods. Consequently, GC techniques remain the preferred choice for speciated analysis (Niederbacher et al., 2015).
The PTR-MS technique was utilized to determine VOCs emitted from poplars, apple, tomato and truffles (Eller et al., 2012; Farneti et al., 2012, 2014; Splivallo et al., 2012), however, there are no data available about its use in pulse crops.
5 Emerging VOC sensing methods
The electronic nose (E-nose), also known as artificial olfaction devices, is a novel analytical approach for investigating biological VOCs. It presents a simple and adaptable tool for the non-destructive, rapid monitoring and detection of various gas samples (Cellini et al., 2017).
An E-nose consists of an array of different gas-sensitive chemical sensors that interact with VOCs and generate electronic signals proportional to the concentration and type of VOCs detected. These signals are then processed using pattern recognition algorithms to identify and quantify the specific VOCs present (Cui et al., 2018). Measurement of sensor array response provides individual responses per sensor, each possessing distinct features (MacDougall et al., 2022).
However, E-noses exhibit several limitations, including low detection sensitivity, limited chemical specificity, and signal drifts resulting from environmental factors such as humidity and temperature fluctuations. Moreover, E-noses are capable of capturing the overall profile of biological VOCs, but they are unable to detect individual VOC components due to constraints inherent in membrane materials, manufacturing techniques, and data processing methods (Tholl et al., 2021).
Several commercial gas sensors are available for E-nose systems. These sensors can be categorized based on their operational principles into three distinct groups: conductivity sensors, gravimetric sensors, and optical sensors (Table 6).
5.1 Conductivity sensors
These sensors utilize conducting polymer (CP) and/or metal oxide semiconductor (MOS) as their sensing materials, both of which operate on the principle of variations in conductivity or resistance upon exposure to particular gases.
5.2 Gravimetric sensors
E-nose systems use two types of gravimetric sensors: surface acoustic wave (SAW) sensors and quartz crystal microbalance (QCM) sensors. SAW sensors generate a surface wave that propagates along the sensor’s surface, whereas QCM sensors produce a wave that travels through the sensor’s bulk. Both sensors operate on the principle of mass change in the piezoelectric sensor coating caused by gas absorption, leading to alterations in the resonant frequency when exposed to VOCs.
5.3 Optical sensors
The principal of optical sensors is based on changes in chemical properties, including the reactivity, redox potential, and acid-base interactions. These sensors incorporate a wavelength-selectable light source, a light detector, and sensor materials that interact with gases. Colorimetry and fluorometry represent the common techniques utilized to analyze the signal obtained from optical sensors.
To evaluate the performance of gas sensing methods or sensors, several key indicators should be considered such as sensitivity (the minimum concentration of target gases required for detection), selectivity (the ability of sensors to distinguish a specific gas from a mixture), response time (the duration between gas concentration reaching a threshold and the sensor generating a signal), energy consumption, reversibility (whether sensing materials can revert to their original state after detection), adsorptive capacity (which also influences sensitivity and selectivity), and fabrication cost (Liu et al., 2012).
Cai et al. (2021) examined the impact of roasting levels on the physicochemical, sensory, and volatile profiles of soybeans utilizing E-nose and HS-SPME-GC‐MS techniques.
They employed a commercial PEN3 E-nose equipped with 10 semiconductor metal oxide chemical sensing elements, each designed to detect specific volatile substances, to analyze the headspace gas of roasted and unroasted soybean flours. The analysis identified 41 volatile compounds, with 2,5-dimethylpyrazine being the most prevalent.
Additionally, HS-SPME-GC‐MS was utilized to determine volatile compounds emitted from soybean flours, using a DVB/CAR/PDMS fiber needle for VOC extraction followed by GC-MS analysis. This traditional analysis method provides high sensitivity and excellent selectivity, enabling the identification and quantification of individual VOCs in complex mixtures with high precision. However, it requires longer analysis times due to sample preparation, separation, and data processing steps. In contrast, E-nose analysis is generally rapid, allowing for high throughput screening of samples within a short period. Besides, it can detect complex mixtures of VOCs. However, they may lack selectivity, as individual sensors respond to multiple compounds, and identification is based on pattern recognition. Moreover E-nose devices are less expensive and simpler to operate compared to traditional GC-MS systems (Cai et al., 2021).
