- 1Program in Fisheries and Aquatic Sciences, School of Forest Resources and Conservation, Institute of Food and Agricultural Sciences, University of Florida, Gainesville, FL, United States
- 2School of Biological Sciences, University of Essex, Essex, United Kingdom
- 3Climate Change Cluster, University of Technology Sydney, Ultimo, NSW, Australia
- 4School of Mathematical and Physical Sciences, University of Technology Sydney, Ultimo, NSW, Australia
Coral communities are increasingly found to populate non-reef habitats prone to high environmental variability. Such sites include seagrass meadows, which are generally not considered optimal habitats for corals as a result of limited suitable substrate for settlement and substantial diel and seasonal fluctuations in physicochemical conditions relative to neighboring reefs. Interest in understanding the ability of corals to persist in non-reef habitats has grown, however little baseline data exists on community structure and recruitment of scleractinian corals in seagrass meadows. To determine how corals populate seagrass meadows, we surveyed the established and recruited coral community over 25 months within seagrass meadows at Little Cayman, Cayman Islands. Simultaneous surveys of established and recruited coral communities at neighboring back-reef sites were conducted for comparison. To fully understand the amount of environmental variability to which corals in each habitat were exposed, we conducted complementary surveys of physicochemical conditions in both seagrass meadows and back-reefs. Despite overall higher variability in physicochemical conditions, particularly pH, compared to the back-reef, 14 coral taxa were capable of inhabiting seagrass meadows, and multiple coral families were also found to recruit to these sites. However, coral cover and species diversity, richness, and evenness were lower at sites within seagrass meadows compared to back-reef sites. Although questions remain regarding the processes governing recruitment, these results provide evidence that seagrass beds can serve as functional habitats for corals despite high levels of environmental variability and suboptimal conditions compared to neighboring reefs.
Introduction
Scleractinian corals occupy a range of habitat types and form the architectural framework within different reef zones (e.g., Goreau, 1959; Logan, 2013). However, corals also colonize non-reef habitats, such as mangrove systems and seagrass beds that can exhibit high levels of variability in abiotic conditions relative to neighboring reefs (e.g., Riegl, 1999; Perry and Larcombe, 2003; Yates et al., 2014; Camp et al., 2016a). Despite the presence of corals in these non-reef habitats, many assessments of coral communities focus only on fore-reef zones (e.g., Mumby et al., 2007; Castillo et al., 2012). Few studies exist to characterize coral populations outside such classical reef systems.
To understand the importance of non-reef habitats for corals, it is critical to establish baseline data on the structure of coral assemblages in such habitats. Habitat monitoring and assessment data is widely acknowledged as a critical factor in the success of conservation initiatives and adaptive management (e.g., Jackson et al., 2014). However, prioritizing indicators for monitoring ecosystem health can be challenging (Borja et al., 2014). Live coral cover alone is increasingly acknowledged as an insufficient metric for characterizing the ability of a coral-bearing habitat to persist over time (Hughes et al., 2010). For example, recruitment is recognized as a valuable metric for evaluating coral community function, as it can provide insight into a system's capacity for replenishment, a critical component of resilience (Nyström et al., 2008; Hughes et al., 2010). A holistic examination of a coral community should therefore characterize both established and recruited coral assemblages.
Here we focus on seagrass beds that, to-date, have been understudied with respect to their suitability as a non-reef coral habitat. Typically, seagrass beds have not been considered important coral habitats due to a lack of solid substrate (Jackson, 1986; Manzello et al., 2012). Coral communities inhabiting seagrass meadows have been reported from both the Caribbean (Rützler and Macintyre, 1982; Johnson, 1992) and Indo-Pacific (Higuchi et al., 2014; Camp et al., 2016a). These studies have focused on the ecology of free-living coral species (Johnson, 1992) and physiology of corals (Higuchi et al., 2014; Camp et al., 2016a,b) in such habitats, or have described coral colonies in seagrass meadows as part of larger reef systems (Rützler and Macintyre, 1982). Johnson (1992) investigated coral recruitment within a seagrass habitat, however this study focused on a single coral species rather than the coral community as a whole. Others have briefly noted the presence of corals living among seagrass during unrelated experiments (e.g., Wulff, 2008).
For coral communities in seagrass beds, measurements of physicochemical conditions are important given the characteristic variability in ocean chemistry associated with these sites (Higuchi et al., 2014; Camp et al., 2016a). Seagrass systems routinely experience highly variable environmental conditions relative to coral reefs, such as reduced pH and increased temperature (Semesi et al., 2009; Challener et al., 2016), which could be critical in shaping resident coral communities. Studies of corals living among these high-variability systems can therefore also provide insight into which coral species are able to successfully acclimatize or adapt to survive under such conditions (Barshis, 2015). We are unaware of any study that has comprehensively characterized coral community structure within a seagrass meadow, particularly in the context of adjacent reef communities and the physicochemical variability within the system. A lack of baseline data on the composition and condition of coral communities in seagrass meadows inhibits our ability to understand how these systems may change over time.
We conducted a 25-month study to characterize the coral community at understudied seagrass sites in a location with minimal direct anthropogenic impact (Little Cayman, Cayman Islands). To fully understand how coral communities in seagrass meadows differ from those of local reefs, adjacent back-reefs were also surveyed for comparison. We assessed key attributes to provide a robust characterization of each site, specifically: (i) physicochemical conditions, (ii) benthic structure, and (iii) coral recruitment, including the relationship between the established and recruited coral community. Our study thus provides detailed insight into an important but poorly understood coral habitat, as well as a comprehensive comparison to an adjacent but very different shallow reef habitat. In doing so, we provide novel information on how environmentally-variable seagrass beds can function as a coral habitat.
Methods
Study Site
This study was conducted off the north coast of Little Cayman, Cayman Islands (19.6897°N, 80.0367°W), at three shallow (≤ 2 m) sites with adjacent back-reefs and seagrass meadows (Figure 1). The first site was located in Grape Tree Bay, (GTB), a lagoon approximately 0.10 km wide and 1.50 km long, situated within the Bloody Bay Marine Park. The two remaining sites were located at either end of the larger Mary's Bay, approximately 0.15 km wide and 4.00 km long. The two sites within Mary's Bay were designated as Mary's Bay West (MBW) and Mary's Bay East (MBE). At each site, three sampling replicates, spaced 50 m apart, were selected within each habitat type [i.e., seaward back-reef (n = 3) and inshore seagrass meadow (n = 3); Figure 1]. Across sites, seagrass was comprised of 88.0 ± 1.5% Thalassia testudinum and 12 ± 0.6% Syringodium filiforme.
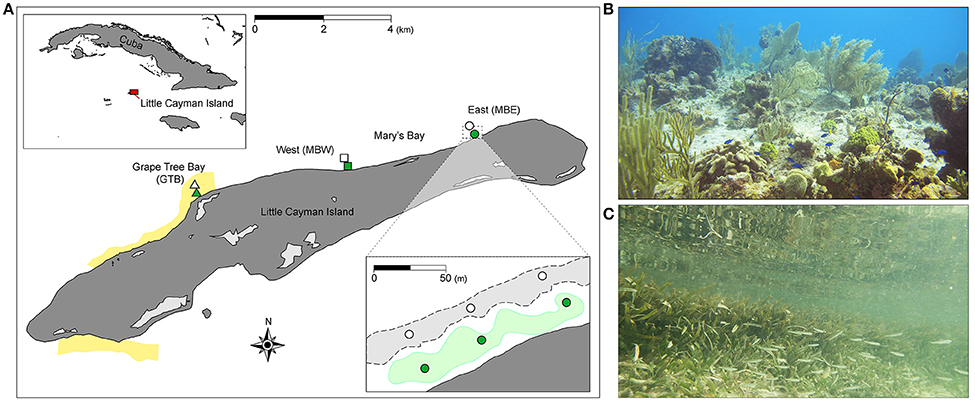
Figure 1. Study sites on Little Cayman Island. Map of Little Cayman Island (A) located within the Caribbean Sea (upper inset), yellow highlighting denotes the location of marine parks. Three locations, Mary's Bay East (MBE, circles) and West (MBW, squares), and Grape Tree Bay (GTB, triangles) were chosen as study sites. Back-reef (light gray areas with dashed lines, lower inset) and seagrass (green areas, lower inset) sites were selected within each study location (n = 3 per site spaced 50 m apart, lower inset). Typical benthic scenes are indicated for back-reef and seagrass sites in (B,C), respectively. The base data for the map (A) were collected from www.openstreetmap.org (© OpenStreetMap contributors) under the Open Database License and customized in Adobe Illustrator (version 16).
Physicochemical Conditions
To characterize the physicochemistry of each site, discrete seawater samples were collected over two 6-week periods, one in the wet season (July-August) and one in the dry season (February–March) of 2012. Within each sampling period, three 24 h sampling sessions were conducted during both neap and spring tidal phases. Samples were collected every 3 h over the 24 h period at each site and replicate (n = 48 total samples per site, per sampling session). In addition, diurnal (1 h before sunrise and 1 h after sunset) open-ocean water samples were collected (n = 6 samples per site) to act as an off-shore reference for the ocean chemistry. Discrete water samples were collected from a depth of ca. 0.5 m following Carbon Dioxide Information Analysis Center (CDIAC) standards. Samples were collected in borosilicate bottles and fixed with HgCl2 [0.05 ml of saturated (aq) solution] (Dickson et al., 2007). Stoppers were inserted to ensure a gas tight seal and samples were stored in the dark until they were returned to the laboratory (within 30 min) for analysis.
