- 1Department of Obstetrics and Gynaecology, Li Ka Shing (LKS) Faculty of Medicine, The University of Hong Kong, Hong Kong, Hong Kong
- 2The University of Hong Kong Shenzhen Key Laboratory of Fertility Regulation, The University of Hong Kong-Shenzhen Hospital, Shenzhen, China
Exosomes are a subset of extracellular vesicles with an average diameter of ~100nm. Exosomes are released by all cells through an endosome-dependent pathway and carry nucleic acids, proteins, lipids, cytokines and metabolites, mirroring the state of the originating cells. The function of exosomes has been implicated in various reproduction processes, such as embryo development, implantation, decidualization and placentation. Placenta-derived exosomes (pEXO) can be detected in the maternal blood as early as 6 weeks after conception and their levels increase with gestational age. Importantly, alternations in the molecular signatures of pEXO are observed in pregnancy-related complications. Thus, these differentially expressed molecules could be the potential biomarkers for diagnosis of the pregnancy-associated diseases. Recent studies have demonstrated that pEXO play a key role in the establishment of maternal immune tolerance, which is critical for a successful pregnancy. To gain a better understanding of the underlying mechanism, we highlighted the advanced studies of pEXO on immune cells in pregnancy.
Introduction
Pregnancy is a complex process associated with numerous biological changes in the maternal body and our understanding of the complicated relationship between the mother and its semi-allograft fetus is still limited (1). An immune tolerant environment is a prerequisite to a successful pregnancy. However, the understanding of how the fetus avoids maternal immune rejection is an enigma. During pregnancy, the mother needs to have a competent immune system against infection but is tolerant to the developing fetus. Any disruption of the immune tolerance would lead to adverse pregnancy outcomes such as recurrent pregnancy loss (2), miscarriage (3) and preeclampsia (4).
The maternal immune system undergoes a wide variety of biological changes during pregnancy. These include decidual immune cell mobilization, re-distribution and polarization at a local level (5–7) and a universal immunosuppressive state at a systemic level (8, 9). In humans, the trophectoderm of blastocyst protects the growing embryo at implantation (10). After implantation, the syncytiotrophoblast (STB) derived from the trophectoderm, surrounds most of the chorionic villi, and prevents the fetus from a direct contract with the maternal blood. The trophoblasts have a unique human leukocyte antigen (HLA) profile (11). For example, the STBs are HLA null and are considered as immunologically neutral, while the extravillous trophoblast cells (EVTs) express an unusual repertoire of HLA-I molecules including HLA-G, HLA-C and HLA-E (12, 13). Furthermore, the STBs produce various immunoregulatory factors such as interleukin 10 (IL10) (14), macrophage colony-stimulating factor (M-CSF) (15) and IL-35 (16), which contribute to maternal immune tolerance as well.
Exosomes, firstly regarded as cell burden, are involved in the process of antigen presentation, signal transduction and immune responses. Placenta STB has been demonstrated to continuously releases extracellular vesicles (EVs), microvesicles and exosomes, to the maternal circulation (17, 18). The study on pEXO can date back to 1999 (19) and our understanding of pEXO are significantly increased due to advances in technologies of exosome purification in the last decade. Beyond that, exosomes from other sources-such as stem cells and tumor-have a critical role in growth, metabolism and development. The function of pEXO has been implicated in conferring viral resistance to non-placenta cells, inhibiting T cells recognition and activation, and promoting macrophage differentiation and polarization during pregnancy. Here, we summarize the current knowledge of pEXO in the establishment of maternal immune tolerance and outlined an overview role of its application in disease diagnosis.
Placenta-Derived Extracellular Vesicles
Extracellular Vesicles
Communication among our body cells is traditionally considered to be through autocrine, paracrine, endocrine and direct cell-cell contact. Other than that, EVs are another means of cell-cell communication. According to the guidelines of the International Society for Extracellular Vesicles (ISEV), EVs are lipid-bound vesicles with a diameter ranging from 30 nm to 2 μm released from all kinds of cells (20, 21). Based on the biogenesis process, EVs generally fall into two categories, ectosomes and exosomes (Table 1) (20). Ectosomes are vesicles produced by cells via direct outward budding. They can be further divided into microvesicles (MVs, 200 nm ~ 1 μm in diameter) and apoptotic bodies (APs, 1 μm ~ 5 μm in diameter) (22). By contrast, exosomes are nano-sized particles with a size ranging from 30 nm to 200 nm in diameter (100 nm on average) generated by inward budding of the plasma membrane via a multi-vesicular system (21).
Initially, EVs are considered as cell debris for the purpose of maintaining cellular homeostasis (23, 24). EVs carry various molecular cargoes, such as proteins, microRNAs (miRNAs), mRNAs, lipids and metabolites, which endow the EVs with capacity as a natural vehicle for intercellular communication (25). The function of exosomes has been well documented in tumorigenesis, metastasis, regeneration, mammalian reproduction and development (26). Certain miRNAs are enriched in exosomes compared to those in the cells of origin, indicating that the process of exosomes biogenesis is not random, but in a pre-primed manner (27, 28). However, the mechanism underlying the exosomal cargo incorporation is still unclear.
Placenta Syncytial Nuclear Aggregates, Microvesicles and Exosomes
During pregnancy, the placenta actively releases EVs into the bloodstream of the mother. The STB is the major source of placenta-derived EVs in the maternal blood (29, 30). Unlike those EVs that originate from other tissues, placenta-derived EVs are divided into three categories based on their sizes: syncytial nuclear aggregates (SNAs), microvesicles (MVs) and exosomes (Figure 1) (31).
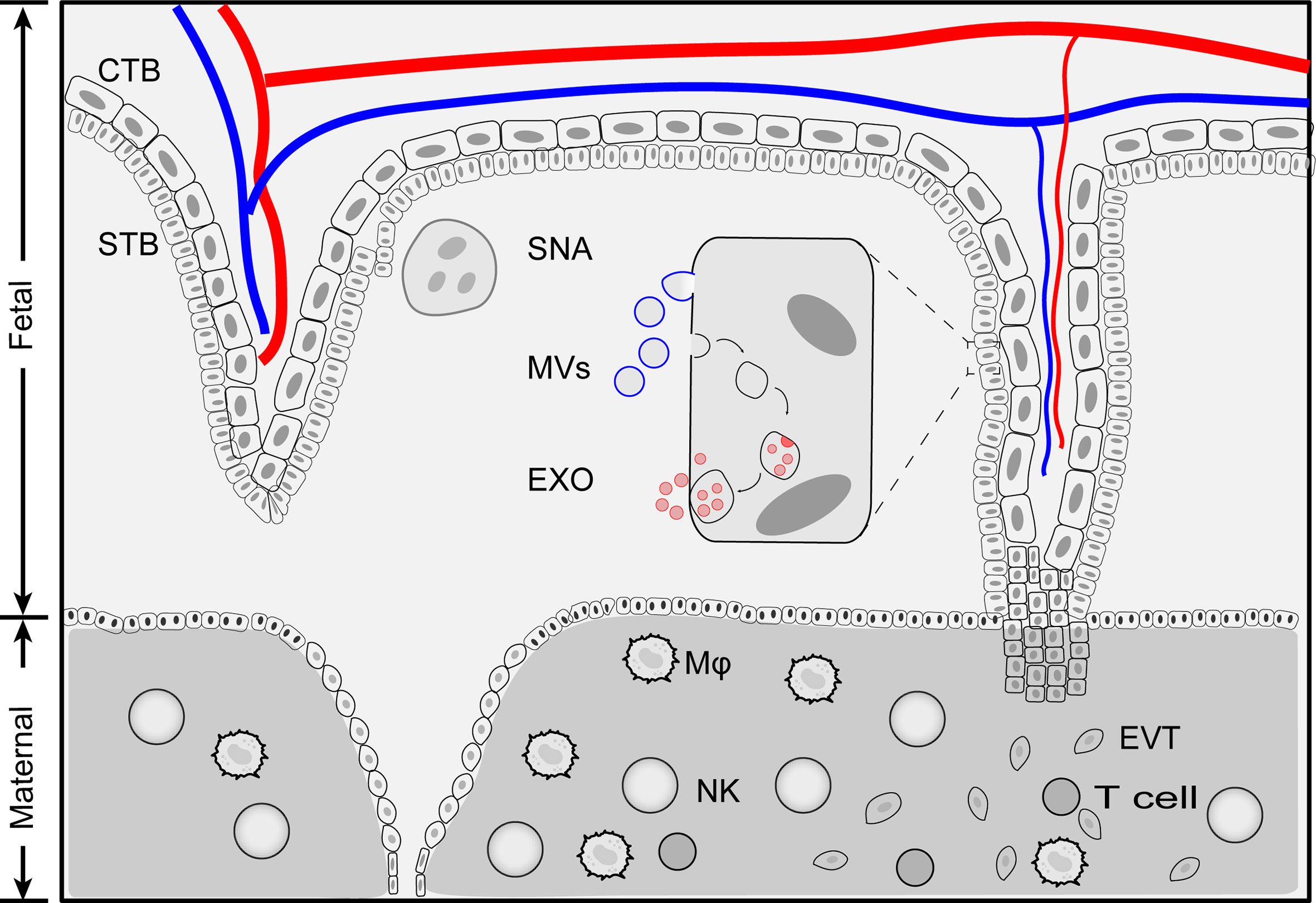
Figure 1 Schematic illustration of placenta extracellular vesicles. Placenta derived extracellular vesicles can be divided into four categories: exosomes, microvesicles, apoptotic bodies and syncytial nuclear aggregates based on size and biogenesis pathway. Exosomes are generated by multivesiculuar body (MVB)-intraluminal vesicles (ILVs) system. first MVBs are generated by plasma membrane inward budding. Further, invagination of the late endosomes forms intraluminal vesicles (exosomes) within multivesiculuar body (MVB). Exosomes release to extracellular space when MVB fuse with membrane plasma. During this processes, membrane components and cytosolic materials are loaded into exosomes. Microvesicles and apoptotic bodies are produced by outward budding of plasma membrane and the size range of 200 nm - 5 μm. Syncytial nuclear aggregates (SNA) are clusters of syncntiotrophoblast with multiple nuclei per SNA. CTB, cytotrophoblast; Exo, exosomes; EVT, extravillous trophoblast; MVs, microvesicles; Mφ, macrophage; NK, Natural killer cells; STB, syncytiotrophoblast; SNA, syncytial nuclear aggregates.
Placenta-derived SNAs, also known as syncytial knot, are the clusters of multinucleated aggregate of syncytial nuclei (20 μm~200 μm in diameter, averaged 60 nuclei per knot) extruded from STB (32). The formation of placenta-derived SNAs is generally considered as a degenerative process, an aging change and an indicator of trophoblastic state when exposed to ischemia or hypoxia (18, 31, 33). The history of placenta-derived SNAs can be dated back to 120 years ago when they were first found in the lungs of post-mortem women (34). However, the origin and formation of SNAs are far from clear. Nuclei within SNAs exhibited condensed morphology compared to the STB and showed little evidence of apoptosis, indicating that SNAs are not fragmented STB (31). SNAs could be used as an alternative source of fetal DNA for prenatal diagnosis (35). The levels of SNAs increase as gestation proceeds and are found to be correlated in pregnancy complications such as preeclampsia (36).
The biological function of placenta-derived MVs is broad, encompassing immune cell activation, proliferation, and endothelial hemostasis (37). MVs collected from normal placenta perfusion have a pro-inflammatory effect via activating monocytes and B cells (38). Inhibition of MV internalization cannot block placenta-derived MV-mediated activation of monocytes and B cells indicating that membrane-bound proteins are the key players of the phenomenon. Proteomic analysis revealed that the differential expressed proteins between MVs from normal pregnancy and preeclampsia patients are related to mitochondria, transmembrane transport and membrane transporter activity (39).
pEXO can interact with various target cells including endothelium, T cells, monocytes, natural killer (NK) cells and macrophages. pEXO are found to protect endothelial cells from viral infection (40), inhibit NK cytotoxicity (41), constrain T cell proliferation (42) and promote monocyte differentiation and macrophage polarization (43). During pregnancy, pEXO can be detected as early as 6 weeks (44) and their number increases gradually and finally peaks at term. Pathologically, the levels of exosomes have been correlated with pregnancy-associated complications such as preeclampsia (45), gestational diabetes mellitus (46) and preterm birth (47), which will be described later in this review. Interestingly, all these complications have been demonstrated to associated with alteration of immune system during pregnancy. However, the detailed roles and mechanisms of pEXO in maternal immune adaption and placental development are still obscure.
pEXO Preparation and Isolation
To date, pEXO are mainly purified from four types of sources: maternal blood, placental perfusate, placental explant culture, and primary trophoblast culture. However, pEXO isolated by different methods have distinct effects on endothelial cells, T cells and other cells [Reviewed in (48–50)]. Generally, the yield of placenta exosomes in the maternal blood is relatively low. On the other hand, the yields of exosomes from placental perfusion and explant culture are relatively high but the purity of the isolated exosomes is a concern. Since differences in content and immunoregulatory activities of exosome from primary cell and its established cell lines have been reported (51–53). Primary cells are currently the best source of exosomes preparation when sample availability is adequate. However, exosomes from trophoblast cell lines with gene manipulation could also provide valuable information regarding trophoblast-specific gene expression and function (54, 55).
Immuno-capture, centrifugation, precipitation, and size exclusion chromatography are commonly used to isolate exosomes from the biological fluid or culture medium (21, 56–59) (Figure 2). The immuno-capture method is commonly used to isolate pEXO in plasma (60). Magnetic beads coated with monoclonal anti-PLAP (placental alkaline phosphatase) antibodies capture placenta-specific exosomes through antigen-antibody interaction. Ultracentrifugation and gradient ultracentrifugation are the most widely used methods in exosome studies. In these methods, EVs are isolated by differential centrifugal forces. Dead cells and cell debris are pelleted with a relatively low centrifugal force (300g for dead cells and 2000g for cellular debris). Higher centrifugal force at 16,500g is then applied to separate the MVs. Exosomes can be harvested by ultracentrifugation at >100,000g, for 60 minutes. To enhance the purity of exosomes, gradient ultracentrifugation is employed to separate different subtypes of exosomes (59). Precipitation is another method for exosome purification (61, 62). Polyethylene glycol (PEG) functions as a water-excluding molecule that precipitates the exosomes out of the aqueous phase. Usually, exosomes are isolated by a low-speed centrifugation after incubating the sample with a precipitation solution containing PEG. However, proteins may also be precipitated by PEG which could result in a lower purity than those generated by ultracentrifugation. Size exclusion chromatography (SEC) has also been used for exosome purification (63, 64). In this method, exosomes and soluble proteins are separated by a porous matrix. Exosomes that are larger than the size cutoff of the matrix are eluted faster than the soluble proteins. Compared to other methods, exosomes isolated by SEC have a higher purity but lower yields. However, all the methods have their limitation in terms of efficiency and purity. To bridge this gap, new technologies and standardization of protocols for pEXO isolation are needed in future studies.