6 Conclusion and future perspectives
In conclusion, efficient monitoring of VOCs enables early detection of pest infestations or pathogen infections, facilitating timely intervention and proper control measures to minimize losses in pulse crops. Conventional methods of sampling, separation and analysis such as HS-GC-MS present a powerful tool for both qualitative and quantitative analysis of VOCs emitted by pulse crops plants such as beans, chickpeas, and peas. Despite their effectiveness, traditional methods have limitations, underscoring the need for more advanced, portable, and real-time detection alternatives.
Extensive research has shown promising results for using electronic noses (e-noses) as non-destructive tools for rapid and early detection of plant pest/pathogen damage, particularly in laboratory environments. Its usage in pulse crops is currently limited, and there are still a number of challenges that need to be improved for field applications. The dynamic nature of VOCs presents a challenge, as emissions vary based on factors such as tissue type, location, and physiological stage, as well as seasonal variations and environmental factors like temperature and humidity. This complexity impact sensor performance and increases the difficulty of characterizing VOC biomarkers for pest or pathogen detection, driving the need for further research and development.
Field conditions further complicate detection efforts due to uncontrollable environmental parameters and low concentrations of VOCs released by plants. Combining E-nose systems with other advanced technologies such as mass spectrometry or gas chromatography could address these challenges and extend their application range. The development of micro-level E-noses offers promise for portable and cost-effective detection solutions, potentially integrated with smartphones for user-friendly applications. Despite these advancements, challenges remain in sensor selectivity, atmospheric interference, and field detection feasibility, necessitating continued research and improvement.
Author contributions
LM: Writing – review & editing, Writing – original draft, Methodology, Formal analysis. KE-F: Writing – review & editing, Methodology, Formal analysis. S-AK: Writing – review & editing, Conceptualization. AA: Writing – review & editing, Visualization. IM: Writing – review & editing, Visualization. ME-B: Writing – review & editing, Conceptualization.
Funding
The author(s) declare financial support was received for the research, authorship, and/or publication of this article. This review is part of the ‘SpectraVOCS’ project, which is funded by the APRD Program and sponsored by the OCP Foundation, Mohammed VI Polytechnic University, the National Center of Scientific and Technical Research (CNRST), and the Ministry of Higher Education, Scientific Research, and Innovation of Morocco.
Acknowledgments
The authors thank OCP Foundation, UM6P, CNRST and DESRS for their financial support. The authors extend their sincere thanks to all staff, students, and trainees from UM6P and ICARDA and for their assistance in preparing this manuscript.
Conflict of interest
The authors declare that the research was conducted in the absence of any commercial or financial relationships that could be construed as a potential conflict of interest.
Publisher’s note
All claims expressed in this article are solely those of the authors and do not necessarily represent those of their affiliated organizations, or those of the publisher, the editors and the reviewers. Any product that may be evaluated in this article, or claim that may be made by its manufacturer, is not guaranteed or endorsed by the publisher.
References
Ahuchaogu E., Ojiako F. O. (2021). Host seed type and volatile compound abundance level mould host location and preference behaviours in Callosobruchus maculatus (Fabricius 1775) (Coleoptera: Chrysomelidae) Christopher. Polish J. Entomology. 90, 152–163. doi: 10.5604/00323780
Ait Taadaouit N., El Fakhouri K., Sabraoui A., Maalouf F., Rohi L., El Bouhssini M. (2021). First sources of resistance in faba bean (Vicia faba L.) to the stem borer weevil, Lixus algirus L. (Coleoptera: Curculionidae). Phytoparasitica 49, 349–356. doi: 10.1007/s12600-021-00885-0