An additional seawater sample was collected at each replicate during every sampling period to allow immediate measurements of pH, temperature, oxygen, and salinity to obtain the in situ conditions used for calculation of carbonate parameters. An Orion™ 5 Star meter (Thermo Scientific™) fitted with a pH/temperature probe and DO probe (Thermo Scientific™) and a handheld refractometer to measure salinity (ExTech®) were used throughout. The pH probe was calibrated with TRIS buffer using the potentiometric technique and the total scale (pHT). Water flow was also measured at each replicate following every sample collection using a mechanical flow meter (General Oceanics). All laboratory and field equipment were calibrated as recommended by their instruction manuals and/or CDIAC protocols (Dickson et al., 2007).
Samples were returned to a climate-controlled laboratory where pH was re-measured to check for pH drift using a pH electrode (Thermo Scientific™) calibrated with TRIS buffer using the potentiometric technique and the total scale (pHT). An open-cell potentiometric titration procedure was used to measure total alkalinity (AT) using the Gran method to determine the second end point of the carbonate system. AT of all samples was determined using a titrator (Metrohm) with an accuracy and precision of ca. ≤ 2 μmol kg−1 as verified with certified reference materials distributed by A. Dickson (Scripps Institution of Oceanography). All carbonate parameters [pCO2, TCO2 and aragonite saturation (Ωarg)] were calculated with CO2SYS from AT and pHT (Riebesell et al., 2000), and in situ temperature, salinity, and sampling depth (m) as a proxy for pressure (Lewis and Wallace, 1998). For CO2SYS the dissociation constants provided by Mehrbach et al. (1973) were used for carbonic acid (as refined by Dickson and Millero, 1987), and those provided by Dickson (1990) were used for boric acid. In addition, HOBO® data loggers (Onset® Computer Corporation) were used to measure light (in lux subsequently converted to PAR, see Long et al., 2012) in the back-reefs and seagrass meadows for a period of 25-months beginning in January 2012. Loggers were screwed onto a PVC mount installed at each site using a galvanized nail and marine epoxy. Loggers were cleaned and data was downloaded monthly in situ using a HOBO® Waterproof Shuttle (Onset® Computer Corporation). Fouling on the loggers was found to alter light quality after the first 10 days following cleaning, therefore only light data collected within these 10 day periods were analyzed (n = 250 days of light data for the full 25-month experiment).
Benthic Surveys
Benthic surveys were conducted in February 2012 and February 2013. To characterize the benthos of the back-reef and seagrass meadow, continuous-line intercept video transects were conducted using a high-definition video camera with an underwater housing (Canon®). At each site and habitat type, three 50 m transects were laid parallel to shore. The start and end points of each transect were recorded using a handheld GPS (Garmin®) and marked in situ using semi-permanent metal pickets. Benthic composition was quantified using categories defined by Atlantic and Gulf Rapid Reef Assessment protocol version 5.4 (Lang et al., 2010), and scleractinian corals were identified to species level.
The size-frequency, density, and health of established scleractinian coral populations were also assessed within each habitat. Established coral populations refer to all coral colonies identified on natural substratum within each habitat (e.g., not recruited on to settlement tiles, see proceeding section). A 20 m2 (5 × 4 m) quadrat was placed at the start point of each transect, and species, size, and condition of each coral (≥1 cm) was recorded. Colonies were measured using calipers or measuring tape. Colony condition characteristics recorded included depigmentation and evidence of new, transitional, or old mortality (as defined by Lang et al., 2010).
Recruitment
Within this study, recruited coral populations refer to corals recruited onto settlement arrays. Settlement arrays were deployed in January 2012 to assess scleractinian coral recruitment patterns within each habitat type. Pre-conditioned limestone settlement tiles were used, as they have demonstrated coral recruitment rates comparable to natural substrate (e.g., Salinas de León et al., 2011). Arrays consisted of six unglazed limestone tiles (10 × 10 × 1 cm) with 0.8 cm holes drilled in the center (modified from Mundy, 2000; Salinas de León et al., 2011). Each tile was labeled for identification and attached to a PVC base with a plastic cable tie. Tiles were conditioned for 1 month in their respective target habitats in Grape Tree Bay prior to installation. Arrays were installed using galvanized nails through either end of the PVC base. The PVC base created a 2.5 cm gap between the tiles and the substrate, which has been shown to facilitate coral settlement (Harriott and Fisk, 1987). Three settlement arrays were deployed at every sampling replicate at each of the three study sites (n = 18 tile arrays per site, n = 54 arrays (324 tiles) total). Arrays were spaced a minimum of 5 m apart (Salinas de León et al., 2011).
Tiles two, three, and six (selected at random prior to deployment) were removed and replaced with new, conditioned tiles 1 month following installation and monthly thereafter. Tile removal allowed for the timing and frequency of recruitment to be assessed. Removed tiles were examined microscopically (20x) in the laboratory under blue light (Leica Microsystems). Tiles with coral recruits present were laid in a single layer in a bath treated with 10% aq chlorine solution for 48 h to remove organic matter and examined with the microscope (per Schmidt-Roach et al., 2008). Recruits belonging to the families Acroporidae, Agariciidae, Faviidae (now part of family Mussidae, see Budd et al., 2012), Poritidae, and Siderastreidae were identified using photomicrographs published previously (English et al., 1994; Babcock et al., 2003; de Putron, 2005, 2007). Recruits belonging to other families (for which photomicrographs were unavailable), or those that could not be identified, were recorded as other (per Schmidt-Roach et al., 2008). All tile arrays were removed after 25 months and analyzed following the same protocol as the monthly assessments.
Statistical Analysis
Mean and coefficient of variation (CV) of each abiotic factor (i.e. pHT, temperature, AT, Ωarg, salinity, and water velocity) were compared between back-reefs and seagrass meadows and relative to the open-ocean sites using ANOVA and post hoc Tukey tests. Benthic structure of each habitat was compared between years using a Principal Component Analysis (PCA), with unpaired t-tests on the first and second principal components extracted for each site. Data were square-root transformed and clusters (80 and 90% similarity) were generated from a Bray-Curtis similarity matrix of all benthic data. The PCA vectors were generated by Pearson's Correlations exceeding R > 0.6, between plot ordinations and benthic categories. To characterize the established coral populations of each habitat, coral abundance, disease prevalence, species richness, diversity (Shannon-Wiener Index) and evenness (Shannon's Evenness) were calculated. The abundance of established corals per year, for each site, were compared by a 2 × 2 contingency table and a Pearson's Chi-squared test. Size-frequency of the established coral communities of each habitat was binned into size categories; larger categories were pooled together to avoid the problems associated with low expected counts (Starnes et al., 2012). The maximum diameter for each colony was used for comparison to ensure standardization among colonies with different growth forms (per Suggett et al., 2012). Fits of exponential decay across the counts in each bin were performed for both 2012 and 2013 to assess the population size-classes (Santangelo et al., 2004). The log count in each bin was plotted against the middle size category, with fits only done on categories until the first zero bin was reached; thus, avoiding issues with non-defined logarithms. Population sizes between years, for each habitat, were also assessed initially by a 2 × 2 × 5 contingency table and then with Pearson's chi-squared test.
To analyze recruitment, all tile data was pooled and considered as a mean per site (see Hurlbert, 1984). The density of recruits was determined per family. For both the recruited coral population (determined from settlement tiles) and established coral populations (determined from the quadrat surveys), the proportional abundance of each species was determined for the back-reef and seagrass meadow habitats. Differences between the established and recruited coral populations were assessed through the z-test. Data was pooled from the two communities to obtain a pooled sample abundance of:
where prec is the sample proportion for the recruited population, Nrec is the sample size for the recruited population, pest is the sample proportion for the established population, and Nest is the sample size for the established population.
With a pooled standard error of:
Using test statistic , p-values were calculated for each family for the null hypothesis that the recruited and established coral population proportions were equal. At the end of the study, the recruited benthic taxa were determined by the CPCe analysis and compared between habitat types (n = 9 replicates per habitat type) using MANOVA with post-hoc Tukey tests. Statistical analysis was performed using R (R Core Team, 2014) and Graphpad Prism (version 7.01). Data were assessed and found to meet the assumptions of linearity, independence, homogeneity of variance, and normality.
Results
Physicochemical Conditions
Both the back-reef and seagrass meadow sites had significantly greater variability (CV) in abiotic conditions compared to the open-ocean sites (Tables 1, 2). With the exception of salinity, all mean abiotic variables were also different between the seagrass meadows and back-reefs compared to the open-ocean reference (Tables 1, 2). Specifically, mean pHT was elevated at both of the inshore habitats (back-reef = 8.17 ± 0.01 and seagrass meadow = 8.16 ± 0.01) relative to the open-ocean (8.12 ± 0.01), irrespective of season or phase of the lunar cycle (Tables 1, 2). The elevation in mean pHT and corresponding depletion in pCO2 was significant enough to elevate mean Ωarg in the seagrass meadow and back-reef habitats relative to the open-ocean sites [F(2, 105) = 166.45, p < 0.001, Tables 1, 2].
