- 1Coral Reef Laboratory, Ocean and Earth Science, University of Southampton, Southampton, United Kingdom
- 2Institute for Life Sciences, University of Southampton, Southampton, United Kingdom
Photodamage of symbiotic algae exposed to thermal stress is involved in mass coral bleaching, a major cause of reef decline. Photoprotection is therefore a vital part of coral stress physiology. Corals produce a variety of green fluorescent protein (GFP)-like proteins, some of which screen the symbiotic algae from excess sun light. Different tissue concentrations of these GFP-like proteins distinguish color morphs that are characteristic for many coral species. The question arises whether these pigmentation differences may diversify the niches that can be occupied by corals along the steep light gradient that structures coral reef communities. We assessed the implications of GFP-like protein expression in two color morphs of the symbiotic coral Hydnophora grandis, both associated with the same Symbiodinium sp. (subclade C40). The color morphs of this species (high fluorescent, HF; and low fluorescent, LF), characterized by markedly different contents of a cyan fluorescent protein, were exposed to different quantities of blue light (470 nm) that matched the major absorption band of the host pigment (473 nm). High intensities of blue light caused less photodamage to the symbiotic algae of the HF morph and resulted in higher growth rates of these corals compared to representatives of the LF morph. In contrast, under low intensities of blue light, the HF morph showed lower growth rates than the LF morph, indicating that trade-offs are associated with high levels of fluorescent protein expression under this condition. Both morphs showed highest growth rates at medium light intensities with no obvious influence of the tissue pigmentation. Reef coral color polymorphism caused by photoprotective GFP-like proteins may therefore be a product of balancing selection in which high pigment contents may be beneficial at the upper and detrimental at the lower end of the depth distribution range of symbiotic corals. Conversely, color morphs with GFP-like proteins that function to optimize symbiont photosynthesis in low light environments could gain an advantage from the benefits offered by high pigment levels in deeper waters.
Introduction
Elevated sea water temperatures are a major cause of mass bleaching events and associated coral mortality (Hoegh-Guldberg, 1999; Hughes et al., 2017). Heat stress inhibits the repair of photosystem II (Takahashi et al., 2009) and lowers the photoinhibitory threshold of the symbiotic algae (Jones et al., 1998; Warner et al., 1999; Bhagooli and Hidaka, 2003; Takahashi et al., 2009), thereby facilitating light-induced damage to Photosystem II reaction centers, particularly the D1 protein (Aro et al., 1993). The resulting faults in energy transfer and electron transport can lead to oxidative stress (reviewed by Pospíšil, 2016) that can cause the breakdown of the symbiotic association and loss of algal cells from the coral tissue (Lesser, 1997; Rehman et al., 2016). The susceptibility of corals to heat and light stress-induced bleaching is increased by unfavorable levels of nutrients in the water, in particular by phosphate deficiency (D'Angelo and Wiedenmann, 2014; Rosset et al., 2017). Bleached corals die unless the symbiont population recovers (Glynn et al., 2001; Baker et al., 2008). Corals and zooxanthellae have therefore evolved protective mechanisms to reduce the impact of light stress. In zooxanthellae, these mechanisms include: xanthophyll cycling (Ambarsari et al., 1997), the use of alternative electron pathways (Reynolds et al., 2008); and the downregulation of photosystem II reaction centers (Gorbunov et al., 2001). Both corals and zooxanthellae produce antioxidant enzymes (Lesser, 2006; Levy et al., 2006) and mycosporine-like amino acids (Banaszak et al., 2000; Shick, 2004; Starcevic et al., 2010) that help to protect the organisms from the negative impact of high light intensities.
A photoprotective function has been also assigned to fluorescent pigments that are often produced by corals and other cnidarians (Wiedenmann et al., 1999; Salih et al., 2000). These pigments belong to the family of GFP-like proteins (Matz et al., 1999; Dove et al., 2001; Alieva et al., 2008). Based on their optical properties they can be divided into fluorescent proteins (FPs) and non-fluorescent chromo-proteins (CPs), noting that CPs are the product of multiple parallel evolution (Gittins et al., 2015). GFP-like proteins can contribute up to ~7–14% to the soluble cellular proteins in coral tissues (Leutenegger et al., 2007; Oswald et al., 2007) suggesting that they fulfill a significant function within the host. Experimental studies have confirmed that CPs (Smith et al., 2013) as well as green and red FPs (Salih et al., 2000; Gittins et al., 2015) in shallow water corals can exert a photoprotective function by screening the algal symbionts from excess light. However, this function may not always be evident (Dove et al., 1995; Mazel et al., 2003). High levels of fluorescent proteins also occur in mesophotic corals (Eyal et al., 2015; Roth et al., 2015) and other deep-water cnidarians (Vogt et al., 2008), suggesting that these pigments can have functions other than photoprotection. In low light conditions, for example, coral GFP-like proteins have been suggested to enhance light availability for photosynthesis of the algal symbionts (Salih et al., 2000; Eyal et al., 2015; Lyndby et al., 2016). Indeed, recent studies have demonstrated that Förster Resonance Energy Transfer (FRET)-mediated wavelength conversion by orange-red fluorescent proteins promotes the light penetration in symbiont-containing tissue in corals from blue-light dominated deep water habitats (Bollati et al., 2017; Smith et al., 2017).
Among FPs, four color groups have been identified: cyan, green, yellow, and red (Alieva et al., 2008; Nienhaus and Wiedenmann, 2009). In contrast to yellow FPs that are rarely encountered in reef organisms (Alieva et al., 2008), red fluorescent proteins are fairly common. Based on the biochemistry of the chromophore and distinct spectral features, red FPs can be divided into a group in which the red chromophore is formed in an autocatalytic reaction (Matz et al., 1999; Wiedenmann et al., 2002) and a group in which the chromophore is formed by photoconversion of a green precursor (Ando et al., 2002; Wiedenmann et al., 2004; Oswald et al., 2007). The latter optimize the internal light environment for the symbionts in corals from less light exposed habitats (Smith et al., 2017) whereas members of the first group can be found in shallow water corals where they exert a photoprotective function similar to CPs (Smith et al., 2013; Gittins et al., 2015). Cyan and green fluorescent proteins (GFPs) can be frequently encountered in most reef environments (Salih et al., 2000; Eyal et al., 2015; Roth et al., 2015) and representatives of this color group have also been shown to be photoprotective in shallow water corals (Salih et al., 2000). Cyan fluorescent proteins (CFPs) contain the same chromophore as GFPs (Henderson and Remington, 2005) but have characteristically broad absorption/excitation spectra with peaks at 440–460 nm (Alieva et al., 2008) that overlap with the absorption / excitation spectrum of photosynthetic pigments of the symbiotic algae (Smith et al., 2013). CFPs with emission maxima around 485–495 nm have evolved as a product of positive natural selection (Field et al., 2006) and are therefore recognized as a separate color class (Alieva et al., 2008). Their production in corals is often regulated by the intensities of blue light (D'Angelo et al., 2008).