Ait Taadaouit N., El Fakhouri K., Sabraoui A., Rohi L., El Bouhssini M. (2022). Exploratory survey of lixus algirus L. (Coleoptera: curculionidae) and its natural enemies in Morocco. Insects 13, 681. doi: 10.3390/insects13080681
Ajayi E., Morawo T. O., Fadamiro H. Y. (2015). Preference of flight morph of Callosobruchus maculatus (Coleoptera: Chrysomelidae) for three plant legumes Olufunmilayo. Int. J. Trop. Insect Science 2015, 1–11. doi: 10.1017/S1742758418000152
Akkad R., Kharraz E., Han J., House J. D., Curtis J. M. (2021). The effect of short-term storage temperature on the key headspace volatile compounds observed in Canadian faba bean flour. Food Sci. Technol. Int. 28 (2), 135–143. doi: 10.1177/1082013221998843
Akkad R., Kharraza E., Hanb J., Housec J., Curtisa J. (2019). Characterisation of the volatile flavour compounds in low and high tannin faba beans (Vicia faba var. minor) grown in Alberta, Canada. Food Res. Int. 120, 285–294. doi: 10.1016/j.foodres.2019.02.044
Allmann S., Späthe A., Bisch-Knaden S., Kallenbach M., Reinecke A., Sachse S. (2013). Feeding-induced rearrangement of green leaf volatiles reduces moth oviposition. eLife 2, e00421. doi: 10.7554/eLife.00421.017
Azarnia S., Boye J. I., Warkentin T., Malcolmson L., Sabik H., Bellido A. S. (2011). Volatile flavour profile changes in selected field pea cultivars as affected by crop year and processing. Food Chem. 124, 326–335. doi: 10.1016/j.foodchem.2010.06.041
Baldwin I. T., Kessler A., Halitschke R. (2002). Volatile signaling in plant– plant–herbivore interactions: what is real? Curr. Opin. Plant Biol. 5, 351–354. doi: 10.1016/S1369-5266(02)00263-7
Blake R. S., Mons P. S., Ellis A. M. (2009). Proton-transfer reaction mass spectrometry. Chem. Rev. 109, 861–896. doi: 10.1021/cr800364q
Brilli F., Tsonev T., Mahmood T., Velikova V., Loreto F., Centritto M. (2013). Ultradian variation of isoprene emission, photosynthesis, mesophyll conductance and optimum temperature sensitivity for isoprene emission in water-stressed Eucalyptus citriodora saplings. J. Exp. Bot. 64, 519–528. doi: 10.1093/jxb/ers353
Bruce T. J. A., Martin J. L., Smart L. E., Pickett J. A. (2011). Development of semiochemical attractants for monitoring bean seed beetle, Bruchus rufimanus. Pest Manage. Sci. 67, 1303–1308. doi: 10.1002/ps.2186
Bruno D., Grossi G., Salvia R., Scala A., Farina D., Grimaldi A., et al. (2018). Sensilla morphology and complex expression pattern of odorant binding proteins in the vetch aphid megoura viciae (Hemiptera: aphididae). Front. Physiol. 9, 777. doi: 10.3389/fphys.2018.00777
Burdock G. A. (2010). Handbook of flavor ingredients. 6th ed (Boca Raton, FL, USA: CRC Press/Taylor & Francis Group).
Cai J., Zhua Y. Y., Maa R., Thakura K., Zhanga J. G. (2021). Effects of roasting level on physicochemical, sensory, and volatile profiles of soybeans using electronic nose and HS-SPME-GC–MS. Food Chem. 340, 127880. doi: 10.1016/j.foodchem.2020.127880
Castro A. M., Tapias J., Ortiz A., Benavides P., Góngora C. E. (2017). Identification of attractant and repellent plants to coffee berry borer, Hypothenemus hampei. Entomologia Experimentalis Applicata 164, 120–130.
Ceballos R., Fernández N., Zúñiga S., Zapata N. (2015). Electrophysiological and behavioral responses of pea weevil Bruchus pisorum L. (Coleóptera: Bruchidae) to volatiles collected from its host Pisum sativum L. Chilean. J. Agric. Res. 75 (2), 202-209. doi: 10.4067/S0718–58392015000200009
Cellini A., Blasioli S., Biondi E., Bertaccini A., Braschi I., Spinelli F. (2017). Potential applications and limitations of electronic nose devices for plant disease diagnosis. Sensors 17, 2596. doi: 10.3390/s17112596
Cellini A., Spinelli F., Donati I., Ryu C. M., Kloepper J. W. (2021). Bacterial volatile compound-based tools for crop management and quality. Trends Plant Sci. 26, 968–983. doi: 10.1016/j.tplants.2021.05.006
Centritto M., Brilli F., Fodale R., Loreto F. (2011). Different sensitivity of isoprene emission, respiration, and photosynthesis to high growth temperature coupled with drought stress in black poplar (Populus nigra). Tree Physiol. 31, 275–286. doi: 10.1093/treephys/tpq112
Choudhary D. K., Johri B. N., Prakash A. (2008). Volatiles as priming agents that initiate plant growth and defence responses. Curr. Sci. 94, 595–604. Available at: https://www.jstor.org/stable/24100299.