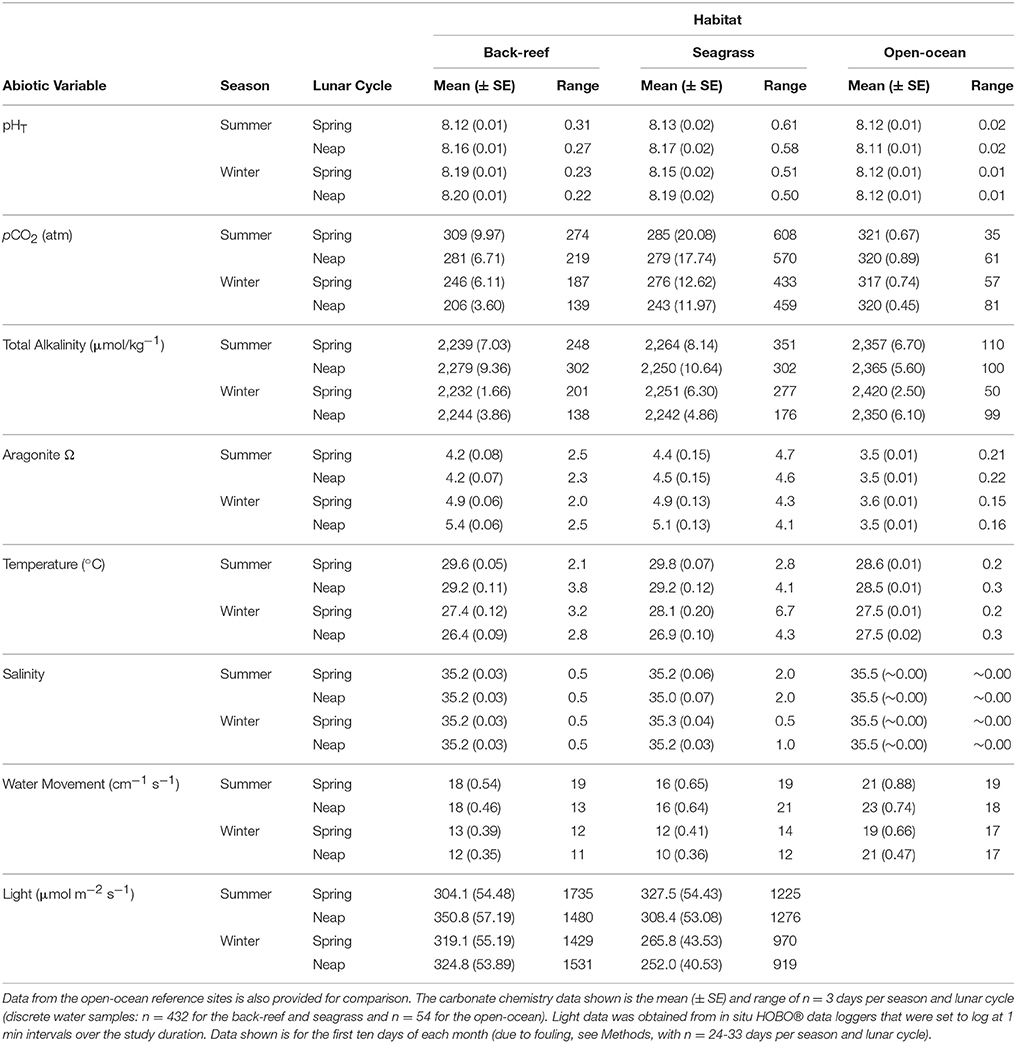
Table 1. Physicochemical data for the seagrass and back-reef habitats on Little Cayman, Cayman Islands, British West Indies.
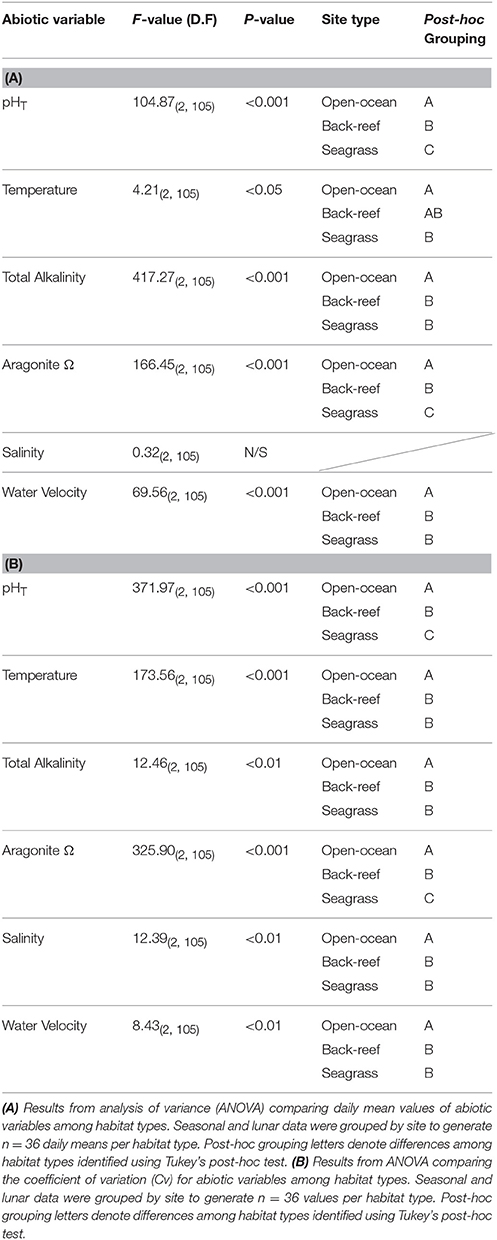
Table 2. Statistical results for comparisons of abiotic variables among the back-reef and seagrass habitat types and relative to the open-ocean reference sites.
The seagrass meadows had greater variability in environmental conditions across the measured parameters than the back-reefs (see Table 1), with pHT the most variable condition measured. Specifically, the pHT range in the seagrass meadows over diurnal (0.51 ± 0.07), seasonal (0.62 ± 0.03) and lunar cycle (0.58 ± 0.04) time scales was ca. double that of the back-reefs (diurnal 0.22 ± 0.02, seasonal 0.29 ± 0.04, lunar 0.24 ± 0.05). Both habitats experienced a greater range of pHT conditions during the summer season and on spring tides (Table 1), with the seagrass meadows reaching a maximum pHT of 8.28 ± 0.02 around mid-day and a low of 7.80 ± 0.01 at night (Figure 2). Oxygen co-varied with pH, with maximum levels experienced in the day (7.11 ± 0.2) and lowest levels occurring at night (4.6 ± 0.1). Mean values of temperature, light, AT, salinity, and water velocity did not differ between the back-reefs and seagrass meadows (Table 1).
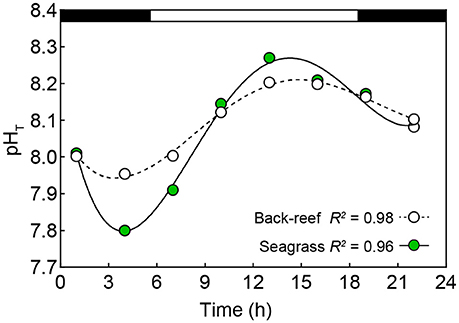
Figure 2. Mean (±SE, note: not visible below symbols) pHT (total scale) values for the back-reef (open symbols) and seagrass habitats (green symbols) obtained at eight-time points over a full night (black horizontal bar) and day (white horizontal bar) cycle (discrete water samples: n = 432). R2 produced by fitting the data to a fourth order polynomial function (Y = a + bx + cx2 + dx3 + ex4).
Benthic Structure
In the seagrass meadows, benthic cover was primarily photoautotrophs (seagrass and non-calcifying algae), with the back-reefs predominantly comprised of abiotic-hard and abiotic-soft ground (Figure 3). Across years there was little variation in the benthic composition of each site (see Supplementary Figure 1). Only the GTB back-reef site was different between 2012 and 2013 [two-tailed t-test, t(4) = 3.79, p < 0.05], resulting from changes in the composition of photoautotrophs and a 1.7 fold increase in scleractinian coral cover in 2013.
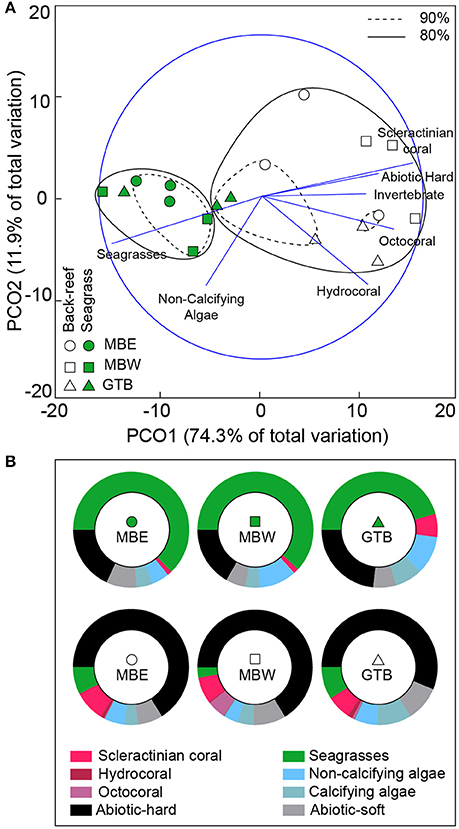
Figure 3. Benthic composition of back-reef and seagrass sites. Principal co-ordinate analysis (PCA) plot (A) of the benthic categories pooled across both 2012 and 2013 (using all species and abiotic categories) per sites with 80% (solid) and 90% (hashed) similarities shown. Pearson's correlations that exceed R > 0.6 are represented as vectors in blue. The proportional composition of each benthic category pooled across years is shown in (B). Note: invertebrates other than the Cnidarians are not visible due to their low density.
The back-reefs had ca. twice the mean coral cover of the seagrass meadows (back-reef: 6.5 ± 1.2%, seagrass: 2.7 ± 0.4%) and coral community composition within each habitat did not differ between years (Table 3). Coral species richness, diversity, and evenness were also higher in the back-reefs compared to the seagrass meadows in both years (Table 3). Although coral cover was higher in the back-reefs, there was a greater density of corals in the seagrass meadows than in the back-reefs (back-reef 119.5 ± 12.8, seagrass 236 ± 95.6 per 20 m2). No dependence between year and habitat type was detected for absolute counts of corals. Coral colonies were relatively small in both habitats, however faster exponential decay rates suggests that seagrass communities were dominated by higher numbers of small coral colonies, while counts were more even across size classes in the back-reefs (Figure 4). Size profile of colonies did not differ significantly across the two years of study. Coral disease prevalence was low across habitats for both years (<1%). However, the prevalence of old mortality was higher on the back-reefs compared to the seagrass meadows [back-reef: 14 ± 0.07%, seagrass: 2 ± 0.02%; F(2, 36) = 253.46, p < 0.001].

Table 3. Summary of established coral community characteristics in the back-reef and seagrass habitats of Little Cayman, Cayman Islands.
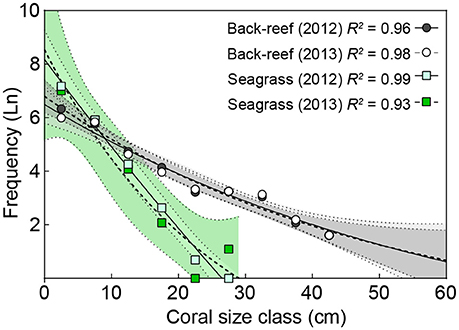
Figure 4. A comparison of coral colony sizes in back-reef and seagrass habitats. Exponential decay model of coral colony size vs. the natural log (Ln) of the frequency of corals within each size class for 2012 (solid lines) and 2013 (hashed lines) in back-reef (circles) and seagrass (squares) habitats. Data are pooled across study sites (n = 3) and 95% confidence bands of each model are shown by areas colored corresponding to symbol colors.
Seagrass coral communities were comprised almost entirely of Poritidae (49.0 ± 0.02%) and Siderastreidae (49.8 ± 0.04%), while these families comprised 65.0 ± 0.03% of total coral cover on the back-reefs (followed by Faviidae which contributed 11.4 ± 0.01%). The dominant coral species in the back-reefs for both years was Porites astreoides (2012: 32 ± 0.7%, 2013: 36 ± 0.4%), while Siderastrea radians was the dominant species in the seagrass meadows both years (2012: 49 ± 0.3%, 2013: 52 ± 0.5%). Supplementary Table 1 provides a full list of coral species observed in each habitat across both years of study.
Recruitment
Coral recruitment onto settlement tiles was only observed during the months of August and September (in both 2012 and 2013). More coral spats recruited to the tile arrays in the back-reefs compared to the seagrass meadows [Figure 5A; t(17) = 8.84, p < 0.001]. A greater diversity of corals also recruited to the back-reef compared to the seagrass meadows (Figure 5). Poritidae and Siderastreidae were the most common families observed on the tiles from both habitat types (Figure 5). In general, the proportional density of coral species in the recruited populations mirrored the proportional density in the established populations (Figure 5). Acroporidae and Agariciidae were absent from both the recruited and established population in the seagrass. However, Faviidae was absent from only the established population in the seagrass (Figure 5C). In the back-reefs, the proportional density of Acroporidae, Agariciidae and “other” recruits were significantly higher compared to that of the established population (p < 0.01). Also, on the back-reef the proportional density of Faviidae recruits was significantly lower compared to that of the established population (p < 0.01). Both the back-reefs and seagrass meadows had more corals that belonged to families other than Acroporidae, Agariciidae, Poritidae, Siderastreidae and Faviidae (and thus fell into the “other” category) in the recruited population compared to the established population (p < 0.001).
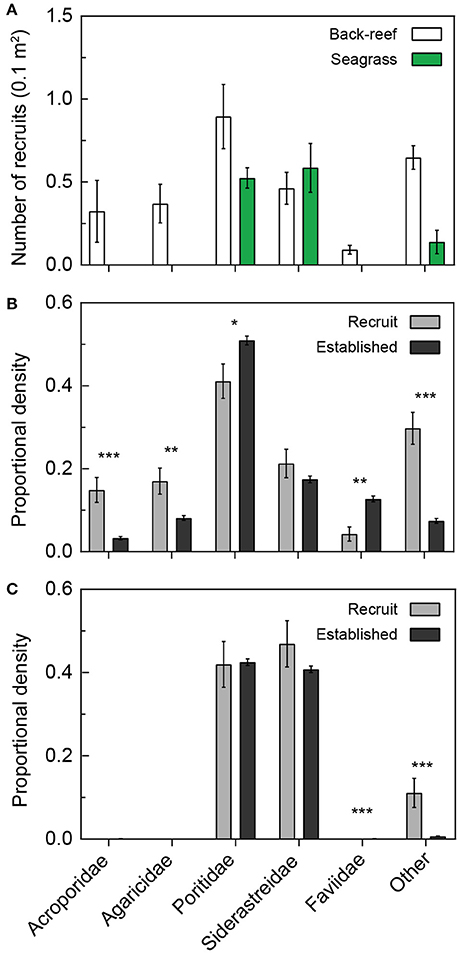
Figure 5. Recruitment of corals into back-reef and seagrass habitats relative to the established population. Number of coral recruits (0.1 m2) on settlement tiles (A) located in back-reef (white bars) and seagrass (green bars) habitats pooled across 2012 and 2013. Coral recruits (gray bars) are compared to the respective proportions of established corals (dark gray bars) within the same families in back-reef and seagrass habitats in (B,C), respectively. Significant differences between the proportions of established and recruited corals are denoted by asterisks, specifically p < 0.05 (*), < 0.01(**), and < 0.001 (***).
Analysis of the composition of all benthic taxa on the experimental tiles following the full 25-month study revealed significant differences between the back-reefs and seagrass meadows [F(4, 13) = 200.73, p < 0.01; Supplementary Figure 2]. Notably, the percent cover of recruited corals was less than 1% in both habitats. Also, cover of calcareous Rhodophyta (i.e., crustose coralline algae) was significantly higher on tiles in the back-reefs compared to the seagrass meadows (post-hoc Tukey test: p < 0.05).
Discussion
Seagrasses are recognized as important primary producers (Duarte and Chiscano, 1999) and for their roles in sediment stabilization and carbon sequestration (Duarte et al., 2013), but the role of seagrass meadows as a habitat in which scleractinian corals can recruit, grow, and reproduce has not been well-studied. We observed up to 14 established scleractinian taxa living within seagrass meadows, despite considerable physicochemical variability. Our data also demonstrate that the larvae of multiple coral families are able to recruit into seagrass meadows. These findings are consistent with previous reports of established coral communities among seagrasses (Rützler and Macintyre, 1982; Johnson, 1992; Higuchi et al., 2014).
Physicochemical Conditions
Seagrass beds were characterized by higher variability in both temperature and pH compared to the adjacent back-reef. This is consistent with previous studies of physicochemical conditions at this study site (Barry et al., 2013; Camp et al., 2016a,b). High physicochemical variability has also been reported for shallow seagrass beds globally; a diel temperature range of as much as 6°C has been reported for shallow seagrass beds (Semesi et al., 2009), consistent with the range of up to 6.7°C recorded in the present study. Similarly, mean diel fluctuations of 0.34 (Saderne et al., 2013) to 0.35 pH units (Challener et al., 2016) have been reported previously from studies of macrophyte beds. One study reported diel fluctuations in excess of 1 pH unit (Semesi et al., 2009). The pH range recorded in Little Cayman seagrass beds (0.51–0.62 pH units) is therefore consistent with previous reports, and is similar to the pH range reported from a study of Grape Tree Bay in 2011 (0.47 pH units, Barry et al., 2013). These substantial fluctuations in pH result from changes in pCO2 driven by seagrass photosynthesis and respiration (Semesi et al., 2009; Saderne et al., 2013; Challener et al., 2016). Covariance of DO with pH is also consistent with previous reports (Barry et al., 2013), and supports the idea that seawater chemistry is altered by seagrasses (Challener et al., 2016). Although physicochemical conditions fluctuated less on the back-reef, variability was still high compared to the open ocean reference site. This is likely due to the effects of the adjacent seagrass meadow; seagrasses have been shown to alter carbonate chemistry of seawater on adjacent reefs (Manzello et al., 2012).
Living under more variable physicochemical conditions can place an enhanced energetic burden on physiological maintenance (Hofmann and Todgham, 2010). Although extreme temperatures are known to be detrimental to coral populations (Eakin et al., 2009), some populations have shown capability for adapting or acclimating to temperature extremes (Craig et al., 2001; Palumbi et al., 2014; Schoepf et al., 2015). Thermal tolerance is also known to vary among coral taxa (Darling et al., 2012), and this variability could therefore explain why some taxa were only observed in the less-variable back-reef in the present study. Similarly, although some sites have been shown to maintain high coral cover and diversity in low-pH conditions (e.g. Golbuu et al., 2016), reduced pH has been shown to limit the abundance (Price et al., 2012) and diversity (Fabricius et al., 2011; Crook et al., 2012) of calcifying organisms at other locations. In the present study, pH at the seagrass sites routinely dropped to values considered unfavorable for most corals (e.g., <7.8, Figure 2; see also Camp et al., 2016a), and therefore may have resulted in environmental filtering against some coral species (Sommer et al., 2014). The ability to tolerate reduced pH varies among coral species (McCulloch et al., 2012), and importantly, low pH appears to inhibit settlement of Acropora palmata, potentially through alteration of larval settlement processes or chemical settlement cues from crustose coralline algae (Albright et al., 2010). Together these factors governing sensitivity provide one possible explanation for the lack of Acroporidae spats either on settlement tiles or in the established coral community at the seagrass sites despite the presence of established and recruited Acropora spp. in the adjacent back-reef. It is however critical to note that the ability of seagrasses to alter seawater carbonate chemistry is dependent on the seagrass species present, water depth, water residence time, and a variety of other local conditions (Unsworth et al., 2012). It is therefore possible that seagrass meadows in other locations could harbor a higher diversity of coral species.
Benthic Structure
Previously published data on coral community composition within seagrass meadows is limited, however our observations of Manicina areolata (Johnson, 1992), Porites furcata (Wulff, 2008), and S. radians (Rützler and Macintyre, 1982) populating seagrass meadows are consistent with previous Caribbean studies. In contrast to our observations, Rützler and Macintyre (1982) report occasional Acropora cervicornis colonies within seagrass patches in Belize; however this data was published prior to the widespread regional decline of Caribbean acroporids (Aronson and Precht, 2001), which could partially explain its absence within seagrass meadows in our study. Low mean coral cover within seagrass meadows in the present study (2.7 ± 0.4%) is also generally consistent with, although somewhat lower compared to, reported coral cover in Indian Ocean (4.20% coral cover) and Pacific Ocean (5.40% coral cover) seagrass meadows (Camp et al., 2016a).
Although size data for corals in seagrass meadows are also rare, previous studies suggest that coral colonies within seagrass in the Caribbean are generally small (Johnson, 1992; Wulff, 2008), consistent with our findings of relatively small colonies in Little Cayman seagrass meadows. Small colony size is characteristic of species that are better able to survive poor conditions (Darling et al., 2012) that may occur frequently in shallow seagrass meadows, including sedimentation, thermal stress, and reduced pH. Small size is also characteristic of the dominant coral species in the seagrass meadow, S. radians (Lirman and Manzello, 2009). Decreased colony size has also been directly associated with low pH conditions (Crook et al., 2012), which regularly occurred in the seagrass meadows studied.
Although the seagrass meadows and back-reefs assessed in this study were in close proximity to one another, species richness and diversity of the established coral community were lower in seagrass meadows. Given that availability of hard substrate is fundamental in structuring benthic communities (Jackson, 1977; Taylor and Wilson, 2003), higher availability of hard substrate in the back-reef is likely a significant factor in the observed higher cover and diversity of corals. Hard substrate is characteristic of reefs, as reef structure is formed by deposition of calcium carbonate skeleton by living corals (Hallock, 2015). In contrast, seagrasses generally require soft sediment, and therefore seagrass meadows typically lack abundant hard substrate (Hemminga and Duarte, 2000). Many of the common coral species in the seagrass meadows studied (i.e., S. radians, Porites divaricata, P. porites) are capable of recruiting to suboptimal habitats (Darling et al., 2012). S. radians in particular has been shown to be highly tolerant of sediment burial (Lirman and Manzello, 2009), which is likely frequent in the soft-sediment seagrass meadow.
Recruitment
Recruitment onto experimental tiles during the 25-month study was only observed during the months of August and September, which is consistent with observations of multiple species spawning in the Cayman Islands during these months in 2012 and 2013 (C. McCoy, Cayman Islands Department of Environment, personal communication), and with published reports for spawning times among Caribbean broadcast-spawning corals (e.g., Jul-Sept, Szmant, 1986). However, our results contrast with previous studies that have documented year-round planulation in Caribbean brooding species with varying species-specific annual peaks in larval release (Szmant, 1986; Chornesky and Peters, 1987; Soong, 1991; Schlöder and Guzman, 2008). Recently reported coral recruit densities vary among sites within both the Caribbean [e.g., 0.14–1.17 spat m−2 (Edmunds et al., 2014), 30 ± 46 – 236 ± 143 spat m−2 (Humanes and Bastidas, 2015)] and the Indo-Pacific [e.g., 1.42 ± 0.33 – 1.72 ± 0.64 spat m−2 (Salinas de León et al., 2011), 54.74 spat m−2 (Bauman et al., 2015)]. Johnson (1992) reported recruitment ranging from <1 to 5 spat m−2 for M. areolata in a seagrass meadow. Recruit densities in our study (i.e., back-reef 1.2 ± 0.05 spat m−2, seagrass: 0.5 ± 0.03 spat m−2) fall within the range reported from Caribbean sites in the published literature.
Factors governing coral recruitment are complex, and recruitment success can be temporally and spatially variable due to factors such as temporal variability in fecundity and physical disturbances (Fitzhardinge, 1985; Williams et al., 2008). The presence or absence of other species can also influence recruitment. For example, crustose coralline algae and microbial biofilms are known to provide cues that induce coral settlement (Heyward and Negri, 1999; Webster et al., 2004). Though our current data set provides new information regarding coral recruitment into seagrass meadows and adjacent back-reefs, it precludes fully understanding the complex nature of differences in recruitment between habitats.
Comparison of Established and Recruited Communities
Although the diversity and density of coral recruits differed between the seagrass and back-reef, community composition of recruited corals largely mirrored that of established corals within sites. This contrasts with multiple studies of coral reefs, which have reported variability in the composition of established and recruited coral communities (e.g., Dunstan and Johnson, 1998; Bramanti and Edmunds, 2016). However, our findings are consistent with those of Johnson (1992), who found that recruitment rates for M. areolata in a seagrass bed related to local density of reproductive adult colonies. The established and recruited coral communities in seagrass meadows were comprised almost entirely of brooding species (Figure 5), which are believed to have a relatively short dispersal distance as a result of rapid settlement following release of planulae (Szmant, 1986; Harriott, 1992; Johnson, 1992; Carlon and Olson, 1993; Kenkel et al., 2013). Brooded planulae also typically receive zooxanthellae from their parent colonies in Caribbean species (Szmant, 1986; Thornhill et al., 2006), and planulae may therefore have a physiological advantage to settle locally, as symbionts and hosts are both adapted to local abiotic conditions (Kenkel et al., 2013). Short dispersal distance combined with local adaptation may therefore explain why the recruited population was highly similar to the established population in each habitat, and also why brooding Agariciidae were not found to disperse from the back-reef to the seagrass.
Despite overall similarity between the recruited and established community within habitats, differences were apparent for some coral families. These intra-habitat differences may also be explained by differences in reproductive strategy. Broadcast spawning corals are particularly susceptible to “Allee effects” and subsequent sexual recruitment failure, especially when densities of mature colonies are low (Knowlton, 2001). For example, Acroporidae are known to have low sexual reproductive success and recruitment in the Caribbean, and thus rely heavily on asexual fragmentation as a reproductive mechanism (Tunnicliffe, 1981; Richmond and Hunter, 1990; Williams et al., 2008). Reliance on asexual fragmentation could explain why relatively high cover of established Acroporidae was observed in the back-reef despite low levels of recruitment across both years of study. Asexual fragmentation is also used as a reproductive mechanism in some poritids, such as P. furcata (Highsmith, 1982). This could potentially explain the abundance of Poritidae in the established population in both the back-reefs and seagrass meadows, as fragmentation can play a role in establishing local dominance by certain species (Highsmith, 1982). Local abundance of Poritidae achieved through fragmentation could ultimately lead to higher rates of sexual reproduction and subsequent recruitment, which was also observed in both habitats. In contrast, lower relative cover of established Faviidae compared to the recruited population could suggest post-settlement mortality, which was found to be common for Orbicella faveolata (Szmant and Miller, 2006; Miller, 2014). Dispersal of coral larvae from distant reefs could also explain observed differences in community composition between established and recruited corals (Harriott and Fisk, 1988). We currently lack data to determine whether outer reef sites may have served as a larval source for recruits in either habitat, however recent work has demonstrated high levels of connectivity between reefs (Schill et al., 2015); it is therefore conceivable that larval exchange between the outer reef, back-reef, and seagrass meadow is possible.
Conclusions and Future Considerations
Our study demonstrates that multiple coral species are able to live within and recruit to seagrass meadows, suggesting that corals are capable of using such systems as a habitat regardless of naturally high variability in environmental conditions relative to neighboring reefs. Fluctuating physicochemical conditions in seagrass meadows can expose corals to both periods of high temperature and periods of low pH, which could enhance tolerance to thermal and chemical stress, respectively (Camp et al., 2016a). Evidence suggests that transgenerational acclimatization is possible following exposure to extreme temperature and pH conditions (Putnam and Gates, 2015); however, exposure of the corals P. astreoides and A. palmata to environmental variance found within seagrass meadows resulted in no enhanced resistance to subsequent temperature and pH stress (Camp et al., 2016b). Furthermore, some seagrass meadows have been shown to buffer against ocean acidification by elevating mean pH in their vicinity, potentially improving the ability of downstream corals to calcify (Manzello et al., 2012). The potential biogeochemical-services seagrass meadows can provide under climate change remains unresolved. Given that seagrasses will be potentially selected for under future climates (Garrard and Beaumont, 2014), they may continue to provide habitat for some coral species. Clearly large gaps still remain in understanding the nature of coral populations in these habitats and the extent to which they can persist in a changing climate. Further studies are needed across other geographic locations to fully understand the extent to which seagrass beds may serve as coral habitats. Importantly however, the added role of seagrass systems in providing coral habitat emphasizes the critical importance in managing and conserving these sites.
Author Contributions
All authors have agreed to be listed on and approve this manuscript. EC, DJSu, and DJSm conceived and designed the study; KL and EC collected the data; EC, AD, MN, and SW analyzed the data; MN prepared all figures; KL, EC, and DJSu wrote the manuscript; all authors discussed and critically edited the manuscript.
Conflict of Interest Statement
The authors declare that the research was conducted in the absence of any commercial or financial relationships that could be construed as a potential conflict of interest.
Acknowledgments
The authors acknowledge C. Manfrino for contributions toward the funding, resources, and context necessary for the completion of this project. We would also like to thank IBEX Earth for their financial support. The authors are grateful for logistical support from the staff of the Little Cayman Research Centre, local volunteers, as well as technical staff from the University of Essex. Sincere thanks also go to the Cayman Islands Department of Environment for issuing two permits required to conduct this study. The permit for settlement tile installation and monitoring was issued to C. Manfrino and K. Foster, and an additional permit for ongoing monitoring and abiotic sampling was issued to E. Camp. This project was part of the Central Caribbean Marine Institute's Climate Change and Coral Reef Stress Program and represents contribution 2017:04.
Supplementary Material
The Supplementary Material for this article can be found online at: https://www.frontiersin.org/articles/10.3389/fmars.2017.00388/full#supplementary-material
References
Albright, R., Mason, B., Miller, M., and Langdon, C. (2010). Ocean acidification compromises recruitment success of the threatened Caribbean coral Acropora palmata. Proc. Natl. Acad. Sci. U.S.A. 107, 20400–20404. doi: 10.1073/pnas.1007273107
Aronson, R. B., and Precht, W. F. (2001). White-band disease and the changing face of Caribbean coral reefs. Hydrobiologia 460, 25–38. doi: 10.1023/A:1013103928980
Babcock, R., Baird, A., Piromvaragorn, S., Thomson, D. P., and Willis, B. L. (2003). Identification of scleractinian coral recruits from indo-pacific reefs. Zool. Stud. 42, 221–226.
Barry, S. C., Frazer, T. K., and Jacoby, C. A. (2013). Production and carbonate dynamics of Halimeda incrassata (Ellis) Lamouroux altered by Thalassia testudinum banks and soland ex König. J. Exp. Mar. Biol. Ecol. 444, 73–80. doi: 10.1016/j.jembe.2013.03.012
Barshis, D. J. (2015). “Genomic potential for coral survival of climate change,” in Coral Reefs in the Anthropocene, ed C. Birkeland (Dordrecht: Springer Science and Business Media), 133–146.
Bauman, A. G., Guest, J. R., Dunshea, G., Low, J., Todd, P. A., and Steinberg, P. D. (2015). Coral settlement on a highly disturbed equatorial reef system. PLoS ONE 10:e0127874. doi: 10.1371/journal.pone.0127874
Borja, A., Prins, T. C., Simboura, N., Andersen, J. H., Berg, T., Marques, J., et al. (2014). Tales from a thousand and one ways to integrate marine ecosystem components when assessing the environmental status. Front. Mar. Sci. 1:72. doi: 10.3389/fmars.2014.00072
Bramanti, L., and Edmunds, P. J. (2016). Density-associated recruitment mediates coral population dynamics on a coral reef. Coral Reefs 35, 543–553. doi: 10.1007/s00338-016-1413-4
Budd, A. F., Fukami, H., Smith, N. D., and Knowlton, N. (2012). Taxonomic classification of the reef coral family Mussidae (Cnidaria: Anthozoa: Scleractinia). Zool. J. Linn. Soc. 166, 465–529. doi: 10.1111/j.1096-3642.2012.00855.x
Camp, E. F., Smith, D. J., Evenhuis, C., Enochs, I., Manzello, D., Woodcock, S., et al. (2016b). Acclimatization to high-variance habitats does not enhance physiological tolerance of two key Caribbean corals to future temperature and pH. P. R. Soc. B. 283, 1831. doi: 10.1098/rspb.2016.0442
Camp, E. F., Suggett, D. J., Gendron, G., Jompa, J., Manfrino, C., and Smith, D. J. (2016a). Mangrove and seagrass beds provide different biogeochemical services for corals threatened by climate change. Front. Mar. Sci. 3:52. doi: 10.3389/fmars.2016.00052
Carlon, D. B., and Olson, R. R. (1993). Larval dispersal distance as an explanation for adult spatial pattern in two Caribbean reef corals. J. Exp. Mar. Biol. Ecol. 173, 247–263. doi: 10.1016/0022-0981(93)90056-T
Castillo, K. D., Ries, J. B., Weiss, J. M., and Lima, F. P. (2012). Decline of forereef corals in response to recent warming linked to history of thermal exposure. Nat. Clim. Change 2, 756–760. doi: 10.1038/nclimate1577
Challener, R. C., Robbins, L. L., and McClintock, J. B. (2016). Variability of the carbonate chemistry in a shallow, seagrass-dominated ecosystem: implications for ocean acidification experiments. Mar. Freshw. Res. 67, 163–172. doi: 10.1071/MF14219
Chornesky, E. A., and Peters, E. C. (1987). Sexual reproduction and colony growth in the scleractinian coral Porites astreoides. Biol. Bull. 172, 161–177. doi: 10.2307/1541790
Craig, P., Birkeland, C., and Belliveau, S. (2001). High temperatures tolerated by a diverse assemblage of shallow-water corals in American Samoa. Coral Reefs 20, 185–189. doi: 10.1007/s003380100159
Crook, E. D., Potts, D., Rebolledo-Vieyra, M., Hernandez, L., and Paytan, A. (2012). Calcifying coral abundance near low-pH springs: implications for future ocean acidification. Coral Reefs 31, 239–245. doi: 10.1007/s00338-011-0839-y
Darling, E. S., Alvarez-Filip, L., Oliver, T. A., McClanahan, T. R., and Côté, I. M. (2012). Evaluating life-history strategies of reef corals from species traits. Ecol. Lett. 15, 1378–1386. doi: 10.1111/j.1461-0248.2012.01861.x
de Putron, S. (2005). Coral Spat Identification Booklet. (Unpublished data). St. George's: Bermuda Institute of Ocean Sciences.
de Putron, S. (2007). An Examination of Degraded Coral Reefs. (Unpublished data). St. George's: Bermuda Institute of Ocean Sciences.
Dickson, A. G. (1990). Thermodynamics of the dissociation of boric acid in synthetic seawater from 273.15 to 318.15 K. Deep Sea Res. 37, 755–766. doi: 10.1016/0198-0149(90)90004-F
Dickson, A. G., and Millero, F. J. (1987). A comparison of the equilibrium constants for the dissociation of carbonic acid in seawater media. Deep Sea Res. 34, 1733–1743. doi: 10.1016/0198-0149(87)90021-5
Dickson, A., Sabine, C., and Christian, R. (Eds.) (2007). Guide to Best Practices for Ocean CO2 Measurements. Sidney, NSW: North Pacific Marine Science Organization.
Duarte, C. M., and Chiscano, C. L. (1999). Seagrass biomass and production: a reassessment. Aquat. Bot. 65, 159–174. doi: 10.1016/S0304-3770(99)00038-8
Duarte, C. M., Kennedy, H., Marbà, N., and Hendriks, I. (2013). Assessing the capacity of seagrass meadows for carbon burial: current limitations and future strategies. Ocean Coast. Manage. 83, 32–38. doi: 10.1016/j.ocecoaman.2011.09.001
Dunstan, P. K., and Johnson, C. R. (1998). Spatio-temporal variation in coral recruitment at different scales on Heron Reef, southern great barrier reef. Coral Reefs 17, 71–81. doi: 10.1007/s003380050098
Eakin, C. M., Lough, J. M., and Heron, S. F. (2009). “Climate variability and change: monitoring data and evidence for increased coral bleaching stress,” in Coral Bleaching: Patterns, Processes, Causes and Consequences, eds M. J. H. van Oppen and J. Lough (Berlin: Springer), 41–67.
Edmunds, P. J., Nozawa, Y., and Villanueva, R. D. (2014). Refuges modulate coral recruitment in the Caribbean and the Pacific. J. Exp. Mar. Biol. Ecol. 454, 78–84. doi: 10.1016/j.jembe.2014.02.009
English, S., Wilkinson, C., and Baker, V. (1994). Survey Manual for Tropical Marine Resources. Townsville, QLD: Australian Institute of Marine Science.
Fabricius, K. E., Langdon, C., Uthicke, S., Humphrey, C., Noonan, S., De'ath, G., et al. (2011). Losers and winners in coral reefs acclimatized to elevated carbon dioxide concentrations. Nat. Clim. Change 1, 165–169. doi: 10.1038/nclimate1122
Fitzhardinge, R. (1985). “Spatial and temporal variability in coral recruitment in Kaneohe Bay (Oahu, Hawaii),” in Proceedings of the 5th International Coral Reef Congress (Tahiti).
Garrard, S. L., and Beaumont, N. J. (2014). The effect of ocean acidification on carbon storage and sequestration in seagrass beds; a global and UK context. Mar. Pollut. Bull. 86, 138–146. doi: 10.1016/j.marpolbul.2014.07.032
Golbuu, Y., Gouezo, M., Kurihara, H., Rehm, L., and Wolanski, E. (2016). Long-term isolation and local adaptation in Palau's Nikko Bay help corals thrive in acidic waters. Coral Reefs 35, 909–918. doi: 10.1007/s00338-016-1457-5
Goreau, T. F. (1959). The ecology of Jamaican coral reefs I. species composition and zonation. Ecology 40, 67–90. doi: 10.2307/1929924
Hallock, P. (2015). “Changing influences between life and limestones in Earth history,” in Coral Reefs in the Anthropocene, ed C. Birkeland (Dordrecht: Springer Science and Business Media), 17–42.
Harriott, V., and Fisk, D. (1987). A comparison of settlement plate types for experiments on the recruitment of scleractinian corals. Mar. Ecol. Prog. Ser. 37, 201–208. doi: 10.3354/meps037201
Harriott, V., and Fisk, D. (1988). Recruitment patterns of scleractinian corals: a study of three reefs. Mar. Freshw. Res. 39:409. doi: 10.1071/MF9880409
Harriott, V. J. (1992). Recruitment patterns of scleractinian corals in an isolated sub-tropical reef system. Coral Reefs 11, 215–219. doi: 10.1007/BF00301996
Heyward, A., and Negri, A. (1999). Natural inducers for coral larval metamorphosis. Coral Reefs 18, 273–279. doi: 10.1007/s003380050193
Highsmith, R. C. (1982). Reproduction by fragmentation in corals. Mar. Ecol. Prog. Ser. 7, 207–226. doi: 10.3354/meps007207
Higuchi, T., Takagi, K. K., Matoba, K., Kobayashi, S., Tsurumi, R., Arakaki, S., et al. (2014). The nutrient and carbon dynamics that mutually benefit coral and seagrass in mixed habitats under the influence of groundwater at Bise coral reef, Okinawa, Japan. Int. J. Mar. Sci. 4, 1–15. doi: 10.5376/ijms.2014.04.0001
Hofmann, G. E., and Todgham, A. E. (2010). Living in the now: physiological mechanisms to tolerate a rapidly changing environment. Annu. Rev. Physiol. 72, 127–145. doi: 10.1146/annurev-physiol-021909-135900
Hughes, T. P., Graham, N. A. J., Jackson, J. B. C., Mumby, P. J., and Steneck, R. S. (2010). Rising to the challenge of sustaining coral reef resilience. Trends Ecol. Evol. 25, 633–642. doi: 10.1016/j.tree.2010.07.011
Humanes, A., and Bastidas, C. (2015). In situ settlement rates and early survivorship of hard corals: a good year for a Caribbean reef. Mar. Ecol. Prog. Ser. 539, 139–151. doi: 10.3354/meps11501
Hurlbert, S. H. (1984). Pseudoreplication and the design of ecological field experiments. Ecol. Monograph. 54, 187–211. doi: 10.2307/1942661
Jackson, J. B. C. (1977). Competition on marine hard substrata: the adaptive significance of solitary and colonial strategies. Am. Nat. 111, 743–767. doi: 10.1086/283203
Jackson, J. B. C. (1986). “Distribution and ecology of clonal and aclonal benthic invertebrates,” in Population Biology and Evolution of Clonal Organisms, eds J. B. C. Jackson, L. W. Buss, R. E. Cook (New Haven, CT: Yale University Press), 297–356.
Jackson, J., Donovan, M., Cramer, K., and Lam, V. (eds.). (2014). Status and Trends of Caribbean Coral Reefs: 1970-2012. Gland: Global Coral Reef Monitoring Network.
Johnson, K. G. (1992). Population dynamics of a free-living coral: recruitment, growth and survivorship of Manicina areolata (Linnaeus) on the Caribbean coast of Panama. J. Exp. Mar. Biol. Ecol. 164, 171–191. doi: 10.1016/0022-0981(92)90173-8
Kenkel, C. D., Goodbody-Gringley, G., Caillaud, D., Davies, S. W., Bartels, E., and Matz, M. V. (2013). Evidence for a host role in thermotolerance divergence between populations of the mustard hill coral (Porites astreoides) from different reef environments. Mol. Ecol. 22, 4335–4348. doi: 10.1111/mec.12391
Knowlton, N. (2001). The future of coral reefs. Proc. Natl. Acad. Sci. U.S.A. 98, 5419–5425. doi: 10.1073/pnas.091092998
Lang, J. C., Marks, K. W., Kramer, P. A., Kramer, P. R., and Ginsburg, R. N. (2010). Atlantic and Gulf Rapid Reef Assessment Protocols Version 5.4. Atlantic and Gulf Rapid Reef Assessment.
Lewis, E., and Wallace, D. (1998). Program Developed for CO2 System Calculations. ORNL/CDIAC-105. Oak Ridge, TN: Carbon Dioxide Information Analysis Center, Oak Ridge National Laboratory, US Department of Energy.
Lirman, D., and Manzello, D. (2009). Patterns of resistance and resilience of the stress-tolerant coral Siderastrea radians (Pallas) to sub-optimal salinity and sediment burial. J. Exp. Mar. Biol. Ecol. 369, 72–77. doi: 10.1016/j.jembe.2008.10.024
Logan, A. (2013). “Coral reefs of the cayman islands,” in Coral Reefs of the United Kingdom Overseas Territories, Coral Reefs of the World 4, ed C. R. C. Sheppard (Dordrecht: Springer Science and Business Media), 61–68.
Long, M. H., Rheuban, J. E., Berg, P., and Zieman, J. C. (2012). A comparison and correction of light intensity loggers to photosynthetically active radiation sensors. Limnol. Oceanogr. Methods 10, 416–424. doi: 10.4319/lom.2012.10.416
Manzello, D. P., Enochs, I. C., Melo, N., Gledhill, D. K., and Johns, E. M. (2012). Ocean acidification refugia of the Florida reef tract. PLoS ONE 7:e41715. doi: 10.1371/journal.pone.0041715
McCulloch, M., Falter, J., Trotter, J., and Montagna, P. (2012). Coral resilience to ocean acidification and global warming through pH up-regulation. Nat. Clim. Change 2, 623–627. doi: 10.1038/nclimate1473
Mehrbach, C., Culberson, C. H., Hawley, J. E., and Pytkowicz, R. M. (1973). Measurement of the apparent dissociation constants of carbonic acid in seawater at atmospheric pressure. Limnol. Oceanogr. 18, 897–907. doi: 10.4319/lo.1973.18.6.0897
Miller, M. W. (2014). Post-settlement survivorship in two Caribbean broadcasting corals. Coral Reefs 33, 1041–1046. doi: 10.1007/s00338-014-1177-7
Mumby, P. J., Hastings, A., and Edwards, H. J. (2007). Thresholds and the resilience of Caribbean coral reefs. Nature 450, 98–101. doi: 10.1038/nature06252
Mundy, C. N. (2000). An appraisal of methods used in coral recruitment studies. Coral Reefs 19, 124–131. doi: 10.1007/s003380000081
Nyström, M., Graham, N. A. J., Lokrantz, J., and Norström, A. V. (2008). Capturing the cornerstones of coral reef resilience: linking theory to practice. Coral Reefs 27, 795–809. doi: 10.1007/s00338-008-0426-z
Palumbi, S. R., Barshis, D. J., Traylor-Knowles, N., and Bay, R. A. (2014). Mechanisms of reef coral resistance to future climate change. Science 344, 895–898. doi: 10.1126/science.1251336
Perry, C. T., and Larcombe, P. (2003). Marginal and non-reef-building coral environments. Coral Reefs 22, 427–432. doi: 10.1007/s00338-003-0330-5
Price, N. N., Martz, T. R., Brainard, R. E., and Smith, J. E. (2012). Diel variability in seawater pH relates to calcification and benthic community structure on coral reefs. PLoS ONE 7:e43843. doi: 10.1371/journal.pone.0043843
Putnam, H. M., and Gates, R. D. (2015). Preconditioning in the reef-building coral Pocillopora damicornis and the potential for trans-generational acclimatization in coral larvae under future climate change conditions. J. Exp. Biol. 218, 2365–2372. doi: 10.1242/jeb.123018
R Core Team (2014). R: A Language and Environment for Statistical Computing. Vienna: R Foundation for Statistical Computing.
Richmond, R., and Hunter, C. (1990). Reproduction and recruitment of corals: comparisons among the Caribbean, the tropical pacific, and the red sea. Mar. Ecol. Prog. Ser. 60, 185–203. doi: 10.3354/meps060185
Riebesell, U., Zondervan, I., Rost, B., Tortell, P. D., Zeebe, R. E., and Morel, F. M. (2000). Reduced calcification of marine plankton in response to increased atmospheric CO2. Nature 407, 364–367. doi: 10.1038/35030078
Riegl, B. M. (1999). Corals in a non-reef setting in the southern Arabian Gulf (Dubai, UAE): fauna and community structure in response to recurring mass mortality. Coral Reefs 18, 63–73. doi: 10.1007/s003380050156
Rützler, K., and Macintyre, I. G. (1982). “The habitat distribution and community structure of the barrier reef complex at Carrie Bow Cay, Belize,” in The Atlantic Barrier Reef Ecosystem at Carrie Bow Cay, Belize, I: Structure and Communities, eds K. Rützler, and I. G. Macintyre (Washington, DC: Smithsonian Institution Press), 9–45.
Saderne, V., Fietzek, P., and Herman, P. M. J. (2013). Extreme variations of pCO2 and pH in a macrophyte meadow of the Baltic Sea in summer: evidence of the effect of photosynthesis and local upwelling. PLoS ONE 8:e62689. doi: 10.1371/journal.pone.0062689
Salinas de León, P., Costales-Carrera, A., Zeljkovic, S., Smith, D. J., and Bell, J. J. (2011). Scleractinian settlement patterns to natural cleared reef substrata and artificial settlement panels on an Indonesian coral reef. Estuar. Coast. Shelf. Sci. 93, 80–85. doi: 10.1016/j.ecss.2011.02.016
Santangelo, G., Maggi, E., Bramanti, L., and Bongiorni, L. (2004). Demography of the over-exploited Mediterranean red coral (Corrallium rubrum L. 1758). Sci. Mar. 68, 199–204. doi: 10.3989/scimar.2004.68s1199
Schill, S. R., Raber, G. T., Roberts, J. J., Tremi, E. A., Brenner, J., and Halpin, P. N. (2015). No reef is an island: integrating coral reef connectivity data into the design of regional-scale marine protected area networks. PLoS ONE 10:e0144199. doi: 10.1371/journal.pone.0144199
Schlöder, C., and Guzman, H. M. (2008). Reproductive patterns of the Caribbean coral Porites furcata (Anthozoa, Scleractinia, Poritidae) in Panama. Bull. Mar. Sci. 82, 107–117.
Schmidt-Roach, S., Kunzmann, A., and Martinez Arbizu, P. (2008). In situ observation of coral recruitment using fluorescence census techniques. J. Exp. Mar. Biol. Ecol. 367, 37–40. doi: 10.1016/j.jembe.2008.08.012
Schoepf, V., Stat, M., Falter, J. L., and McCulloch, M. T. (2015). Limits to the thermal tolerance of corals adapted to a highly fluctuating, naturally extreme temperature environment. Sci. Rep. 5:17639. doi: 10.1038/srep17639
Semesi, I. S., Beer, S., and Björk, M. (2009). Seagrass photosynthesis controls rates of calcification and photosynthesis of calcareous macroalgae in a tropical seagrass meadow. Mar. Ecol. Prog. Ser. 382, 41–47. doi: 10.3354/meps07973
Sommer, B., Harrison, P. L., Beger, M., and Pandolfi, J. M. (2014). Trait-mediated environmental filtering drives assembly at biogeographic transition zones. Ecology 95, 1000–1009. doi: 10.1890/13-1445.1
Soong, K. (1991). Sexual reproductive patterns of shallow-water reef corals in Panama. Bull. Mar. Sci. 49, 832–846.
Starnes, D. S., Yates, D., and Moore, D. S. (2012). The Practice of Statistics, 4th Edn. New York, NY: W.H. Freeman and Company.
Suggett, D. J., Hall-Spencer, J. M., Rodolfo-Metalpa, R., Boatman, T. G., Payton, R., Tye Pettay, D., et al. (2012). Sea anemones may thrive in a high CO2 world. Glob. Chang. Biol. 18, 3015–3025. doi: 10.1111/j.1365-2486.2012.02767.x
Szmant, A. M. (1986). Reproductive ecology of Caribbean reef corals. Coral Reefs 5, 43–53. doi: 10.1007/BF00302170
Szmant, A. M., and Miller, M. W. (2006). “Settlement preferences and post-settlement mortality of laboratory cultured and settled larvae of the Caribbean hermatypic coral Montastraea faveolata and Acropora palmata in the Florida keys, USA,” in Proceedings of the 10th International Coral Reef Symposium (Okinawa).
Taylor, P. D., and Wilson, M. A. (2003). Palaeoecology and evolution of marine hard substrate communities. Earth Sci. Rev. 62, 1–103. doi: 10.1016/S0012-8252(02)00131-9
Thornhill, D. J., Fitt, W. K., and Schmidt, G. (2006). Highly stable symbioses among western Atlantic brooding corals. Coral Reefs 25, 515–519. doi: 10.1007/s00338-006-0157-y
Tunnicliffe, V. (1981). Breakage and propagation of the stony coral Acropora cervicornis. Proc. Natl. Acad. Sci. U.S.A. 78, 2427–2431. doi: 10.1073/pnas.78.4.2427
Unsworth, R. K. F., Collier, C. J., Henderson, G. M., and McKenzie, L. J. (2012). Tropical seagrass meadows modify seawater carbon chemistry: implications for coral reefs impacted by ocean acidification. Environ. Res. Lett. 7:24026. doi: 10.1088/1748-9326/7/2/024026
Webster, N. S., Smith, L. D., Heyward, A. J., Watts, J. E., Webb, R. I., Blackall, L. L., et al. (2004). Metamorphosis of a scleractinian coral in response to microbial biofilms. Appl. Environ. Microbiol. 70, 1213–1221. doi: 10.1128/AEM.70.2.1213-1221.2004
Williams, D., Miller, M., and Kramer, K. (2008). Recruitment failure in Florida keys Acropora palmata, a threatened Caribbean coral. Coral Reefs 27, 697–705. doi: 10.1007/s00338-008-0386-3
Wulff, J. L. (2008). Collaboration among sponge species increases sponge diversity and abundance in a seagrass meadow. Mar. Ecol. 29, 193–204. doi: 10.1111/j.1439-0485.2008.00224.x
Keywords: seagrass, coral, recruitment, environmental variability, marginal habitats, benthic ecology, climate change, marine chemistry
Citation: Lohr KE, Smith DJ, Suggett DJ, Nitschke MR, Dumbrell AJ, Woodcock S and Camp EF (2017) Coral Community Structure and Recruitment in Seagrass Meadows. Front. Mar. Sci. 4:388. doi: 10.3389/fmars.2017.00388
Received: 01 May 2017; Accepted: 16 November 2017;
Published: 30 November 2017.
Edited by:
Hajime Kayanne, The University of Tokyo, JapanReviewed by:
Steeve Comeau, University of Western Australia, AustraliaSusana Enríquez, Universidad Nacional Autónoma de México, Mexico
Copyright © 2017 Lohr, Smith, Suggett, Nitschke, Dumbrell, Woodcock and Camp. This is an open-access article distributed under the terms of the Creative Commons Attribution License (CC BY). The use, distribution or reproduction in other forums is permitted, provided the original author(s) or licensor are credited and that the original publication in this journal is cited, in accordance with accepted academic practice. No use, distribution or reproduction is permitted which does not comply with these terms.
*Correspondence: Kathryn E. Lohr, a2Vsb2hyQHVmbC5lZHU=