Many coral species exhibit color polymorphisms caused by stable differences in CP and FP expression (Kelmanson and Matz, 2003; Klueter et al., 2006; Oswald et al., 2007; Gittins et al., 2015). In Acropora millepora, the pigment concentration in the tissue of different morphs is strongly correlated with the number of gene copies with a particular promoter type, indicating that color polymorphism in reef corals is caused by the differential expression of multicopy genes (Gittins et al., 2015). We have proposed that the balancing selection driving the evolution of color morphs could be due to the benefits from photoprotection offered by the pigments in niches with high levels of light stress and the trade-offs associated with the productions of large quantities of pigments in the absence of light stress (Gittins et al., 2015). Such a balancing selection may allow different color morphs to persist within a population and increase the range of niches that can be occupied by a species across the steep light gradient that structures coral reef communities. However, at present it is unknown whether measurable trade-offs are associated with the expression of photoprotective FPs. On the one hand, no other enzymes and co-factors are required for the pigment production and the proteins have a slow turnover with a half-life of ~20 days, suggesting the energetic costs for their production could be relatively low (Leutenegger et al., 2007). On the other hand, a high-level expression of at least eight FP gene copies is required to accumulate pigment concentrations in the tissue that are sufficiently high to fulfill a light screening function (Gittins et al., 2015). Therefore, we have assessed whether the expression of a photoprotective FP in Hydnophora grandis is associated with trade-offs that manifest in changes of growth rates in different light environments. The two color morphs of choice were associated with the same Symbiodinium sp. (C40), but differed markedly in their expression of CFPs (D'Angelo et al., 2008). The capacity of the CFPs to exert a photoprotective function by selective screening of symbionts in the blue spectral range was assessed by exposing the corals to either blue or orange-red light. Orange-red light was chosen for the control treatment as it is not absorbed by the CFPs and should therefore negatively affect both the high and low fluorescent morphs due to the detrimental effect of this waveband on algal photosynthesis (Smith et al., 2013; Wijgerde et al., 2014).
We predicted that the CFP plays a photoprotective role in H. grandis and that therefore the high fluorescent (HF) color morph would show a higher growth rate than the low fluorescent (LF) color morph under high intensities of blue light. We also predicted that removal of essential photons by CFP under low intensities of blue light together with the energetic cost of their high level production would cause the HF morph grow at a slower rate than the LF morph.
Materials and Methods
Origin and Maintenance of Corals
The experimental corals, produced in aquaculture at Fiji, were obtained via Tropical Marine Centre London. Corals were cultured in the experimental mesocosm of the Coral Reef Laboratory at the University of Southampton (D'Angelo and Wiedenmann, 2012) and propagated by fragmentation for >5 years before the start of the experiments. Hence, the corals were fully acclimated to the conditions in the experimental aquarium. While the being genetically homogeneous, replicate colonies within each color morph represent independent units with individual life histories.
Identification of Algal Symbionts
The Symbiodinium present in each color morph of H. grandis were identified to the subclade level by analyzing the ITS2 region of genomic DNA samples from each color morph. A modified CTAB type extraction was carried out (D'Angelo et al., 2015). The region spanning the 18S, ITS1, 5.8S, ITS2, 28S region of the Symbiodinium spp. ribosomal DNA was amplified by performing a PCR using the primers SYM-VAR-FWD, and SYM-VAR-REV (Hume et al., 2013).
Amplification was accomplished in the presence of 2 μl of 50 × dNTP mix, 1 μl of each primer, 1 μl of template DNA, 0.5 μl of Advantage 2 polymerase mix (Clontech), 5 μl of 10 x Advantage 2 PCR buffer, and 40 μl PCR grade water. The PCR encompassed an initial denaturation step of 1 min at 95°C, followed by 30 cycles of denaturation (95°C for 30 s), annealing (62°C for 30 s), and elongation (68°C for 30 s). A final elongation step of 5 min at 70°C performed. The PCR products were then cloned as describes in the StrataClone PCR Cloning Kit protocol. Plasmid DNA was prepared from 10 Escherichia coli colonies using a ThermoScientific GeneJET Plasmid Miniprep Kit. Sequencing services were provided by Eurofins MWG.
The ITS2 region of the PCR fragment was isolated using reference sequences available in Genbank using the BLAST tool (www.ncbi.nlm.nih.gov/blast). The software package Geneious was used to visualize the vector sequences. The ITS2 regions of the 10 samples were then compared to reference sequences available in Genbank.
Light Exposure Experiments
Coral growth and changes in CFP concentration were assessed in three experiments set up in different flow-through compartments of our experimental mesocosm system (D'Angelo and Wiedenmann, 2012). Five replicate colonies of each color morph were used per condition. All experimental tanks were connected to the same recirculating water system (D'Angelo and Wiedenmann, 2012). Replete levels of dissolved inorganic nutrients were used since imbalanced nutrient levels or low phosphate concentrations can significantly increase the susceptibility of symbiotic corals to light stress (D'Angelo and Wiedenmann, 2014; Rosset et al., 2015, 2017). The water temperature was kept constant at ~25°C. Prior to the start of the light exposure experiments, the corals were cultured under white metal halide light (Aqualine 10000) at a photon flux of 250 μmol photons m−2s−1 on a 10:14 h light:dark cycle. LED stripes (Reef Blue, Aquaray, Tropical Marine Centre London) with controllable output were used to expose the corals to narrow-banded blue light (~470 nm) that matches the excitation spectrum of the coral CFPs (Figure 1C). Colonies were acclimated for 3 weeks to the experimental light condition by changing the light intensities of blue LEDs gradually from ~150 μmol photons m−2s−1 to reach final photon fluxes of 1,100 μmol m−2s−1 (high light treatment), 300 μmol m−2s−1 medium light treatment), and 10 μmol m−2s−1 (low light treatment). Light intensity was measured using a LI-COR LI-250A Light Meter directly at the water surface level, situated ~3 cm above the coral colonies. The height of the LEDs above the water surface was adjusted until the desired light intensity was reached. The weight of the replicate colonies was determined after a 30 s drip-off step on absorbing tissue to remove excess water. Quantitative fluorescence data of the colonies were measured every 2–3 weeks (D'Angelo et al., 2008) using a Varian Cary Eclipse fluorescence spectrophotometer for recording of spectra in the range of 250–510 nm with the emission wavelength set to 540 nm (slit-width 5; scan speed medium). For imaging purposes, coral fluorescence was excited by ~470 nm light provided by the Aquaray Reef Blue LEDS, which closely matches to the absorption maximum of CFPs. Photographs were taken with a Olympus Tough camera (Olympus Corporation, Tokyo, Japan) equipped with a yellow long-pass filter (Nightsea, Andover, USA) to block reflected excitation light. Due to the optical properties of the filter, only the green portion of light emitted by the CFPs is detected by the camera and, accordingly, the corals in the photographs appear green. Since the corals under study contain only CFPs, these images nevertheless give a truthful representation of intensity of cyan tissue fluorescence.