Cui S., Ling P., Zhu H., Keener H. M. (2018). Plant pest detection using an artificial nose system: A review. Sensors 18, 378. doi: 10.3390/s18020378
Das A., Lee S. H., Hyun T. K., Kim S. W., Kim J. Y. (2012). Plant volatiles as method of communication. Plant Biotechnol. Rep. 7, 9–26. doi: 10.1007/s11816-012-0236-1
Das B. C., Sarker P. K., Rahman M. M. (2008). Aphidicidal activity of some indigenous plant extracts against bean aphid Aphis craccivora Koch (Homoptera: Aphididae). J. Pest Sci. 81, 153–159. doi: 10.1007/s10340-008-0200-6
Delory B. M., Delaplace P., Fauconnier M. L., du Jardin P. (2016). Rootemitted volatile organic compounds: can they mediate belowground plant-plant interactions? Plant Soil 402, 1–26. doi: 10.1007/s11104–016-2823–3
Diabate S., Deletre E., Murungi L. K., Fiaboe K. M., Wesonga J., Martin T. (2019). Behavioural response of alate Aphis craccivora Koch (Homoptera: Aphididae) to volatiles from different cowpea cultivars. J. Appl. Entomol. 143, 659–669. doi: 10.1111/jen.12633
Dicke M. (2009). Behavioural and community ecology of plants that cry for help. Plant Cell Environ. 32, 654–665. doi: 10.1111/j.1365-3040.2008.01913.x
Dicke M., Loreto F. (2010). Induced plant volatiles: from genes to climate change. Trends Plant Science. 15, 115–117. doi: 10.1016/j.tplants.2010.01.007
Dudareva N., Negre F., Nagegowda D. A., Orlova I. (2006). Plant volatiles: recent advances and future perspectives. Crit. Rev. Plant Sci. 25, 417–440. doi: 10.1080/07352680600899973
Effah E., Holopainen J. K., McCormick A. C. (2019). Potential roles of volatile organic compounds in plant competition. Perspect. Plant Ecol. Evol. Syst. 38, 58–63. doi: 10.1016/j.ppees.2019.04.003
Effmert U., Kalderas J., Warnke R., Piechulla B. (2012). Volatile mediated interactions between bacteria and fungi in the soil. J. Chem. Ecol. 38, 665–703. doi: 10.1007/s10886-012-0135-5
El Fakhouri K., Huang J., El Bouhssini M., Kadmiri I., Aasfar A., Gut L. (2021). Screening of volatile compounds used in host location by the faba bean stem borer, Lixus algirus on faba bean in Morocco (Denver, Colorado: The Entomological Society of America's Annual Meeting).
Eller A. S. D., Graus M., Monson R. K. (2012). Variation among different genotypes of hybrid poplar with regard to leaf volatile organic compound emissions. Ecol. Applications. 22, 1865–1875. doi: 10.1890/11-2273.1
Fang Y., Ramasamy R. P. (2015). Current and prospective methods for plant disease detection. Biosensors 5, 537–561. doi: 10.3390/bios5030537
Fares S., Mahmood T., Liu S., Loreto F., Centritto M. (2011). Influence of growth temperature and measuring temperature on isoprene emission, diffusive limitations of photosynthesis and respiration in hybrid poplars. Atmos Environ. 45, 155–161. doi: 10.1016/j.atmosenv.2010.09.036
Farneti B., Cristescu S. M., Costa G., Harren F. J. M., Woltering E. J. (2012). Rapid tomato volatile profiling by using proton-transfer reaction mass spectrometry (PTR-MS). J. Food Science. 77, 551–559. doi: 10.1111/j.1750-3841.2012.02679.x
Farneti B., Khomenko I., Cappellin L., Ting V., Romano A., Biasioli F., et al. (2014). Comprehensive VOC profiling of an apple germplasm collection by PTR-ToF-MS. Metabolomics 11, 838–850. doi: 10.1007/s11306-014-0744-9
Ferawati F., Witthöft C., Bergström M. (2020). Characterization of volatile compounds in Swedish yellow and gray peas: Implications for new legume-based ingredients. Legume Sci. 2, 55. doi: 10.1002/leg3.55
Ficke F., Asalf B., Norli H. R. (2021). Volatile organic compound profiles from wheat diseases are pathogen-specific and can be exploited for disease classification. Front. Microbiol. 12. doi: 10.3389/fmicb.2021.803352
Fineschi S., Loreto F. (2012). Leaf volatile isoprenoids: an important defensive armament in forest tree species. IForest 5, 13–17. doi: 10.3832/ifor0607-009