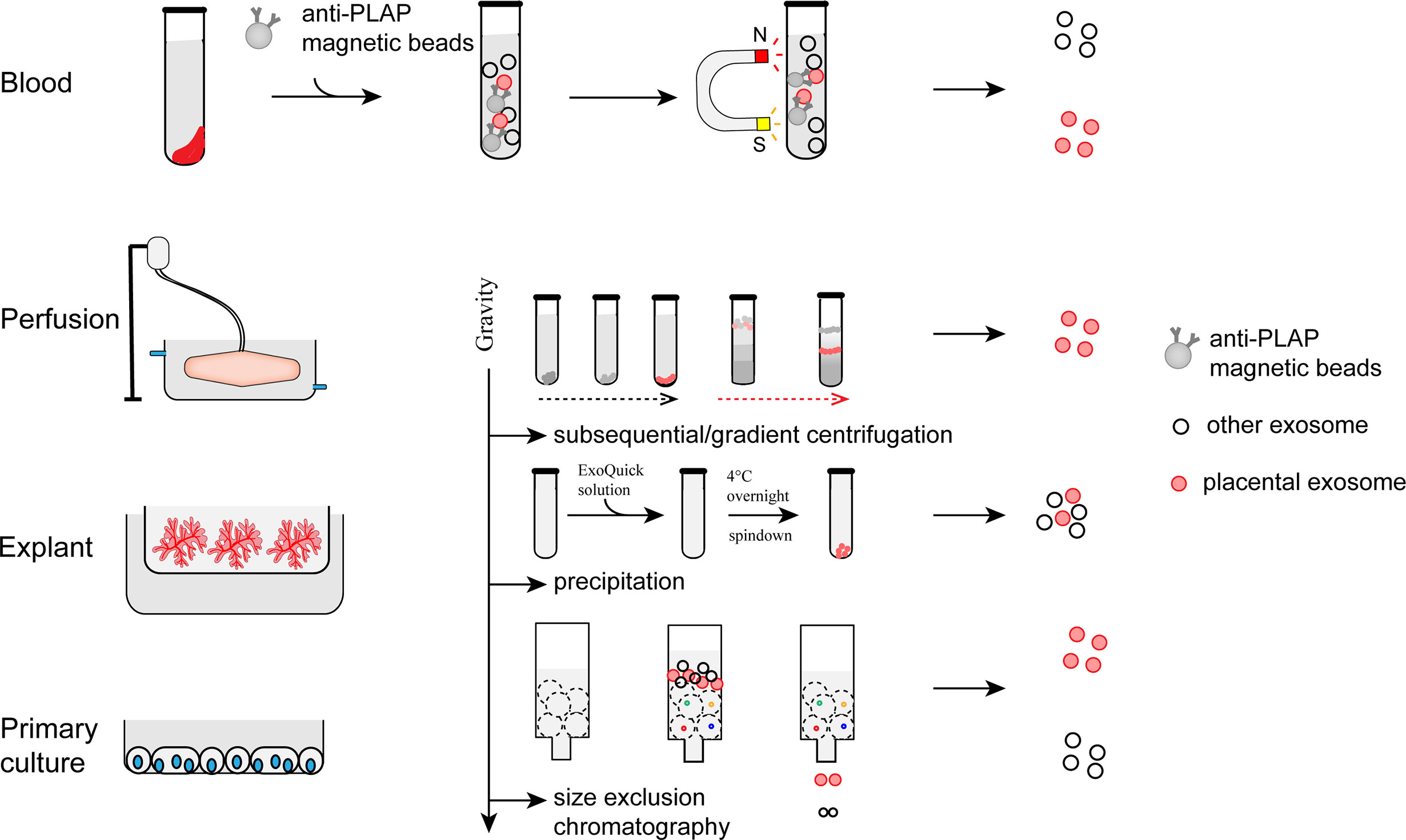
Figure 2 Preparation and isolation of pEXO. Exosomes extruded from placenta can be purified from blood plasma, medium of placental perfusion, explant culture and primary trophoblast culture through immune-capture, ultracentrifugation, gradient centrifugation, or size exclusion chromatography.
Maternal Adaptation of Immune System Response at Early Pregnancy
Placenta-driven immune tolerance is a hallmark of a successful pregnancy when exposed to fetal antigens (65–69). Paternal antigens encounter the maternal immune system when the placenta villi are in contact with the maternal blood and when the EVTs interact with the human decidua. Strikingly, the maternal immune cells are abundant in the human decidua in early pregnancy accounting for 40% of the total decidual cells. Among them, NK cells (70%) and macrophages (20%) are the two largest subpopulations, with the rest constituted by T cells. Dendritic cells and B cells are almost absent in the human decidua (70). Interestingly, endometrium exhibits a sharp increase in NK cells and macrophages and a steep decline in T cells during the secretory phase of menstrual cycle, indicating that hormones may influence immune cell population and functions. Although the total cell numbers of the decidual immune cells in the peri-implantation and post-implantation periods are similar, their phenotypes and functions are dramatically different (71, 72).
It is generally accepted that a T-helper type-2 (Th2) cytokine prevailing environment is important in pregnancy (73). The proportion of Th2 cytokines-secreting cells in the endometrium are significantly higher in pregnant women in the first trimester than in non-pregnant women (73–75). On the other hand, Th1 cytokine-dominated immune responses are associated with implantation failures (76), abortion (77) and preeclampsia (78). The excessive Th1 cytokines are also associated with an elevated number of activated CD8+ T cells (79), M1 macrophages (80), Th-17 cells (81) in the decidua. However, several Th1 cytokines such as interferon (IFN)-γ and tumor necrosis factor-α are important in uterine vascular remodeling (82) and implantation (83), suggesting that the Th-1/Th-2 paradigm for pregnancy may be too simplistic. Recently, the concept of Th-1/Th-2 paradigm was gradually expanded to Th1/Th2/Th17/Treg paradigm due to the discovery of new Th cell subsets at the maternal-fetal interface (84, 85).
Systemic changes in peripheral immune cells are also essential for a successful pregnancy. It is supported by the observations in immunodeficient mice (Table 2). In general, adaptive immune cell-deficient female mice are fertile, whereas innate immune cell-deficient female mice are often accompanied by a compromised reproductive performance. However, the mechanism responsible for this observation is still unknown. The significance of peripheral immune cells in pregnancy is also well manifested in pregnant mothers with rheumatoid arthritis, an autoimmune disease which was partially subsided during pregnancy (97). Peripheral Treg cells, granulocytes and monocytic myeloid-derived suppressor cells (M-MDSC) are significantly increased when compared to non-pregnant women (98–100). In contrast, the number of T cells and B cells remain stable (8, 101). Moreover, the cytotoxicity of peripheral NK cells from pregnant women is well constrained when compared to non-pregnant individuals (102, 103).
pEXO as a Modulator of Maternal Immune Tolerance
Given that exosomes, but not other EVs, are generated through the endosomal pathway, biological molecules encompassed by the exosomes are believed to have specific functions in cell-cell crosstalk. The placenta secretes a large number of exosomes into the maternal circulation. The NK cells, macrophages and T-cells are the three largest cell types making up >90% of the immune cells at the fetal-maternal interface in the post-implantation period. Thus, this review focuses on the effect of pEXO on these three immune cell populations. Yet it should be emphasized that exosomes are also involved in mediating the bi-direction communications between endometrium and embryo during peri-implantation and implantation phase (104–106). For example, endometrial epithelial cell-derived exosomes promote embryo attachment during implantation via miR-30d-dependent upregulation of integrins or through activation of focal adhesion kinase (FAK) signaling pathway (107, 108). Another study shows that diapausing endometrial epithelial cell-derived exosomes enriched with miR-let-7 can protect the embryo from collapsing (109). Conversely, embryo-derived exosomes have been detected in spent embryo culture medium. These exosomes can be internalized by endometrial epithelial/stromal cells (110, 111) and promote endometrial receptivity (112–114).
Natural Killer Cells
Peripheral Blood Natural Killer Cells
NK cells in peripheral blood are divided into two groups: over 90% of peripheral NK cells (pNK) are CD56dim CD16+ which are cytotoxic cells; the rest are CD56+ CD16- NK cells which are less cytotoxic and can migrate into peripheral tissues. Compare to non-pregnant, pNK of pregnant women have a higher expression of Tim-3 (115), galectin-1 (102) and lower secretion of IFN-γ (103). The cytotoxic activities of pNK at early pregnancy are controversial (101, 116). Moreover, overactivated pNK are associated with repeated implantation and unexplained spontaneous abortion (117). Currently, there is no report on the effect of pEXO on pNK.
Endometrial NK Cells (eNK) and Decidual NK Cells (dNK)
NK cells represent the largest fraction of lymphocytes in the endometrium during the late-secretory phase and early pregnancy. Unlike pNK, majority of the endometrium NK cells (eNK) are CD56+ CD16- with a minority being cytotoxic CD56dim CD16+. The transformation from eNK to decidual NK cells (dNK) occurs upon implantation, resulting in two cell subsets with distinct transcriptional profiles. Strikingly, the eNK are more active than the dNK as 70% of differentially expressed genes are highly expressed in the eNK (118). On the other hand, the eNK have no expression of NKp30 and cannot produce VEGF and placental growth factor (119). The phenotype and KIR repertoire are also different between the two type NKs; the dNK have a higher expression of KIR2D, the killer immunoglobulin-like receptor for HLA-C recognition than the circulating NK and the non-pregnant eNK (72, 120).
Decidual NK cells (dNK) are abundant in the maternal-fetal interface at early pregnancy- accounting for up to 70% of total lymphocytes in decidua (121). The number of dNK gradually increases upon embryo implantation, peaks at 8-10 weeks of gestation, and returns to the original level at term. In general, dNK have low cytotoxicity and are prone to produce more growth factors with immunomodulatory activities (122). Elevated dNK cytotoxicity is associated with recurrent spontaneous abortion due to increased lysis activity (123). This was, in part, mediated by the up-regulation of NKp44 and NKp46 cytotoxicity receptors on CD56bright CD16- and CD56dimCD16+ cells (124). In fetal/neonatal alloimmune thrombocytopenia (FNAIT), activated dNK with increased cytotoxicity induce trophoblast apoptosis (125). A recent single-cell study (126) classified the dNK into three subsets: dNK1 (CD39+KIR2DL+ITGB2-CD103-), dNK2 (CD39-KIR2DL+ITGB2+CD103-) and dNK3 (CD39-KIR2DL-ITGB2+). The origin of the dNK remains uncertain, though they are thought to be derived from the NK precursor in the endometrium, or recruited from the circulating NK (126) and/or renewed by the CD34+ progenitor cells (127).
dNK are localized closely to EVT and spiral artery (128). They are vital to various processes of pregnancy including embryo implantation, immunomodulation, trophoblast differentiation and invasion, and endothelial cell remodeling. dNK also express unique NK receptors (e.g. 2B4, KIR2DL, ILT2) for interaction with their corresponding ligands (e.g. HLA-C, -E, -G) on EVT to fine-tune their cytolytic activity (129) within the maternal-fetal interface during the first trimester of pregnancy. Recent studies also suggest novel properties of dNK such as providing osteoglycin (OGN) and osteopontin (OPT) for fetal development (130) and selectively killing the pathogenic bacteria inside the trophoblast by injection of granulysin through nanotubes (131).
Effect of pEXO on NK Cells
pEXO can be internalized by NK cells in vivo (132) and in vitro (Supplementary Table 1) (133), which mediate the crosstalk between the placenta and the maternal immune system. The cytotoxic activity of NK cells mainly attributes to its activating receptors on the plasma membrane. NK group 2 member D (NKG2D) is widely expressed on the NK cells, activated CD8+ T cells and macrophages for removal of infected cells or foreign pathogens. NKG2D is remarkably downregulated in NK cells by NKG2D ligands expressed on pEXO (Supplementary Table 1) (134).
NKp30 is another activating receptor on the NK cells responsible for eliminating cancer cells and inducing dendritic cells maturation by secretion of tumor necrosis factor-alpha (TNF-α), interferon-gamma (IFN-γ), perforins and granzymes (135). B7H6, one of NKp30 endogenous ligands, is widely expressed on cancer cells and trophoblasts, while soluble B7H6 (sB7H6) was a decoy agent for ligand-receptor interaction and compromising NK cytotoxicity. High levels of exosome-packed sB7H6 or soluble B7H6 are correlated with poor tumor prognosis, likely due to inhibition of the NK cytotoxicity against the tumor cells (136). During pregnancy, both exosome-packed B7H6 and sB7H6 are present in the serum of pregnant women (Supplementary Table 1), indicating its potential contribution via a similar mechanism to inhibit NK cells in the establishment of maternal immune tolerance (137).
In addition to reducing the cytotoxicity of NK cells, exosomes from the serum of pregnant women can selectively increase the caspase-3 activity in CD56dim NK cells, pointing to an alternative way of exosome-mediated immune tolerance by inducing apoptosis of the CD56dim NK cells (Supplementary Table 1) (133). pEXO proteomic study showed that glycodelin A (GdA), a glycoprotein with immunosuppressive activities, is abundantly expressed in human decidua and pEXO (138). We demonstrated that decidua-derived GdA stimulated the conversion of peripheral CD56bright CD16- NK cells to cells with a decidual NK cell-like phenotype via upregulation of CD9, CD49a and production of VEGF (139). Together, this evidence indicated that the pEXO contribute to maternal immune tolerance through modulating NK cytotoxicity, inducing CD56dim NK cells apoptosis and promoting the development of decidual NK cell-like phenotype.
Monocytes and Macrophages
Peripheral Blood Monocytes
Circulating monocytes are the primary phagocytic cells and the major APCs in blood (140). Notably, monocytes are able to differentiate into dendritic cells and macrophages for antigen presentation and removal of foreign pathogens, respectively. In humans, peripheral monocytes can be divided into three main subtypes based on the expression of CD14 and CD16 (141): classical monocytes (CD14++CD16-); intermediate monocytes (CD14+CD16+) and non-classical monocytes (CD14-CD16++). Approximately 80% of the total monocytes are classical monocytes, while the non-classical monocytes comprise about 2-11%. Non-classical monocytes retain a highly inflammatory characteristic and their number is elevated in both chronic and acute inflammation. The population of the intermediate monocytes (2-8%) with both inflammatory and phagocytic capacities expands during ZIKA viral infection and is the main target for ZIKA infection during pregnancy (142). Despite the conflicting results on the proportion of classical monocytes in peripheral blood between pregnant and non-pregnant women, classical monocyte number is lower in pregnancy complications such as preeclampsia (143, 144), indicating a possible regulatory role of monocyte in pregnancy.
Decidual Macrophages
Decidual macrophages are the second most abundant type of lymphocytes (~20%) and the major antigen-presenting cells (APC) in human decidua during early pregnancy (70). They contribute to maternal-fetal immune homeostasis, spiral artery remodeling and trophoblast functions (145). Decidual macrophages display the transcriptional profile of both classically activated macrophages (M1 macrophages) for immune activation and alternatively activated macrophages (M2 macrophages) with anti-inflammatory and immunosuppressive functions (146, 147). Thus, the decidual macrophages do not fit into the conventional M1/M2 classification of macrophages. Indeed, decidual macrophages show dynamic changes throughout pregnancy (13). For instance, seminal plasma-induced M1 macrophage infiltration contributes to embryo implantation in mice (5) and early placentation (5, 67, 148). As pregnancy proceeds, the M2-dominated microenvironment protects the fetus from rejection. At the time of parturition, M1 macrophage accumulation facilitates uterine contraction (149). The driving forces underlying the phenotype changes remain unclear, yet it is generally believed that the surrounding micro-environment is essential for macrophage transformation and maturation.
Tissue-resident macrophages arise from three subsets of precursors: early yolk sac macrophages, fetal liver monocytes and bone marrow-derived monocytes (150, 151). In other words, the tissue-resident macrophages can be generated by self-renewable macrophages or replenished from circulating monocytes. However, the origin of human decidual macrophages remains uncertain. Kammerer et al. reported a unique CD209+CD14+CD68+ HLA-DR+ CD83- proliferating APCs in the decidual of early human pregnancy, suggesting that the human decidual macrophages maintain themselves through self-renewal (152). On the other hand, a gene knockout mice study indicates that the decidual macrophages are replenished by peripheral monocytes expressing circulating lymphocyte antigen 6 complex (Ly6C)hi via a Chemokine (C-C Motif) Ligand 2 (CCL2) - CC chemokine receptor-like 2 (CCR2) dependent pathway driven by CSF-1 (153).
Effect of pEXO on Circulating Monocytes and Decidual Macrophages
Early pregnancy is in a pro-inflammatory state. Monocytes in the maternal blood are progressively activated in pregnant women compared to non-pregnant women (144). Placenta-derived EVs can transform the phagocytic classical monocytes (CD14++CD16-) to the intermediate monocytes (CD14+CD16+) (143) with enhanced migratory capacity and secretion of pro-inflammatory factors such as IL-1β, IL-6, serpinE1, granulocyte-macrophage colony-stimulating factor (GM-CSF), M-CSF and TNF-α (Supplementary Table 1) (154, 155). On the other hand, the number of CD14+HLA-DRlow monocytes is elevated in the maternal blood of the first trimester of pregnancy, and displays an immunosuppressive phenotype when compared with non-pregnant controls (99). Downregulation of HLA-DR endows monocytes with a tolerogenic ability (156). Similarly, tumor-derived exosomes contribute to a systemic immune tolerance via modulating the monocyte phenotype. Exosomes from chronic lymphocytic leukemia induce a high expression of PD-L1 in monocytes in a Toll-like receptors 7 (TLR7)-dependent manner (157). Head and neck squamous cell carcinoma-derived exosomes promote monocytes differentiation into an M2 macrophage-like phenotype via activation of miR-21 (158).
Studies of pEXO on decidual macrophage are sparse. On the other hand, Nguyen et al. demonstrated that pEXO from pregnant mice are specifically targeted to the lungs and liver, and are taken up by lung interstitial macrophages (97). However, the physiological implications of this observation are unclear. Interestingly, tumor-derived exosomes play a critical role in modulating the differentiation of tumor-associated macrophages (TAMs) via exosomal miRNAs, proteins and metabolites (26, 159–161). Similarly, exosomes from the trophoblastic cell line (Swan 71) induce monocyte recruitment and differentiation (Supplementary table 1) (155). Another study found that exosome-carrying fibronectin stimulates the production of IL-1β from macrophages (Supplementary table 1) (162). Of note, pEXO contain molecules known to promote the induction of decidual macrophages. For example, programmed death-ligand 1 (PD-L1), a factor mainly released by trophoblast in early pregnancy, is identified in trophoblast-derived exosomes, and trophoblast-derived soluble PD-L1 promotes decidual macrophages polarization (163–165). Taken together, pEXO favor pregnancy maintenance by inducing monocyte activation, differentiation and decidual macrophage polarization.
T Cells
Decidual and Peripheral Blood T Cells
T cells are the main cell types responsible for immune surveillance, pathogen recognition and elimination. CD3+ T cells constitute ~10% of the decidual lymphocytes in the first trimester. Among them, the CD4+ and the CD8+ T cells are the two largest groups of T cells accounting for 30-45% and 45-75% of the population respectively (3, 70). During pregnancy, these T cells are immunologically tolerant to the fetus and remain in a constrained cytotoxic phenotype (166). Compared to the circulating CD8+ cells, the decidual CD8+ T cells are unable to differentiate into the CD8+ effector cells as validated by low production of perforin and granzyme B. Moreover, the decidual CD8+ T cells show exhausted T cell phenotype with high expression of PD-1, lymphocyte-activation gene 3 (LAG3), cytotoxic T-lymphocyte-associated protein 4 (CTLA4) and T cell immunoglobulin and mucin domain 3 (Tim3) (167). Recent studies further reveal that the CD8+ cells are expandable in the decidua with upregulated expression of cell activation markers such as CD25, CD38, CD69 and HLA-DR, as well as enhanced expression of IFN-γ and IL-17A. These partially activated decidual CD8+ T cells may be associated with trophoblast invasion and spiral artery remodeling after endothelial monolayer destabilization (126, 168).
Other than the CD8+ T cells, CD4+ T helper cells (Th) are critical in modulating the immune tolerance to fetal antigens as well. The Th1/Th2 paradigm has been demonstrated to be essential for a successful pregnancy. Furthermore, recent reports have shown that a Th17/Treg balance is well maintained during pregnancy. The number of regulatory Treg cells in both the human decidua and circulation is increased during pregnancy (169–171). Decreased level of CD25+Foxp3+ Treg is associated with spontaneous abortion (172), preeclampsia (173), and spontaneous preterm birth (174). Furthermore, acute Treg depletion after conception causes embryo resorption along with maternal systemic inflammation and poor endothelial function (92).
Th17 cells are a subset of CD4+ T cells presenting a pro-inflammatory phenotype. Although accounting for only ~2% of CD4+ T cells, elevated frequency of Th17 cells is related to spontaneous abortion and chorioamnionitis (85, 175–177). Interestingly, the study of Wu et al., showed that Th17 cell numbers in both peripheral blood and decidua are elevated in the first trimester of pregnancy and IL17 could promote trophoblast migration and invasion (82). An inverse relationship of Treg cells and Th17 cells are observed in a wide range of pregnancy complications (81, 85). Thus, the new Th1/Th2/Th17/Treg paradigm indicates that T cell homeostasis is an indispensable factor in pregnancy.
Effect of pEXO on T Cells
The roles of pEXO in T cell response have been widely documented. Recent progress suggests that the pEXO mediate immunosuppression via transfer of exosomal proteins to the T cells, leading to T cell apoptosis, inhibition of T cell proliferation, induction of Treg differentiation and reduction of T cell cytotoxicity.
T Cell Apoptosis
It has long been known that T cell apoptosis in human decidua is a characteristic of early pregnancy. Fas ligand/receptor triggered apoptosis is instrumental in the establishment of immune privilege of the fetus and safeguards its development. pEXO with surface Fas ligand and TNF-related apoptosis-inducing ligand (TRAIL) can induce apoptosis in the Jurkat T cells and activate peripheral blood mononuclear cells (PBMCs) in a dose-dependent manner in vitro (42). Moreover, pEXO from maternal blood inhibit T cell activation by down-regulation of CD3 ζ and JAK3, with a more notable effect on CD8+ T cells than on CD4+ T cells (Supplementary Table 1) (178).
Treg Differentiation
The role of exosomes in the differentiation of Treg cells has been implicated in tumor immunology (179–181). Tumor-derived exosomes inhibit T cell proliferation, cytotoxic activities and macrophage polarization (179, 182, 183). Exosomes isolated from the normal placenta via perfusion also inhibit lymphocyte proliferation and induce Treg/memory T cells differentiation (Supplementary Table 1) (184–186). Placental mesenchymal stromal cells (PMSC)-derived exosomes alleviate tubulointerstitial fibrosis by increasing infiltration of the Foxp3+/IL17+ cells in kidneys of the unilateral ureteral obstruction animal model, indicating the involvement of PMSC-exosomes in Treg differentiation (187). Together, these findings indicate that the pEXO are one of the modulators in Treg differentiation during pregnancy.
Cytotoxicity Activity of T Cell
NKG2D ligands such as MHC class I chain-related (MIC) and UL-16 binding protein (ULBP) are expressed on pEXO. Interestingly, the levels of the soluble forms of the MIC protein A and B are negatively correlated with the survival time of cancer patients. The soluble MIC supports tumor escape via binding to NKG2D and downregulating its expression on cytotoxic T cells and NK cells. Similarly, pEXO carrying MIC and ULBP down-regulates the expression of NKG2D receptor on CD8+ T cells and cytotoxic activities of the CD8+ and gamma delta T (γς T) cells (Supplementary Table 1) (134). The expression of syncytin-2, an endogenous retroviral protein exclusively expressed on the human placenta, on the pEXO is down-regulated in preeclampsia patients. Lokossou et al. reported that the pEXO bearing syncytin-2 are immunosuppressive via reducing Th1 cytokine production in activated PBMCs (Supplementary Table 1) (188). Together, these findings indicate that exosomes contribute to immune tolerance through the presentation of MHC molecules or other surface ligands.
Exosomes in Pregnancy Complications
Pregnancy-associated complications such as preeclampsia, gestational diabetes mellitus and preterm birth, are the major threats to human reproductive health. Despite advances in technology and understanding of pregnancy, the rates of pregnancy-related morbidity and mortality increased slightly over the last two decades (189). The current preventive and prognostic approaches for these complications are limited. Thus, a comprehensive understanding of pregnancy-related complications is much needed for better diagnosis and treatment.
Peripheral blood represents the most widely used biological sample for clinical diagnosis. Circulating fetal DNA in maternal plasma and serum has been used for non-invasive prenatal diagnosis (190). Alterations in pEXO have been demonstrated in pregnancy complications. Thus, pEXO might be a promising alternative for screening the following disorders in pregnancy.
Preeclampsia
Preeclampsia, characterized by new onset of hypertension and proteinuria, is one of the most severe complications in pregnancy affecting 5% of pregnant women globally (45). Nonetheless, the most effective treatment for preeclampsia is delivery. For decades, its pathogenesis has largely been attributed to 1) compromised trophoblast invasion (191); 2) dysregulated maternal immune tolerance (192) and 3) endothelial dysfunction (193). However, preeclampsia patients often have more than one defect. It is not clear, to what extent, how each of these causes contributed to preeclampsia as a whole. Considering the growing body of evidence that pEXO are key in modulating maternal homeostasis, we hereby summarize these studies to provide new insight on preeclampsia treatment.
pEXO levels in maternal blood of preeclamptic patients are remarkably increased compared to those of normal pregnancy. Moreover, omics data found that the molecular signatures of pEXO are largely different between preeclampsia and normal pregnancy. For example, proteomic analysis of exosomes isolated from maternal plasma by cholera toxin B chain and annexin V binding show that exosomes from preeclamptic patients have a higher expression of serpin peptidase inhibitor (PAI)-1, porphyria cutanea tarda (PCT), S100 calcium-binding protein B (S100b), TGF-β, VEGFR1, natriuretic peptide B (BNP), placental growth factor (PGF) (194, 195). Non-coding RNA-seq of plasma exosomes reveals that miR-486-1-5p and miR-486-2-5p are significant enriched in the preeclampsia group and could be used as potential diagnosis biomarkers (196). More importantly, exosomes from preeclamptic patients elicit preeclamptic symptoms (hypertension and proteinuria) in mice when injected via tail veins (197), indicating their indispensable role in preeclampsia occurrence.
Trophoblast invasion and migration are critical in spiral artery remodeling and placentation. In preeclamptic patients, miR-210 is highly enriched in the plasma exosomes compared with that in normal pregnancy, and this in turn contributes to preeclampsia by inhibition of trophoblast invasion through downregulating the potassium channel modulatory factor 1 (198). In addition to the comprised trophoblast function, the miRNA profile is disrupted in preeclampsia exosomes as well. For instance, high levels of miR-517-5p, miR-518b and miR-520h are associated with late-onset preeclampsia (199). Controversially, another study observed that down-regulation of miR-517-5p, miR-520a-5p and miR-525-5p in patients are related to late-onset of preeclampsia (200). The discrepancy might be due to differences in sample preparation, donor ethnicity and gestational age. Given that pEXO only accounts for a small proportion (15-20%) of the total circulating exosomes and remarkable differences in miRNA profiles between pEXO and total plasma exosomes, the data should be interpreted with caution.
Endothelial function is fundamental in modulating blood pressure. Nitric oxide (NO) mediates vasorelaxation via an endothelium-dependent pathway. While the diminished activity of endothelial nitric oxide synthase (eNOS), a key enzyme for NO production, is observed in endothelial cells after treatment with preeclamptic pEXO (201). Moreover, preeclamptic patients have a higher level of miR-155 in plasma compared to healthy control and further study showed that it can inhibit eNOS expression in human umbilical vein endothelial cells (HUVEC) (55). An in vitro study showed that the macro-EVs from normal pregnancy but not preeclampsia could protect endothelial cells from activation (138). Moreover, an animal study found that human pEXO could relax mesenteric arteries after injection into pregnant mice (202). Another study showed that trophoblast-derived exosomes could promote vascular smooth muscle cell migration (203).
Disrupted maternal immune tolerance is another hallmark of preeclampsia. Syncytin-1/2, which can inhibit T cell activation and proliferation, is reduced in exosomes from preeclamptic patients (204). PD-L1, involved in decidual macrophage polarization and Treg cell differentiation, was found to be remarkably reduced in the placenta and pEXO of preeclamptic patients. Although the proteomic data on pEXO is rare, tissue proteomic results could be an alternative for the exosome study. For example, the expression of neprilysin (NEP), a membrane-bound metalloprotease associated with hypertension, is increased in the preeclamptic placenta at delivery. Interestingly, Manjot et al. recently demonstrated that exosomes from preeclamptic placenta have a higher expression of active NEP when compared to that of in normal placenta (60).
Gestational Diabetes Mellitus
Gestational diabetes mellitus (GDM) is one of the most common metabolic disorders during pregnancy. It affects ~13.2% of the pregnant mothers in developed countries (205). Without treatment, it may lead to preterm birth, fetal death and other pregnancy complications due to poor placentation induced by hyperglycemia. Although GDM is usually preventable and manageable, infants of mothers with GDM are at increased risk for heart disease, obesity or type 2 diabetes (205–207).
GDM patients have a relatively higher level of total exosomes and pEXO level in maternal plasma (46). Moreover, an in vitro study showed that exosomes from GDM patients induced endothelial activation, indicating the importance of pEXO in modulating maternal vascular homeostasis. miRNA compositions in urine-derived exosome and explant culture are different. Exosomes isolated from the urine of GDM patients in the 3rd trimester of gestation have a low level of miR-516-5p, miR-517-3p, miR-518-5p, miR-222-3p and miR-16-5p (208). Those from placental explant culture express another group of miRNAs (miR-125a-3p, miR-99b-5p, miR-197-3p, miR-22-3p and miR-224-5p) (209). Dipeptidyl peptidase IV (DDPIV) modulates glucose hemostasis by cleavage of glucagon-like peptide 1 (GLP-1) and DDPIV inhibitors are used for type 2 diabetes treatment. Manu Vatish et al. found that exosomes isolated from GDM placenta through perfusion had an upregulation of DDPIV by 8-fold (210). Moreover, exosomes from GDM pregnancy remarkably reduce migration and glucose uptake of skeletal muscle cells (209). Similarly, plasma exosomes from GDM patients also induce glucose intolerance, reduce glucose-induced insulin secretion and cause poor insulin responsiveness in mouse model (211).
GDM may arise from metabolic dysregulation of adipose tissue, which is critical in the modulation of insulin sensitivity (212). In general, normal pregnancy is accompanied by increased total adipose mass. Maternal body mass index (BMI) has a strong association with the risk of GDM, indicating excessive adipocytes are a potential stressor for placentation (213). Adiponectin and leptin, mainly produced by the placenta during pregnancy, have a wide range of functions in adipose tissue such as vascularization, adipocyte enlargement and expansion (207). Exosomes from adipose tissue of GDM patients altered placental glucose metabolism by increasing gene expression of the glycolysis and gluconeogenesis pathways (214). Thus, pEXO may participate in maternal metabolism via modulating the activity of adipose tissue.
Preterm Birth
Preterm birth, also known as premature birth, generally refers to birth at less than 37 weeks of gestational age (215). Nowadays, preterm birth is the leading cause of perinatal morbidity and mortality and has a long-term effect on the health of the fetus (216). For instance, premature infants are vulnerable to heart defects, cognitive disabilities, and respiratory illnesses (217). Nonetheless, the cause of preterm birth is still unclear.
Studies on pEXO of preterm birth are rare. Unlike preeclampsia and GDM, the level of pEXO in preterm birth is significantly decreased compared to full-term pregnancy (218). Placenta senescence and fetal membrane inflammation are generally believed to be the causes of preterm birth. Proteomic study of exosomes from preterm plasma indicates that alterations in protein composition are associated with inflammatory and metabolic signals (219). A similar result was found in amniotic fluid-derived exosomes of preterm patients (220). In animals, Plasma exosomes of CD-1 mice from late-gestation (E18), not early-gestation (E9), induce preterm labor in mice, indicating that exosomes might function as one trigger in labor initiation (221). Moreover, the exosomal miRNA profile of maternal plasma is different between mothers delivering at term and preterm (222, 223). A comprehensive analysis of the exosomal miRNAs reveals that the miRNAs target genes are associated with TGF-β signaling, p53, and glucocorticoid receptor signaling (222). Despite the inconsistency and irreproducibility of miRNA sequencing results, exosomal miRNAs are still suggested as an alternative approach for the diagnosis of preterm birth. Together, these studies indicate that pEXO participate in the processes of labor and delivery.
Discussion
Studies of exosome had been tremendously increased in the last two decades and exosomes are gradually demonstrated to be a perfect tool for drug delivery. However, our understanding of exosome biogenesis and the underlying forces that navigate them to their destination is still lacking.
Currently, most studies on pEXO are conducted in vitro due to ethical constraints in regard to manipulation of the maternal-feto-placental unit and lack of proper animal models. pEXO isolated from placenta tissue at mid (Abortion)- or term (Delivery)-gestation, may not represent it’s in vivo functions at early pregnancy. Therefore, the content and biological activities of pEXO at different gestation periods should be investigated. Furthermore, the alternation of pEXO signatures observed from late gestational samples in clinical studies would possibly be the consequence rather than the cause of the pregnancy complications. A large prospective study of the first trimester pEXO isolated from plasma/placenta tissue from pregnant women who develop pregnancy complications at late gestation should be carried out. Apart from that, in vitro manipulations (Such as perfusion and explant culture) during exosome isolation may disrupt the molecular signature of pEXO. Thus, pEXO isolated by different isolation methods should be compared in order to establish a standard isolation technique and to set a standard parameter for diagnostic purposes.
In summary, pregnancy is a complex physiological process with a wide range of systemic adaptations in the mother’s body. The placenta, the frontline of the maternal-fetal interface, makes these happened in a coordinated way. Exosome, as a signal carrier, links the mother and the fetus and is a key player in immune cell activation, differentiation, maturation and endovascular homeostasis (Figure 3). Thus, advances in pEXO research will deepen our understanding of pregnancy and may provide new insight on the prevention and treatment of pregnancy-related complications.
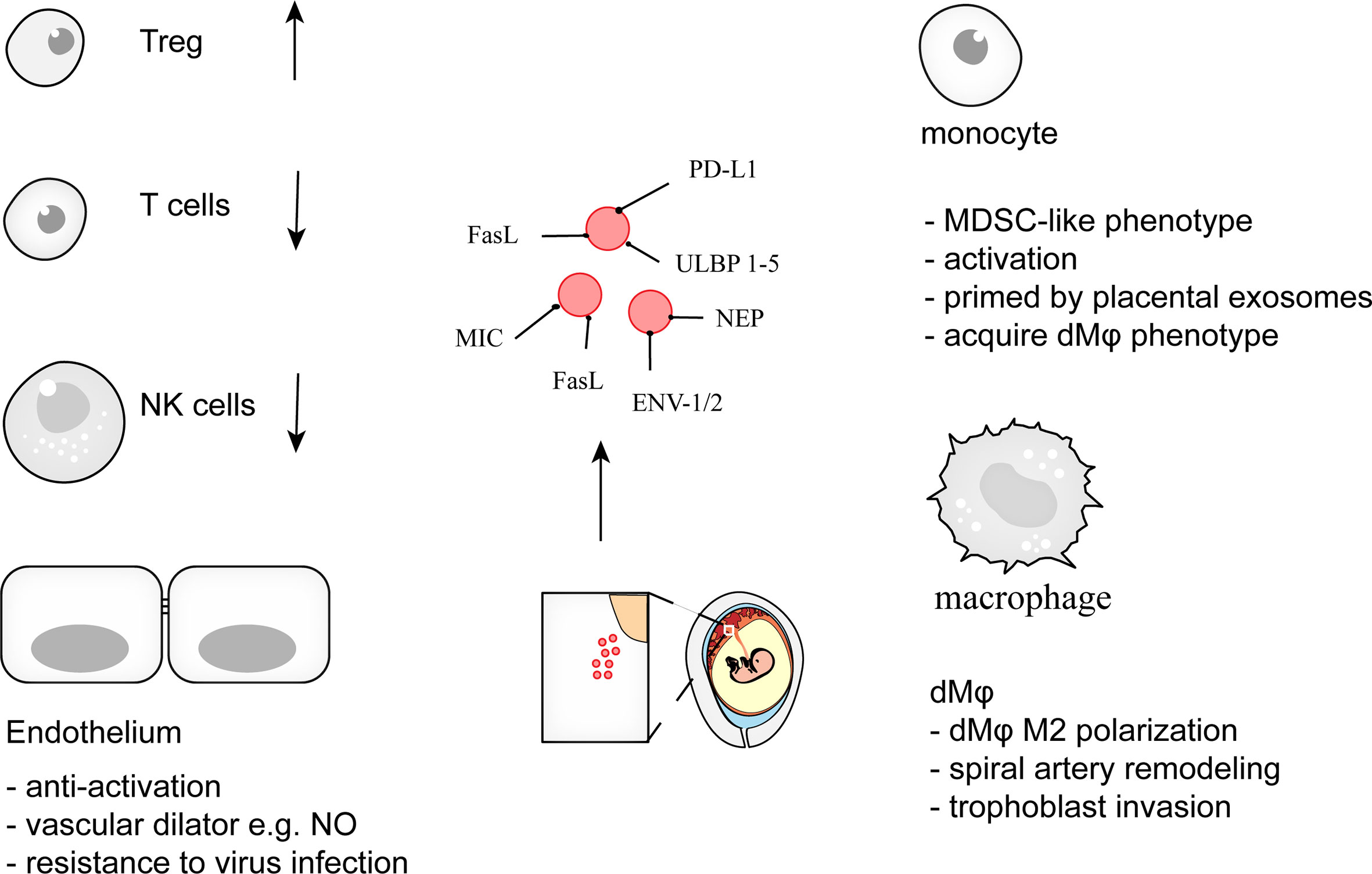
Figure 3 pEXO contribute to maternal tolerance toward the fetus during pregnancy. Exosomes from placenta, syncytiotrophoblast (STB) in particular, support pregnancy via induction of Treg differentiation, restraint of cytotoxic activities of T cells and NK cells, promotion of decidual macrophage polarization, and endowing endothelial cells with viral resistance. Disruption of maternal immune tolerance is associated with adverse pregnancy complications such as miscarriage, preeclampsia. The specific cargoes within the pEXO represent the potential target for prenatal diagnosis and pregnancy-related disease screening.
Author Contributions
Conceptualization – PC, C-LL and KB. Writing – original draft preparation, KB. Writing – review and editing, XL, JZ, C-LL, PC, EN and WY. Illustration – XL and KB. Supervision – C-LL and PC. Funding acquisition – C-LL and EN. All authors contributed to the article and approved the submitted version.
Funding
This work was supported in part by the Hong Kong Research Grant Council Grant (17115619 and 17118917), National Natural Science Foundation of China (81971396) and Sanming Project of Medicine in Shenzhen.
Conflict of Interest
The authors declare that the research was conducted in the absence of any commercial or financial relationships that could be construed as a potential conflict of interest.
Supplementary Material
The Supplementary Material for this article can be found online at: https://www.frontiersin.org/articles/10.3389/fimmu.2021.671093/full#supplementary-material
References
1. Ander SE, Diamond MS, Coyne CB. Immune Responses At the Maternal-Fetal Interface. Sci Immunol (2019) 4:eaat6114. doi: 10.1126/sciimmunol.aat6114
2. Deshmukh H, Way SS. Immunological Basis for Recurrent Fetal Loss and Pregnancy Complications. Annu Rev Pathol Mech Dis (2019) 14:185–210. doi: 10.1146/annurev-pathmechdis-012418-012743
3. Yang F, Zheng Q, Jin L. Dynamic Function and Composition Changes of Immune Cells During Normal and Pathological Pregnancy At the Maternal-Fetal Interface. Front Immunol (2019) 10:2317. doi: 10.3389/fimmu.2019.02317
4. Erlebacher A. Immunology of the Maternal-Fetal Interface. Annu Rev Immunol (2013) 31:387–411. doi: 10.1146/annurev-immunol-032712-100003
5. Sharkey DJ, Tremellen KP, Jasper MJ, Gemzell-Danielsson K, Robertson SA. Seminal Fluid Induces Leukocyte Recruitment and Cytokine and Chemokine Mrna Expression in the Human Cervix After Coitus. J Immunol (2012) 188:2445–54. doi: 10.4049/jimmunol.1102736
6. Abu-Raya B, Michalski C, Sadarangani M, Lavoie PM. Maternal Immunological Adaptation During Normal Pregnancy. Front Immunol (2020) 11:575197. doi: 10.3389/fimmu.2020.575197
7. Huhn O, Ivarsson MA, Gardner L, Hollinshead M, Stinchcombe JC, Chen P, et al. Distinctive Phenotypes and Functions of Innate Lymphoid Cells in Human Decidua During Early Pregnancy. Nat Commun (2020) 11:381. doi: 10.1038/s41467-019-14123-z
8. Kühnert M, Strohmeier R, Stegmüller M, Halberstadt E. Changes in Lymphocyte Subsets During Normal Pregnancy. Eur J Obstet Gyn R B (1998) 76:147–51. doi: 10.1016/s0301-2115<(>97<)>00180-2
9. Laird SM, Tuckerman EM, Cork BA, Linjawi S, Blakemore AIF, Li TCA. Review of Immune Cells and Molecules in Women With Recurrent Miscarriage. Hum Reprod Update (2003) 9:163–74. doi: 10.1093/humupd/dmg013
10. Abbas Y, Turco MY, Burton GJ, Moffett A. Investigation of Human Trophoblast Invasion In Vitro. Hum Reprod Update (2020) 26:501–13. doi: 10.1093/humupd/dmaa017
11. Papúchová H, Meissner TB, Li Q, Strominger JL, Tilburgs T. The Dual Role of HLA-C in Tolerance and Immunity At the Maternal-Fetal Interface. Front Immunol (2019) 10:2730. doi: 10.3389/fimmu.2019.02730
12. Apps R, Murphy SP, Fernando R, Gardner L, Ahad T, Moffett A. Human Leucocyte Antigen (Hla) Expression of Primary Trophoblast Cells and Placental Cell Lines, Determined Using Single Antigen Beads to Characterize Allotype Specificities of Anti-HLA Antibodies. Immunology (2009) 127:26–39. doi: 10.1111/j.1365-2567.2008.03019.x
13. Petroff MG. Immune Interactions At the Maternal–Fetal Interface. J Reprod Immunol (2005) 68:1–13. doi: 10.1016/j.jri.2005.08.003
14. Svensson-Arvelund J, Mehta RB, Lindau R, Mirrasekhian E, Rodriguez-Martinez H, Berg G, et al. The Human Fetal Placenta Promotes Tolerance Against the Semiallogeneic Fetus by Inducing Regulatory T Cells and Homeostatic M2 Macrophages. J Immunol (2015) 194:1534–44. doi: 10.4049/jimmunol.1401536
15. Zhang Y-H, He M, Wang Y, Liao A-H. Modulators of the Balance Between M1 and M2 Macrophages During Pregnancy. Front Immunol (2017) 8:120. doi: 10.3389/fimmu.2017.00120
16. Liu J, Hao S, Chen X, Zhao H, Du L, Ren H, et al. Human Placental Trophoblast Cells Contribute to Maternal–Fetal Tolerance Through Expressing IL-35 and Mediating Itr35 Conversion. Nat Commun (2019) 10:4601. doi: 10.1038/s41467-019-12484-z
17. Mitchell MD, Peiris HN, Kobayashi M, Koh YQ, Duncombe G, Illanes SE, et al. Placental Exosomes in Normal and Complicated Pregnancy. Am J Obstet Gynecol (2015) 213:S173–81. doi: 10.1016/j.ajog.2015.07.001
18. Tannetta D, Masliukaite I, Vatish M, Redman C, Sargent I. Update of Syncytiotrophoblast Derived Extracellular Vesicles in Normal Pregnancy and Preeclampsia. J Reprod Immunol (2017) 119:98–106. doi: 10.1016/j.jri.2016.08.008
19. Knight M, Redman CWG, Linton EA, Sargent IL. Shedding of Syncytiotrophoblast Microvilli Into the Maternal Circulation in Pre-Eclamptic Pregnancies. Bjog Int J Obstetrics Gynaecol (1998) 105:632–40. doi: 10.1111/j.1471-0528.1998.tb10178.x
20. Kalluri R, LeBleu VS. The Biology, Function, and Biomedical Applications of Exosomes. Science (2020) 367:eaau6977. doi: 10.1126/science.aau6977
21. Gandham S, Su X, Wood J, Nocera AL, Alli SC, Milane L, et al. Technologies and Standardization in Research on Extracellular Vesicles. Trends Biotechnol (2020) 38:1066–98. doi: 10.1016/j.tibtech.2020.05.012
22. Shao H, Im H, Castro CM, Breakefield X, Weissleder R, Lee H. New Technologies for Analysis of Extracellular Vesicles. Chem Rev (2018) 118:1917–50. doi: 10.1021/acs.chemrev.7b00534
23. Wolf P. The Nature and Significance of Platelet Products in Human Plasma. Brit J Haematol (1967) 13:269–88. doi: 10.1111/j.1365-2141.1967.tb08741.x
24. Pan B-T, Johnstone RM. Fate of the Transferrin Receptor During Maturation of Sheep Reticulocytes in Vitro: Selective Externalization of the Receptor. Cell (1983) 33:967–78. doi: 10.1016/0092-8674<(>83<)>90040-5
25. Familari M, Cronqvist T, Masoumi Z, Hansson SR. Placenta-Derived Extracellular Vesicles: Their Cargo and Possible Functions. Reprod Fertil Dev (2015) 29:433. doi: 10.1071/rd15143
26. Frank A-C, Ebersberger S, Fink AF, Lampe S, Weigert A, Schmid T, et al. Apoptotic Tumor Cell-Derived Microrna-375 Uses CD36 to Alter the Tumor-Associated Macrophage Phenotype. Nat Commun (2019) 10:1135. doi: 10.1038/s41467-019-08989-2
27. Hoshino A, Kim HS, Bojmar L, Gyan KE, Cioffi M, Hernandez J, et al. Extracellular Vesicle and Particle Biomarkers Define Multiple Human Cancers. Cell (2020) 182:1044–1061.e18. doi: 10.1016/j.cell.2020.07.009
28. Koppers-Lalic D, Hackenberg M, Bijnsdorp IV, van Eijndhoven MAJ, Sadek P, Sie D, et al. Nontemplated Nucleotide Additions Distinguish the Small Rna Composition in Cells From Exosomes. Cell Rep (2014) 8:1649–58. doi: 10.1016/j.celrep.2014.08.027
29. Salomon C, Guanzon D, Scholz-Romero K, Longo S, Correa P, Illanes SE, et al. Placental Exosomes as Early Biomarker of Preeclampsia: Potential Role of Exosomal Micrornas Across Gestation. J Clin Endocrinol Metab (2017) 102:3182–94. doi: 10.1210/jc.2017-00672
30. Devor E, Santillan D, Scroggins S, Warrier A, Santillan M. Trimester-Specific Plasma Exosome Microrna Expression Profiles in Preeclampsia. J Maternal-fetal Neonatal Med (2019) 33:1–9. doi: 10.1080/14767058.2019.1569614
31. Coleman SJ, Gerza L, Jones CJP, Sibley CP, Aplin JD, Heazell AEP. Syncytial Nuclear Aggregates in Normal Placenta Show Increased Nuclear Condensation, But Apoptosis and Cytoskeletal Redistribution are Uncommon. Placenta (2013) 34:449–55. doi: 10.1016/j.placenta.2013.02.007
32. Jones CJ, Fox H. Syncytial Knots and Intervillous Bridges in the Human Placenta: An Ultrastructural Study. J Anat (1977) 124:275–86.
33. Fogarty NME, Ferguson-Smith AC, Burton GJ. Syncytial Knots (Tenney-Parker Changes) in the Human Placenta Evidence of Loss of Transcriptional Activity and Oxidative Damage. Am J Pathol (2013) 183:144–52. doi: 10.1016/j.ajpath.2013.03.016
34. Chua S, Wilkins T, Sargent I, Redman C. Trophoblast Deportation in Pre-Eclamptic Pregnancy. Bjog Int J Obstetrics Gynaecol (1991) 98:973–9. doi: 10.1111/j.1471-0528.1991.tb15334.x
35. Holland O, Kroneis T, El-Heliebi A, McDowell-Hook M, Stone P, Sedlmayr P, et al. Detection of Fetal Sex, Aneuploidy and a Microdeletion From Single Placental Syncytial Nuclear Aggregates. Fetal Diagn Ther (2017) 41:32–40. doi: 10.1159/000445112
36. Calvert SJ, Jones CJP, Sibley CP, Aplin JD, Heazell AEP. Analysis of Syncytial Nuclear Aggregates in Preeclampsia Shows Increased Sectioning Artefacts and Decreased Inter-Villous Bridges Compared to Healthy Placentas. Placenta (2013) 34:1251–4. doi: 10.1016/j.placenta.2013.10.001
37. Nair S, Salomon C. Extracellular Vesicles as Critical Mediators of Maternal-Fetal Communication During Pregnancy and Their Potential Role in Maternal Metabolism. Placenta (2020) 98:60–8. doi: 10.1016/j.placenta.2020.06.011
38. Messerli M, May K, Hansson SR, Schneider H, Holzgreve W, Hahn S, et al. Feto-Maternal Interactions in Pregnancies: Placental Microparticles Activate Peripheral Blood Monocytes. Placenta (2009) 31:106–12. doi: 10.1016/j.placenta.2009.11.011
39. Baig S, Kothandaraman N, Manikandan J, Rong L, EE KH, Hill J, et al. Proteomic Analysis of Human Placental Syncytiotrophoblast Microvesicles in Preeclampsia. Clin Proteom (2014) 11:40. doi: 10.1186/1559-0275-11-40
40. Delorme-Axford E, Donker RB, Mouillet J-F, Chu T, Bayer A, Ouyang Y, et al. Human Placental Trophoblasts Confer Viral Resistance to Recipient Cells. Proc Natl Acad Sci (2013) 110:12048–53. doi: 10.1073/pnas.1304718110
41. Mincheva-Nilsson L, Nagaeva O, Chen T, Stendahl U, Antsiferova J, Mogren I, et al. Placenta-Derived Soluble Mhc Class I Chain-Related Molecules Down-Regulate NKG2D Receptor on Peripheral Blood Mononuclear Cells During Human Pregnancy: A Possible Novel Immune Escape Mechanism for Fetal Survival. J Immunol (2006) 176:3585–92. doi: 10.4049/jimmunol.176.6.3585
42. Stenqvist A-C, Nagaeva O, Baranov V, Mincheva-Nilsson L. Exosomes Secreted by Human Placenta Carry Functional Fas Ligand and TRAIL Molecules and Convey Apoptosis in Activated Immune Cells, Suggesting Exosome-Mediated Immune Privilege of the Fetus. J Immunol (2013) 191:5515–23. doi: 10.4049/jimmunol.1301885
43. Atay S, Gercel-Taylor C, Suttles J, Mor G, Taylor DD. Trophoblast-Derived Exosomes Mediate Monocyte Recruitment and Differentiation. Am J Reprod Immunol (2010) 65:65–77. doi: 10.1111/j.1600-0897.2010.00880.x
44. Sarker S, Scholz-Romero K, Perez A, Illanes SE, Mitchell MD, Rice GE, et al. Placenta-Derived Exosomes Continuously Increase in Maternal Circulation Over the First Trimester of Pregnancy. J Transl Med (2014) 12:204. doi: 10.1186/1479-5876-12-204
45. Phipps EA, Thadhani R, Benzing T, Karumanchi SA. Pre-Eclampsia: Pathogenesis, Novel Diagnostics and Therapies. Nat Rev Nephrol (2019) 15:275–89. doi: 10.1038/s41581-019-0119-6
46. Salomon C, Scholz-Romero K, Sarker S, Sweeney E, Kobayashi M, Correa P, et al. Gestational Diabetes Mellitus is Associated With Changes in the Concentration and Bioactivity of Placenta-Derived Exosomes in Maternal Circulation Across Gestation. Diabetes (2015) 65:598–609. doi: 10.2337/db15-0966
47. Lal CV, Olave N, Travers C, Rezonzew G, Dolma K, Simpson A, et al. Exosomal Microrna Predicts and Protects Against Severe Bronchopulmonary Dysplasia in Extremely Premature Infants. JCI Insight (2018) 3:e93994. doi: 10.1172/jci.insight.93994
48. Gupta AK, Rusterholz C, Huppertz B, Malek A, Schneider H, Holzgreve W, et al. Comparative Study of the Effect of Three Different Syncytiotrophoblast Micro-Particles Preparations on Endothelial Cells. Placenta (2005) 26:59–66. doi: 10.1016/j.placenta.2004.04.004
49. Gupta AK, Holzgreve W, Hahn S. Decrease in Lipid Levels of Syncytiotrophoblast Micro-Particles Reduced Their Potential to Inhibit Endothelial Cell Proliferation. Arch Gynecol Obstet (2008) 277:115–9. doi: 10.1007/s00404-007-0425-2
50. Gupta AK, Rusterholz C, Holzgreve W, Hahn S. Syncytiotrophoblast Micro-Particles do Not Induce Apoptosis in Peripheral T Lymphocytes, But Differ in Their Activity Depending on the Mode of Preparation. J Reprod Immunol (2005) 68:15–26. doi: 10.1016/j.jri.2005.05.003
51. Xia Y, Zhang Q, Zhen Q, Zhao Y, Liu N, Li T, et al. Negatively Regulation of Tumor-Infiltrating Nk Cell in Clear Cell Renal Cell Carcinoma Patients Through the Exosomal Pathway. Oncotarget (2014) 5:37783–95. doi: 10.18632/oncotarget.16354
52. Hsu Y-L, Hung J-Y, Chang W-A, Lin Y-S, Pan Y-C, Tsai P-H, et al. Hypoxic Lung Cancer-Secreted Exosomal Mir-23a Increased Angiogenesis and Vascular Permeability by Targeting Prolyl Hydroxylase and Tight Junction Protein Zo-1. Oncogene (2017) 36:4929–42. doi: 10.1038/onc.2017.105
53. Gangoda L, Liem M, Ang C, Keerthikumar S, Adda CG, Parker BS, et al. Proteomic Profiling of Exosomes Secreted by Breast Cancer Cells With Varying Metastatic Potential. Proteomics (2017) 17:1600370. doi: 10.1002/pmic.201600370
54. Takahashi H, Ohkuchi A, Kuwata T, Usui R, Baba Y, Suzuki H, et al. Endogenous and Exogenous Mir-520c-3p Modulates Cd44-Mediated Extravillous Trophoblast Invasion. Placenta (2017) 50:25–31. doi: 10.1016/j.placenta.2016.12.016
55. Shen L, Li Y, Li R, Diao Z, Yany M, Wu M, et al. Placenta-Associated Serum Exosomal Mir-155 Derived From Patients With Preeclampsia Inhibits Enos Expression in Human Umbilical Vein Endothelial Cells. Int J Mol Med (2018) 41:1731–9. doi: 10.3892/ijmm.2018.3367
56. Jung HH, Kim J-Y, Lim JE, Im Y-H. Cytokine Profiling in Serum-Derived Exosomes Isolated by Different Methods. Sci Rep-uk (2020) 10:14069. doi: 10.1038/s41598-020-70584-z
57. Coumans FAW, Brisson AR, Buzas EI, Dignat-George F, Drees EEE, El-Andaloussi S, et al. Methodological Guidelines to Study Extracellular Vesicles. Circ Res (2017) 120:1632–48. doi: 10.1161/circresaha.117.309417
58. Greening DW, Xu R, Ji H, Tauro BJ, Simpson RJ. Proteomic Profiling, Methods and Protocols. Methods Mol Biol (2015) 1295:179–209. doi: 10.1007/978-1-4939-2550-6_15
59. Witwer KW, Buzás EI, Bemis LT, Bora A, Lässer C, Lötvall J, et al. Standardization of Sample Collection, Isolation and Analysis Methods in Extracellular Vesicle Research. J Extracell Vesicles (2013) 2:20360. doi: 10.3402/jev.v2i0.20360
60. Gill M, Motta-Mejia C, Kandzija N, Cooke W, Zhang W, Cerdeira AS, et al. Placental Syncytiotrophoblast-Derived Extracellular Vesicles Carry Active NEP (Neprilysin) and are Increased in Preeclampsia. Hypertension (2019) 73:1112–9. doi: 10.1161/hypertensionaha.119.12707
61. Niu Z, Pang RTK, Liu W, Li Q, Cheng R, Yeung WSB. Polymer-Based Precipitation Preserves Biological Activities of Extracellular Vesicles From an Endometrial Cell Line. PloS One (2017) 12:e0186534. doi: 10.1371/journal.pone.0186534
62. Peng Q, Zhang J, Zhou G. Comparison of Plasma Exosomes by Differential Ultracentrifugation and Solvent Precipitation Methods. Clin Lab (2018) 64:991–8. doi: 10.7754/clin.lab.2018.180104
63. Guan S, Yu H, Yan G, Gao M, Sun W, Zhang X. Characterization of Urinary Exosomes Purified With Size Exclusion Chromatography and Ultracentrifugation. J Proteome Res (2020) 19:2217–25. doi: 10.1021/acs.jproteome.9b00693
64. Diaz G, Bridges C, Lucas M, Cheng Y, Schorey JS, Dobos KM, et al. Protein Digestion, Ultrafiltration, and Size Exclusion Chromatography to Optimize the Isolation of Exosomes From Human Blood Plasma and Serum. J Vis Exp (2018) 134:e57467. doi: 10.3791/57467
65. Tay C-S, Tagliani E, Collins MK, Erlebacher A. Cis-Acting Pathways Selectively Enforce the Non-Immunogenicity of Shed Placental Antigen for Maternal Cd8 T Cells. PloS One (2013) 8:e84064. doi: 10.1371/journal.pone.0084064
66. Erlebacher A, Vencato D, Price KA, Zhang D, Glimcher LH. Constraints in Antigen Presentation Severely Restrict T Cell Recognition of the Allogeneic Fetus. J Clin Invest (2007) 117:1399–411. doi: 10.1172/jci28214
67. Moldenhauer LM, Diener KR, Thring DM, Brown MP, Hayball JD, Robertson SA. Cross-Presentation of Male Seminal Fluid Antigens Elicits T Cell Activation to Initiate the Female Immune Response to Pregnancy. J Immunol (2009) 182:8080–93. doi: 10.4049/jimmunol.0804018
68. Jasti S, Farahbakhsh M, Nguyen S, Petroff BK, Petroff MG. Immune Response to a Model Shared Placenta/Tumor-Associated Antigen Reduces Cancer Risk in Parous Mice. Biol Reprod (2017) 96:134–44. doi: 10.1095/biolreprod.116.144907
69. Alam SMK, Jasti S, Kshirsagar SK, Tannetta DS, Dragovic RA, Redman CW, et al. Trophoblast Glycoprotein (TPGB/5T4) in Human Placenta: Expression, Regulation, and Presence in Extracellular Microvesicles and Exosomes. Reprod Sci (2018) 25:185–97. doi: 10.1177/1933719117707053
70. Suryawanshi H, Morozov P, Straus A, Sahasrabudhe N, Max KEA, Garzia A, et al. Single-Cell Survey of the Human First-Trimester Placenta and Decidua. Sci Adv (2018) 4:eaau4788. doi: 10.1126/sciadv.aau4788
71. Strunz B, Bister J, Jönsson H, Filipovic I, Crona-Guterstam Y, Kvedaraite E, et al. Continuous Human Uterine NK Cell Differentiation in Response to Endometrial Regeneration and Pregnancy. Sci Immunol (2021) 6:eabb7800. doi: 10.1126/sciimmunol.abb7800
72. Male V, Sharkey A, Masters L, Kennedy PR, Farrell LE, Moffett A. The Effect of Pregnancy on the Uterine Nk Cell Kir Repertoire. Eur J Immunol (2011) 41:3017–27. doi: 10.1002/eji.201141445
73. Kelly RW, Critchley HOD. a T-Helper-2 Bias in Decidua: The Prostaglandin Contribution of the Macrophage and Trophoblast. J Reprod Immunol (1997) 33:181–7. doi: 10.1016/s0165-0378<(>97<)>00021-1
74. Terzieva A, Dimitrova V, Djerov L, Dimitrova P, Zapryanova S, Hristova I, et al. Early Pregnancy Human Decidua is Enriched With Activated, Fully Differentiated and Pro-Inflammatory Gamma/Delta T Cells With Diverse TCR Repertoires. Int J Mol Sci (2019) 20:687. doi: 10.3390/ijms20030687
75. Miyazaki S, Tsuda H, Sakai M, Hori S, Sasaki Y, Futatani T, et al. Predominance of Th2-Promoting Dendritic Cells in Early Human Pregnancy Decidua. J Leukocyte Biol (2003) 74:514–22. doi: 10.1189/jlb.1102566
76. Lédée N, Petitbarat M, Chevrier L, Vitoux D, Vezmar K, Rahmati M, et al. The Uterine Immune Profile may Help Women With Repeated Unexplained Embryo Implantation Failure After in Vitro Fertilization. Am J Reprod Immunol (2016) 75:388–401. doi: 10.1111/aji.12483
77. Ng SC, Gilman-Sachs A, Thaker P, Beaman KD, Beer AE, Kwak-Kim J. Expression of Intracellular Th1 and Th2 Cytokines in Women With Recurrent Spontaneous Abortion, Implantation Failures After IVF/ET or Normal Pregnancy. Am J Reprod Immunol (2002) 48:77–86. doi: 10.1034/j.1600-0897.2002.01105.x
78. Dong M, He J, Wang Z, Xie X, Wang H. Placental Imbalance of Th1- and Th2-Type Cytokines in Preeclampsia. Acta Obstet Gyn Scan (2005) 84:788–93. doi: 10.1111/j.0001-6349.2005.00714.x
79. Lager S, Sovio U, Eddershaw E, Linden MW, Yazar C, Cook E, et al. Abnormal Placental Cd8+ T-Cell Infiltration is a Feature of Fetal Growth Restriction and Pre-Eclampsia. J Physiol (2020) 598:5555–71. doi: 10.1113/jp279532
80. Zhang Y, Ma L, Hu X, Ji J, Mor G, Liao A. The Role of the PD-1/PD-L1 Axis in Macrophage Differentiation and Function During Pregnancy. Hum Reprod (2018) 34:25–36. doi: 10.1093/humrep/dey347
81. Wang W-J, Hao C-F, Yi-Lin, Yin G-J, Bao S-H, Qiu L-H, et al. Increased Prevalence of T Helper 17 (Th17) Cells in Peripheral Blood and Decidua in Unexplained Recurrent Spontaneous Abortion Patients. J Reprod Immunol (2010) 84:164–70. doi: 10.1016/j.jri.2009.12.003
82. Wu H-X, Jin L-P, Xu B, Liang S-S, Li D-J. Decidual Stromal Cells Recruit Th17 Cells Into Decidua to Promote Proliferation and Invasion of Human Trophoblast Cells by Secreting Il-17. Cell Mol Immunol (2014) 11:253–62. doi: 10.1038/cmi.2013.67
83. Chaouat G. The Th1/Th2 Paradigm: Still Important in Pregnancy? Semin Immunopathol (2007) 29:95–113. doi: 10.1007/s00281-007-0069-0
84. Wang W, Sung N, Gilman-Sachs A, Kwak-Kim JT. Helper (Th) Cell Profiles in Pregnancy and Recurrent Pregnancy Losses: Th1/Th2/Th9/Th17/Th22/Tfh Cells. Front Immunol (2020) 11:2025. doi: 10.3389/fimmu.2020.02025
85. Saito S, Nakashima A, Shima T, Ito M. Review Article: Th1/Th2/Th17 and Regulatory T-Cell Paradigm in Pregnancy. Am J Reprod Immunol (2010) 63:601–10. doi: 10.1111/j.1600-0897.2010.00852.x
86. Hetherington CM, Hegan MA. Breeding Nude (Nu/Nu) Mice. Lab Anim (1975) 9:19–20. doi: 10.1258/002367775780994907
87. Croy BA, Chapeau C. Evaluation of the Pregnancy Immunotrophism Hypothesis by Assessment of the Reproductive Performance of Young Adult Mice of Genotype Scid/Scid.Bg/Bg. Reproduction (1990) 88:231–9. doi: 10.1530/jrf.0.0880231
88. Kumagai K, Kubota N, Saito TI, Sasako T, Takizawa R, Sudo K, et al. Generation of Transgenic Mice on an NOD/SCID Background Using the Conventional Microinjection Technique1. Biol Reprod (2011) 84:682–8. doi: 10.1095/biolreprod.110.087106
89. Lin Y, Chen Y, Zeng Y, Wang T, Zeng S. Lymphocyte Phenotyping and NK Cell Activity Analysis in Pregnant Nod/Scid Mice. J Reprod Immunol (2005) 68:39–51. doi: 10.1016/j.jri.2005.05.002
90. Mombaerts P, Iacomini J, Johnson RS, Herrup K, Tonegawa S, Papaioannou VE. Rag-1-Deficient Mice Have No Mature B and T Lymphocytes. Cell (1992) 68:869–77. doi: 10.1016/0092-8674<(>92<)>90030-g
91. Gorantla S, Sneller H, Walters L, Sharp JG, Pirruccello SJ, West JT, et al. Human Immunodeficiency Virus Type 1 Pathobiology Studied in Humanized Balb/C-Rag2–/–Γc–/– Mice▿. J Virol (2006) 81:2700–12. doi: 10.1128/jvi.02010-06
92. Care AS, Bourque SL, Morton JS, Hjartarson EP, Robertson SA, Davidge ST. Reduction in Regulatory T Cells in Early Pregnancy Causes Uterine Artery Dysfunction in Mice. Hypertension (2018) 72:177–87. doi: 10.1161/hypertensionaha.118.10858
93. Pollard JW, Hunt JS, Wiktor-Jedrzejczak W, Stanley ERA. Pregnancy Defect in the Osteopetrotic (Opop) Mouse Demonstrates the Requirement for CSF-1 in Female Fertility. Dev Biol (1991) 148:273–83. doi: 10.1016/0012-1606<(>91<)>90336-2
94. Wiktor-Jedrzejczak W, Bartocci A, Ferrante AW, Ahmed-Ansari A, Sell KW, Pollard JW, et al. Total Absence of Colony-Stimulating Factor 1 in the Macrophage-Deficient Osteopetrotic (Op/Op) Mouse. Proc Natl Acad Sci (1990) 87:4828–32. doi: 10.1073/pnas.87.12.4828
95. Care AS, Diener KR, Jasper MJ, Brown HM, Ingman WV, Robertson SA. Macrophages Regulate Corpus Luteum Development During Embryo Implantation in Mice. J Clin Invest (2013) 123:3472–87. doi: 10.1172/jci60561
96. Ren J, Zeng W, Tian F, Zhang S, Wu F, Qin X, et al. Myeloid-Derived Suppressor Cells Depletion may Cause Pregnancy Loss Via Upregulating the Cytotoxicity of Decidual Natural Killer Cells. Am J Reprod Immunol (2019) 81:e13099. doi: 10.1111/aji.13099
97. Gerosa M, Schioppo T, Meroni PL. Challenges and Treatment Options for Rheumatoid Arthritis During Pregnancy. Expert Opin Pharmaco (2016) 17:1–9. doi: 10.1080/14656566.2016.1197204
98. Pan T, Zhong L, Wu S, Cao Y, Yang Q, Cai Z, et al. 17β-Oestradiol Enhances the Expansion and Activation of Myeloid-Derived Suppressor Cells Via Signal Transducer and Activator of Transcription (Stat)-3 Signalling in Human Pregnancy. Clin Exp Immunol (2016) 185:86–97. doi: 10.1111/cei.12790
99. Zhang Y, Qu D, Sun J, Zhao L, Wang Q, Shao Q, et al. Human Trophoblast Cells Induced Mdscs From Peripheral Blood Cd14(+) Myelomonocytic Cells Via Elevated Levels of CCL2. Cell Mol Immunol (2016) 13:615–27. doi: 10.1038/cmi.2015.41
100. Bartmann C, Junker M, Segerer SE, Häusler SF, Krockenberger M, Kämmerer U. Cd33(+) /Hla-DR(Neg) and CD33(+) /Hla-Dr(+/-) Cells: Rare Populations in the Human Decidua With Characteristics of MDSC. Am J Reprod Immunol (2016) 75:539–56. doi: 10.1111/aji.12492
101. Watanabe M, Iwatani Y, Kaneda T, Hidaka Y, Mitsuda N, Morimoto Y, et al. Changes in T, B, and NK Lymphocyte Subsets During and After Normal Pregnancy. Am J Reprod Immunol (1997) 37:368–77. doi: 10.1111/j.1600-0897.1997.tb00246.x
102. Molvarec A, Blois SM, Stenczer B, Toldi G, Tirado-Gonzalez I, Ito M, et al. Peripheral Blood Galectin-1-Expressing T and Natural Killer Cells in Normal Pregnancy and Preeclampsia. Clin Immunol (2011) 139:48–56. doi: 10.1016/j.clim.2010.12.018
103. Higuma-Myojo S, Sasaki Y, Miyazaki S, Sakai M, Siozaki A, Miwa N, et al. Cytokine Profile of Natural Killer Cells in Early Human Pregnancy. Am J Reprod Immunol (2005) 54:21–9. doi: 10.1111/j.1600-0897.2005.00279.x
104. Mori M, Bogdan A, Balassa T, Csabai T, Szekeres-Bartho J. The Decidua—the Maternal Bed Embracing the Embryo—Maintains the Pregnancy. Semin Immunopathol (2016) 38:635–49. doi: 10.1007/s00281-016-0574-0
105. Garrido-Gomez T, Dominguez F, Quiñonero A, Diaz-Gimeno P, Kapidzic M, Gormley M, et al. Defective Decidualization During and After Severe Preeclampsia Reveals a Possible Maternal Contribution to the Etiology. Proc Natl Acad Sci (2017) 114:E8468–77. doi: 10.1073/pnas.1706546114
106. Woods L, Perez-Garcia V, Kieckbusch J, Wang X, DeMayo F, Colucci F, et al. Decidualisation and Placentation Defects are a Major Cause of Age-Related Reproductive Decline. Nat Commun (2017) 8:352. doi: 10.1038/s41467-017-00308-x
107. Vilella F, Moreno-Moya JM, Balaguer N, Grasso A, Herrero M, Martínez S, et al. Hsa-Mir-30d, Secreted by the Human Endometrium, is Taken Up by the Pre-Implantation Embryo and Might Modify Its Transcriptome. Development (2015) 142:3210–21. doi: 10.1242/dev.124289
108. Evans J, Rai A, Nguyen HPT, Poh QH, Elglass K, Simpson RJ, et al. Human Endometrial Extracellular Vesicles Functionally Prepare Human Trophectoderm Model for Implantation: Understanding Bidirectional Maternal-Embryo Communication. Proteomics (2019) 19:1800423. doi: 10.1002/pmic.201800423
109. Liu WM, Cheng RR, Niu ZR, Chen AC, Ma MY, Li T, et al. Let-7 Derived From Endometrial Extracellular Vesicles is an Important Inducer of Embryonic Diapause in Mice. Sci Adv (2020) 6:eaaz7070. doi: 10.1126/sciadv.aaz7070
110. Saadeldin IM, Kim SJ, Choi YB, Lee BC. Improvement of Cloned Embryos Development by Co-Culturing With Parthenotes: A Possible Role of Exosomes/Microvesicles for Embryos Paracrine Communication. Cell Reprogramming Former Cloning Stem Cells (2014) 16:223–34. doi: 10.1089/cell.2014.0003
111. Burns GW, Brooks KE, Spencer TE. Extracellular Vesicles Originate From the Conceptus and Uterus During Early Pregnancy in Sheep1. Biol Reprod (2016) 94(Article 56):1–11. doi: 10.1095/biolreprod.115.134973
112. Pallinger E, Bognar Z, Bogdan A, Csabai T, Abraham H, Szekeres-Bartho J. Pibf+ Extracellular Vesicles From Mouse Embryos Affect IL-10 Production by CD8+ Cells. Sci Rep-uk (2018) 8:4662. doi: 10.1038/s41598-018-23112-z
113. Faust ZS, Laškarin G, Rukavina D. Progesterone-Induced Blocking Factor Inhibits Degranulation of Natural Killer Cells. Am J Reprod Immunol (1999) 42:71–5. doi: 10.1111/j.1600-0897.1999.tb00468.x
114. Ferreira LMR, Meissner TB, Tilburgs T, Strominger JL. Hla-G: At the Interface of Maternal–Fetal Tolerance. Trends Immunol (2017) 38:272–86. doi: 10.1016/j.it.2017.01.009
115. Li Y, Zhang J, Zhang D, Hong X, Tao Y, Wang S, et al. Tim-3 Signaling in Peripheral Nk Cells Promotes Maternal-Fetal Immune Tolerance and Alleviates Pregnancy Loss. Sci Signal (2017) 10:eaah4323. doi: 10.1126/scisignal.aah4323
116. Borzychowski AM, Croy BA, Chan WL, Redman CWG, Sargent IL. Changes in Systemic Type 1 and Type 2 Immunity in Normal Pregnancy and Pre-Eclampsia may Be Mediated by Natural Killer Cells. Eur J Immunol (2005) 35:3054–63. doi: 10.1002/eji.200425929
117. Fukui A, Funamizu A, Yokota M, Yamada K, Nakamua R, Fukuhara R, et al. Uterine and Circulating Natural Killer Cells and Their Roles in Women With Recurrent Pregnancy Loss, Implantation Failure and Preeclampsia. J Reprod Immunol (2011) 90:105–10. doi: 10.1016/j.jri.2011.04.006
118. Koopman LA, Kopcow HD, Rybalov B, Boyson JE, Orange JS, Schatz F, et al. Human Decidual Natural Killer Cells are a Unique Nk Cell Subset With Immunomodulatory Potential. J Exp Med (2003) 198:1201–12. doi: 10.1084/jem.20030305
119. Manaster I, Mizrahi S, Goldman-Wohl D, Sela HY, Stern-Ginossar N, Lankry D, et al. Endometrial Nk Cells are Special Immature Cells That Await Pregnancy. J Immunol (2008) 181:1869–76. doi: 10.4049/jimmunol.181.3.1869
120. Kopcow HD, Eriksson M, Mselle TF, Damrauer SM, Wira CR, Sentman CL, et al. Human Decidual Nk Cells From Gravid Uteri and NK Cells From Cycling Endometrium are Distinct Nk Cell Subsets. Placenta (2010) 31:334–8. doi: 10.1016/j.placenta.2010.01.003
121. Jabrane-Ferrat N. Features of Human Decidual NK Cells in Healthy Pregnancy and During Viral Infection. Front Immunol (2019) 10:1397. doi: 10.3389/fimmu.2019.01397
122. Vivier E, Tomasello E, Baratin M, Walzer T, Ugolini S. Functions of Natural Killer Cells. Nat Immunol (2008) 9:503–10. doi: 10.1038/ni1582
123. Chao K, Yang Y, Ho G, Chen S, Chen H, Dai H, et al. Decidual Natural Killer Cytotoxicity Decreased in Normal Pregnancy But Not in Anembryonic Pregnancy and Recurrent Spontaneous Abortion. Am J Reprod Immunol (1995) 34:274–80. doi: 10.1111/j.1600-0897.1995.tb00953.x
124. Zhang Y, Zhao A, Wang X, Shi G, Jin H, Lin Q. Expressions of Natural Cytotoxicity Receptors and NKG2D on Decidual Natural Killer Cells in Patients Having Spontaneous Abortions. Fertil Steril (2008) 90:1931–7. doi: 10.1016/j.fertnstert.2007.08.009
125. Yougbaré I, Tai W-S, Zdravic D, Oswald BE, Lang S, Zhu G, et al. Activated NK Cells Cause Placental Dysfunction and Miscarriages in Fetal Alloimmune Thrombocytopenia. Nat Commun (2017) 8:224. doi: 10.1038/s41467-017-00269-1
126. Vento-Tormo R, Efremova M, Botting RA, Turco MY, Vento-Tormo M, Meyer KB, et al. Single-Cell Reconstruction of the Early Maternal–Fetal Interface in Humans. Nature (2018) 563:347–53. doi: 10.1038/s41586-018-0698-6
127. Keskin DB, Allan DSJ, Rybalov B, Andzelm MM, Stern JNH, Kopcow HD, et al. Tgfβ Promotes Conversion of CD16+ Peripheral Blood Nk Cells Into CD16– Nk Cells With Similarities to Decidual Nk Cells. Proc Natl Acad Sci (2007) 104:3378–83. doi: 10.1073/pnas.0611098104
128. PrabhuDas M, Bonney E, Caron K, Dey S, Erlebacher A, Fazleabas A, et al. Immune Mechanisms At the Maternal-Fetal Interface: Perspectives and Challenges. Nat Immunol (2015) 16:328–34. doi: 10.1038/ni.3131
129. Hanna J, Goldman-Wohl D, Hamani Y, Avraham I, Greenfield C, Natanson-Yaron S, et al. Decidual NK Cells Regulate Key Developmental Processes At the Human Fetal-Maternal Interface. Nat Med (2006) 12:1065–74. doi: 10.1038/nm1452
130. Gamliel M, Goldman-Wohl D, Isaacson B, Gur C, Stein N, Yamin R, et al. Trained Memory of Human Uterine NK Cells Enhances Their Function in Subsequent Pregnancies. Immunity (2018) 48:951–962.e5. doi: 10.1016/j.immuni.2018.03.030
131. Crespo Â.C., Mulik S, Dotiwala F, Ansara JA, Santara SS, Ingersoll K, et al. Decidual NK Cells Transfer Granulysin to Selectively Kill Bacteria in Trophoblasts. Cell (2020) 182:1125–1139.e18. doi: 10.1016/j.cell.2020.07.019
132. Ishida Y, Zhao D, Ohkuchi A, Kuwata T, Yoshitake H, Yuge K, et al. Maternal Peripheral Blood Natural Killer Cells Incorporate Placenta-Associated Micrornas During Pregnancy. Int J Mol Med (2015) 35:1511–24. doi: 10.3892/ijmm.2015.2157
133. Nardi F, Da S, Michelon TF, Neumann J, Manvailer LFS, Wagner B, et al. High Levels of Circulating Extracellular Vesicles With Altered Expression and Function During Pregnancy. Immunobiology (2016) 221:753–60. doi: 10.1016/j.imbio.2016.03.001
134. Hedlund M, Stenqvist A-C, Nagaeva O, Kjellberg L, Wulff M, Baranov V, et al. Human Placenta Expresses and Secretes Nkg2d Ligands Via Exosomes That Down-Modulate the Cognate Receptor Expression: Evidence for Immunosuppressive Function. J Immunol (2009) 183:340–51. doi: 10.4049/jimmunol.0803477
135. Brandt CS, Baratin M, Yi EC, Kennedy J, Gao Z, Fox B, et al. The B7 Family Member B7-H6 is a Tumor Cell Ligand for the Activating Natural Killer Cell Receptor Nkp30 in Humans. J Exp Med (2009) 206:1495–503. doi: 10.1084/jem.20090681
136. Zhou Y, Xu Y, Chen L, Xu B, Wu C, Jiang J. B7-H6 Expression Correlates With Cancer Progression and Patient’s Survival in Human Ovarian Cancer. Int J Clin Exp Patho (2015) 8:9428–33.
137. Gutierrez-Franco J, Hernandez-Gutierrez R, Bueno-Topete MR, Haramati J, Navarro-Hernandez RE, Escarra-Senmarti M, et al. Characterization of B7H6, an Endogenous Ligand for the NK Cell Activating Receptor Nkp30, Reveals the Identity of Two Different Soluble Isoforms During Normal Human Pregnancy. Immunobiology (2018) 223:57–63. doi: 10.1016/j.imbio.2017.10.012
138. Tong M, Chen Q, James JL, Stone PR, Chamley LW. Micro- and Nano-Vesicles From First Trimester Human Placentae Carry Flt-1 and Levels are Increased in Severe Preeclampsia. Front Endocrinol (2017) 8:174. doi: 10.3389/fendo.2017.00174
139. Lee C-L, Vijayan M, Wang X, Lam KKW, Koistinen H, Seppala M, et al. Glycodelin-a Stimulates the Conversion of Human Peripheral Blood Cd16–Cd56bright NK Cell to a Decidual Nk Cell-Like Phenotype. Hum Reprod (2018) 34:689–701. doi: 10.1093/humrep/dey378
140. Uhlen M, Karlsson MJ, Zhong W, Tebani A, Pou C, Mikes J, et al. A Genome-Wide Transcriptomic Analysis of Protein-Coding Genes in Human Blood Cells. Science (2019) 366:eaax9198. doi: 10.1126/science.aax9198
141. Sampath P, Moideen K, Ranganathan UD, Bethunaickan R. Monocyte Subsets: Phenotypes and Function in Tuberculosis Infection. Front Immunol (2018) 9:1726. doi: 10.3389/fimmu.2018.01726
142. Michlmayr D, Andrade P, Gonzalez K, Balmaseda A, Harris E. Cd14+Cd16+ Monocytes are the Main Target of Zika Virus Infection in Peripheral Blood Mononuclear Cells in a Paediatric Study in Nicaragua. Nat Microbiol (2017) 2:1462–70. doi: 10.1038/s41564-017-0035-0
143. Al-ofi E, Coffelt SB, Anumba DO. Monocyte Subpopulations From Pre-Eclamptic Patients are Abnormally Skewed and Exhibit Exaggerated Responses to Toll-Like Receptor Ligands. PloS One (2012) 7:e42217. doi: 10.1371/journal.pone.0042217
144. Luppi P, Haluszczak C, Betters D, Richard CAH, Trucco M, DeLoia JA. Monocytes are Progressively Activated in the Circulation of Pregnant Women. J Leukocyte Biol (2002) 72:874–84. doi: 10.1189/jlb.72.5.874
145. Sun F, Wang S, Du M. Functional Regulation of Decidual Macrophages During Pregnancy. J Reprod Immunol (2020) 143:103264. doi: 10.1016/j.jri.2020.103264
146. Gustafsson C, Mjösberg J, Matussek A, Geffers R, Matthiesen L, Berg G, et al. Gene Expression Profiling of Human Decidual Macrophages: Evidence for Immunosuppressive Phenotype. PloS One (2008) 3:e2078. doi: 10.1371/journal.pone.0002078
147. Svensson J, Jenmalm MC, Matussek A, Geffers R, Berg G, Ernerudh J. Macrophages At the Fetal–Maternal Interface Express Markers of Alternative Activation and are Induced by M-CSF and IL-10. J Immunol (2011) 187:3671–82. doi: 10.4049/jimmunol.1100130
148. Paktinat S, Hashemi SM, Novin MG, Mohammadi-Yeganeh S, Salehpour S, Karamian A, et al. Seminal Exosomes Induce Interleukin-6 and Interleukin-8 Secretion by Human Endometrial Stromal Cells. Eur J Obstet Gyn R B (2019) 235:71–6. doi: 10.1016/j.ejogrb.2019.02.010
149. Hamilton S, Oomomian Y, Stephen G, Shynlova O, Tower CL, Garrod A, et al. Macrophages Infiltrate the Human and Rat Decidua During Term and Preterm Labor: Evidence That Decidual Inflammation Precedes Labor1. Biol Reprod (2012) 86(Article 39):1–9. doi: 10.1095/biolreprod.111.095505
150. Bian Z, Gong Y, Huang T, Lee CZW, Bian L, Bai Z, et al. Deciphering Human Macrophage Development At Single-Cell Resolution. Nature (2020) 582:571–6. doi: 10.1038/s41586-020-2316-7
151. Blériot C, Chakarov S, Ginhoux F. Determinants of Resident Tissue Macrophage Identity and Function. Immunity (2020) 52:957–70. doi: 10.1016/j.immuni.2020.05.014
152. Kämmerer U, Eggert AO, Kapp M, McLellan AD, Geijtenbeek TBH, Dietl J, et al. Unique Appearance of Proliferating Antigen-Presenting Cells Expressing DC-SIGN (CD209) in the Decidua of Early Human Pregnancy. Am J Pathol (2003) 162:887–96. doi: 10.1016/s0002-9440<(>10<)>63884-9
153. Tagliani E, Shi C, Nancy P, Tay C-S, Pamer EG, Erlebacher A. Coordinate Regulation of Tissue Macrophage and Dendritic Cell Population Dynamics by CSF-1. J Exp Med (2011) 208:1901–16. doi: 10.1084/jem.20110866
154. Al-ofi E, Coffelt SB, Anumba DO. Fibrinogen, an Endogenous Ligand of Toll-Like Receptor 4, Activates Monocytes in Pre-Eclamptic Patients. J Reprod Immunol (2014) 103:23–8. doi: 10.1016/j.jri.2014.02.004
155. Aldo PB, Racicot K, Craviero V, Guller S, Romero R, Mor G. Trophoblast Induces Monocyte Differentiation Into Cd14+/Cd16+ Macrophages. Am J Reprod Immunol (2014) 72:270–84. doi: 10.1111/aji.12288
156. Huber V, Vallacchi V, Fleming V, Hu X, Cova A, Dugo M, et al. Tumor-Derived Micrornas Induce Myeloid Suppressor Cells and Predict Immunotherapy Resistance in Melanoma. J Clin Invest (2018) 128:5505–16. doi: 10.1172/jci98060
157. Haderk F, Schulz R, Iskar M, Cid LL, Worst T, Willmund KV, et al. Tumor-Derived Exosomes Modulate PD-L1 Expression in Monocytes. Sci Immunol (2017) 2:eaah5509. doi: 10.1126/sciimmunol.aah5509
158. Hsieh C-H, Tai S-K, Yang M-H. Snail-Overexpressing Cancer Cells Promote M2-Like Polarization of Tumor-Associated Macrophages by Delivering Mir-21-Abundant Exosomes. Neoplasia (2018) 20:775–88. doi: 10.1016/j.neo.2018.06.004
159. Takano Y, Masuda T, Iinuma H, Yamaguchi R, Sato K, Tobo T, et al. Circulating Exosomal Microrna-203 is Associated With Metastasis Possibly Via Inducing Tumor-Associated Macrophages in Colorectal Cancer. Oncotarget (2017) 8:78598–613. doi: 10.18632/oncotarget.20009
160. Mantovani A, Sozzani S, Locati M, Allavena P, Sica A. Macrophage Polarization: Tumor-Associated Macrophages as a Paradigm for Polarized M2 Mononuclear Phagocytes. Trends Immunol (2002) 23:549–55. doi: 10.1016/s1471-4906<(>02<)>02302-5
161. Sahraei M, Chaube B, Liu Y, Sun J, Kaplan A, Price NL, et al. Suppressing Mir-21 Activity in Tumor-Associated Macrophages Promotes an Antitumor Immune Response. J Clin Invest (2019) 129(12):5518–36. doi: 10.1172/jci127125
162. Atay S, Gercel-Taylor C, Taylor DD. Human Trophoblast-Derived Exosomal Fibronectin Induces Pro-Inflammatory Il-1β Production by Macrophages. Am J Reprod Immunol (2011) 66:259–69. doi: 10.1111/j.1600-0897.2011.00995.x
163. Petroff MG, Chen L, Phillips TA, Azzola D, Sedlmayr P, Hunt JS. B7 Family Molecules are Favorably Positioned At the Human Maternal-Fetal Interface1. Biol Reprod (2003) 68:1496–504. doi: 10.1095/biolreprod.102.010058
164. Enninga EAL, Harrington SM, Creedon DJ, Ruano R, Markovic SN, Dong H, et al. Immune Checkpoint Molecules Soluble Program Death Ligand 1 and Galectin-9 are Increased in Pregnancy. Am J Reprod Immunol (2018) 79:e12795. doi: 10.1111/aji.12795
165. Kshirsagar SK, Alam SM, Jasti S, Hodes H, Nauser T, Gilliam M, et al. Immunomodulatory Molecules are Released From the First Trimester and Term Placenta Via Exosomes. Placenta (2012) 33:982–90. doi: 10.1016/j.placenta.2012.10.005
166. Iwatani Y, Amino N, Tachi J, Kimura M, Ura I, Mori M, et al. Changes of Lymphocyte Subsets in Normal Pregnant and Postpartum Women: Postpartum Increase of NK/K (Leu 7) Cells. Am J Reprod Im Mic (1988) 18:52–5. doi: 10.1111/j.1600-0897.1988.tb00235.x
167. Kinder JM, Turner LH, Stelzer IA, Miller-Handley H, Burg A, Shao T-Y, et al. Cd8+ T Cell Functional Exhaustion Overrides Pregnancy-Induced Fetal Antigen Alloimmunization. Cell Rep (2020) 31:107784. doi: 10.1016/j.celrep.2020.107784
168. Zeng W, Liu X, Liu Z, Zheng Y, Yu T, Fu S, et al. Deep Surveying of the Transcriptional and Alternative Splicing Signatures for Decidual Cd8+ T Cells At the First Trimester of Human Healthy Pregnancy. Front Immunol (2018) 9:937. doi: 10.3389/fimmu.2018.00937
169. Wienke J, Brouwers L, Burg LM, Mokry M, Scholman RC, Nikkels PGJ, et al. Human Tregs at the Maternal-Fetal Interface Show Site-Specific Adaptation Reminiscent of Tumor Tregs. Jci Insight (2020) 5(18):e137926. doi: 10.1172/jci.insight.137926
170. Kahn DA, Baltimore D. Pregnancy Induces a Fetal Antigen-Specific Maternal T Regulatory Cell Response That Contributes to Tolerance. Proc Natl Acad Sci (2010) 107:9299–304. doi: 10.1073/pnas.1003909107
171. Salvany-Celades M, Van Der Zwan A, Benner M, Setrajcic-Dragos V, Gomes HAB, Iyer V, et al. Three Types of Functional Regulatory T Cells Control T Cell Responses At the Human Maternal-Fetal Interface. Cell Rep (2019) 27:2537–2547.e5. doi: 10.1016/j.celrep.2019.04.109
172. Woidacki K, Meyer N, Schumacher A, Goldschmidt A, Maurer M, Zenclussen AC. Transfer of Regulatory T Cells Into Abortion-Prone Mice Promotes the Expansion of Uterine Mast Cells and Normalizes Early Pregnancy Angiogenesis. Sci Rep-uk (2015) 5:13938. doi: 10.1038/srep13938
173. Hsu P, Santner-Nanan B, Dahlstrom JE, Fadia M, Chandra A, Peek M, et al. Altered Decidual Dc-Sign+ Antigen-Presenting Cells and Impaired Regulatory T-Cell Induction in Preeclampsia. Am J Pathol (2012) 181:2149–60. doi: 10.1016/j.ajpath.2012.08.032
174. Schjenken JE, Moldenhauer LM, Zhang B, Care AS, Groome HM, Chan H-Y, et al. Microrna Mir-155 is Required for Expansion of Regulatory T Cells to Mediate Robust Pregnancy Tolerance in Mice. Mucosal Immunol (2020) 13:609–25. doi: 10.1038/s41385-020-0255-0
175. Ito M, Nakashima A, Hidaka T, Okabe M, Bac ND, Ina S, et al. A Role for IL-17 in Induction of an Inflammation At the Fetomaternal Interface in Preterm Labour. J Reprod Immunol (2010) 84:75–85. doi: 10.1016/j.jri.2009.09.005
176. Nakashima A, Ito M, Shima T, Bac ND, Hidaka T, Saito S. Accumulation of IL-17-Positive Cells in Decidua of Inevitable Abortion Cases. Am J Reprod Immunol (2010) 64:4–11. doi: 10.1111/j.1600-0897.2010.00812.x
177. Mjsberg J, Berg G, Jenmalm MC, Ernerudh J. Foxp3 Regulatory T Cells and T Helper 1, T Helper 2, and T Helper 17 Cells in Human Early Pregnancy Decidua1. Biol Reprod (2010) 82:698–705. doi: 10.1095/biolreprod.109.081208
178. Sabapatha A, Gercel-Taylor C, Taylor DD. Specific Isolation of Placenta-Derived Exosomes From the Circulation of Pregnant Women and Their Immunoregulatory Consequences1. Am J Reprod Immunol (2006) 56:345–55. doi: 10.1111/j.1600-0897.2006.00435.x
179. Mikami N, Kawakami R, Chen KY, Sugimoto A, Ohkura N, Sakaguchi S. Epigenetic Conversion of Conventional T Cells Into Regulatory T Cells by CD28 Signal Deprivation. Proc Natl Acad Sci (2020) 117:12258–68. doi: 10.1073/pnas.1922600117
180. Liu Y, Gu Y, Cao X. The Exosomes in Tumor Immunity. Oncoimmunology (2015) 4:e1027472. doi: 10.1080/2162402x.2015.1027472
181. Diskin B, Adam S, Cassini MF, Sanchez G, Liria M, Aykut B, et al. Pd-L1 Engagement on T Cells Promotes Self-Tolerance and Suppression of Neighboring Macrophages and Effector T Cells in Cancer. Nat Immunol (2020) 21:442–54. doi: 10.1038/s41590-020-0620-x
182. Yu Y-R, Imrichova H, Wang H, Chao T, Xiao Z, Gao M, et al. Disturbed Mitochondrial Dynamics in CD8+ Tils Reinforce T Cell Exhaustion. Nat Immunol (2020) 21:1540–51. doi: 10.1038/s41590-020-0793-3
183. Wang X, Xiang Z, Tsao GS-W, Tu W. Exosomes Derived From Nasopharyngeal Carcinoma Cells Induce IL-6 Production From Macrophages to Promote Tumorigenesis. Cell Mol Immunol (2020) 18:501–3. doi: 10.1038/s41423-020-0420-0
184. Khalfoun B, Degenne D, Crouzat-Reynes G, Bardos P. Effect of Human Syncytiotrophoblast Plasma Membrane-Soluble Extracts on in Vitro Mitogen-Induced Lymphocyte Proliferation. A Possible Inhibition Mechanism Involving the Transferrin Receptor. J Immunol Baltim Md 1950 (1986) 137:1187–93.
185. Degenne D, Khalfoun B, Bardos P. In Vitro Inhibitory Effect of Human Syncytiotrophoblast Plasma Membranes on the Cytolytic Activities of CTL and NK Cells. Am J Reprod Im Mic (1986) 12:106–10. doi: 10.1111/j.1600-0897.1986.tb00074.x
186. Thibault G, Degenne D, Girard AC, Guillaumin JM, Lacord M, Bardos P. The Inhibitory Effect of Human Syncytiotrophoblast Plasma Membrane Vesicles on in Vitro Lymphocyte Proliferation is Associated With Reduced Interleukin 2 Receptor Expression. Cell Immunol (1991) 138:165–74. doi: 10.1016/0008-8749(91)90141-w
187. Zhu Z, Han C, Xian S, Zhuang F, Ding F, Zhang W, et al. Placental Mesenchymal Stromal Cells (Pmscs) and PMSC-Derived Extracellular Vesicles (Pmsc-Evs) Attenuated Renal Fibrosis in Rats With Unilateral Ureteral Obstruction (UUO) by Regulating Cd4+ T Cell Polarization. Stem Cells Int (2020) 2020:1–12. doi: 10.1155/2020/2685820
188. Lokossou AG, Toudic C, Nguyen PT, Elisseeff X, Vargas A, Rassart R, et al. Endogenous Retrovirus-Encoded Syncytin-2 Contributes to Exosome-Mediated Immunosuppression of T Cells. Biol Reprod (2019) 102:185–98. doi: 10.1093/biolre/ioz124
189. Burgess APH, Dongarwar D, Spigel Z, Salihu H, Moaddab A, Clark S, et al. Pregnancy-Related Mortality in the U.S. 2003-2016 ; Age, Race and Place of Death. Am J Obstet Gynecol (2020) 222:489.e1–8. doi: 10.1016/j.ajog.2020.02.020
190. Lo YMD, Corbetta N, Chamberlain PF, Rai V, Sargent IL, Redman CW, et al. Presence of Fetal DNA in Maternal Plasma and Serum. Lancet (1997) 350:485–7. doi: 10.1016/s0140-6736<(>97<)>02174-0
191. Ridder A, Giorgione V, Khalil A, Thilaganathan B. Preeclampsia: The Relationship Between Uterine Artery Blood Flow and Trophoblast Function. Int J Mol Sci (2019) 20:3263. doi: 10.3390/ijms20133263
192. Laresgoiti-Servitje E, Gómez-López N, Olson DM. An Immunological Insight Into the Origins of Pre-Eclampsia. Hum Reprod Update (2011) 17:291–1. doi: 10.1093/humupd/dmq055
193. Boeldt DS, Bird IM. Vascular Adaptation in Pregnancy and Endothelial Dysfunction in Preeclampsia. J Endocrinol (2017) 232:R27–44. doi: 10.1530/joe-16-0340
194. Tan KH, Tan SS, Ng MJ, Tey WS, Sim WK, Allen JC, et al. Extracellular Vesicles Yield Predictive Pre-Eclampsia Biomarkers. J Extracell Vesicles (2017) 6:1408390. doi: 10.1080/20013078.2017.1408390
195. Tan KH, Tan SS, Sze SK, Lee WKR, Ng MJ, Lim SK. Plasma Biomarker Discovery in Preeclampsia Using a Novel Differential Isolation Technology for Circulating Extracellular Vesicles. Am J Obstet Gynecol (2014) 211:380.e1–380.e13. doi: 10.1016/j.ajog.2014.03.038
196. Li H, Ouyang Y, Sadovsky E, Parks WT, Chu T, Sadovsky Y. Unique Microrna Signals in Plasma Exosomes From Pregnancies Complicated by Preeclampsia. Hypertension (2020) 75:762–71. doi: 10.1161/hypertensionaha.119.14081
197. Han C, Wang C, Chen Y, Wang J, Xu X, Hilton T, et al. Placenta-Derived Extracellular Vesicles Induce Preeclampsia in Mouse Models. Haematologica (2019) 105:1686–94. doi: 10.3324/haematol.2019.226209. haematol.2019.226209.
198. Luo R, Shao X, Xu P, Liu Y, Wang Y, Zhao Y, et al. Microrna-210 Contributes to Preeclampsia by Downregulating Potassium Channel Modulatory Factor 1. Hypertension (2018) 64:839–45. doi: 10.1161/hypertensionaha.114.03530
199. Hromadnikova I, Kotlabova K, Ivankova K, Krofta L. First Trimester Screening of Circulating C19mc Micrornas and the Evaluation of Their Potential to Predict the Onset of Preeclampsia and IUGR. PloS One (2017) 12:e0171756. doi: 10.1371/journal.pone.0171756
200. Hromadnikova I, Dvorakova L, Kotlabova K, Krofta L. The Prediction of Gestational Hypertension, Preeclampsia and Fetal Growth Restriction Via the First Trimester Screening of Plasma Exosomal C19MC Micrornas. Int J Mol Sci (2019) 20:2972. doi: 10.3390/ijms20122972
201. Motta-Mejia C, Kandzija N, Zhang W, Mhlomi V, Cerdeira AS, Burdujan A, et al. Placental Vesicles Carry Active Endothelial Nitric Oxide Synthase and Their Activity is Reduced in Preeclampsia. Hypertension (2017) 70:372–81. doi: 10.1161/hypertensionaha.117.09321
202. Tong M, Stanley JL, Chen Q, James JL, Stone PR, Chamley LW. Placental Nano-Vesicles Target to Specific Organs and Modulate Vascular Tone In Vivo. Hum Reprod (2017) 32:2188–98. doi: 10.1093/humrep/dex310
203. Salomon C, Yee S, Scholz-Romero K, Kobayashi M, Vaswani K, Kvaskoff D, et al. Extravillous Trophoblast Cells-Derived Exosomes Promote Vascular Smooth Muscle Cell Migration. Front Pharmacol (2014) 5:175. doi: 10.3389/fphar.2014.00175
204. Levine L, Habertheuer A, Ram C, Korutla L, Schwartz N, Hu RW, et al. Syncytiotrophoblast Extracellular Microvesicle Profiles in Maternal Circulation for Noninvasive Diagnosis of Preeclampsia. Sci Rep-uk (2020) 10:6398. doi: 10.1038/s41598-020-62193-7
205. Melchior H, Kurch-Bek D, Mund M. The Prevalence of Gestational Diabetes: A Population-Based Analysis of a Nationwide Screening Program. Deutsches Aerzteblatt Online (2017) 114:412–8. doi: 10.3238/arztebl.2017.0412
206. Sáez T, Vos P, De; Sobrevia L, Faas MM. Is There a Role for Exosomes in Foetoplacental Endothelial Dysfunction in Gestational Diabetes Mellitus? Placenta (2018) 61:48–54. doi: 10.1016/j.placenta.2017.11.007
207. Rojas-Rodriguez R, Ziegler R, DeSouza T, Majid S, Madore AS, Amir N, et al. Pappa-Mediated Adipose Tissue Remodeling Mitigates Insulin Resistance and Protects Against Gestational Diabetes in Mice and Humans. Sci Transl Med (2020) 12:eaay4145. doi: 10.1126/scitranslmed.aay4145
208. Oostdam A.S.H.-V., Toro-Ortíz JC, López JA, Noyola DE, García-López DA, Durán-Figueroa NV, et al. Placental Exosomes Isolated From Urine of Patients With Gestational Diabetes Exhibit a Differential Profile Expression of Micrornas Across Gestation. Int J Mol Med (2020) 46:546–60. doi: 10.3892/ijmm.2020.4626
209. Nair S, Jayabalan N, Guanzon D, Palma C, Scholz-Romero K, Elfeky O, et al. Human Placental Exosomes in Gestational Diabetes Mellitus Carry a Specific Set of Mirnas Associated With Skeletal Muscle Insulin Sensitivity. Clin Sci (2018) 132:2451–67. doi: 10.1042/cs20180487
210. Kandzija N, Zhang W, Motta-Mejia C, Mhlomi V, McGowan-Downey J, James T, et al. Placental Extracellular Vesicles Express Active Dipeptidyl Peptidase IV; Levels are Increased in Gestational Diabetes Mellitus. J Extracell Vesicles (2019) 8:1617000. doi: 10.1080/20013078.2019.1617000
211. James-Allan LB, Rosario FJ, Barner K, Lai A, Guanzon D, McIntyre HD, et al. Regulation of Glucose Homeostasis by Small Extracellular Vesicles in Normal Pregnancy and in Gestational Diabetes. FASEB J (2020) 34:5724–39. doi: 10.1096/fj.201902522rr
212. Jayabalan N, Nair S, Nuzhat Z, Rice GE, Zuñiga FA, Sobrevia L, et al. Cross Talk Between Adipose Tissue and Placenta in Obese and Gestational Diabetes Mellitus Pregnancies Via Exosomes. Front Endocrinol (2017) 8:239. doi: 10.3389/fendo.2017.00239
213. Howell KR, Powell TL. Effects of Maternal Obesity on Placental Function and Fetal Development. Reproduction (2017) 153:R97–R108. doi: 10.1530/rep-16-0495
214. Jayabalan N, Lai A, Ormazabal V, Adam S, Guanzon D, Palma C, et al. Adipose Tissue Exosomal Proteomic Profile Reveals a Role on Placenta Glucose Metabolism in Gestational Diabetes Mellitus. J Clin Endocrinol Metab (2018) 104:1735–52. doi: 10.1210/jc.2018-01599
215. Frey HA, Klebanoff MA. The Epidemiology, Etiology, and Costs of Preterm Birth. Semin Fetal Neonatal Med (2016) 21:68–73. doi: 10.1016/j.siny.2015.12.011
216. Luu TM, Mian MOR, Nuyt AM. Long-Term Impact of Preterm Birth Neurodevelopmental and Physical Health Outcomes. Clin Perinatol (2017) 44:305–14. doi: 10.1016/j.clp.2017.01.003
217. Natarajan G, Shankaran S. Short- and Long-Term Outcomes of Moderate and Late Preterm Infants. Am J Perinat (2016) 33:305–17. doi: 10.1055/s-0035-1571150
218. Menon R, Shahin H. Extracellular Vesicles in Spontaneous Preterm Birth. Am J Reprod Immunol (2020) 85:e13353. doi: 10.1111/aji.13353
219. Menon R, Dixon CL, Sheller-Miller S, Fortunato SJ, Saade GR, Palma C, et al. Quantitative Proteomics by SWATH-MS of Maternal Plasma Exosomes Determine Pathways Associated With Term and Preterm Birth. Endocrinology (2019) 160:639–50. doi: 10.1210/en.2018-00820
220. Dixon CL, Sheller-Miller S, Saade GR, Fortunato SJ, Lai A, Palma C, et al. Amniotic Fluid Exosome Proteomic Profile Exhibits Unique Pathways of Term and Preterm Labor. Endocrinology (2018) 159:2229–40. doi: 10.1210/en.2018-00073
221. Sheller-Miller S, Trivedi J, Yellon SM, Menon R. Exosomes Cause Preterm Birth in Mice: Evidence for Paracrine Signaling in Pregnancy. Sci Rep-uk (2019) 9:608. doi: 10.1038/s41598-018-37002-x
222. Menon R, Debnath C, Lai A, Guanzon D, Bhatnagar S, Kshetrapal PK, et al. Circulating Exosomal Mirna Profile During Term and Preterm Birth Pregnancies: A Longitudinal Study. Endocrinology (2019) 160:249–75. doi: 10.1210/en.2018-00836
Keywords: placenta, exosomes, maternal immune tolerance, preeclampsia, gestational diabetes mellitus, preterm
Citation: Bai K, Li X, Zhong J, Ng EHY, Yeung WSB, Lee C-L and Chiu PCN (2021) Placenta-Derived Exosomes as a Modulator in Maternal Immune Tolerance During Pregnancy. Front. Immunol. 12:671093. doi: 10.3389/fimmu.2021.671093
Received: 23 February 2021; Accepted: 26 April 2021;
Published: 11 May 2021.
Edited by:
Paula Barbim Donate, University of São Paulo, BrazilReviewed by:
Margaret Petroff, Michigan State University, United StatesNathalie Ledee, Hôpital Cochin, France
Copyright © 2021 Bai, Li, Zhong, Ng, Yeung, Lee and Chiu. This is an open-access article distributed under the terms of the Creative Commons Attribution License (CC BY). The use, distribution or reproduction in other forums is permitted, provided the original author(s) and the copyright owner(s) are credited and that the original publication in this journal is cited, in accordance with accepted academic practice. No use, distribution or reproduction is permitted which does not comply with these terms.
*Correspondence: Cheuk-Lun Lee, kcllee@hku.hk; Philip C. N. Chiu, pchiucn@hku.hk