- 1Institute of Oceanography and Fisheries, Split, Croatia
- 2P.P. Shirshov Institute of Oceanology, Russian Academy of Sciences, Moscow, Russia
- 3Fisheries and Oceans Canada, Institute of Ocean Sciences, Sidney, BC, Canada
- 4Department of Physics, University of the Balearic Islands, Palma de Mallorca, Spain
The understanding of meteotsunamis—significant atmospherically generated long ocean waves in the tsunami frequency band—has advanced considerably during the last two decades. Scientists and specialists use near-field in situ data and remote observations, as well as atmospheric and ocean modeling, to study destructive events. The phenomenon has been reported and investigated worldwide, indicating its relevance as a marine natural hazard and demonstrating the urgent need for meteotsunami warning systems for certain countries. In this paper we summarize the present knowledge of the phenomenon, identify particular research gaps, and propose near-future critical components of meteotsunami research. We emphasize a potential concept of merging yet-to-be-developed meteotsunami warning systems and existing tsunami or multi-hazard early warning systems.
Introduction
Nomitsu (1935) was first to write about “tsunamis of atmospheric origin” and to describe significant atmospherically induced tsunami-like oscillations observed in certain harbors and bays of the Japanese islands. Defant (1961) indicated that similar oscillations are also observed in some other regions of the world oceans and recommended the general term “meteorological tsunami” or “meteotsunami” for this type of phenomena. Rabinovich and Monserrat (1996, 1998) introduced this term to the tsunami community. The similarity of seismically generated tsunamis and meteotsunamis was obvious; also, it became clear that many “tsunamis of unknown origin” described in tsunami catalogs (e.g., Soloviev and Go, 1974; Lander et al., 1993) are, in fact, meteorological tsunamis. After an overview paper by Monserrat et al. (2006) the term “meteotsunami” became widely used and respective long oceanic waves began to be recognized as other natural hazards.
Meteotsunamis have the same temporal and spatial scales as ordinary tsunami waves and can affect coastal areas in a similar destructive way, but they are generated by traveling atmospheric disturbances, rather than by underwater earthquakes, landslides or volcanic eruptions (Monserrat et al., 2006). A specific property of meteotsunamis is that they are phenomena of resonance: intensive waves can be produced only through resonant transfer of energy from the atmosphere to the ocean via Proudman resonance (Proudman, 1929), U = c, or Greenspan resonance (Greenspan, 1956), U = cj, where U is the speed of atmospheric disturbances, is the long wave speed, cj is the speed of one of the first modes of edge waves, h is the ocean depth and g is the gravitational acceleration. Normally, catastrophic meteotsunamis occur only in particular regions that have extensive shelf areas promoting these types of resonances, with depths ranging from 25 to 150 m, conducive for long wave speeds of 16 to 40 m/s. Specific local topographic features facilitating the substantial amplification of arriving waves are a V-shape for the external embayment opened toward the incoming long ocean waves and narrow-entrance internal bays/harbors with high Q-factor (Miles and Munk, 1961).
In certain harbors and bays of the world oceans, the phenomenon has been known for a long time and is called by local names: “abiki” in Japan, “rissaga” on the Balearic Islands, “šćiga” in the Adriatic Sea, “marrobbio” (“marrubbio”) in Sicily, and “milghuba” in Malta (Monserrat et al., 2006; Rabinovich, 2009). The science of meteotsunamis has developed rapidly in the last two decades, documenting the phenomenon along the coasts of all continents except Antarctica (Figure 1). Much of this work has been consolidated in two special issues: Physics and Chemistry of the Earth (Rabinovich et al., 2009) and Natural Hazards1 (Vilibić et al., 2014).
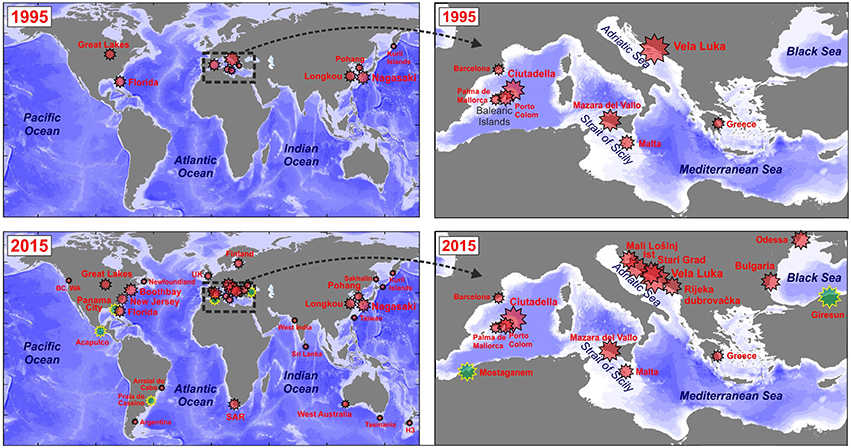
Figure 1. Locations where meteotsunamis had been documented by the year 1995 (upper panels), and by the year 2015 (lower panels). Size of stars is proportional to intensity of the documented events. The information shown in the figure was constructed using the data presented by Rabinovich and Monserrat (1996), Monserrat et al. (2006), Rabinovich (2009), Šepić et al. (2015a), and Pattiaratchi and Wijeratne (2015). Green stars indicate locations where meteotsunami-like events occurred (Acapulco – 2 May 2015; Giresun – 24 September 2014; Mostaganem – 3 August 2007; Panama City – 28 March 2014; and Praia do Cassino – 9 February 2014) but still have not been proven by research studies.
Meteotsunami research concentrates on several issues: (i) what processes and conditions in the atmosphere are responsible for the generation of meteotsunamis; (ii) how is the atmospheric energy transferred to the ocean waves, (iii) what types of resonant properties control the process; (iv) how does bathymetry affect the propagation and amplification of meteotsunami waves; and (v) what architecture and protocols should be used for timely and reliable detection of tsunamigenic atmospheric disturbances and early meteotsunami warning? The last question implies that the knowledge of the phenomenon is appropriate for real-time detection and prediction of meteotsunamis. It is also the ultimate question to be answered to mitigate damage caused by coastal flooding and/or strong currents.
The 1978 Vela Luka meteotsunami (Adriatic Sea, Croatia) caused a loss of $7 M (in 1978 prices), equal to of the annual income of the entire island of Korčula (Vučetić et al., 2009). The 2006 Balearic meteotsunami (“rissaga”) sank or damaged several tens of boats and yachts in Ciutadella Harbor (Menorca Island, Spain) with a total cost of more than $30M euros (Monserrat et al., 2006). The 1954 Great Lakes meteotsunami (Ewing et al., 1954), the 1979 “abiki” in Nagasaki Bay (Hibiya and Kajiura, 1982), and several events observed at the UK coast (Haslett and Bryant, 2009; Tappin et al., 2013; Sibley et al., 2016) resulted in human casualties and severe destruction. Two recent examples came from Odessa (Ukraine, the northwestern Black Sea) and Fremantle Harbor (Western Australia). In Odessa on 27 June 2014 a meteotsunami devastated some beach areas and injured 6 people (Šepić et al., 2015a). In Fremantle on 17 August 2014 a ship broke a mooring line and hit a major railway bridge, causing it to be shut down for more than 2 weeks (Pattiaratchi and Wijeratne, 2015). An operational meteotsunami warning system and safety procedures at civil protection and coastal operations could significantly reduce the risk for human lives and mitigate the damage coming from destructive meteotsunami events (Golnaraghi, 2012). In some regions meteotsunami warning systems can be included into multi-hazard warning systems (MHWS), in particular, into the systems responsible for forecasting typhoons, hurricanes, and associated storm surges (cf. Pattiaratchi and Wijeratne, 2015).
Research Gaps and Perspectives
Atmospherically-induced long waves in the open ocean have spatial and temporal scales similar to the mesoscale atmospheric disturbances which generate them. An individual tsunamigenic atmospheric disturbance has typical horizontal scales of 10-100 km and can propagate over 50-500 km (Belušić et al., 2007). Meteotsunami waves are commonly formed over extensive shelf areas where the speed of the atmospheric disturbance matches the long wave speed (Šepić et al., 2015b). Meteotsunamis propagating onshore from the open ocean actively accumulate energy and are further amplified approaching the coast due to the shoaling effect (cf. Lamb, 1932); focusing in some bays and inlets they can cause catastrophic effects (Šepić et al., 2015b). Because these phenomena are relatively small-scale, a full numerical reproduction of the respective tsunamigenic atmospheric disturbances and associated long ocean waves is still a challenge. The modeling problem can be broken into four components: atmospheric modeling, ocean modeling, coupled atmospheric-ocean modeling, and modeling of coastal inundation and damage.
Aside from the exact knowledge of the atmospheric physics responsible for the creation of tsunamigenic disturbances (duct waves, wave-CISK2, storms, frontal passages, gales or squall lines, etc.; see Belušić et al., 2007; Tanaka, 2010), the source mechanism and generation of atmospheric gravity waves or other meteotsunami sources are still not properly reproduced by atmospheric models. Observations are typically inadequate to resolve the generation of atmospheric mesoscale structures and associated surface pressure disturbances. Numerical modeling of these structures is also a problem: a number of simulations may be needed for reasonable reproduction of the atmospheric conditions and traveling small-scale disturbances visible in surface air pressures or winds (Belušić et al., 2007). Similar initial atmospheric forcing—e.g., a train of atmospheric waves—traveling over a few hundred kilometres can produce completely different responses at a given “hot-spot,” depending on the specific track: disturbances, approaching the site, can intensify and produce strong sea level response or weaken and produce negligible response (Šepić and Vilibić, 2011). Novel ensemble-based methods, like stochastic convection, can overcome these shortcomings (Teixeira and Reynolds, 2008).
A critical issue in tsunami modeling is the proper reproduction of the tsunami source (Satake and Fujii, 2014). This issue is partly overcome in meteotsunami studies by imposing an artificial atmospheric disturbance, which travels over a tsunamigenic region (Whitmore and Knight, 2014; Šepić et al., 2015b). Such an approach may be effective for the hazard risk assessments, which aim to estimate and map expected extreme meteotsunami wave heights, but is inappropriate for an operative real-time meteotsunami forecast. Altogether, the reproduction of meteotsunami sources is a challenge for atmospheric scientists modeling mesoscale processes, directing their research not just to a pure increase of the model resolution (that can be done with more powerful computers), but to preserve feasible physics at these spatial and temporal scales, and to create new parameterization schemes within models.
If the atmospheric forcing is known, the ocean modeling of meteotsunamis is straightforward, as physics of generated ocean waves is barotropic to first order. This enables us to use 2D models, similar to those applied for tsunami research (Monserrat et al., 2006). Another very important issue is bathymetry, that needs to be at high spatial resolution (~10–50 m) and properly integrated into the model, especially in coastal areas and in areas of rapidly changing depth (shelf breaks, submarine channels, etc.) where meteotsunamis amplify and modify (Monserrat et al., 2006). This is particularly true for bays and harbors with large amplification factors, which are prone to frequent meteotsunami events. A small change in the model coastline or depths can result in significant changes in eigen frequencies of the respective basin and maximum estimated wave-heights (Vilibić et al., 2008).
The development of coupled atmospheric-oceanic models, which are required for accurate meteotsunami reproduction, is also important. At present, there are no reliable coupled models, since existing atmospheric models are not able to properly reproduce the evolution of traveling air pressure waves over water basins. An attempt to take into account the effect of such coupling has so far only been made by Renault et al. (2011) for the region of the Balearic Islands, however, simulated ocean wave heights at Ciutadella, the main Balearic meteotsunami “hot spot,” were significantly underestimated. Therefore, this is a principal issue where substantial advancement is needed in the future.
Precise high-resolution capacities for continuous monitoring and detection of tsunamigenic disturbances and meteotsunami waves are of primary importance. Various observational networks are available for both atmospheric and oceanic measurements, but no standards for meteotsunami observations have yet been developed and therefore not a single network was adopted to properly capture meteotsunamis. Investigations of meteotsunamis are mainly based on standard meteorological and oceanographic networks, which mostly have insufficient accuracy and too coarse temporal resolution (e.g., 6-min NOAA CO-OPS air pressure network or 10-min regional meteo-ocean buoy network in the Gulf of Maine) and do not properly capture high-frequency processes at a minute timescale (Thomson et al., 2009; Šepić and Rabinovich, 2014). Some operational networks are more advanced: in particular, on the Balearic Islands (Marcos et al., 2009; Tintoré et al., 2013) and in the Adriatic Sea (Šepić and Vilibić, 2011), but they are either in the pilot-phase of development or not incorporated into a meteotsunami warning system. A decision on new standards based on 1 min or better time resolution and 1 Pa pressure measurements should be established for the regions affected by meteotsunamis. This is especially important for meteorological observations commonly following the standards of the World Meteorological Organisation (WMO), in particular within the frame of Multi-Hazard Early Warning Systems (MHEWS; Golnaraghi, 2012).
Recently, 1-min resolution sea level observations around the world oceans became available through the IOC Sea Level Station Monitoring Facility service (http://www.ioc-sealevelmonitoring.org). These observations will definitely allow better assessment of high-frequency oscillations in many regions. The service has 100 data providers, indicating that the urgent need for high-resolution sea level monitoring, largely coming from the tsunami community, has finally pushed tide gauge observation standards to 1-min time resolution. However, spatial resolution of such a global network is insufficient for measurements of highly variable processes with scales of a few tens of kilometres or less.
Another important issue, to be potentially used in a meteotsunami warning system, is the obvious correlation of tsunamigenic atmospheric disturbances with specific weather conditions, at least in the Mediterranean region (Jansà et al., 2007) and Japan (Tanaka, 2010). These conditions are largely associated with the wave-ducting theory (Lindzen and Tung, 1976): a strong shear of unstable air masses in the mid-troposphere creates atmospheric disturbances which then propagate in the stable lower troposphere over long distances. High-resolution atmospheric and sea level data in the Mediterranean indicated a high correlation between meteotsunamis and synoptic patterns over the entire basin (Šepić et al., 2015b). It is quite possible that some other mechanisms might control meteotsunamigenic processes at other “hot spot areas.” An illustrative example is the U.S. East Coast where meteotsunamis are often generated by hurricanes or large scale derecho systems (Wertman et al., 2014; Šepić and Rabinovich, 2014).
“Classical” sea level instruments and approaches might not be a solution for proper measurements of meteotsunami waves, as it is too costly to have a dense tide gauge network at meteotsunami hot spots. A densified network of cheap autonomous water level loggers might be a better choice, as it requires no maintenance and is easily deployed and recovered at piers, cliffs and the sea bottom. However, these water level data are not available in real time and may be used only for research purposes. Multi-hazard standard observatories, satellites mapping the spatial and temporal characteristics of tsunamigenic disturbances (Belušić and Strelec Mahović, 2009) and meteorological (Anderson et al., 2015) or high-frequency ocean radars (Lipa et al., 2014) for early detection of meteotsunami waves, may be other ways of collecting the data for real-time meteotsunami warning. Unfortunately, these observation systems are expensive, cannot cover all meteotsunami hot spots and their applicability for meteotsunami detection still needs to be quantified.
Most of the above research issues are still focused on reproduction of tsunamigenic atmospheric disturbances and associated oceanic waves. This respective knowledge is a prerequisite for building a meteotsunami warning system. However, there are other aspects relevant for creation of a system appropriate for a priori studies, in particular, for assessment of meteotsunami hazard, vulnerability and risk, including socio-economic effects (Geist et al., 2014).
In summary, the critical components of meteotsunami research that, in our opinion, need to be advanced for better understanding of the phenomenon and eventual creation of a reliable meteotsunami warning system are:
(i) Improvement of atmospheric models and of their high-resolution physics, resulting in reliable reproduction of tsunamigenic atmospheric disturbances;
(ii) Development of coupled atmosphere-ocean models to reproduce meteotsunami events;
(iii) Construction of high-resolution bathymetry grids for coastal regions and critical depth features (shelf breaks, canyons, shoals, sills, etc.);
(iv) Connection of intense high-frequency sea level oscillations with particular synoptic conditions and definition of site-dependant and region-dependant meteotsunami indices;
(v) Installation of meteorological radars for continuous monitoring of tsunamigenic atmospheric disturbances and elaboration of efficient detection algorithms to identify these disturbances;
(vi) Verification of new technological solutions and instrumentation for detection of spatial and temporal characteristics of tsunamigenic atmospheric disturbances and associated ocean waves, and their amplification approaching the coast;
(vii) Risk assessment of meteotsunamis and mitigation of their socio-economic impact.
Toward Meteotsunami Warning Systems
A meteotsunami warning system for particular hot-spot areas can be created based on the following four approaches: (i) identification of tsunamigenic atmospheric synoptic conditions; (ii) real-time detection of tsunamigenic atmospheric disturbances using a microbarograph network; (iii) measurement and tracking of high-frequency sea level oscillations by high-resolution digital tide gauges; and (iv) numerical simulation of meteotsunamis based on coupling of atmosphere-ocean numerical models.
The first approach is already operational on the Balearic Islands, where a meteotsunami forecast is given a few days ahead, but only at the qualitative level (Jansà et al., 2007). This forecast is based on identification of favorable synoptic conditions which include: (i) weak winds at the sea surface, (ii) an inflow of hot and dry air masses from Africa in the lower troposphere, overtopped by (iii) a strong mid-troposphere jet characterized by (iv) unstable conditions which favor the growth of mid and upper troposphere convective formations.
The second approach has been preliminary tested at a pilot microbarograph network in the Adriatic Sea (Šepić and Vilibić, 2011), and it is based on real-time detection of intense air pressure disturbances. The intensity is determined from 5-min air pressure segments. Parameters of the identified tsunamigenic disturbances are then automatically calculated. These parameters include disturbance intensity, rate of change and propagation direction and speed, which are then compared with the prescribed values in the meteotsunami warning matrix estimated from historical meteotsunami events (Šepić and Vilibić, 2011). This is similar to a procedure that has been used for seismic tsunamis (Tinti et al., 2012). Such an approach can be used for an operative forecast of potentially destructive events an hour or less before their arrival at the coast.
The main idea of the third approach is sea level monitoring at a “beacon” station positioned off the hot-spot region, providing again approximately an hour of advance time for the most endangered locations (Marcos et al., 2009). This approach is also similar to the procedures developed for early tsunami warning, which is based on open-ocean tsunami detection systems (Mungov et al., 2013). However, since meteotsunamis are generated over shelf areas or along coasts, the respective warning system should be based on measurements at available island stations, shelf buoys or coastal stations that are positioned along the wave path.
The fourth approach is operational within the BRIFS (Balearic Rissaga Forecasting System, www.socib.eu). Present coupled atmospheric-oceanic models are able to provide qualitative reproduction of meteotsunami waves, but still underestimate their amplitude and the potential for damage (Renault et al., 2011). This is because these models do not describe properly the evolution of tsunamigenic disturbances propagating onshore.
None of the above approaches can yet provide reliable early warning. A meteotsunami warning system should have identification-to-warning time on a minute scale, it has to be able to identify most potentially destructive events and to produce a minimum number of false warnings, following criteria developed for tsunami warning systems (Igarashi et al., 2011; Pararas-Carayannis, 2015). Therefore, the architecture of a meteotsunami warning system has to be based on a multilevel structure (Figure 2). Preconditioning (detection of tsunamigenic synoptic conditions), source detection (modeling and real-time tracking of atmospheric disturbances) and real-time numerical modeling of meteotsunami generation, propagation and transformation in the coastal zone should be part of an integrated system. Such a system needs to become a part of the general tsunami warning system, or of a broader MHEWS system, once it has advanced to the operative level of providing reliable disaster warnings. The system could be supplemented with additional procedures, including radar or satellite detection of tsunamigenic atmospheric disturbances (Belušić and Strelec Mahović, 2009; Anderson et al., 2015) and hazardous long ocean waves (Lipa et al., 2014). The warning system should also be site- or region-specific, as the phenomenon seems to have regionally dependant characteristics and on particular occasions can affect thousands of kilometres within a few days (Šepić et al., 2015a).
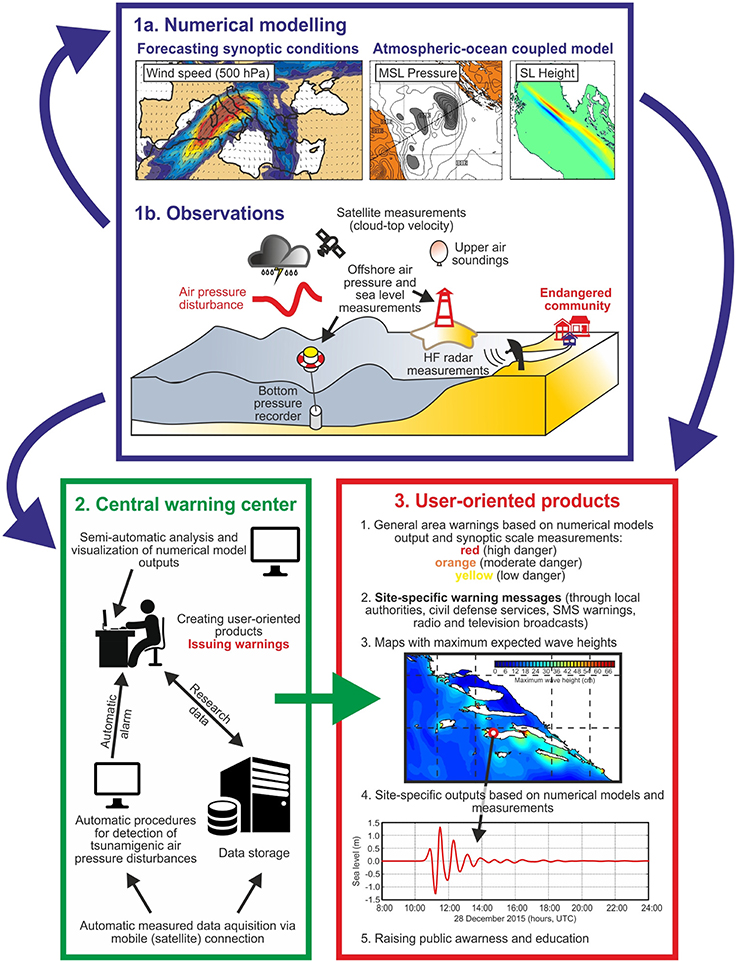
Figure 2. The multilayer architecture of the proposed meteotsunami warning system, which includes observational and numerical modeling capacities, processing tools for detection of tsunamigenic conditions, and capacities for dissemination of the warning to civil authorities and the public.
Constructing a meteotsunami warning system for any region should take into account the cost of such a system in comparison with the meteotsunami risk over a reasonable timescale. It is obvious that such a system is primarily important for “hot spots,” i.e., for specific areas where destructive meteotsunamis can be expected. Active international cooperation and close coordination of efforts, exchange of ideas, knowledge, and approaches are crucial for successful investigation and mitigation of this natural hazard.
Author Contributions
IV coordinated the work on the manuscript and wrote the initial version of the manuscript, JS prepared the figures and revised the text substantially, AR did a substantial polishing of text and figures, while all authors actively contributed to the development of the manuscript idea, to its writing and preparation of figures.
Conflict of Interest Statement
The authors declare that the research was conducted in the absence of any commercial or financial relationships that could be construed as a potential conflict of interest.
Acknowledgments
We wish to thank Fred Stephenson of the Canadian Hydrographic Service, Institute of Ocean Sciences (Sidney, British Columbia) for editing the text and helpful advice. The work of IV and JS has been supported by the Croatian Science Foundation under the project MESSI (UKF Grant No. 25/15) and SCOOL (IP-2014-09-5747), and for AR by the Russian Science Foundation (grant 14-50-00095) and funds of IO RAS.
Footnotes
1. ^It is also published as a book by Springer (Dordrecht, The Netherlands, 2014).
2. ^Conditional Instability of the Second Kind.
References
Anderson, E. J., Bechle, A. J., Wu, C. H., Schwab, D. J., Mann, G. E., and Lombardy, K. A. (2015). Reconstruction of a meteotsunami in Lake Erie on May 27, 2012: roles of atmospheric conditions on hydrodynamic response in enclosed basins. J. Geophys. Res. 120, 8020–8038. doi: 10.1002/2015JC010883
Belušić, D., Grisogono, B., and Bencetić Klaić, Z. (2007). Atmospheric origin of the devastating coupled air-sea event in the east Adriatic. J. Geophys. Res. 112, D17111. doi: 10.1029/2006JD008204
Belušić, D., and Strelec Mahović, N. (2009). Detecting and following atmospheric disturbances with a potential to generate meteotsunamis in the Adriatic. Phys. Chem. Earth 34, 918–927. doi: 10.1016/j.pce.2009.08.009
Ewing, M., Press, F., and Donn, W. L. (1954). An explanation of the Lake Michigan wave of 26 June 1954. Science 120, 684–686. doi: 10.1126/science.120.3122.684
Geist, E. L., ten Brink, U. S., and Gove, M. (2014). A framework for the probabilistic analysis of meteotsunamis. Nat. Hazards 74, 123–142. doi: 10.1007/s11069-014-1294-1
Golnaraghi, M. (2012). Institutional Partnerships in Multi-Hazard Early Warning Systems. Heidelberg: Springer.
Greenspan, H. P. (1956). The generation of edge waves by moving pressure distributions. J. Fluid Mech. 1, 575–592. doi: 10.1017/S002211205600038X
Haslett, S. K., and Bryant, E. A. (2009). Meteorological tsunamis in Southern Britain: an historical review. Geogr. Rev. 99, 146–163. doi: 10.1111/j.1931-0846.2009.tb00424.x
Hibiya, T., and Kajiura, K. (1982). Origin of “abiki” phenomenon (kind of seiches) in Nagasaki Bay. J. Oceanogr. Soc. Jap. 38, 172–182. doi: 10.1007/BF02110288
Igarashi, Y., Kong, L., Yamamoto, M., and McCreery, C. S. (2011). Anatomy of historical tsunamis: lessons learned for tsunami warning. Pure Appl. Geophys. 168, 2043–2063. doi: 10.1007/s00024-011-0287-1
Jansà, A., Monserrat, S., and Gomis, D. (2007). The rissaga of 15 June 2006 in Ciutadella (Menorca), a meteorological tsunami. Adv. Geosci. 12, 1–4. doi: 10.5194/adgeo-12-1-2007
Lander, J. F., Lockridge, P. A., and Kozuch, M. J. (1993). Tsunamis affecting the West Coast of the United States, 1806-1992. Boulder: Colorado, National Geophysical Data Center, 242.
Lindzen, R. S., and Tung, K.-K. (1976). Banded convective activity and ducted gravity waves. Mon. Wea. Rev. 104, 1602–1617.
Lipa, B., Parikh, H., Barrick, D., Roarty, H., and Glenn, S. (2014). High-frequency radar observations of the June 2013 US East Coast meteotsunami. Nat. Hazards 74, 109–122. doi: 10.1007/s11069-013-0992-4
Marcos, M., Monserrat, S., Medina, R., Orfila, A., and Olabarrieta, M. (2009). External forcing of meteorological tsunamis at the coast of the Balearic Islands. Phys. Chem. Earth 34, 938–947. doi: 10.1016/j.pce.2009.10.001
Monserrat, S., Vilibić, I., and Rabinovich, A. B. (2006). Meteotsunamis: atmospherically induced destructive ocean waves in the tsunami frequency band. Nat. Hazards Earth Syst. Sci. 6, 1035–1051. doi: 10.5194/nhess-6-1035-2006
Mungov, G., Eblé, M., and Bouchard, R. (2013). DART® Tsunameter retrospective and real-time data: a reflection on 10 years of processing in support of tsunami research and operations. Pure Appl. Geophys. 170, 1369–1384. doi: 10.1007/s00024-012-0477-5
Nomitsu, T. (1935). A theory of tsunamis and seiches produced by wind and barometric gradient. Mem. Coll. Sci. Imp. Univ. Kyoto A 18, 201–214.
Pararas-Carayannis, G. (2015). Tsunami warning system in the Pacific: brief historical review of its establishment and institutional support. Sci. Tsunami Hazards 34, 101–139.
Pattiaratchi, C. B., and Wijeratne, E. M. S. (2015). Are meteotsunamis an underrated hazard? Philos. Trans. R. Soc. A 373, 20140377. doi: 10.1098/rsta.2014.0377
Proudman, J. (1929). The effects on the sea of changes in atmospheric pressure. Geophys. Suppl. Mon. Notices R. Astr. Soc. 2, 197–209. doi: 10.1111/j.1365-246X.1929.tb05408.x
Rabinovich, A. B. (2009). “Seiches and harbor oscillations,” in Handbook of Coastal and Ocean Engineering, ed Y. C. Kim (Singapore: World Scientific Publishing Company), 193−236.
Rabinovich, A. B., and Monserrat, S. (1996). Meteorological tsunamis near the Balearic and Kuril Islands: descriptive and statistical analysis. Nat. Hazards 13, 55–90. doi: 10.1007/BF00156506
Rabinovich, A. B., and Monserrat, S. (1998). Generation of meteorological tsunamis (large amplitude seiches) near the Balearic and Kuril Islands. Nat. Hazards 18, 27–55. doi: 10.1023/A:1008096627047
Rabinovich, A. B., Vilibić, I., and Tinti, S. (2009). Meteorological tsunamis: atmospherically induced destructive ocean waves in the tsunami frequency band. Phys. Chem. Earth 34, 891–893. doi: 10.1016/j.pce.2009.10.006
Renault, L., Vizoso, G., Jansà, A., Wilkin, J., and Tintoré, J. (2011). Toward the predictability of meteotsunamis in the Balearic Sea using regional nested atmosphere and ocean models. Geophys. Res. Lett. 38, L10601. doi: 10.1029/2011gl047361
Satake, K., and Fujii, Y. (2014). Review: source models of the 2011 Tohoku Earthquake and long-term forecast of large earthquakes. J. Disaster Res. 9, 272–280. doi: 10.20965/jdr.2014.p0272
Šepić, J., and Rabinovich, A. B. (2014). Meteotsunami in the Great Lakes and on the Atlantic coast of the United States generated by the “derecho” of June 29–30, 2012. Nat. Hazards 74, 75–107. doi: 10.1007/s11069-014-1310-5
Šepić, J., Vilibić, I., and Fine, I. (2015b). Northern Adriatic meteorological tsunamis: assessment of their potential through ocean modeling experiments. J. Geophys. Res. 120, 2993–3010. doi: 10.1002/2015JC010795
Šepić, J., Vilibić, I., Rabinovich, A. B., and Monserrat, S. (2015a). Widespread tsunami-like waves of 23-27 June in the Mediterranean and Black Seas generated by high-altitude atmospheric forcing. Sci. Rep. 5, 11682. doi: 10.1002/2015JC010795
Šepić, J., and Vilibić, I. (2011). The development and implementation of a real-time meteotsunami warning network for the Adriatic Sea. Nat. Hazards Earth Syst. Sci. 11, 83–91. doi: 10.5194/nhess-11-83-2011
Sibley, A., Cox, D., Long, D., Tappin, D., and Horseburgh, K. (2016). Meteorologically generated tsunami-like waves in the North Sea on 1/2 July 2015 and 28 May 2008. Weather 71, 68–74. doi: 10.1002/wea.2696
Soloviev, S. L., and Go, C.h. N. (1974). Catalogue of Tsunamis on the Western Shore of the Pacific Ocean. Moscow: Nauka. [in Russian; English Translation: Canadian Transl. Fish. Aquatic Sci., No. 5078, Ottawa, 1984, 439].
Tanaka, K. (2010). Atmospheric pressure-wave bands around a cold front resulted in a meteotsunami in the East China Sea in February 2009. Nat. Hazards Earth Syst. Sci. 10, 2599–2610. doi: 10.5194/nhess-10-2599-2010
Tappin, D., Sibley, A., Horsburgh, K., Daubord, C., Cox, D., and Long, D. (2013). The English Channel tsunami of 27 June 2011 – a probable meteorological source. Weather 68, 144–152. doi: 10.1002/wea.2061
Teixeira, J., and Reynolds, C. A. (2008). Stochastic nature of physical parameterizations in ensemble prediction: a stochastic convection approach. Mon. Wea. Rev. 136, 483–496. doi: 10.1175/2007MWR1870.1
Thomson, R. E., Rabinovich, A. B., Fine, I. V., Sinnott, D. C., McCarthy, A., Sutherland, N. A. S., et al. (2009). Meteorological tsunamis on the coasts of British Columbia and Washington. Phys. Chem. Earth 34, 971–988. doi: 10.1016/j.pce.2009.10.003
Tinti, S., Graziani, L., Brizuela, B., Maramai, A., and Gallazzi, S. (2012). Applicability of the decision matrix of North Eastern Atlantic, Mediterranean and connected seas Tsunami Warning System to the Italian tsunamis. Nat. Hazards Earth Syst. Sci. 12, 843–857. doi: 10.5194/nhess-12-843-2012
Tintoré, J., Vizoso, G., Casas, B., Heslop, E., Pascual, A., Orfila, A., et al. (2013). SOCIB: The Balearic Islands Coastal Ocean Observing and Forecasting System responding to science, technology and society needs. Mar. Technol. Soc. J. 47, 101–117. doi: 10.4031/MTSJ.47.1.10
Vilibić, I., Monserrat, S., and Rabinovich, A. B. (2014). Meteorological tsunamis on the US East Coast and in other regions of the World Ocean. Nat. Hazards 74, 1–9. doi: 10.1007/s11069-014-1350-x
Vilibić, I., Monserrat, S., Rabinovich, A. B., and Mihanović, H. (2008). Numerical modelling of the destructive meteotsunami of 15 June 2006 on the coast of the Balearic Islands. Pure Appl. Geophys. 165, 2169–2195. doi: 10.1007/s00024-008-0426-5
Vučetić, T., Vilibić, I., Tinti, S., and Maramai, A. (2009). The Great Adriatic flood of 21 June 1978 revisited: an overview of the reports. Phys. Chem. Earth 34, 894–903. doi: 10.1016/j.pce.2009.08.005
Wertman, C. A., Yablonsky, R. M., Shen, Y., Merrill, J., Kincaid, C. R., and Pockalny, R. A. (2014). Mesoscale convective system surface pressure anomalies responsible for meteotsunamis along the US East Coast on June 13th, 2013. Sci. Rep. 4, 7143, doi: 10.1038/srep07143
Keywords: meteotsunami, natural hazard, air-sea interaction, world oceans, research gaps, sea level measurements, early warning system
Citation: Vilibić I, Šepić J, Rabinovich AB and Monserrat S (2016) Modern Approaches in Meteotsunami Research and Early Warning. Front. Mar. Sci. 3:57. doi: 10.3389/fmars.2016.00057
Received: 23 October 2015; Accepted: 11 April 2016;
Published: 03 May 2016.
Edited by:
Ivan David Haigh, University of Southampton, UKReviewed by:
Matthew John Eliot, Damara WA Pty Ltd, AustraliaKevin James Horsburgh, National Oceanography Centre, UK
Copyright © 2016 Vilibić, Šepić, Rabinovich and Monserrat. This is an open-access article distributed under the terms of the Creative Commons Attribution License (CC BY). The use, distribution or reproduction in other forums is permitted, provided the original author(s) or licensor are credited and that the original publication in this journal is cited, in accordance with accepted academic practice. No use, distribution or reproduction is permitted which does not comply with these terms.
*Correspondence: Ivica Vilibić, dmlsaWJpY0Bpem9yLmhy