Gualtieri L., Monti M. M., Mele F., Russo A., Pedata P. A., Ruocco M. (2022). Volatile organic compound (VOC) profiles of different trichoderma species and their potential application. J. Fungi 8, 989. doi: 10.3390/jof8100989
Holopainen J. K., Gershenzon J. (2010). Multiple stress factors and the emission of plant VOCs. Trends Plant Sci. 15, 176–184. doi: 10.1016/j.tplants.2010.01.006
Hossain M. M., Hossain N., Sultana F., Islam S. M. N., Islam M. S., Bhuiyan M. K. A. (2013). Integrated management of Fusarium wilt of chickpea (Cicer arietinum L.) caused by Fusarium oxysporum f. sp. ciceris with microbial antagonist, botanical extract and fungicide. Afr. J. Microbiol. 12, 4699–4706. doi: 10.5897/AJB2013.12503
Houasli C., Sahri A., Nsarellah N. (2020). Chickpea (Cicer arietinum L.) breeding in Morocco: genetic gain and stability of grain yield and seed size under winter planting conditions. Euphytica 217, 159. doi: 10.1007/s10681–021-02885-x
Ichiki R. T., Kainoh Y., Kugimiya S., Takabayashi J., Nakamura S. (2008). Attraction to herbivore-induced plant volatiles by the host-foraging parasitoid fly Exorista japonica. J. Chem. Ecol. 34, 614–621. doi: 10.1007/s10886-008-9459-6
Jansen R. M. C., Wildt J., Kappers I. F., Bouwmeester H. J., Hofstee J. W., Henten E. J. (2011). Detection of diseased plants by analysis of volatile organic compound emission. Annu. Rev. Phytopathology. 49, 157–174. doi: 10.1146/annurev-phyto-072910-095227
Junker R. R., Tholl D. (2013). Volatile organic compound mediated interactions at the plant-microbe interface. J. Chem. Ecol. 39, 810–825. doi: 10.1007/s10886-013-0325-9
Karolkowski A., Guichard E., Briand L., Salles C. (2021). Volatile compounds in pulses A review. Foods 10, 3140. doi: 10.3390/foods10123140
Kessler A., Baldwin I. T. (2001). Defensive function of herbivore-induced plant volatile emissions in nature. Science 291, 2141–2144. doi: 10.1126/science.291.5511.2141
Kihika R., Murungi L. K., Coyne D., Ng’ang’a M., Hassanali A., Teal P. E. A. (2017). Parasitic nematode Meloidogyne incognita interactions with different capsicum annum cultivars reveal the chemical constituents modulating root herbivory. Sci. Rep. 7, 2903. doi: 10.1038/s41598–017-02379–8
Kindlovits S., Sárosi S., Inotai K., Petrović G., Stojanović G., Németh (2018). Phytochemical characteristics of root volatiles and extracts of achillea collina becker genotypes. J. Essential Oil Res. 30, 330–340. doi: 10.1080/10412905.2018.1470581
Kramer R., Abraham W. R. (2012). Volatile sesquiterpenes from fungi: What are they good for. Phytochem 11, 15–37. doi: 10.1007/s11101-011-9216-2
Kumar M., Kumar V., Rana M., Srivastava S. (2019). Effect of volatile and nonvolatile compounds of Trichoderma spp. Against Fusarium isolates causing chickpea wil in Punja. Plant Archives. 19, 159–162.
Liu X., Cheng S., Liu H., Hu S., Zhang D., Ning H. (2012). A survey on gas sensing technology. Sensors 12, 9635–9665. doi: 10.3390/s120709635
López M. A., Vicente J., Kulasekaran S., Vellosillo T., Martínez M., Irigoyen M. L., et al. (2011). Antagonistic role of 9-lipoxygenase-derived oxylipins and ethylene in the control of oxidative stress, lipid peroxidation and plant defence. Plant J. 67, 447–458. doi: 10.1111/j.1365-313x.2011.04608.x
Loreto F., Schnitzler J. P. (2010). Abiotic stresses and induced BVOCs. Trends Plant Sci. 15, 154–166. doi: 10.1016/j.tplants.2009.12.006
MacDougall S., Bayansal F., Ahmadi A. (2022). Emerging methods of monitoring volatile organic compounds for detection of plant pests and disease. Biosensors 12, 239. doi: 10.3390/bios12040239
Maffei M. E., Mithofer A., Boland W. (2007). Insects feeding on plants: rapid signals and responses preceding the induction of phytochemical release. Phytochemistry 68 (22-24), 2946–2959.