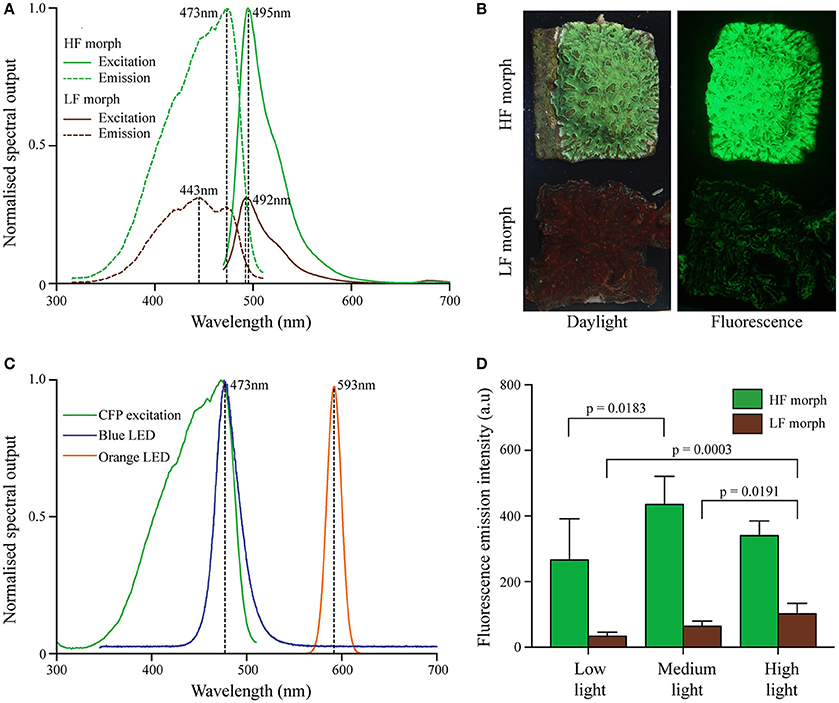
Figure 1. (A) Excitation and emission spectra of two color morphs of Hydnophora grandis. Peak maxima indicated by dashed vertical lines with peak wavelengths (nm) being indicated. Excitation spectra are scaled to the maximum of the corresponding emission spectra. (B) Photographs of representative colonies of HF (top) and LF (bottom) morphs under daylight (left) and blue light excitation (~470 nm; imaged with a yellow long-pass filter) (right). (C) Emission spectra of blue LED (blue line) and orange LED (orange line) used in aquarium experiments. The CFP excitation spectrum of the HF morph is included for comparison (green line). (D) Fluorescence emission intensity (measured at the peak wavelength) of both morphs at the end of each experiment under low (10 μmol photons m−2 s−1), medium (300 μmol photons m−2s−1), and high (1,100 μmol photons m−2s−1) intensities of blue light. Bars display mean values of 5 colonies. Error bars show standard deviation. Brackets above two bars indicate p-values for statistically significant (p < 0.05) differences between the fluorescence emission intensity of each morph at the end of each treatment, determined by one-way ANOVAs.
To measure photodamage of the coral symbionts, three colonies of each morph were placed either under 2,000 μmol photons m−2s−1 of blue light (~470 nm) provided by Aquaray Reef Blue LEDs or under the same photofluxes of orange light (~595 nm) produced by P4 Amber Star LEDs (Seoul Semiconductor, Korea, Smith et al., 2013). Light intensity was measured and adjusted as described above. The colonies were kept under blue light for 2 months and under orange light for 6 days. The maximum quantum yield (Fv/Fm) of the algal symbionts was measured using a Waltz Diving PAM as an indicator of photodamage (Warner et al., 1999). Measurements were taken after 14 h of darkness and colonies were exposed to the minimal light levels during measurements to minimize the effects of chlororespiration (Warner et al., 2010). Dark adapted Fv/Fm was used as an indicator of the efficiency of Photosystem II (Krause and Weis, 1991), and the degree of photodamage suffered by the zooxanthellae (Genty et al., 1989; Warner et al., 1999). A precision hole saw was used to remove circular tissue samples of identical size from the center of the treated area of the flat replicate colonies or from control regions in the periphery of the same corals. The tissue samples were homogenized in sterile seawater and zooxanthellae were isolated by differential centrifugation (1,000 g/10 min). After a wash step in sterile seawater, the algal cells were recovered by centrifugation, resuspended and their numbers were determined using a hemocytometer. Zooxanthellae numbers were then normalized to the surface of the cut-out samples.
Statistical Analysis
Coral growth and fluorescence intensity data were plotted and analyzed using GraphPad Prism 7. Paired t-tests were used to analyse weight data at the end of each experiment. The significance of the differences in fluorescence emission intensity between the two morphs under all light treatments were calculated with a fully orthogonal two-way ANOVA with fixed effects, followed by a Tukey's multiple comparison test. The significance of the differences in the fluorescence emission intensity of each morph at the end of each experiment were calculated with one-way ANOVAs, followed by Tukey's multiple comparison tests.
Fv/Fm data were plotted in GraphPad Prism 7. Data was log transformed prior to generation of linear models using R.
Zooxanthellae numbers from coral tissue samples were plotted and analyzed using GraphPad Prism 7. Unpaired t-tests were used to calculate the significance of the differences in zooxanthellae numbers from tissue samples of treated and untreated areas of tissue in both morphs after exposure to high intensities of blue and orange light.
Results
Characterization of the Color Morphs
The sequences of the ITS2 region isolated from both color morphs under study matched those of Symbiodinium C40a and C40b (GenBank accession numbers AY589747 and AY589748), indicating that the symbiont complement of both color morphs is dominated by the same Symbiodinium sp.
Both morphs contained CFPs, with a broad excitation spectrum ranging from ~350–500 nm with maxima at ~420, 443, and 473 nm (Figures 1A,B). Emission spectra peaked at ~492 nm (LF morph) and ~495 nm (HF morph), respectively. In colonies growing side by side under a photon flux of ~250 μmol photons m−2s−1 of white light illumination prior to the start of the experiment, the cyan fluorescence of the high fluorescent (HF) morph was approximately ~3.1-fold more intense compared to the low fluorescent (LF) morph (Figure 1A). The fluorescence maxima of each morph remained essentially unaltered throughout the light treatment experiment, while the intensity of the fluorescence emission changed under the different treatments (Figure 1D). The HF morph remained more fluorescent than the LF morph in all conditions, but the ratio (HF/LF) of the peak fluorescence emission intensity between the morphs ranged from ~8.0 (low light), ~6.9 (medium light), to ~3.4 (high light). Highest tissue fluorescence was shown at medium light (HF morph) and high light conditions (LF morph). Fluorescence in low light treated corals reached only ~30% (LF morph) to ~60% (HF morph) of these maximal values. A two-way ANOVA showed that the HF morph was significantly more fluorescent than the LF morph under all light condition (p < 0.001), but that there was a significant interaction between the light intensity and color morph (p = 0.0255). A one-way ANOVA showed significant differences in fluorescence of the LF morph between low and high, as well as medium and high light intensities (p = 0.0003 and p = 0.0191 respectively). Compared to the low light treatment, the fluorescence of the HF morph also increased significantly under medium light intensities (p = 0.0183) (Figure 1D).
Photodamage under Intense Blue and Orange Light
The efficiency of algal photosynthesis measured as Fv/Fm in both morphs decreased rapidly over the first 4 days of exposure to intense blue light (2,000 μmol photons m−2s−1, Figure 2A). During this initial phase, the Fv/Fm values from the LF morph were significantly lower compared to those from the HF morph [linear model: F(2, 21) = 5.944, p = 0.0042]. The response of the symbionts from the two morphs remained significantly different in the following period during which the values increased again [linear model: F(2, 201) = 148.1; p < 0.001]. After ~3 weeks, Fv/Fm values of the HF morph reached the initial value of >0.5. In contrast, the photosynthetic efficiency of the LF morph did not show full recovery and the Fv/Fm values remained below 0.5. No bleaching was visible in the HF morphs whereas visible bleaching started among replicates of the LF morphs after 4 days of exposure to blue light. By the end of the experiments, all LF specimens were bleached in the treated central area of the colonies. The HF morph, in contrast, showed only a mild paling in the light exposed areas.
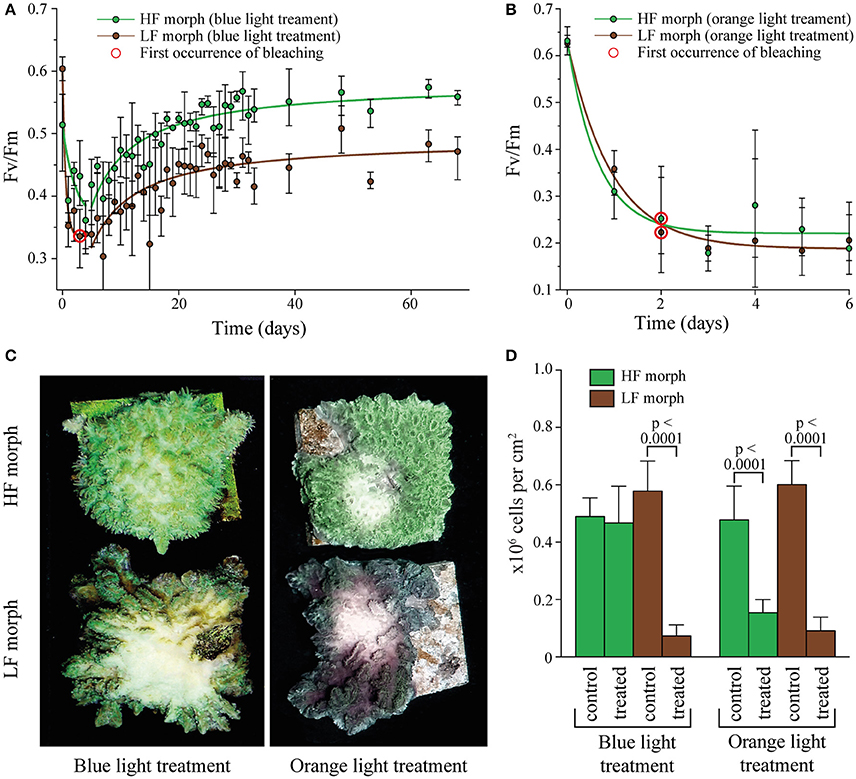
Figure 2. (A) Change in Fv/Fm in two color morphs of Hydnophora grandis under high intensity blue light (2,000 μmol photons m−2s−1). Error bars indicate the range of mean values of 3 replicate colonies. Red circles highlight the first occurrence of visible bleaching. Lines represent exponential decay and saturation fits, respectively. (B) Change in Fv/Fm in the HF and LF color morphs of Hydnophora grandis under high intensity orange light (2,000 μmol photons m−2s−1). Error bars indicate the range of mean values of 3 replicate colonies. The first occurrence of visible bleaching is marked by red circles. Lines represent exponential decay fits. (C) Photographs of representative colonies of the HF morph (top) and LF morph (bottom) taken at the end of the high intensity blue light (left) and orange light (right) treatments. (D) Concentration of zooxanthellae in tissue samples of both morphs after exposure to 2,000 μmol photons m−2s−1 of blue and orange light (treated) and control tissue samples exposed to ambient light intensities (control). Error bars show standard deviation. Brackets above two bars indicate p-values for statistically significant (p < 0.05) differences between the zooxanthellae concentrations in treated and untreated tissue samples of each morph under blue and orange light, determined using unpaired t-tests.
Under the same photon flux of orange light (2,000 μmol photons m−2s−1), symbionts of both morphs showed a rapid decrease in Fv/Fm values down to ~0.2 (Figure 2B). There was no significant difference in the response of both morphs to the treatment [linear model: F(2, 33) = 3.18, p = 0.48]. Bleaching was visible in both morphs after 2 days and became severe after 6 days when the experiment was terminated.
Analysis of zooxanthellae number of the treated parts of the experimental colonies and control areas showed that the visual bleaching (Figure 2C) was caused by a loss of algal cells. The treated regions of the LF colonies suffered significant losses of zooxanthellae under both blue and orange light exposure [unpaired t-test: F(1, 53) = 7.3, p < 0.001; F(1, 35) = 3.0, p < 0.001 respectively]. In contrast, the illuminated region of the HF morph lost significant amounts of zoothanthellae only under orange light [unpaired t-test: F(1, 35) = 6.5, p < 0.001] (Figure 2D).
Coral Growth under Different Light Intensities
Under low intensity blue light (Figure 3A), colonies of the LF morph showed a 25% gain in weight that was significantly higher [paired t-test: F(1, 4) = 3.8, p < 0.001] compared to the HF morph. The latter showed essentially no weight gain over the duration of the experiment. Under high intensities of blue light (Figure 3C), the colonies of the HF morph gained significantly more weight than the LF morph [paired t-test: F(1, 4) = 1.2, p = 0.045]. At the end of the experiment, the weight of the HF morph had increased by ~33%, compared to the ~18% increase of the LF morph colonies. Under medium intensity blue light (Figure 3B), both morphs showed comparable growth (HF morph: ~115%; LF morph 127%, non-significant difference). The growth rates of both morphs were substantially higher under medium light conditions compared to both high and low light treatments (Figure 3D).
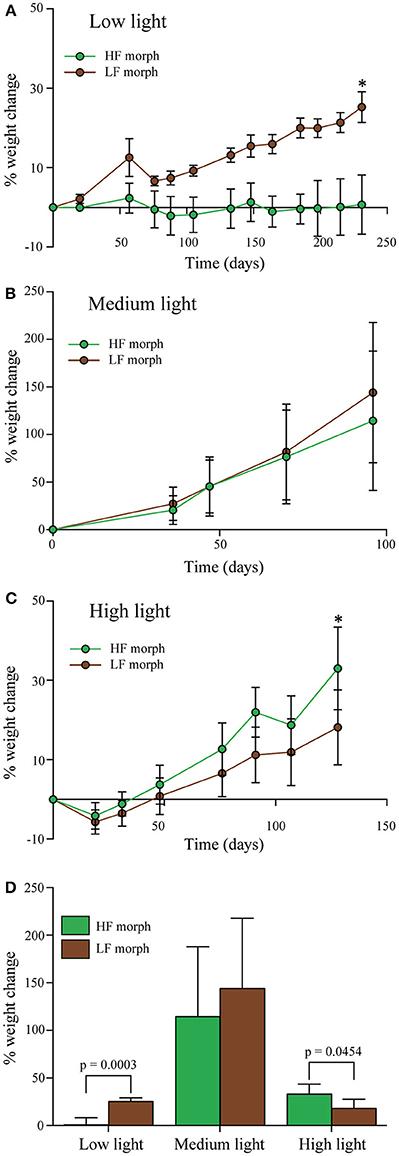
Figure 3. (A–C) Percentage change of wet weight of LF morph (brown circles) and HF morph (green circles) of Hydnophora grandis over time under three different intensities of blue light. (A) Low light (10 μmol photons m−2s−1), (B) Medium light (300 μmol photons m−2s−1), and (C) High light (700–1100 μmol photons m−2s−1). Bars display mean values of 5 colonies. Error bars show standard deviation. Asterisks (*) above data points indicate statistically significant (P < 0.05) differences between the weighs of the colonies of each morph at the end each experiment, determined using paired t-tests. Initial weight for each colony was set to zero. (D) Total weight change of each morph under each light intensity at the end of each experiment given as percent of the initial values. Error bars show standard deviation. Brackets above two bars indicate p-values for statistically significant (p < 0.05) differences between the weighs of the colonies of each morph at the end each experiment, determined using paired t-tests.
Discussion
The diversity of coral colors contributes to the visually arresting appearance of coral reefs. Frequently, representatives of different color morphs can be found side-by-side, raising the question of whether these striking differences in color have any ecological significance. Therefore, we tested our hypothesis that the benefits of photoprotection offered by some coral pigments in niches with high levels of light stress might be outweighed by the trade-offs associated with the productions of large pigment quantities in the absence of light stress. Such a scenario could offer an explanation for the evolution of color morphs as a result of balancing selection (Gittins et al., 2015). We chose the coral H. grandis as a model since the two distinct morphs differ significantly in the amount of cyan fluorescence proteins from the family of GFP-like proteins that they accumulate in their tissue (D'Angelo et al., 2008). The minimal difference in the spectral properties of the tissue fluorescence such as the 3 nm-shift of the emission peak between the LF morph expressing the protein hgrFP492 (D'Angelo et al., 2008) and the HF morph could be a response to the cellular environment in combination with the deviating pigment concentrations or due to small differences in the amino acid sequence of the proteins (Alieva et al., 2008). Importantly, in both morphs the host pigment absorbs incident photosynthetically active radiation in the blue-green spectral region, covering large sections of the in situ absorption spectrum of photosynthetic pigments of the algal symbionts (Smith et al., 2013). We showed that both morphs used in our study were associated with the same Symbiodinium subclade, thereby reducing, yet not excluding, the probability that differential responses were due to physiological differences associated with the taxonomic background of the symbionts (Robison and Warner, 2006).
We first tested whether the high CFP concentrations in the tissue of the HF morph could indeed protect the symbionts by screening excess light as it was previously demonstrated for the purple-blue chromoproteins and some green and red fluorescent proteins in shallow water corals (Salih et al., 2000; Smith et al., 2013; Gittins et al., 2015). Measuring changes in the photosynthetic efficiency after dark recovery (Warner et al., 2010) and loss of algal cells in response to exposure of high fluxes of blue light, we show that the HF morph was more tolerant to the light stress. In agreement with a wavelength-dependent screening function of the CFPs, both morphs displayed comparably intense signs of light stress when they were illuminated with orange light outside of the spectral window covered by the protective host pigment. Orange-red light has been shown to negatively affect the photophysiology of Symbiodinium in coral tissues (Smith et al., 2013; Wijgerde et al., 2014). The comparable susceptibility of the two morphs to photodamage in this spectral range supports the conclusion that higher resilience of the HF morph under blue light stress is due to the screening by the CFP rather than by other photoprotective mechanisms that might be more dominant in this morph. Our photodamage data are in line with results of an earlier study in which daytime photoinhibition of the algal symbionts of the green fluorescent morph of Acropora palifera was reduced as compared to less fluorescent morphs (Salih et al., 2000).
To test whether trade-offs are associated with the high-level expression of the photoprotective CFP, we monitored the growth of the HF and LF morphs under different intensities of blue light. Photon fluxes corresponded to light levels that can be measured around noon in depths of ~1 m (high light), ~15 m (medium light), and ~50–60 m (low light) (Stambler et al., 2008; Eyal et al., 2015; Ziegler et al., 2015). It should be noted that our experimental corals experienced these light levels for 10 h per day, not just around noon, so the dose of photons received by them per day was higher compared to corals from aforementioned depths in field settings. Corals showed highest growth rates at medium light intensity with no significant differences between the HF and LF morphs. This may indicate that at favorable light levels and in the absence of other forms of stress, the expression of photoprotective pigments may essentially be neutral in the overall performance of the organism. Such a scenario could be supported by the comparably low energetic costs associated with the production of individual pigment molecules (Leutenegger et al., 2007). However, a previous study has reported a higher tolerance of fluorescence corals to heat-stress-induced bleaching (Salih et al., 2000). It should therefore be considered that there might be effects on fitness parameters such as reproduction rate (Loya et al., 2004) or stress tolerance (Salih et al., 2000) that are not assessed by the present experimental set-up.
In the experimental treatments (high vs. low light) that simulated light conditions similar to those at the upper and lower depth distribution limits of several symbiotic reef corals (Eyal et al., 2015; Ziegler et al., 2015), the growth of the HF and LF morphs was strongly reduced in comparison with the medium light treatment. This indicates that both over- and undersupply of light can negatively affect the performance of the corals. Yet, the causes for the reduced growth rates under the two light levels are likely to be very different. A supply of excess light may require an increased investment in several forms of photoprotection, for example in the synthesis of mycosporine-like amino acids (Shick, 2004; Starcevic et al., 2010), antioxidant defense (Lesser and Shick, 1990), or alternative electron transport pathways such as the Mehler reaction (Roberty et al., 2014). Energy may also be invested in repair processes of the photosynthetic apparatus of the symbionts due to photoinhibition and subsequent damage to the D1 protein (Aro et al., 1993; Warner et al., 1999; Gorbunov et al., 2001; Hill and Takahashi, 2014), although it has been suggested that photoinhibiton may protect against oxidative damage caused by excess light (Adams et al., 2008). Furthermore, high light intensities have been shown to cause nutrient limitation of the zooxanthellae, leading to decreased productivity (Dubinsky and Jokiel, 1994). The associated redistribution of energy resources within the coral holobiont and reduction in productivity of the symbioses may result in lower growth rates observed in specimens from the high light treatment. At low photon fluxes, Symbiodinium photosynthesis provides only ~40% of the carbon needed by the coral host (Falkowski et al., 1984), and therefore colony growth may become carbon-limited (Dubinsky and Jokiel, 1994), a potential explanation for the reduced growth of our low light corals.
The growth rates of the two Hydnophora color morphs differed significantly under both extreme light conditions. In the high light treatment, the HF morph grew faster compared to the LF morph, suggesting that the high-level expression of a photoprotective CFP could indeed offer a measurable benefit for coral fitness in environments with exposure to excess light. In contrast, under low light levels, the highly pigmented HF specimens ceased to grow, while the LF colonies showed slow yet steady growth. Under these conditions, the expression of photoprotective pigments by the HF morph may represent a disadvantage since it requires an energy investment in the form of the accumulation of large amounts of proteins that offer no functional benefits. On the contrary, the screening of photons by the coral CFPs may actually be detrimental for the productivity of the symbionts since it further deprives photosynthesis from much-needed photons under light-limited conditions. The downregulation of FP expression under low light levels (D'Angelo et al., 2008), visible also in the present experiments, offers a mechanism to reduce these unfavorable side-effects. However, similar to the highly pigmented morphs of Acropora sp. (Gittins et al., 2015), this is possible only to a limited extend since the constitutive expression of photoprotective pigments of the HF morph still reaches ~60% of the maximal expression under high light.
Our study shows that, depending on the light environment, high-level expression of photoprotective GFP-like proteins can have either beneficial or detrimental effects on the fitness of symbiotic corals. Therefore, some form of niche partitioning across the steep light gradient characteristic for coral reefs could be expected. Indeed, corals expressing photoprotective chromoproteins (CPs) (Smith et al., 2013) are most abundant in 1 m depth (Salih et al., 2006). Their share of the total population decreased continuously to depths of 40 m. Also, fluorescent corals were most abundant in 1 m depth and their proportion decreased with depth. At the same time, non-pigmented corals became more abundant (Salih et al., 2006). Furthermore, a recent study showed that CFP-expressing corals were less numerous at lower end of their depth distribution range as compared to GFP-containing or non-fluorescent corals (Roth et al., 2015). Also, the color morphs of Anemonia viridis expressing high amounts of cyan and red fluorescent proteins as well as chromoproteins have their distribution maximum in the shallowest waters whereas the non-pigmented morph tends to be more abundant at greater depth (Wiedenmann et al., 1999). Furthermore, a fluorescent clone of this species was more successful than a non-pigmented clone in a long-term competition for space in a shallow water habitat (Wiedenmann et al., 2007). Future work should aim to provide insights in the mechanisms underlying the trade-offs associated with the high-level expression of GFP-like proteins.
We conclude that the extreme light conditions at the upper and lower end of the depth distribution range of symbiotic reef corals exposes them to opposing selective pressures that promote the evolution and persistence of intraspecific color polymorphism. However, as the present study shows, both color morphs performed comparably well under medium light in the absence of other forms of stress. This neutral behavior of the pigmentation under ambient conditions may explain why color morphs can co-exist even in shallow sites (Wiedenmann et al., 1999; Paley and Bay, 2012; Gittins et al., 2015) as the selective advantage of high level pigmentation may become only effective during sporadic episodes of increased stress (Salih et al., 2000; Paley and Bay, 2012). Thereby, color polymorphisms may diversify the niches that can be occupied by a coral species, a capacity that is further enhanced by the light intensity-driven regulation of genes encoding photoprotective GFP-like proteins (D'Angelo et al., 2008).
Notably, the neutral behavior of the pigmentation under ambient conditions together with the fact that multiple gene copies are involved in the color polymorphism could have been a major driver of the evolution of the staggering diversity of GFP-like proteins: mutated gene-copies may have been balanced by functional copies until a gain of function eventually stabilized the novel variants in the gene pool. Examples are chromoproteins in A. millepora that are almost exclusively expressed in larvae and have likely evolved from a red fluorescent ancestor protein that also gave rise to a red fluorescent variant with a photoprotective function in adults (Gittins et al., 2015). Interestingly, these larval CPs evolved independently from photoprotective CPs produced in adult corals (Gittins et al., 2015). Other examples include the green-to-red photoconvertible FPs (Ando et al., 2002; Wiedenmann et al., 2004; Oswald et al., 2007; Eyal et al., 2015) that have gained their red fluorescent chromophores by parallel evolution of a green ancestor protein (Field and Matz, 2010). Along with green fluorescent variants (Lyndby et al., 2016), the latter proteins are prevalent in coral species that live preferably in less light exposed habitats where they optimize the internal light environment for symbiont photosynthesis in deeper tissue levels (Bollati et al., 2017; Smith et al., 2017). Thereby, they offer an explanation for the observation that fluorescent corals become again more abundant at greater depth after initially decreasing from the shallowest sites downwards (Salih et al., 2006; Roth et al., 2015; Smith et al., 2017). Due to the different function of the pigments, strongly fluorescent morphs gain an advantage at greater depths (Smith et al., 2017), whereas the trade-offs associated with the high-level production of the pigments give a selective advantage to less colored variants in shallower water.
Our study highlights the critical need to assess different types of GFP-like proteins separately when analyzing their biological function and their ecological significance in structuring reef communities.
Author Contributions
CD and JW: provided the research question and designed experiments; CQ: conducted experiments and produced data; CD, JW, and CQ: analyzed and discussed the data; CQ, CD, and JW: wrote the paper.
Funding
The study was funded by NERC (NE/I01683X/1 & NE/K00641X/1 to JW), European Research Council under the European Union's Seventh Framework Programme (FP/2007-2013)/ERC Grant Agreement n. 311179 to JW and an IFLS studentship Award to CD.
Conflict of Interest Statement
The authors declare that the research was conducted in the absence of any commercial or financial relationships that could be construed as a potential conflict of interest.
Acknowledgments
We thank Tropical Marine Centre (London) and Tropic Marin (Wartenberg) for sponsoring the Coral Reef Laboratory at the University of Southampton.
References
Adams, W. W. III., Zarter, C. R., Mueh, K. E., Amiard, V., and Demmig-Adams, B. (2008). “Energy dissipation and photoinhibition: a continuum of photoprotection,” in Photoprotection, Photoinhibition, Gene Regulation, and Environment, eds B. Demmig-Adams, W. W. Adams III, and A. Mattoo (Dordrecht: Springer), 49–64.
Alieva, N. O., Konzen, K. A., Field, S. F., Meleshkevitch, E. A., Hunt, M. E., Beltran-Ramirez, V., et al. (2008). Diversity and evolution of coral fluorescent proteins. PLoS ONE 3:e2680. doi: 10.1371/journal.pone.0002680
Ambarsari, I., Brown, B. E., Barlow, R. G., Britton, G., and Cummings, D. (1997). Fluctuations in algal chlorophyll and carotenoid pigments during solar bleaching in the coral Goniastrea aspera at Phuket, Thailand. Mar. Ecol. Prog. Ser. 159, 303–307. doi: 10.3354/meps159303
Ando, R., Hama, H., Yamamoto-Hino, M., Mizuno, H., and Miyawaki, A. (2002). An optical marker based on the UV-induced green-to-red photoconversion of a fluorescent protein. Proc. Natl. Acad. Sci. U.S.A. 99, 12651–12656. doi: 10.1073/pnas.202320599
Aro, E. M., Virgin, I., and Andersson, B. (1993). Photoinhibition of photosystem-2 - inactivation, protein damage and turnover. Biochim. Biophys. Acta 1143, 113–134. doi: 10.1016/0005-2728(93)90134-2
Baker, A. C., Glynn, P. W., and Riegl, B. (2008). Climate change and coral reef bleaching: an ecological assessment of long-term impacts, recovery trends and future outlook. Estuar. Coast. Shelf Sci. 80, 435–471. doi: 10.1016/j.ecss.2008.09.003
Banaszak, A. T., LaJeunesse, T. C., and Trench, R. K. (2000). The synthesis of mycosporine-like amino acids (MAAs) by cultured, symbiotic dinoflagellates. J. Exp. Mar. Biol. Ecol. 249, 219–233. doi: 10.1016/S0022-0981(00)00192-1
Bhagooli, R., and Hidaka, M. (2003). Comparison of stress susceptibility of in hospite and isolated zooxanthellae among five coral species. J. Exp. Mar. Biol. Ecol. 291, 181–197. doi: 10.1016/S0022-0981(03)00121-7
Bollati, E., Plimmer, D., D'Angelo, C., and Wiedenmann, J. (2017). FRET-mediated long-range wavelength transformation by photoconvertible fluorescent proteins as an efficient mechanism to generate orange-red light in symbiotic deep water corals. Int. J. Mol. Sci. 18:1174. doi: 10.3390/ijms18071174
D'Angelo, C., Denzel, A., Vogt, A., Matz, M. V., Oswald, F., Salih, A., et al. (2008). Blue light regulation of host pigment in reef-building corals. Mar. Ecol. Prog. Ser. 364, 97–106. doi: 10.3354/meps07588
D'Angelo, C., Hume, B. C., Burt, J., Smith, E. G., Achterberg, E. P., and Wiedenmann, J. (2015). Local adaptation constrains the distribution potential of heat-tolerant Symbiodinium from the Persian/Arabian Gulf. Isme J. 9, 2551–2560. doi: 10.1038/ismej.2015.80
D'Angelo, C., and Wiedenmann, J. (2012). An experimental mesocosm for long-term studies of reef corals. J. Mar. Biol. Assoc. U.K. 92, 769–775. doi: 10.1017/S0025315411001883
D'Angelo, C., and Wiedenmann, J. (2014). Impacts of nutrient enrichment on coral reefs: new perspectives and implications for coastal management and reef survival. Curr. Opin. Environ. Sustain. 7, 82–93. doi: 10.1016/j.cosust.2013.11.029
Dove, S. G., Hoegh-Guldberg, O., and Ranganathan, S. (2001). Major colour patterns of reef-building corals are due to a family of GFP-like proteins. Coral Reefs 19, 197–204. doi: 10.1007/PL00006956
Dove, S. G., Takabayashi, M., and Hoegh-Guldberg, O. (1995). Isolation and partial characterization of the pink and blue pigments of pocilloporid and acroporid corals. Biol. Bull. 189, 288–297. doi: 10.2307/1542146
Dubinsky, Z., and Jokiel, P. L. (1994). Ratio of energy and nutrient fluxes regulates symbiosis between zooxanthellae and corals. Pac. Sci. 48, 313–324.
Eyal, G., Wiedenmann, J., Grinblat, M., D'Angelo, C., Kramarsky-Winter, E., Treibitz, T., et al. (2015). Spectral diversity and regulation of coral fluorescence in a mesophotic reef habitat in the Red Sea. PLoS ONE 10:e0128697. doi: 10.1371/journal.pone.0128697
Falkowski, P. G., Dubinsky, Z., Muscatine, L., and Porter, J. W. (1984). Light and the bioenergetics of a symbiotic coral. Bioscience 34, 705–709. doi: 10.2307/1309663
Field, S. F., Bulina, M. Y., Kelmanson, I. V., Bielawski, J. P., and Matz, M. V. (2006). Adaptive evolution of multicolored fluorescent proteins in reef-building corals. J. Mol. Evol. 62, U332–U315. doi: 10.1007/s00239-005-0129-9
Field, S. F., and Matz, M. V. (2010). Retracing evolution of red fluorescence in GFP-like proteins from Faviina corals. Mol. Biol. Evol. 27, 225–233. doi: 10.1093/molbev/msp230
Genty, B., Briantais, J. M., and Baker, N. R. (1989). The relationship between the quantum yield of photosynthetic electron-transport and quenching of chlorophyll fluorescence. Biochim. Biophys. Acta 990, 87–92. doi: 10.1016/S0304-4165(89)80016-9
Gittins, J. R., D'Angelo, C., Oswald, F., Edwards, R. J., and Wiedenmann, J. (2015). Fluorescent protein-mediated colour polymorphism in reef corals: multicopy genes extend the adaptation/acclimatization potential to variable light environments. Mol. Ecol. 24, 453–465. doi: 10.1111/mec.13041
Glynn, P. W., Maté, J. L., Baker, A. C., and Calderón, M. O. (2001). Coral bleaching and mortality in Panama and Ecuador during the 1997–1998 El Ni-o–Southern Oscillation event: spatial/temporal patterns and comparisons with the 1982–1983 event. Bull. Mar. Sci. 69, 79–109.
Gorbunov, M. Y., Kolber, Z. S., Lesser, M. P., and Falkowski, P. G. (2001). Photosynthesis and photoprotection in symbiotic corals. Limnol. Oceanogr. 46, 75–85. doi: 10.4319/lo.2001.46.1.0075
Henderson, J. N., and Remington, S. J. (2005). Crystal structures and mutational analysis of amFP486, a cyan fluorescent protein from Anemonia majano. Proc. Natl. Acad. Sci. U.S.A. 102, 12712–12717. doi: 10.1073/pnas.0502250102
Hill, R., and Takahashi, S. (2014). Photosystem II recovery in the presence and absence of chloroplast protein repair in the symbionts of corals exposed to bleaching conditions. Coral Reefs 33, 1101–1111. doi: 10.1007/s00338-014-1188-4
Hoegh-Guldberg, O. (1999). Climate change, coral bleaching and the future of the world's coral reefs. Mar. Freshw. Res. 50, 839–866. doi: 10.1071/MF99078
Hughes, T. P., Kerry, J. T., Álvarez-Noriega, M., Álvarez-Romero, J. G., Anderson, K. D., Baird, A. H., et al. (2017). Global warming and recurrent mass bleaching of corals. Nature 543, 373–377. doi: 10.1038/nature21707
Hume, B., D'Angelo, C., Burt, J., Baker, A. C., Riegl, B., and Wiedenmann, J. (2013). Corals from the Persian/Arabian Gulf as models for thermotolerant reef-builders: prevalence of clade C3 Symbiodinium, host fluorescence and ex situ temperature tolerance. Mar. Pollut. Bull. 72, 313–322. doi: 10.1016/j.marpolbul.2012.11.032
Jones, R. J., Hoegh-Guldberg, O., Larkum, A. W. D., and Schreiber, U. (1998). Temperature-induced bleaching of corals begins with impairment of the CO2 fixation mechanism in zooxanthellae. Plant Cell Environ. 21, 1219–1230. doi: 10.1046/j.1365-3040.1998.00345.x
Kelmanson, I. V., and Matz, M. V. (2003). Molecular basis and evolutionary origins of color diversity in great star coral Montastraea cavernosa (Scleractinia: Faviida). Mol. Biol. Evol. 20, 1125–1133. doi: 10.1093/molbev/msg130
Klueter, A., Loh, W., Hoegh-Guldberg, O., and Dove, S. (2006). Physiological and genetic properties of two fluorescent colour morphs of the coral Montipora digitata. Symbiosis 42, 123–134.
Krause, G. H., and Weis, E. (1991). Chlorophyll fluorescence and photosynthesis - the basics. Annu. Rev. Plant Physiol. Plant Molec. Biol. 42, 313–349. doi: 10.1146/annurev.pp.42.060191.001525
Lesser, M. P. (1997). Oxidative stress causes coral bleaching during exposure to elevated temperatures. Coral Reefs 16, 187–192. doi: 10.1007/s003380050073
Lesser, M. P. (2006). Oxidative stress in marine environments: biochemistry and physiological ecology. Annu. Rev. Physiol. 68, 253–278. doi: 10.1146/annurev.physiol.68.040104.110001
Lesser, M. P., and Shick, J. M. (1990). Photoadaption and defenses against oxygen toxicity in zooxanthellae from natural populations of symbiotic cnidarians. J. Exp. Mar. Biol. Ecol. 134, 129–141. doi: 10.1016/0022-0981(90)90105-L
Leutenegger, A., D'Angelo, C., Matz, M. V., Denzel, A., Oswald, F., Salih, A., et al. (2007). It's cheap to be colorful. Anthozoans show a slow turnover of GFP-like proteins. FEBS J. 274, 2496–2505. doi: 10.1111/j.1742-4658.2007.05785.x
Levy, O., Achituv, Y., Yacobi, Y. Z., Stambler, N., and Dubinsky, Z. (2006). The impact of spectral composition and light periodicity on the activity of two antioxidant enzymes (SOD and CAT) in the coral Favia favus. J. Exp. Mar. Biol. Ecol. 328, 35–46. doi: 10.1016/j.jembe.2005.06.018
Loya, Y., Lubinevsky, H., Rosenfeld, M., and Kramarsky-Winter, E. (2004). Nutrient enrichment caused by in situ fish farms at Eilat, Red Sea is detrimental to coral reproduction. Mar. Pollut. Bull. 49, 344–353. doi: 10.1016/j.marpolbul.2004.06.011
Lyndby, N. H., Kühl, M., and Wangpraseurt, D. (2016). Heat generation and light scattering of green fluorescent protein-like pigments in coral tissue. Sci. Rep. 6:26599. doi: 10.1038/srep26599
Matz, M. V., Fradkov, A. F., Labas, Y. A., Savitsky, A. P., Zaraisky, A. G., Markelov, M. L., et al. (1999). Fluorescent proteins from nonbioluminescent Anthozoa species. Nat. Biotechnol. 17, 1227. doi: 10.1038/70787
Mazel, C. H., Lesser, M. P., Gorbunov, M. Y., Barry, T. M., Farrell, J. H., Wyman, K. D., et al. (2003). Green-fluorescent proteins in Caribbean corals. Limnol. Oceanogr. 48, 402–411. doi: 10.4319/lo.2003.48.1_part_2.0402
Nienhaus, G. U., and Wiedenmann, J. (2009). Structure, dynamics and optical properties of fluorescent proteins: perspectives for marker development. Chemphyschem 10, 1369–1379. doi: 10.1002/cphc.200800839
Oswald, F., Schmitt, F., Leutenegger, A., Ivanchenko, S., D'Angelo, C., Salih, A., et al. (2007). Contributions of host and symbiont pigments to the coloration of reef corals. FEBS J. 274, 1102–1109. doi: 10.1111/j.1742-4658.2007.05661.x
Paley, A. S., and Bay, L. K. (2012). “Bleaching condition varies among Acropora millepora color morphs,” in Proceedings of the 12th International Coral Reef Symposium (Cairns, QLD), 1–5.
Pospíšil, P. (2016). Production of reactive oxygen species by photo system ii as a response to light and temperature stress. Front. Plant Sci. 7:1950. doi: 10.3389/fpls.2016.01950
Rehman, A. U., Szabó, M., Deák, Z., Sass, L., Larkum, A., Ralph, P., et al. (2016). Symbiodinium sp cells produce light-induced intra- and extracellular singlet oxygen, which mediates photodamage of the photosynthetic apparatus and has the potential to interact with the animal host in coral symbiosis. New Phytol. 212, 472–484. doi: 10.1111/nph.14056
Reynolds, J. M., Bruns, B. U., Fitt, W. K., and Schmidt, G. W. (2008). Enhanced photoprotection pathways in symbiotic dinoflagellates of shallow-water corals and other cnidarians. Proc. Natl. Acad. Sci. U.S.A. 105, 13674–13678. doi: 10.1073/pnas.0805187105
Roberty, S., Bailleul, B., Berne, N., Franck, F., and Cardol, P. (2014). PSI Mehler reaction is the main alternative photosynthetic electron pathway in Symbiodinium sp., symbiotic dinoflagellates of cnidarians. New Phytol. 204, 81–91. doi: 10.1111/nph.12903
Robison, J. D., and Warner, M. E. (2006). Differential impacts of photoacclimation and thermal stress on the photobiology of four different phylotypes of Symbiodinium (Pyrrhophyta). J. Phycol. 42, 568–579. doi: 10.1111/j.1529-8817.2006.00232.x
Rosset, S., D'Angelo, C., and Wiedenmann, J. (2015). Ultrastructural biomarkers in symbiotic algae reflect the availability of dissolved inorganic nutrients and particulate food to the reef coral holobiont. Front. Mar. Sci. 2:103. doi: 10.3389/fmars.2015.00103
Rosset, S., Wiedenmann, J., Reed, A. J., and D'Angelo, C. (2017). Phosphate deficiency promotes coral bleaching and is reflected by the ultrastructure of symbiotic dinoflagellates. Mar. Pollut. Bull. 118, 180–187. doi: 10.1016/j.marpolbul.2017.02.044
Roth, M. S., Padilla-Gamino, J. L., Pochon, X., Bidigare, R. R., Gates, R. D., Smith, C. M., et al. (2015). Fluorescent proteins in dominant mesophotic reef-building corals. Mar. Ecol. Prog. Ser. 521, 63–79. doi: 10.3354/meps11108
Salih, A., Cox, G., Szymczak, R., Coles, S. L., Baird, A. H., Dunstan, A., et al. (2006). “The role of host-based color and fluorescent pigments in photoprotection and in reducing bleaching stress in corals”, in Proceedings of 10th International Coral Reef Symposium (Okinawa), 746–756.
Salih, A., Larkum, A., Cox, G., Kühl, M., and Hoegh-Guldberg, O. (2000). Fluorescent pigments in corals are photoprotective. Nature 408, 850–853. doi: 10.1038/35048564
Shick, J. M. (2004). The continuity and intensity of ultraviolet irradiation affect the kinetics of biosynthesis, accumulation, and conversion of mycosporine-like amino acids (MAAS) in the coral Stylophora pistillata. Limnol. Oceanogr. 49, 442–458. doi: 10.4319/lo.2004.49.2.0442
Smith, E. G., D'Angelo, C., Salih, A., and Wiedenmann, J. (2013). Screening by coral green fluorescent protein (GFP)-like chromoproteins supports a role in photoprotection of zooxanthellae. Coral Reefs 32, 463–474. doi: 10.1007/s00338-012-0994-9
Smith, E. G., D'Angelo, C., Sharon, Y., Tchernov, D., and Wiedenmann, J. (2017). Acclimatization of symbiotic corals to mesophotic light environments through wavelength transformation by fluorescent protein pigments. Proc. Biol. Sci. 284:20170320. doi: 10.1098/rspb.2017.0320
Stambler, N., Levy, O., and Vaki, L. (2008). Photosynthesis and respiration of hermatypic zooxanthellate Red Sea corals from 5-75-m depth. Isr. J. Plant Sci. 56, 45–53. doi: 10.1560/IJPS.56.1-2.45
Starcevic, A., Dunlap, W. C., Cullum, J., Shick, J. M., Hranueli, D., and Long, P. F. (2010). Gene expression in the scleractinian acropora microphthalma exposed to high solar irradiance reveals elements of photoprotection and coral bleaching. PLoS ONE 5:e13975. doi: 10.1371/journal.pone.0013975
Takahashi, S., Whitney, S. M., and Badger, M. R. (2009). Different thermal sensitivity of the repair of photodamaged photosynthetic machinery in cultured Symbiodinium species. Proc. Natl. Acad. Sci. U.S.A. 106, 3237–3242. doi: 10.1073/pnas.0808363106
Vogt, A., D'Angelo, C., Oswald, F., Denzel, A., Mazel, C. H., Matz, M. V., et al. (2008). A green fluorescent protein with photoswitchable emission from the Deep Sea. PLoS ONE 3:e3766. doi: 10.1371/journal.pone.0003766
Warner, M. E., Fitt, W. K., and Schmidt, G. W. (1999). Damage to photosystem II in symbiotic dinoflagellates: a determinant of coral bleaching. Proc. Natl. Acad. Sci. U.S.A. 96, 8007–8012. doi: 10.1073/pnas.96.14.8007
Warner, M. E., Lesser, M. P., and Ralph, P. J. (2010). “Chlorophyll fluorescence in reef building corals,” in Chlorphyll a Fluorescence in Aquatic Sciences: Methods and Applications, eds D. J. Suggett, O. Prasil, and M. A. Borowitzka (Dordrecht: Springer), 209–222. doi: 10.1007/978-90-481-9268-7_10
Wiedenmann, J., Ivanchenko, S., Oswald, F., Schmitt, F., Röcker, C., Salih, A., et al. (2004). EosFP, a fluorescent marker protein with UV-inducible green-to-red fluorescence conversion. Proc. Natl. Acad. Sci. U.S.A. 101, 15905–15910. doi: 10.1073/pnas.0403668101
Wiedenmann, J., Leutenegger, A., Gundel, S., Schmitt, F., D'Angelo, C., and Funke, W. (2007). Long-term monitoring of space competition among fluorescent and nonfluorescent sea anemones in the Mediterranean Sea. J. Mar. Biol. Assoc. U.K. 87, 851–852. doi: 10.1017/S0025315407057050
Wiedenmann, J., Röcker, C., and Funke, W. (1999). “The morphs of Anemonia aff. sulcata (Cnidaria, