- 1School of Geography, College of Life and Environmental Sciences, University of Exeter, Exeter, United Kingdom
- 2College of Marine and Environmental Sciences, James Cook University, Townsville, QLD, Australia
High sea surface temperatures (SSTs) on the Great Barrier Reef (GBR) during summer 2015/2016 caused extensive coral bleaching, with aerial and in-water surveys confirming high (but variable) bleaching-related coral mortality. In contrast, bleaching impacts on nearshore turbid-zone reefs, traditionally considered more “marginal” coral habitats, remain poorly documented. This is because rapid ecological surveys are difficult in these turbid water settings, and baseline coral community data from which to quantify disturbance are rare. However, models suggest that the extreme environmental conditions characteristic of nearshore settings (e.g., fluctuating turbidity, light, and temperature) may acclimate corals to the thermal anomalies associated with bleaching on offshore reefs, although validation by field evidence has to-date been sparse. Here we present a novel pre- (June 2013/2014) and post-warming (August 2016) assessment of turbid-zone coral communities and examine the response of corals to prolonged and acute heat stress within the Paluma Shoals reef complex, located on the central GBR. Our analysis of 2,288 still video frames (~1,200 m2) which include 11,374 coral colonies (24 coral genera) suggest a high tolerance of turbid-zone corals to bleaching, with no significant changes in coral cover (pre: 48 ± 20%; post: 55 ± 26%) or coral community structure (e.g., Acropora, Montipora, Turbinaria, Porites) following the warming event. Indeed, only one coral colony (Lobophyllia sp.) exhibited full colony bleaching, and just 1.5% of colonies displayed partial pigmentation loss (<20% colony surface). Taxa-specific responses to this thermal stress event contrast with clear-water assessments, as Acropora corals which are normally reported as highly susceptible to bleaching on clear-water reefs were least impacted at Paluma Shoals, a phenomena that has been observed within other turbid settings. Importantly, field surveys confirm regional SSTs were sufficiently high to induce coral bleaching (i.e., comparable number of degree heating days in nearshore and offshore areas), but bleaching severity was much higher at central GBR offshore sites. A more optimistic outlook than is generally offered for nearshore reefs on the central GBR may be implied by our results, which highlights the importance of these resilient but often overlooked coral reef habitats as potential refugia during climate-related disturbances.
Introduction
Elevated global ocean temperatures since 2014 have caused widespread coral bleaching throughout all major reef-building regions, with temperatures peaking in 2015/2016. This temperature anomaly resulted in the onset of the “third global coral bleaching event” on record. The term “coral bleaching” refers to a loss of color in corals after they expel their endosymbionts (Symbiodinium) and occurs most often as a stress response to elevated sea surface temperatures (Brown, 1997; Baker et al., 2008). Resultant deleterious physiological effects include reduced coral growth and increased mortality (Douglas, 2003), and at a community level, reductions in coral cover and shifts in coral community structure to less diverse assemblages composed of the most resilient taxa only (Marshall and Baird, 2000; Hughes et al., 2007). This most recent warming event, which has been unprecedented in its magnitude and duration, has affected vast areas of coral-dominated habitat within the central Indian Ocean (Maldives), Western Australia, Pacific Ocean, the Red Sea, the Caribbean, and the Great Barrier Reef (GBR) (Lafratta et al., 2016; Hughes et al., 2017; Perry and Morgan, 2017; see DeCarlo et al., 2017). On the GBR, above-average sea surface temperatures (SSTs) occurred in the late austral summer (2015/2016). Aerial surveys of 911 mid- and outer-shelf reefs indicated high levels of bleaching (~90% of surveyed reefs) within the northern and central GBR, with subsequent in-water assessments confirming highest mortality occurring within the northern GBR sector and reducing on the southern GBR (Great Barrier Reef Marine Park Authority, 2016; Hughes et al., 2017). Surveys of recently bleached reefs have focused on those that could be observed from the air and easily surveyed in the water. Thus the condition of nearshore turbid-zone reefs within the affected region was not investigated as; (1) both aerial and in-water surveys are difficult due to high turbidity; and (2) ecological baseline data against which to quantitatively assess bleaching impacts are available for very few of these reefs.
The response of nearshore turbid-zone coral assemblages to conditions responsible for the severe and widespread bleaching of clear-water reefs is, however, of major research interest as it has been hypothesized that they may represent potential refugia for corals from large-scale climatic disturbances (Potts and Jacobs, 2000; van Woesik et al., 2012; Cacciapaglia and van Woesik, 2015). Turbid-zone reefs have traditionally been perceived as unsuitable, or at best “marginal” for healthy coral growth because corals are exposed to high turbidity, low-light availability, large salinity and temperature fluctuations, high tidal ranges and prolonged aerial exposure (Kleypas, 1996; Kleypas et al., 1999). However, a growing number of field studies have documented the diversity and adaptive capacity of turbid-zone coral communities across a range of geographic locations and time-scales (Bull, 1982; Perry et al., 2009; Browne et al., 2010; Roche et al., 2011; Butler et al., 2013; Fellegara et al., 2013; Richards et al., 2015; Guest et al., 2016; Lafratta et al., 2016; Morgan et al., 2016a; Ryan et al., 2016). This collective body of research suggests that these reefs occupy far larger areas than previously thought and support high coral cover including taxa (e.g., Acropora) typically regarded as highly susceptible to thermal stress (Marshall and Baird, 2000; Browne et al., 2010; Morgan et al., 2016a; Hughes et al., 2017). Key to the success and ability of these corals to grow under light-limited conditions (often at rates similar to clear-water corals; Browne, 2012) appears to be their capacity to effectively utilize both phototropic/heterotrophic feeding and sediment sloughing mechanisms (Anthony and Fabricius, 2000; Anthony et al., 2005). Environmental factors (e.g., elevated suspended sediment concentrations) may also inhibit the harmful interaction between heat stress and high light intensity on corals within turbid settings by rapidly attenuating sunlight through the water column (Storlazzi et al., 2015). Combined, these factors form the basis of global-scale modeling efforts that have identified turbid reef areas (which include nearshore areas of the GBR) as potential coral refugia locations (Cacciapaglia and van Woesik, 2015). Critical to our understanding of whether turbid-zone corals may indeed have the capacity to withstand thermal extremes associated with major coral bleaching events is empirical field data documenting coral response during (or soon after) thermal stress has occurred, but such data are scarce.
Fortuitously, ecological surveys were conducted across the Paluma Shoals reef complex (PSRC), a series of nearshore turbid-zone reefs located in Halifax Bay on the central GBR, prior to the summer 2016 SST warming. These surveys revealed thriving coral communities that have displayed a remarkable resilience to the major factors which have contributed to the degradation of clear-water reefs further offshore (e.g., declining water quality, cyclone impacts, crown-of-thorns sea stars; De'ath et al., 2012). This existing dataset, collected using video during a period of exceptionally calm and clear water conditions captured substantial baseline information on coral community composition within these normally high turbidity settings close to the initial onset and subsequent progression of the mid-2016 warming event (data were collected in July 2013 and 2014). These data provide: (1) a unique opportunity to quantitatively assess the extent to which the 2016 ocean warming impacted turbid-zone coral communities at PSRC; and (2) to test the hypothesis that turbid-zone reefs may function as refugia from increased SSTs. Here we report data from repeat field surveys and subsequent bleaching assessments immediately following the warming event in the early austral winter (August 2016), and present pre- and post-warming coral community data to examine the response of turbid-zone reefs to prolonged thermal stress.
Materials and Methods
Study Site
The Paluma Shoals reef complex (PSRC) covers an area of 15.5 km2 (Latitude: −19.1145°, Longitude: 146.5497°) and is located within Halifax Bay on the central GBR (Figure 1). The area encompasses seven discrete reef structures, each of which presently exhibits different stages of geomorphic maturity (sensu Hopley et al., 2007) defined by the current depth relative to lowest astronomical tide (LAT; reef surfaces range from −0.6 to +0.5 m below LAT). The regional distribution and morphology of these reef structures has recently been described from acoustic surveys of seafloor bathymetry (see Morgan et al., 2016a for full physical and ecological descriptions) and shows coral reef growth to be concentrated above large submarine bedforms (1–1.7 km long and 200 m wide) orientated perpendicular to the coast (Larcombe and Carter, 2004; Morgan et al., 2016a). The topography of these bedforms provides elevation for corals above the muddy seafloor and into the shallower sediment resuspension zone (sensu Wolanski et al., 2005), as well as increasing access to light for coral photosynthesis. Under these conditions reef initiation in the area began on coarse lithic gravels between ~2000 and 700 cal. y BP (calibrated years before present), and thus these reefs are geologically very young features with short growth histories as a consequence of their relatively recent initiation (Smithers and Larcombe, 2003; Morgan et al., 2016b). Since this time vertical reef growth has been driven by the carbonate production of established framework-building coral taxa (Acropora, Montipora, and Turbinaria) and interstitial terrigenous sediment inputs (Palmer et al., 2010; Perry et al., 2013; Morgan et al., 2016b; Johnson et al., 2017). The growth history and ecological communities (and the environmental conditions they inhabit) of these nearshore reefs are thus distinct from those inner-shelf (or “inshore”) reefs on the central GBR further seaward which have recently experienced large-scale ecological disturbance (e.g., Pelorus Reef; Roff et al., 2013).