Maffei M. E., Gertsch J., Appendino G. (2011). Plant volatiles: production, function and pharmacology. Nat. Prod. Rep. 28, 1359–1380. doi: 10.1039/c1np00021g
Majchrzak T., Wojnowski W., Wasik A. (2019). Proton Transfer Reaction Mass spectrometry for plant metabolomics. Trends Plant Sci. 25 (3), 313–314. doi: 10.1016/j.tplants.2019.08.002
Materic D., Bruhn D., Turner C., Morgan G., Mason N., Gauci V. (2015). Methods in plant foliar volatile organic compounds research. Appl. Plant Sci. 3, 1500044. doi: 10.3732/apps.1500044
Merkuz A., Seid A., Chemeda F., Sakhuja P. K., Getachew A. (2011). Effect of mustard green manure and driedplant residue on chickpea wilt (Fusarium oxysporum f.sp. ciceris). Arch. Phytopathol. Pl. Protec. 44, 821–831. doi: 10.1080/03235408.2010.490390
Mhlanga N. M., Murphy A. M., Wamonje F. O., Cunniffe N. J., Caulfield J. C., Glover B. J., et al. (2021). An innate preference of bumblebees for volatile organic compounds emitted by phaseolus vulgaris plants infected with three different viruses. Front. Ecol. Evol. 9. doi: 10.3389/fevo.2021.626851
Mitra P., Das S., Debnath R., Mobarak S. H., Barik A. (2020). Identification of Lathyrus sativus plant volatiles causing behavioral preference of Aphis craccivora. Pest Manag Sci. 77 (1), 285–299. doi: 10.1002/ps.6018
Morath S. U., Hung R., Bennett J. W. (2012). Fungal volatile organic compounds: A review with emphasis on their biotechnological potential. Fungal Biol. Rev. 26, 73–83. doi: 10.1016/j.fbr.2012.07.001
Mumm R., Hilker M. (2006). Direct and indirect chemical defence of pine against folivorous insects. Trends Plant Sci. 11, 351–358. doi: 10.1016/j.tplants.2006.05.007
Murat C., Gourrat K., Jerosch H., Cayot N. (2012). Analytical comparison and sensory representativity of SAFE, SPME, and Purge and Trap extracts of volatile compounds from pea flour. Food Chem. 135, 913–920. doi: 10.1016/j.foodchem.2012.06.015
Murungi L. K., Kirwa H., Coyne D., Teal P. E. A., Beck J. J., Torto B. (2018). Identification of key root volatiles signaling preference of tomato over spinach by the root knot nematode Meloidogyne incognita. J. Agric. Food Chem. 66 (28), 7328–7336. doi: 10.1021/acs.jafc.8b03257
Mutyambai D. M., Bruce T. J., van den Berg J., Midega C. A., Pickett J. A., Khan Z. R. (2016). An indirect defence trait mediated through igg-induced maize volatiles from neighbouring plants. PloS One 11, 0158744. doi: 10.1371/journal.pone.0158744
Niederbacher B., Winkler J. B., Schnitzler J. P. (2015). Volatile organic compounds as non-invasive markers for plant phenotyping. J. Exp. Botany. 66, 5403–5416. doi: 10.1093/jxb/erv219
Ode P. J. (2013). “Plant defences and parasitoid chemical ecology,” in Chemical ecology of insect parasitoids. Eds. Wajnberg E., Colazza S. (Wiley, Oxford), 11–28.
Oomah B. D., Liang L. S. Y. (2007). Volatile compounds of dry beans (Phaseolus vulgaris L.). Plant Foods Hum. Nutr. 62, 177–183. doi: 10.1007/s11130-007-0059-3
Oomah B. D., Razafindrainibe M., Drover J. C. G. (2014). Headspace volatile components of canadian grown low-tannin faba bean (Vicia faba, L.) genotypes. J. Sci. Food Agric. 94, 473–481. doi: 10.1002/jsfa.6272
Papet Y., Brunet B., Mura P. (2010). Headspace (HS) et micro-extraction en phase solide (SPME). Headspace (HS) and solid phase micro-extraction (SPME), Theory and applications. Ann. Toxicol. Anal. 22, 75–79. doi: 10.1051/ata/2010022
Pichersky E., Gershenzon J. (2002). The formation and function of plant volatiles: perfumes for pollinator attraction and defense. Curr. Opin. Plant Biol. 5, 237–243. doi: 10.1016/S1369-5266(02)00251-0
Piechulla B., Lemfack M. C., Kai M. (2017). Effects of discrete bioactive microbial volatiles on plants and fungi. In Plant Cell Environment. 40, 2042–2067. doi: 10.1111/pce.13011
Piesik D., Pańka D., Delaney K. J., Skoczek A., Lamparski R., Weaver D. K. (2011). Cereal crop volatile organic compound induction after mechanical injury, beetle herbivory (Oulema spp.), or fungal infection (Fusarium spp.). Plant Physiol. 168(9), 878–886. doi: 10.1016/j.jplph.2010.11.010
Qualley A. V., Dudareva N. (2009). Metabolomics of plant volatilesMethods, Mol. Biol 553, 329–343. doi: 10.1007/978-1-60327-563-7_17
Reisenman C. E., Lei H., Guerenstein P. G. (2016). Neuroethology of olfactory-guided behavior and its potential application in the control of harmful insects. Front. Physiol. 7, 271. doi: 10.3389/fphys.2016.00271
Rering C. C., Gaffke A. M., Rudolph A. B., Beck J. J., Alborn H. T. (2020). A comparison of collection methods for microbial volatiles. Front. Sustain. Food Syst. 4. doi: 10.3389/fsufs.2020.598967
Roland W. S. U., Pouvreau L., Curran J., van de Velde F., de Kok P. M. T. (2017). Flavor aspects of pulse ingredients. Cereal Chem. J. 94, 58–65. doi: 10.1094/CCHEM-06-16-0161-FI
Ruther J., Kleier S. (2005). Plant–plant signaling: Ethylene synergizes volatile emission in Zea mays induced by exposure to ( Z )-3- Hexen-1-ol. J. Chem. Ecol. 31, 2217–2222. doi: 10.1007/s10886-005-6413-8
Sabraoui A., Lhaloui S., Bouchelta A., El Fakhouri K., El Bouhssini M. (2019). Grain yield losses due to leaf miner (Liriomyza cicerina R.) in winter- and spring-planted chickpea in Morocco. Crop Prot. 117, 115–120. doi: 10.1016/j.cropro.2018.11.021
Santos C. D., Cabot J. C. (2015). Persistent effects after camphor ingestion: A case report and literature review. J. Emergency Med. 48, 298–304. doi: 10.1016/j.jemermed.2014.05.015
Schenkel D., Lemfack M. C., Piechulla B., Splivallo R. (2015). A metaanalysis approach for assessing the diversity and specificity of belowground root and microbial volatiles. Front. Plant Sci. 6. doi: 10.3389/fpls.2015.00707
Schmidt R., Cordovez V., De Boer W., Raaijmakers J., Garbeva P. (2015). Volatile affairs in microbial interactions. ISME J. 9, 2329–2335. doi: 10.1038/ismej.2015.42
Schulz S., Dickschat J. S. (2007). Bacterial volatiles: the smell of small organisms. Nat. Prod. Rep. 24, 814–842. doi: 10.1039/b507392h
Schwartzberg E. G., Böröczky K., Tumlinson J. H. (2011). Pea aphids, acyrthosiphon pisum, suppress induced plant volatiles in Broad Bean, Vicia Faba. J. Chem. Ecol. 37, 1055–1062. doi: 10.1007/s10886-011-0006-5
Semba R. D., Ramsing R., Rahman N. (2021). Legumes as a sustainable source of protein in human diets. Global Food Security. 28, 100520. doi: 10.1016/j.gfs.2021.100520
Singh K. D., Mobolade A. J., Bharali R. (2021). Main plant volatiles as stored grain pest management approach: A review. J. Agric. Food Res. 4, 100127. doi: 10.1016/j.jafr.2021.100127
Splivallo R., Valdez N., Kirchhoff N., Ona M. C., Schmidt J. P., Feussner I., et al. (2012). Intraspecific genotypic variability determines concentrations of key truffle volatiles. New Phytologist. 194, 823–835. doi: 10.1111/j.1469-8137.2012.04077.x
Stierlin E. (2020). Développements analytiques et approche métabolomique pour l'étude des composés volatils d'origine végétale. University of côte d’Azur, Nice, France.
Tholl D., Boland W., Hansel A., Loreto F., Röse U. S. R., Schnitzler J. P. (2006). Practical approaches to plant volatile analysis. Plant J. 45, 540560. doi: 10.1111/j.1365-313X.2005.02612.x
Tholl D., Hossain O., Weinhold A., Röse U. S. R., Wei Q. (2021). Trends and applications in plant volatile sampling and analysis. Plant J. 106, 314–325. doi: 10.1111/tpj.15176
Tisch U., Haick H. (2010). Nanomaterials for cross-reactive sensor arrays. Mrs Bull. 35, 797–780. doi: 10.1557/mrs2010.509
Tyc O., Song C., Dickschat J. S., Vos M., Garbeva P. (2017). The ecological role of volatile and soluble secondary metabolites produced by soil bacteria. Trends Microbiol. 25, 280–292. doi: 10.1016/j.tim.2016.12.002
Vangoethem V. (2017). Characterization of volatile organiual compounds emitted by Fusarium culmorum and cochliobolus sativus under stress and study of their potential in biological control. University of Gembloux Agro-Bio Tech Chicago, Gembloux, Belgium.
Vas G., Vékey K. (2004). Solid-phase microextraction: A powerful sample preparation tool prior to mass spectrometric analysis. J. Mass Spectrometry. 39, 233–254. doi: 10.1002/jms.606
Vincenti S., Mariani M., Alberti J. C., Jacopini S., Brunini-Bronzini de Caraffa V., Berti L., et al. (2019). Biocatalytic synthesis of natural green leaf volatiles using the lipoxygenase metabolic pathway. Catalysts 9, 873.
Webster B., Bruce T. J. A., Dufour S., Birkemeyer C., Birkett M. A., Hardie J. A., et al. (2008). Identification of volatile compounds used in host location by the black bean aphid, aphis fabae. J. Chem. Ecol. 34, 1153–1161. doi: 10.1007/s10886-008-9510-7
Wyatt T. D. (2018). Queen pheromones, colony odors, and better science: a comment on Holman. Behav. Ecol. 29 (6), 1211-1212. doi: 10.1093/beheco/ary074
Xie X., Ling J., Mao Z., Li Y., Zhao J., Yang Y. (2022). Negative regulation of root-knot nematode parasitic behavior by root-derived volatiles of wild relatives of Cucumis metuliferus CM3. Horticulture Res. 9, uhac051. doi: 10.1093/hr/uhac051
Yigezu Y. A., El-Shater T., Boughlala M. (2019). Legume-based rotations have clear economic advantages over cereal monocropping in dry areas. Agron. Sustain. Dev. 39, 58. doi: 10.1007/s13593-019-0602-2
Zhang Z., Li G. (2010). A review of advances and new developments in the analysis of biological volatile organic compounds. Microchem. J. 95, 127–139.
Zhao X., Sun L., Zhang X., Wang M., Liu H., Zhu Y. (2021). Nutritional components, volatile constituents and antioxidant activities of 6 chickpea species. Food Biosci. 41, 100964. doi: 10.1016/j.fbio.2021.100964
Keywords: volatile organic compounds, pulses crops, VOC extraction, analytical techniques, GC-MS, real-time detection, gas-sensing technologies, crop protection
Citation: Makhlouf L, El Fakhouri K, Kemal SA, Aasfar A, Meftah Kadmiri I and El Bouhssini M (2024) Advances in analytical techniques for assessing volatile organic compounds in pulse crops: a comprehensive review. Front. Hortic. 3:1394041. doi: 10.3389/fhort.2024.1394041
Received: 29 February 2024; Accepted: 23 May 2024;
Published: 25 June 2024.
Edited by:
Qingshan Wei, North Carolina State University, United StatesCopyright © 2024 Makhlouf, El Fakhouri, Kemal, Aasfar, Meftah Kadmiri and El Bouhssini. This is an open-access article distributed under the terms of the Creative Commons Attribution License (CC BY). The use, distribution or reproduction in other forums is permitted, provided the original author(s) and the copyright owner(s) are credited and that the original publication in this journal is cited, in accordance with accepted academic practice. No use, distribution or reproduction is permitted which does not comply with these terms.
*Correspondence: Leila Makhlouf, TGVpbGEubWFraGxvdWZAdW02cC5tYQ==