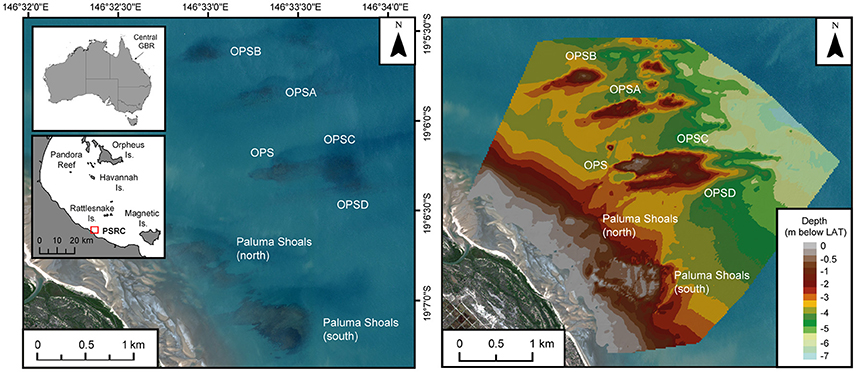
Figure 1. Site map of the Paluma Shoals reef complex (PSRC) located within Halifax Bay on the central Great Barrier Reef, Australia. Satellite imagery and bathymetric seafloor model (m below lowest astronomical tide; LAT) show the position and morphology of the seven discrete nearshore turbid-zone coral reefs that comprise the complex. OPS [A, B, C, D], Offshore Paluma Shoals [A, B, C, D].
Coral cover on many nearshore turbid-zone reefs on the central GBR is high (up to 55%), and they support relatively diverse coral assemblages both within the contemporary communities and the palaeorecord (Perry et al., 2009; Browne et al., 2010; Roche et al., 2011; Morgan et al., 2016a; Ryan et al., 2016; Johnson et al., 2017). These coral communities provide important structural complexity in an otherwise flat soft sediment-dominated coastal zone. However, the abundance of fine clastic sediment, much of which is a legacy of material transported onshore during the last marine transgression and augmented by riverine inputs since sea level stabilized in the mid-Holocene (Larcombe and Woolfe, 1999), produces naturally high turbidity as seafloor sediment is continually resuspended by incident wave energy (Larcombe et al., 1995). The corals that occupy these turbid settings therefore tolerate large fluctuations in turbidity (Larcombe et al., 1995; Browne et al., 2013b; up to 385 mg l−1) and experience the low-light (or mesophotic) conditions typical of these nearshore settings. The term “mesophotic” was first used to describe muddy coastal reef settings in the fossil record (e.g., Rosen et al., 2000; Santodomingo et al., 2016), and whilst it has more recently been associated with coral reefs at depth (>30 m) (e.g., Bridge et al., 2011), it is a descriptor based on light (not depth) and should thus also be used for reefs occupying shallow-water turbid settings, such as those described here.
Sea Surface Temperature Anomalies (2015/2016) On the Great Barrier Reef
Mass coral bleaching occurred on the GBR in 2016 triggered by above normal SSTs (1–1.3°C higher than the 1961–1990 GBR average; Great Barrier Reef Marine Park Authority, 2016). A strong El Niño event across the Pacific indirectly intensified thermal stress on corals by reducing monsoon activity in the region that resulted in relaxed trade winds, long periods with no cloud cover, and clearer and calmer water conditions which warmed surface waters more than normal. During the late austral summer (February-June 2016) SSTs on the central and northern GBR (including coastal areas nearby to Paluma Shoals) were the highest on record, with 31% of reefs experiencing 8–16° Heating Weeks (DHWs) (Hughes et al., 2017; see Supplementary Figure S2). DHWs are an indicator of thermal stress calculated as the cumulative positive anomaly from mean SST and used to identify potential areas of coral bleaching (Liu et al., 2006). A latitudinal gradient in heat stress and coral bleaching was documented across the GBR, with the highest ocean temperature anomalies reported within the northern GBR sector, and “severe” to “minor” warming across the central and southern GBR, respectively. Satellite-derived sea surface temperature anomalies (SSTA) at a finer spatial scale (2 × 2 km grid size) indicate above-average SSTs (2–3°C) in the immediate region surrounding the PSRC between mid-February and early-August 2016 (Figure 2). SSTA are the difference between SST values and the long-term monthly mean SST and were used here to generate 14-day mosaic maps for Halifax Bay from 1-day night-only SST (data sourced from ReefTemp Next Generation; www.bom.gov.au/environment/activities/reeftemp/reeftemp.shtml; Garde et al., 2014). Given the magnitude and length of SSTA within nearshore areas of Halifax Bay and the documented relationship between heat exposure and coral bleaching reported following the 2016 event (see Hughes et al., 2017), it is reasonable to infer high thermal stress at PSRC over this period.
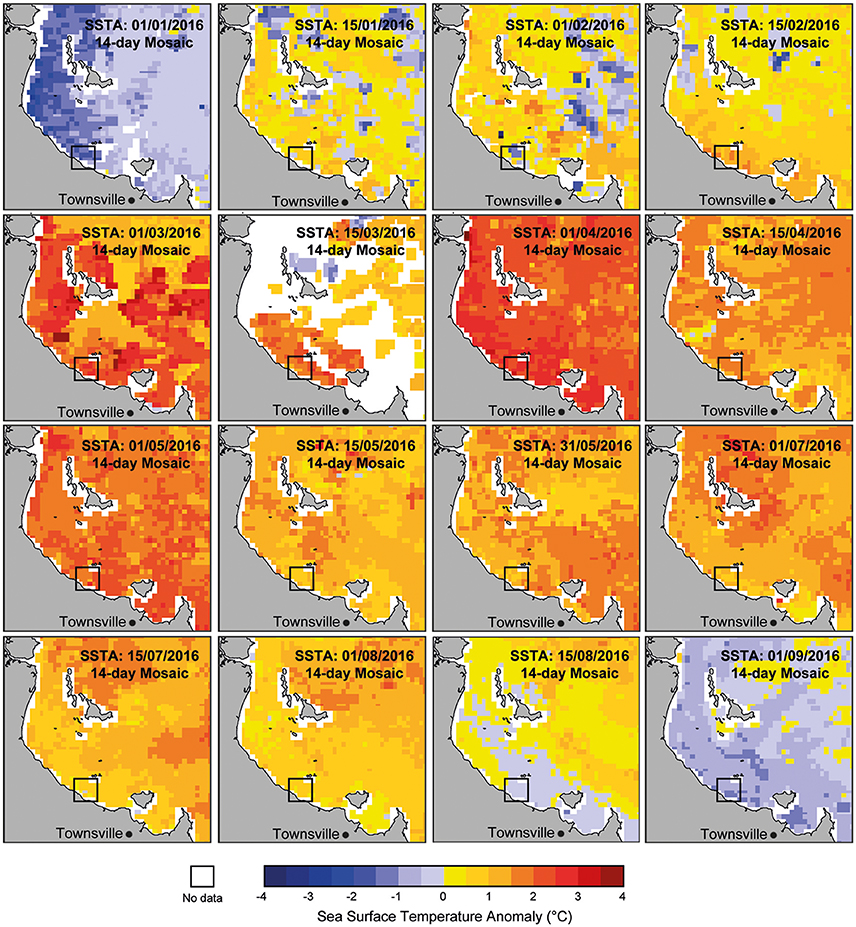
Figure 2. Satellite-derived sea surface temperature anomalies (SSTA) for Halifax Bay (2 × 2 km grid size) showing the increase in SSTs since the start of 2016 in the region. SSTA are the difference between SST values and the long-term monthly mean SST. Maps were generated using 14-day mosaics for Halifax Bay from 1-day night-only SST (Garde et al., 2014). Note that temperatures in the vicinity of the proposed study area (black box) have been 2–3° above “normal” since February 2016. Data source: ReefTemp Next Generation (www.bom.gov.au/environment/activities/reeftemp/reeftemp.shtml).
Coral Community Surveys and Bleaching Assessments
Detailed surveys of coral cover and community assemblages were conducted across the PSRC during July 2013 and 2014 (see Morgan et al., 2016a), prior to the 2016 bleaching event. In early August 2016, at the beginning of the austral winter and immediately following the period of ocean warming, repeat ecological surveys were undertaken across the main PSRC structures to document coral cover and bleaching impacts. Towed video surveys using the same drop-down video system used in our 2013/14 surveys (SeaViewer with Sea-Track™ GPS overlay) were carried out along the same 300 m transect lines (20–40 m spacings). A forward-facing GoPro camera was also attached to the frame to obtain oblique 4k resolution video of coral communities and of coral reef three-dimensionality (for rugosity classification scheme see Morgan et al., 2016a). Still frames (~0.5 m2) were extracted from the drop-down video at automated 8 s intervals (n = 2,288) and a digital 9-point grid overlay was added for coral taxonomic analysis (coral genera and growth morphology) by point-counting corals under each of the nine points. Each frame was assigned a specific depth value relative to LAT using a digital model of seafloor bathymetry for the area (Figure 1). Data were analyzed using a 10-frame moving average across consecutive frames to characterize the relative abundance (%) of coral taxonomic groups. Changes in coral cover and community structure pre- and post-warming were examined by comparing the two survey datasets (2013/2014 and 2016).
All coral colonies within the still images (n = 2,288) were counted and assessed for signs of bleaching or recent mortality (dead in situ coral framework with no/little algal growth). Image analysis was undertaken by a single observer and then validated by two independent observers to ensure consistency. Coral colonies that exhibited bleaching were counted and categorized by the following bleaching states (modified from Beeden et al., 2008): (1) total bleaching = complete loss of symbiotic algae; (2) partial bleaching = small sections of the colony fully bleached; (3) non-focal bleaching = small diffuse patterns of bleaching on colony surfaces; (4) focal bleaching = small multifocal patterns of bleaching with discrete borders to healthy tissue; and (5) paling = lack of pigmentation/reduction in symbiont concentration (i.e., discoloration) (Figure 3). Each observed bleached colony was identified to the lowest taxonomic level possible and the intensity of bleaching across the colony surface estimated categorically (e.g., 0–10, 11–20, 21–30%).
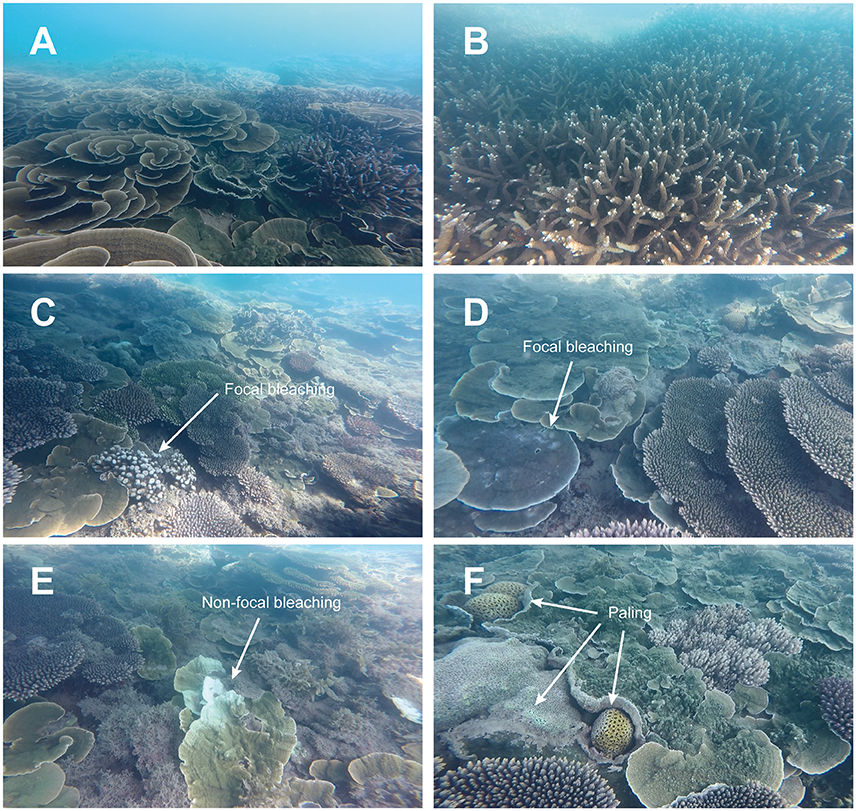
Figure 3. Turbid-zone coral communities of the Paluma Shoals reef complex (PSRC) following the 2016 bleaching event (photos taken at 1–3 m water depth during a period of exceptional water clarity). (A,B) Corals exhibit high coral cover of taxa generally considered most susceptible to bleaching (e.g., branching Acropora). (C,D) Examples of focal bleaching (small multifocal patterns of bleaching with discrete borders to healthy tissue) typically observed on Porites sp. nubbins and Montipora sp. plates. (E) Non-focal bleaching (small diffuse patterns of bleaching on colony surface) affecting part of a Montipora sp. colony. (F) Coral paling (lack of pigmentation) associated with submassive coral colonies.
Light attenuation through the water column at PSRC was measured at 0.5 m depth intervals (to 5 m below LAT) under low (~10 mg l−1) and high (~80 mg l−1) turbidity scenarios (based on values derived by Browne et al., 2013b). A light meter (LiCOR LI-192SA) was lowered through the water to each depth interval and average light intensity over a 15-second period recorded. Measurements were repeated 18 times over 3 days and then averaged to determine a mean value of light decay through the water column.
Data Analysis
Prior to analysis all data were tested for normality. Non-transformed data were then used to test for differences in coral cover (t-test) and reef rugosity (Kruskall-Wallis) between pre- (2013/2014) and post-warming (2016) surveys. Multivariate statistical analysis was used to test for differences in coral community composition, both in terms of the prevalence of different coral genera and growth morphologies using a one-way PERMANOVA based on a Bray-Curtis similarity matrix. Bleaching prevalence was calculated by dividing the pooled number of colonies that showed signs of bleaching by the total number of colonies recorded within the frames (11,374 colonies). A bleaching index (BI) was calculated for each taxon, and is a scaled index of coral thermal response (i.e., a single measure of susceptibility) which accounts for the relative proportion of the coral taxonomic group on the reef (modified from McClanahan et al., 2004). The BI of corals was estimated as follows:
Where c1 to c11 are the different categories of bleaching intensity (% surface area) observed on each of the coral taxa arranged from no bleaching (c1) to full colony bleaching (c11), and expressed as a proportion of the total number of colonies analyzed resulting in a normalized 0–100 scale (McClanahan et al., 2004; Guest et al., 2016).
Results
Pre-and Post-warming Assessments of Coral Communities
A total of 2,288 still frames (covering ~1,200 m2 of reef) from PSRC reefs collected immediately following the warming event (August 2016) were analyzed for coral cover and coral community composition, and compared to existing pre-warming baseline data (2013/2014; n = 4,420). A total of 11,374 coral colonies were analyzed in 2016, with 24 coral genera identified. Average (± s.d.) coral cover and reef structural complexity (R) in 2016 were both high (coral cover = 55 ± 26%; median R = 3; Figure 4A; see Supplementary Figure S1) and showed no statistical difference (p > 0.05 for both) when compared to pre-warming coral community data from comparable reef habitats in 2013/2014 (coral cover = 48 ± 20%; median R = 3). Furthermore, no significant difference in coral assemblages was found between the time-series [one-way PERMANOVA: F(2, 362) = 22.8, p > 0.05; Figure 4B], with communities dominated by Montipora spp. (pre: 50 ± 22%; post: 50 ± 23%), Acropora spp. (pre: 14 ± 15%; post: 15 ± 14%), Turbinaria spp. (pre: 13 ± 23%; post: 8 ± 17%), and to a lesser extent Porites spp. (pre: 1.7 ± 4.9%; post: 0.9 ± 2.4%). Other less abundant coral taxa collectively accounted for only 2.6 ± 0.16% of average post-warming coral cover (see Figure 4B for coral inventory). Similar proportional contributions in terms of coral growth morphologies were also observed pre- and post-warming [one-way PERMANOVA: F(2, 362) = 62.3, p > 0.05]; the morphological classes examined included encrusting (pre: 38 ± 27%; post: 34 ± 34%), foliose (pre: 25 ± 25%; post: 27 ± 19%) and branching (pre: 10 ± 16%; post: 11 ± 18%) corals (Figure 4C). Collectively, these observations indicate no change in the prevalence of any coral genera as a result of the thermal stress experienced, and that the 2016 repeat survey data sufficiently captured the full extent of reef habitat types and coral communities at PSRC, thus providing a reliable basis from which to assess coral bleaching susceptibility.
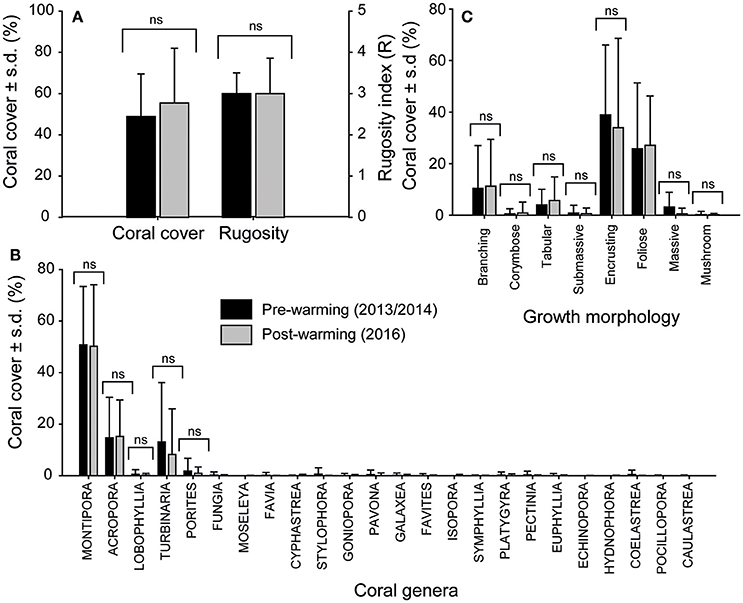
Figure 4. Pre- (black) and post-warming (gray) comparisons of coral assemblages at Paluma Shoals reef complex. Plots show: (A) average (±s.d.) coral cover and rugosity, (B) the proportion of coral growth morphologies within communities, and (C) the prevalence of different coral genera (24 identified) observed in both survey time-series (July 2013/2014 and August 2016). ns, no significant difference between surveys.
Prevalence of Coral Bleaching and Taxonomic Responses to Thermal Stress
Signs of bleaching were observed on only 173 coral colonies, or 1.5% of all colonies examined in our post-warming dataset. However, although evidence of thermal stress was observed on some colonies, the overall response by corals was very minor with only one individual colony (Lobophyllia sp.) exhibiting full bleaching (i.e., complete loss of zooxanthellae). This equates to only 0.008% of corals analyzed at PSRC. Therefore, any coral that showed visual signs of pigmentation loss (i.e., whitening) on the colony surface is referred to as “bleached” hereafter. On this basis, bleaching intensity (% of total tissue cover) on colonies was low, typically <20% (bleaching intensity: 0–10% = 64%; 11–20% = 21%), with only a small proportion of affected colonies (8.6%) exhibiting bleaching on >31% of their surface area (Figure 5). Although minor, the presence of bleaching clearly confirms that SSTs in the region of PSRC were above the thermal tolerance of some corals. Because of the timing of the surveys immediately following the period of high SSTs we were also able to assess recent coral death, and can report that no bleaching-related mortality occurred. Indeed, the most common bleaching response was focal bleaching, where multifocal patterns of bleaching occur scattered over the colony and with discrete borders adjacent to areas of healthy tissue being visible (Figure 3). This style of bleaching occurred on 70% of bleached colonies, and most commonly on foliose coral plates (e.g., Montipora) or massive colony ridges (Figure 5). Coral paling (15%) and partial colony bleaching (9%) was also observed but less commonly.
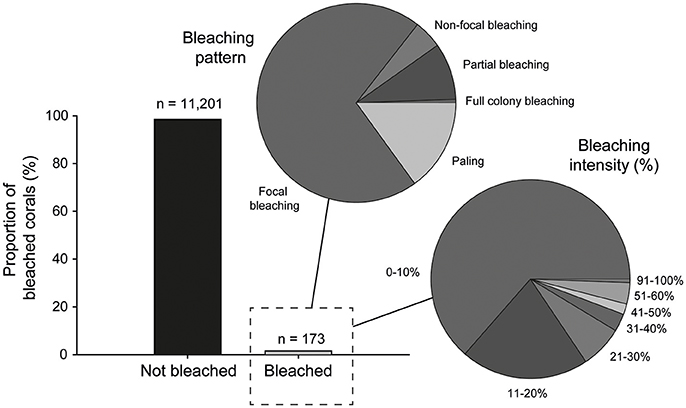
Figure 5. Prevalence of non-bleached (n = 11,201) and bleached corals (n = 173) observed during the August 2016 surveys of the Paluma Shoals reef complex. Pie graphs illustrate the proportion of the bleached colonies that exhibit the different bleaching patterns and intensity (% of total colony surface area). Note that overall bleaching is very minor and mostly expressed as focal bleaching on <20% of the colony surface.
Of the 24 coral genera identified on PSRC, 12 taxa showed some signs of bleaching (Table 1). Porites (40%), Montipora (36%) and Lobophyllia (9%) colonies were most commonly bleached. However, these values can be standardized to reflect the relative abundance of taxa on PSRC to calculate its bleaching index. This index provides a more appropriate estimate of a given coral taxa's response to heat stress than raw count values (see Table 1). The bleaching response of Montipora was very low (BI: 0.1), because although signs of bleaching were observed on a relatively high number of Montipora colonies (n = 64), Montipora is the most abundant genera and thus the relative impact on the total assemblage is negligible. In comparison, the Porites BI was relatively high (BI: 5.9), suggesting Porites are more susceptible to bleaching at these sites (Figure 3C). Acropora corals are common at PSRC, contributing to a high proportion (15 ± 14%) of the coral assemblage (Table 1). Perhaps most interestingly, Acropora are typically very susceptible to thermal stress on clear-water reefs (Marshall and Baird, 2000) but exhibited very low bleaching (n = 4; BI: 0.01) at PSRC. Several less abundant taxa also had high BI values (Coelastrea: 36; Favities: 21; Stylophora: 9.1; Lobophyllia: 5.1), but this was mostly expressed as paling of surface tissue (Figure 3F). Depth-analysis indicates that all corals that exhibited signs of thermal stress inhabited waters <2 m (Figure 6), despite coral growth extending to >4 m depth. Highest bleaching prevalence occurred within the 1–1.5 m depth zone (n = 96).
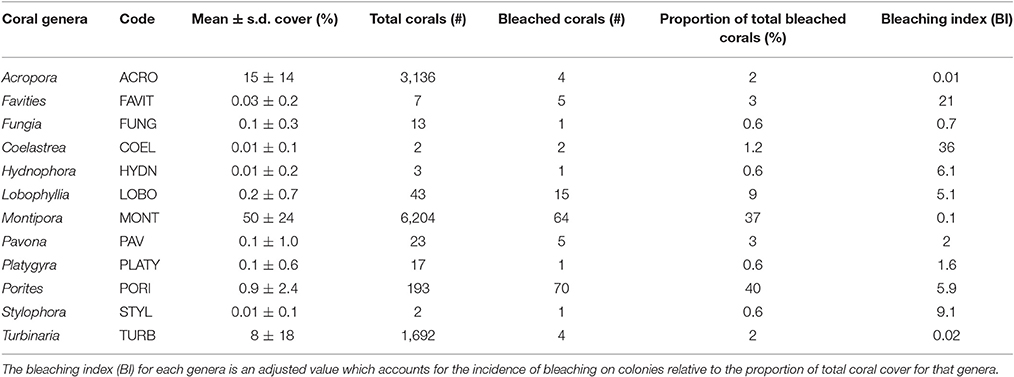
Table 1. Summary of taxa-specific responses to thermal stress of coral on Paluma Shoals reef complex following the 2016 ocean warming event.
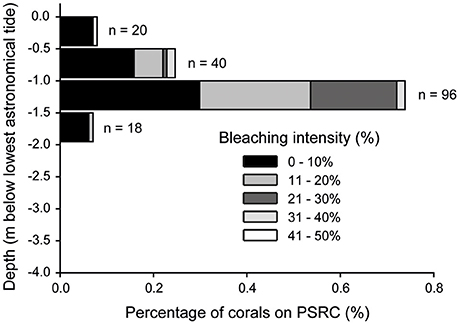
Figure 6. Incidences of coral bleaching with depth (0.5 m depth intervals below lowest astronomical tide) at Paluma Shoals reef complex in August 2016. Bleaching intensity of corals (% of total colony surface) is also shown within each depth category.
Discussion
The above-average SSTs that affected the GBR in 2015/2016 (see Supplementary Figure S2) caused widespread bleaching-related mortality on the remote northern GBR, and offshore reefs within the central GBR region (Hughes et al., 2017). Our findings suggest that nearshore coral communities on the central GBR experienced similar levels of heating, with water temperatures reaching up to 3°C above the mean monthly temperature, but were significantly more resilient to this heat stress with low incidences of minor bleaching observed (1.5% of corals). In the turbid nearshore setting of the PSRC, bleaching was mostly expressed as either partial/focal bleaching across <20% of the total colony surface area or as coral paling, and only one colony (Lobophyllia sp.) underwent complete pigmentation loss. Therefore, although signs of heat stress were visible on corals (Figure 3), confirming that water temperatures in the area did exceed the thermal tolerance of some coral taxa (Douglas, 2003), the overall stress responses exhibited by coral communities at this site were very minor. Importantly, similar high levels of heat stress occurred on both mid- and outer-shelf reefs within the central section of the GBR (compare nearshore and shelf-edge areas in the vicinity of Townsville in Supplementary Figure S2). However, coral bleaching and mortality in the central GBR varied markedly across the shelf, with bleaching-related mortality of up to 30% recorded at the clear-water shelf-edge sites (Great Barrier Reef Marine Park Authority, 2016; see Supplementary Figure S3), well above the very minor bleaching at the turbid-nearshore reefs we report here. Indeed, bleaching severity appears to have increased from inshore turbid-water sites to more optimal (clear-water) settings further offshore at the outer-shelf (Great Barrier Reef Marine Park Authority, 2016; see Supplementary Figure S3), despite thermal stress levels being at least as high (see Supplementary Figure S2) in the nearshore areas around PSRC. Furthermore, average coral cover on PSRC in 2016 remained high (55 ± 26%) with no significant change in cover observed between consecutive surveys indicating that no coral die-off occurred and that the 2016 thermal anomaly had no major impacts on the health of PSRC coral communities (see Supplementary Figure S1).
Arising from these observations is a simple question, why are incidences of coral bleaching so low within these high turbidity settings? Sedimentary processes associated within nearshore coastal settings play a critical role in not only determining the occurrence and extent of coral growth (Larcombe et al., 2001; Browne et al., 2010; Morgan et al., 2016a), but also in defining turbid-zone coral community structure and long-term rates and styles of vertical reef growth (Browne et al., 2013a; Perry et al., 2013; Morgan et al., 2016b; Johnson et al., 2017). High turbidity attenuates solar irradiance more rapidly than in clear-water settings resulting in light-limited growth conditions for corals (Larcombe et al., 1995; Storlazzi et al., 2015; Fabricius et al., 2016). Coral communities that inhabit low-light conditions have of late been increasingly discussed in the context of deep-water habitats along shelf edges (e.g., Bridge et al., 2011). However, they may also comprise a spatially important habitat type within shallow coastal areas on the GBR shelf. Although low light can be perceived as detrimental to coral growth, during lengthy periods of no cloud cover typical of El Niño events on the GBR, the buffering of UV by suspended sediment may have positively benefitted the coral assemblages by alleviating radiative stress (van Woesik et al., 2012). Comparable observations have been made with respect to cloud cover which have been demonstrated to reverse predictions of coral bleaching in statistical models throughout periods of large-scale basinal warming (Sheppard, 1999; Mumby et al., 2001). These same principles may apply to nearshore reefs on the GBR, in which the naturally turbid waters afford a similar degree of protection to corals (van Woesik et al., 2012; Cacciapaglia and van Woesik, 2015). Light measurements collected at PSRC under high (~80 mg l−1) and low turbidity (~10 mg l−1) scenarios (Figure 7) illustrate the rapid reduction in light that occurs over short depth intervals (<5 m). During high turbidity, 90% of light is attenuated by 2 m depth and 100% of light has been attenuated by 4 m depth. These sharp declines in light coincide with the observed lower limits of coral growth at PSRC and maximum photic depths within wider coastal environments on the GBR shelf (Fabricius et al., 2016). Importantly, maximum light penetration also directly relates to the depth limits of coral bleaching observed at PSRC (no bleaching was observed below 2 m depth). Indeed, modeled light attenuation curves using equations generated for clear water settings (see Storlazzi et al., 2015) indicate only a 33% reduction in light over a similar 5 m depth range (Figure 7). Collectively, these data may imply that SST was not the sole driver of the (albeit limited) bleaching observed at PSRC, and that the penetration of UVB may significantly compound conditions of increased SST. However, the lighter wind conditions associated with the 2016 El Niño means that sediment resuspension can be assumed to have been lower throughout the warming event, and thus despite these “less turbid” conditions, corals still maintained a high thermal tolerance to heat stress.
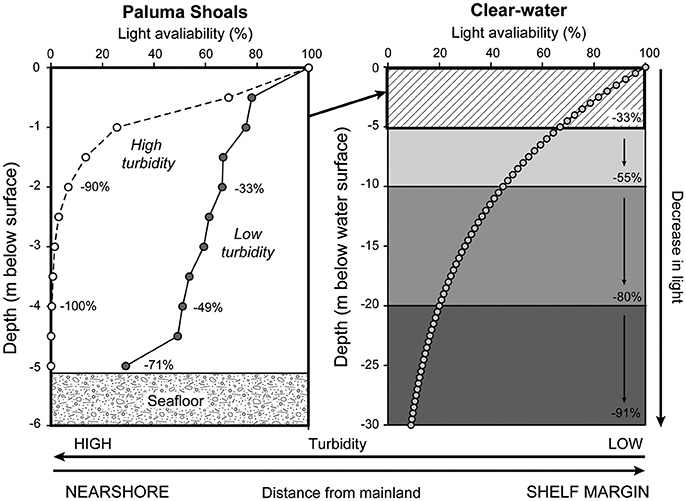
Figure 7. Left-hand plot shows light attenuation data (% decay from the water surface) collected at Paluma Shoals reef complex at 0.5 m depth intervals during low and high turbidity scenarios. Note the rapid reduction in light availability at 2 m depth under high turbidity. Right-hand plot shows modeled light attenuation curve using equations generated for clear water (see Storlazzi et al., 2015).
In this context, it is also pertinent to consider that the PSRC has been shown to support higher than average coral cover compared to clear-water settings on the GBR (Browne et al., 2010; Morgan et al., 2016a). The PSRC also comprises high abundances of coral taxa (e.g., Acropora) that have reportedly suffered catastrophic collapse from declining water quality at inshore (as opposed to nearshore) sites within Halifax Bay (e.g., Pelorus reef; Roff et al., 2013), and which are regarded as being highly susceptible to thermal bleaching (Brown, 1997; Marshall and Baird, 2000; Baker et al., 2008). Underpinning this tolerance is the ability of corals to utilize heterotrophic (e.g., plankton predation, dissolved and particulate organic matter consumption) feeding mechanisms as an alternate food source to offset reduced autotrophy (Anthony and Fabricius, 2000; Anthony et al., 2005; Tremblay et al., 2016). This capability may increase a corals resistance to bleaching by increasing zooxanthellae density and chlorophyll content, as well as their survivorship potential if bleaching does occur by improving the corals ability to reactivate normal nutrient exchanges (Tremblay et al., 2016; Lim et al., 2017). However, experimental studies report that not all coral species can increase their heterotrophic capacity in this way. For example, Montipora corals could meet more than 100% of their daily metabolic energy requirements by increasing their feeding rates and CHAR (per cent contribution of heterotrophically acquired carbon to daily animal respiration) during bleaching, whereas Porites corals could not (Grottoli et al., 2006). This indicates that corals with a high-CHAR capability (such as Montipora) may be more resilient to temperature-induced stress, a fact that may help to explain their dominance on reefs in marginal settings (Grottoli et al., 2006; Done et al., 2007; Browne et al., 2010; Morgan et al., 2016a). Our field surveys support these findings, as Montipora colonies are the principal coral taxa at PSRC (55% of total corals) highlighting the likely importance of heterotrophy in the success and persistence of certain turbid-zone corals.
As discussed above, corals that inhabit turbid (and low-light) nearshore settings, which have abundant particulate organic matter, may become permanently or facultatively heterotrophic (Anthony and Fabricius, 2000). The efficiency of this feeding strategy can surpass the daily energy requirements of corals as they are no longer limited to the same diurnal feeding patterns as autotrophs, and may feed more readily as food supply is not as predictable as in clear-water settings (Anthony, 2000, 2006). Excess energy can be stored as lipids within coral tissues, and may be critical in sustaining turbid-zone corals during stress events, whilst zooxanthellae are expelled, or when normal nutrient exchanges are altered (Tremblay et al., 2016; Lim et al., 2017). Importantly, such energy reserves could lead to an increase in the response time of corals to bleaching events (i.e., the time it takes for corals to bleach under elevated SSTs). The implications of this are that although turbid-zone coral bleaching could be similarly linked to the length and frequency of SST anomalies (much in the same way as their clear-water counterparts), the time-scales over which corals respond may differ and could ultimately influence their long-term resilience if their energy reserves can outlast periods of acute heat stress. As global ocean warming events are predicted to increase under future modeled climate scenarios, consecutive periods of heat stress could be problematic. If accumulated energy reserves within coral tissues cannot sustain corals throughout the duration of the heat stress, then turbid environments may not provide long-term refuges against climate-related disturbance despite exhibiting high single-event bleaching resistance. Our findings, which report low and less severe bleaching at PSRC than on clear-water reefs, provide an important contribution to the ongoing understanding of the turbid-reef refugia hypothesis, and run contrary to recent regional-scale predictive models of coral bleaching sensitivity (e.g., Wooldridge et al., 2017). However, whether the disparity in the bleaching response of corals between shelf settings is driven directly by the naturally high turbidity, or indirectly through prior acclimation of corals to marginal conditions requires further examination.
Variability in the response of certain coral taxonomic groups to thermal stress both on and between reefs are established, with past clear-water bleaching impact assessments reporting a high susceptibility of branching Acropora and Pocillopora corals to bleaching-related mortality, and a greater tolerance within massive and encrusting Porites and Faviids corals (Marshall and Baird, 2000; McClanahan et al., 2004; Great Barrier Reef Marine Park Authority, 2016; Hughes et al., 2017). Our data, however, show no significant changes in coral community composition following the 2016 temperature anomaly, although we do note taxa-specific patterns in the type and intensity of coral bleaching observed. Normally stress tolerant massive and submassive corals (e.g., Porites, Lobophyllia and Coelastrea) had the highest incidences of focal bleaching (typically on colony ridges or nubbins), and had relatively high BI values overall (Table 1). In contrast, shallow-water branching and tabular Acropora corals (<1.5 m depth) exhibited very low levels of bleaching. Such reversals in coral taxonomic susceptibility to thermal stress run contrary to a wide body of data from existing studies from clear-water reef settings, although these findings are similar to bleaching patterns documented within turbid water settings in Singapore, Malaysia and Indonesia following the 2010 global bleaching event (Guest et al., 2012, 2016). On these turbid Southeast Asian reefs, Porites suffered highest incidences of bleaching and Acropora corals were relatively unaffected. This is likely attributed to the fact that “normal” susceptibility is usually determined for autotrophic clear-water corals, and neglects to include these “atypical” coral communities which inhabit different environmental conditions and have a distinct physiology from corals in offshore settings. More recently, reports of coral bleaching within the turbid waters of NW Australia (located 10–15 km off the coast of Pilbara, near Onslow) in 2016 indicated significant bleaching of Turbinaria corals, another genera usually considered tolerant to higher temperature ranges (Lafratta et al., 2016). Lafratta et al. (2016) report no evidence for adaptation or acclimation of corals in Pilbara but recognize that other factors may complicate patterns. However, these Western Australian reefs are located much further offshore than the PSRC (in a comparable setting to Pelorus Reef on the Queensland coast) and therefore likely experience different background environmental conditions which may drive differences in coral thermal response. Regardless, the high variability in coral bleaching response both between and within clear-water and turbid settings highlights the greater need for more field investigations of marginal reef habitats.
Our findings demonstrate a high level of bleaching resistance to the 2016 warming episode, however, the impacts of consecutive warming events are less certain. For an unprecedented second time in 12 months, severe coral bleaching occurred on the GBR and was, this time, focused on the central third of the GBR (see Supplementary Figure S4). Increased efforts have been made to examine a number of inshore reefs, but these sites are still >18 km further offshore PSRC, and experience different environmental conditions from those very nearshore reefs (within <5 km of the mainland coast) that we present here. Therefore, the ongoing fate of turbid-zone coral communities in the region remains unknown and requires further long-term monitoring.
Conclusion
The magnitude and extent of the 2016 mass coral bleaching event that has affected large areas of the GBR demands that steps be taken to learn from it. The sporadic nature and difficulty associated with predicting these climate anomalies means that scientific assessments both during and following these events are critical to furthering our understanding of the response of coral reefs to global environmental change. Our assessment of nearshore reefs that incorporates substantial baseline information and extensive post-bleaching surveys of turbid-zone coral communities adds to the ongoing efforts to better understand these marginal reef types on the GBR. During the 2016 bleaching event, satellite-derived analysis of SST showed above-average temperatures across the wider central GBR. However, bleaching impacts varied from relatively “minor” at inshore sites to “severe” at outer-shelf reefs (Great Barrier Reef Marine Park Authority, 2016). Our results suggest even lower incidence of bleaching (0.008%) occurred on the most nearshore reefs, highlighting large cross-shelf gradients in bleaching prevalence which match well-documented changes in water quality and average coral cover for the region (Fabricius et al., 2016; Morgan et al., 2016a). Lowest incidences of bleaching were observed in shelf areas with poorest water clarity, but increased in more favorable clear-water settings. Therefore, prior acclimation of corals (both phenotypic and morphotypic) to acute thermal fluctuations may help them cope with episodic ocean warming. However, the mechanisms that influence coral bleaching thresholds are complex and remain poorly understood. Although turbid-zone corals at PSRC show high tolerance to single-event climate-related disturbance (2016), it is uncertain whether the resilience of corals will be long-lasting as an unprecedented consecutive bleaching event in 2017 has occurred.
Author Contributions
Project funding was secured by CP, KM, and SS. Field work and logistical support was carried out by KM, JJ, and SS. Data analysis was undertaken by KM and JJ. The manuscript was prepared by KM, CP, SS, and JJ. All authors gave their final approval for publication.
Funding
This work was funded by a UK Natural Environment Research Council Urgency Grant NE/P007694/1 to CP, KM, and SS.
Conflict of Interest Statement
The authors declare that the research was conducted in the absence of any commercial or financial relationships that could be construed as a potential conflict of interest.
The reviewer ER declared a past collaboration with one of the authors SS to the handling Editor, who ensured that the process met the standards of a fair and objective review.
Acknowledgments
We wish to acknowledge the support and assistance of the crew of the R.V. James Kirby research vessel.
Supplementary Material
The Supplementary Material for this article can be found online at: http://journal.frontiersin.org/article/10.3389/fmars.2017.00224/full#supplementary-material
References
Anthony, K. R. N. (2000). Enhanced particle-feeding capacity of corals on turbid reefs (Great Barrier Reef, Australia). Coral Reefs 19, 59–67. doi: 10.1007/s003380050227
Anthony, K. R. N. (2006). Enhanced energy status of corals on coastal, high-turbidity reefs. Mar. Ecol. Prog. Ser. 319, 111–116. doi: 10.3354/meps319111
Anthony, K. R. N., and Fabricius, K. E. (2000). Shifting roles of heterotrophy and autotrophy in coral energetics under varying turbidity. J. Exp. Mar. Biol. Ecol. 252, 221–253. doi: 10.1016/S0022-0981(00)00237-9
Anthony, K. R. N., Hoogenboom, M. O., and Connolly, S. R. (2005). Adaptive variation in coral geometry and the optimization of internal colony light climates. Funct. Ecol. 19, 17–26. doi: 10.1111/j.0269-8463.2005.00925.x
Baker, A., Glynn, P., and Riegl, B. (2008). Climate change and coral reef bleaching: an ecological assessment of long-term impacts, recovery trends and future outlook. Estuar. Coast. Shelf Sci. 80, 435–471. doi: 10.1016/j.ecss.2008.09.003
Beeden, R., Willis, B. L., Raymundo, L. J., Page, C. A., and Weil, E. (2008). Underwater cards for assessing coral health on Indo-Pacific reefs. Coral reef targeted research and capacity building for management program. Currie Commun. Melb. 22. Available online at: http://ccres.net/images/uploads/publications/8/0807_indo_pacific_underwater_id_cards.pdf
Bridge, T. C. L., Fabricius, K. E., Bongaerts, P., Wallace, C. C., Muir, P. R., Done, T. J., et al. (2011). Diversity of Scleractinia and Octocorallia in the mesophotic zone of the Great Barrier Reef, Australia. Coral Reefs 31, 179–189. doi: 10.1007/s00338-011-0828-1
Brown, B. E. (1997). Coral bleaching: causes and consequences. Coral Reefs 16, 129–138. doi: 10.1007/s003380050249
Browne, N. K. (2012). Spatial and temporal variations in coral growth on an inshore turbid reef subjected to multiple disturbances. Mar. Environ. Res. 77, 71–83. doi: 10.1016/j.marenvres.2012.02.005
Browne, N. K., Smithers, S. G., and Perry, C. T. (2010). Geomorphology and community structure of middle Reef, central great Barrier Reef, Australia: an inner-shelf turbid zone reef subject to episodic mortality events. Coral Reefs 29, 683–689. doi: 10.1007/s00338-010-0640-3
Browne, N. K., Smithers, S. G., and Perry, C. T. (2013a). Carbonate and terrigenous sediment budgets for two inshore turbid reefs on the central Great Barrier Reef. Mar. Geol. 346, 101–123. doi: 10.1016/j.margeo.2013.08.011
Browne, N. K., Smithers, S. G., and Perry, C. T. (2013b). Spatial and temporal variations in turbidity on two inshore turbid reefs on the Great Barrier Reef, Australia. Coral Reefs 32, 195–210. doi: 10.1007/s00338-012-0965-1
Bull, G. (1982). Scleractinian coral communities of two inshore high island fringing Reefs at Magnetic Island, North Queensland. Mar. Ecol. Prog. Ser. 7, 267–272. doi: 10.3354/meps007267
Butler, I. R., Sommer, B., Zann, M., Zhao, J. -X., and Pandolfi, J. M. (2013). The impacts of flooding on the high-latitude, terrigenoclastic influenced coral reefs of Hervey Bay, Queensland, Australia. Coral Reefs 32, 1149–1163. doi: 10.1007/s00338-013-1064-7
Cacciapaglia, C., and van Woesik, R. (2015). Reef-coral refugia in a rapidly changing ocean. Glob. Chang. Biol. 21, 2272–2282. doi: 10.1111/gcb.12851
De'ath, G., Fabricius, K. E., Sweatman, H., and Puotinen, M. (2012). The 27-year decline of coral cover on the Great Barrier Reef and its causes. Proc. Natl. Acad. Sci. U.S.A. 109, 17995–17999. doi: 10.1073/pnas.1208909109
DeCarlo, T. M., Cohen, A. L., Wong, G. T. F., Davis, K. A., Lohmann, P., and Soong, K. (2017). Mass coral mortality under local amplification of 2°C ocean warming. Sci. Rep. 7:44586. doi: 10.1038/srep44586
Done, T., Turak, E., Wakeford, M., DeVantier, L., McDonald, A., and Fisk, D. (2007). Decadal changes in turbid-water coral communities at Pandora Reef: loss of resilience or too soon to tell? Coral Reefs 26, 789–805. doi: 10.1007/s00338-007-0265-3
Douglas, A. E. (2003). Coral bleaching - How and why? Mar. Pollut. Bull. 46, 385–392. doi: 10.1016/S0025-326X(03)00037-7
Fabricius, K. E., Logan, M., Weeks, S. J., Lewis, S. E., and Brodie, J. (2016). Changes in water clarity in response to river discharges on the Great Barrier Reef continental shelf: 2002–2013. Estuar. Coast. Shelf Sci. 173, A1–A15. doi: 10.1016/j.ecss.2016.03.001
Fellegara, I., Baird, A. H., and Ward, S. (2013). Coral reproduction in a high-latitude, marginal reef environment (Moreton Bay, south-east Queensland, Australia). Invertebr. Reprod. Dev. 57, 219–223. doi: 10.1080/07924259.2012.752766
Garde, L. A., Spillman, C. M., Heron, S. F., and Beeden MAppSci, R. J. (2014). Reef temp next generation: a new operational system for monitoring reef thermal stress. J. Oper. Oceanogr. 7, 21–33. doi: 10.1080/1755876X.2014.11020150
Great Barrier Reef Marine Park Authority (2016). Interim Report: 2016 Coral Bleaching Event on the Great Barrier Reef. Available at online: http://elibrary.gbrmpa.gov.au/jspui/handle/11017/3044 (Accessed March 31, 2017).
Grottoli, A. G., Rodrigues, L. J., and Palardy, J. E. (2006). Heterotrophic plasticity and resilience in bleached corals. Nature 440, 1186–1189. doi: 10.1038/nature04565
Guest, J. R., Baird, A. H., Maynard, J. A., Muttaqin, E., Edwards, A. J., Campbell, S. J., et al. (2012). Contrasting patterns of coral bleaching susceptibility in 2010 suggest an adaptive response to thermal stress. PLoS ONE 7:e33353. doi: 10.1371/journal.pone.0033353
Guest, J. R., Low, J., Tun, K., Wilson, B., Ng, C., Raingeard, D., et al. (2016). Coral community response to bleaching on a highly disturbed reef. Sci. Rep. 6:20717. doi: 10.1038/srep20717
Hopley, D., Smithers, S. G., and Parnell, K. E. (2007). The Geomorphology of the Great Barrier Reef: Development, Diversity and Change. Cambridge: Cambridge University Press.
Hughes, T. P., Kerry, J., Álvarez-Noriega, M., Álvarez-Romero, J., Anderson, K., Baird, A., et al. (2017). Global warming and recurrent mass bleaching of corals. Nature 543, 373–377. doi: 10.1038/nature21707
Hughes, T. P., Rodrigues, M. J., Bellwood, D. R., Ceccarelli, D., Hoegh-Guldberg, O., McCook, L., et al. (2007). Phase shifts, herbivory, and the resilience of coral reefs to climate change. Curr. Biol. 17, 360–365. doi: 10.1016/j.cub.2006.12.049
Johnson, J. A., Perry, C. T., Smithers, S. G., Morgan, K. M., Santodomingo, N., and Johnson, K. G. (2017). Palaeoecological records of coral community development on a turbid, nearshore reef complex: baselines for assessing ecological change. Coral Reefs. doi: 10.1007/s00338-017-1561-1. [Epub ahead of print].
Kleypas, J. (1996). Coral reef development under naturally turbid conditions: fringing reefs near broad sound, Australia. Coral Reefs 78, 153–167. doi: 10.1007/BF01145886
Kleypas, J. A., McManus, J. W., and Meñez, L. A. B. (1999). Environmental limits to coral reef development: where do we draw the line? Am. Zool. 39, 146–159.
Lafratta, A., Fromont, J., Speare, P., and Schönberg, C. H. L. (2016). Coral bleaching in turbid waters of north-western Australia. Mar. Freshw. Res. 68, 65–75. doi: 10.1071/mf15314
Larcombe, P., and Carter, R. (2004). Cyclone pumping, sediment partitioning and the development of the Great Barrier Reef shelf system: a review. Quat. Sci. Rev. 23, 107–135. doi: 10.1016/j.quascirev.2003.10.003
Larcombe, P., Costen, A., and Woolfe, K. J. (2001). The hydrodynamic and sedimentary setting of nearshore coral reefs, central Great Barrier Reef shelf, Australia: Paluma Shoals, a case study. Sedimentology 48, 811–835. doi: 10.1046/j.1365-3091.2001.00396.x
Larcombe, P., Ridd, P. V., Prytz, A., and Wilson, B. (1995). Factors controlling suspended sediment on inner-shelf coral reefs, Townsville, Australia. Coral Reefs 14, 163–171. doi: 10.1007/BF00367235
Larcombe, P., and Woolfe, K. J. (1999). Increased sediment supply to the Great Barrier Reef will not increase sediment accumulation at most coral reefs. Coral Reefs 18, 163–169. doi: 10.1007/s003380050174
Lim, C. S., Bachok, Z., and Hii, Y. S. (2017). Effects of supplementary polyunsaturated fatty acids on the health of the scleractinian coral Galaxea fascicularis (Linnaeus, 1767). J. Exp. Mar. Biol. Ecol. 491, 1–8. doi: 10.1016/j.jembe.2017.02.009
Liu, G., Strong, A., and Skirving, W. (2006). “Overview of NOAA coral reef watch program's near-real time satellite global coral bleaching monitoring activities,” in Proceedings of the 10th International Coral Reef Symposium (Okinawa).
Marshall, P. A., and Baird, A. H. (2000). Bleaching of corals on the Great Barrier Reef: differential susceptibilities among taxa. Coral Reefs 19, 155–163. doi: 10.1007/s003380000086
McClanahan, T. R., Baird, A. H., Marshall, P. A., and Toscano, M. A. (2004). Comparing bleaching and mortality responses of hard corals between southern Kenya and the Great Barrier Reef, Australia. Mar. Pollut. Bull. 48, 327–335. doi: 10.1016/j.marpolbul.2003.08.024
Morgan, K. M., Perry, C. T., Smithers, S. G., Johnson, J. A., and Daniell, J. J. (2016a). Evidence of extensive reef development and high coral cover in nearshore environments: implications for understanding coral adaptation in turbid settings. Sci. Rep. 6:29616. doi: 10.1038/srep29616
Morgan, K. M., Perry, C. T., Smithers, S. G., Johnson, J. A., and Gulliver, P. (2016b). Transitions in coral reef accretion rates linked to intrinsic ecological shifts on turbid-zone nearshore reefs. Geology 44, 995–998. doi: 10.1130/G38610.1
Mumby, P. J., Chisholm, J. R. M., Edwards, A. J., Andrefouet, S., and Jaubert, J. (2001). Cloudy weather may have saved society Island reef corals during the 1998 ENSO event. Mar. Ecol. Prog. Ser. 222, 209–216. doi: 10.3354/meps222209
Palmer, S. E., Perry, C. T., Smithers, S. G., and Gulliver, P. (2010). Internal structure and accretionary history of a nearshore, turbid-zone coral reef: Paluma Shoals, central Great Barrier Reef, Australia. Mar. Geol. 276, 14–29. doi: 10.1016/j.margeo.2010.07.002
Perry, C. T., and Morgan, K. M. (2017). Bleaching drives collapse in reef carbonate budgets and reef growth potential on southern Maldives reefs. Sci. Rep. 7:40581. doi: 10.1038/srep40581
Perry, C. T., Smithers, S. G., and Gulliver, P. (2013). Rapid vertical accretion on a “young” shore-detached turbid zone reef: offshore Paluma Shoals, central Great Barrier Reef, Australia. Coral Reefs 32, 1143–1148. doi: 10.1007/s00338-013-1063-8
Perry, C. T., Smithers, S. G., and Johnson, K. G. (2009). Long-term coral community records from lugger shoal on the terrigenous inner-shelf of the central Great Barrier Reef, Australia. Coral Reefs 28, 941–948. doi: 10.1007/s00338-009-0528-2
Potts, D., and Jacobs, J. (2000). “Evolution of reef-building scleractinian corals in turbid environments: a paleo-ecological hypothesis,” Proceedings of Ninth International Coral Reef Symposium, vol. 1 (Bali), 249–254.
Richards, Z. T., Garcia, R. A., Wallace, C. C., Rosser, N. L., and Muir, P. R. (2015). A diverse assemblage of reef corals thriving in a dynamic intertidal reef setting (Bonaparte Archipelago, Kimberley, Australia). PLoS ONE 10:e0117791. doi: 10.1371/journal.pone.0117791
Roche, R. C., Perry, C. T., Johnson, K. G., Sultana, K., Smithers, S. G., and Thompson, A., a. (2011). Mid-Holocene coral community data as baselines for understanding contemporary reef ecological states. Palaeogeogr. Palaeoclimatol. Palaeoecol. 299, 159–167. doi: 10.1016/j.palaeo.2010.10.043
Roff, G., Clark, T. R., Reymond, C. E., Zhao, J. X., Feng, Y., McCook, L. J., et al. (2013). Palaeoecological evidence of a historical collapse of corals at Pelorus Island, inshore Great Barrier Reef, following European settlement. Proc. R. Soc. Lond. B Biol. Sci. 280:20122100. doi: 10.1098/rspb.2012.2100
Rosen, B., Aillud, G., and Bosellini, F. (2000). “Platy coral assemblages: 200 million years of functional stability in response to the limiting effects of light and turbidity,” in Proceedings 9th International Coral Reef Symposium, Vol. 1 (Bali), 255–264.
Ryan, E., Smithers, S., and Lewis, S. (2016). Chronostratigraphy of Bramston Reef reveals a long-term record of fringing reef growth under muddy conditions in the central Great Barrier Reef. Palaeogeogr. Palaeoclimatol. Palaeoecol. 441, 734–747. doi: 10.1016/j.palaeo.2015.10.016
Santodomingo, N., Renema, W., and Johnson, K. G. (2016). Understanding the murky history of the coral triangle: miocene corals and reef habitats in East Kalimantan (Indonesia). Coral Reefs. 35, 765–781. doi: 10.1007/s00338-016-1427-y
Sheppard, C. (1999). Coral decline and weather patterns over 20 years in the Chagos Archipelago, Central Indian Ocean. Ambio 28, 472–478.
Smithers, S., and Larcombe, P. (2003). Late Holocene initiation and growth of a nearshore turbid-zone coral reef: Paluma Shoals, central Great Barrier Reef, Australia. Coral Reefs 22, 499–505. doi: 10.1007/s00338-003-0344-z
Storlazzi, C. D., Norris, B. K., and Rosenberger, K. J. (2015). The influence of grain size, grain color, and suspended-sediment concentration on light attenuation: why fine-grained terrestrial sediment is bad for coral reef ecosystems. Coral Reefs 34, 967–975. doi: 10.1007/s00338-015-1268-0
Tremblay, P., Gori, A., Maguer, J. F., Hoogenboom, M., and Ferrier-Pagès, C. (2016). Heterotrophy promotes the re-establishment of photosynthate translocation in a symbiotic coral after heat stress. Sci. Rep. 6:38112. doi: 10.1038/srep38112
van Woesik, R., Houk, P., Isechal, A. L., Idechong, J. W., Victor, S., and Golbuu, Y. (2012). Climate-change refugia in the sheltered bays of Palau: analogs of future reefs. Ecol. Evol. 2, 2474–2484. doi: 10.1002/ece3.363
Wolanski, E., Fabricius, K., Spagnol, S., and Brinkman, R. (2005). Fine sediment budget on an inner-shelf coral-fringed island, Great Barrier Reef of Australia. Estuar. Coast. Shelf Sci. 65, 153–158. doi: 10.1016/j.ecss.2005.06.003
Wooldridge, S. A., Heron, S. F., Brodie, J. E., Done, T. J., Masiri, I., and Hinrichs, S. (2017). Excess seawater nutrients, enlarged algal symbiont densities and bleaching sensitive reef locations: 2. a regional-scale predictive model for the Great Barrier Reef, Australia. Marine Poll. Bull. 114, 343–354. doi: 10.1016/j.marpolbul.2016.09.045
Keywords: turbid-zone, coral bleaching, Great Barrier Reef, refugia hypothesis, environmental change, turbidity
Citation: Morgan KM, Perry CT, Johnson JA and Smithers SG (2017) Nearshore Turbid-Zone Corals Exhibit High Bleaching Tolerance on the Great Barrier Reef Following the 2016 Ocean Warming Event. Front. Mar. Sci. 4:224. doi: 10.3389/fmars.2017.00224
Received: 05 May 2017; Accepted: 06 July 2017;
Published: 19 July 2017.
Edited by:
Emma Camp, University of Technology, Sydney, AustraliaReviewed by:
Michael O'Leary, Curtin University, AustraliaChristian Grenz, Mediterranean Institute of Oceanography, France
Emma Jean Ryan, University of Auckland, New Zealand
Copyright © 2017 Morgan, Perry, Johnson and Smithers. This is an open-access article distributed under the terms of the Creative Commons Attribution License (CC BY). The use, distribution or reproduction in other forums is permitted, provided the original author(s) or licensor are credited and that the original publication in this journal is cited, in accordance with accepted academic practice. No use, distribution or reproduction is permitted which does not comply with these terms.
*Correspondence: Kyle M. Morgan, ay5tb3JnYW5AZXhldGVyLmFjLnVr
Chris T. Perry, Yy5wZXJyeUBleGV0ZXIuYWMudWs=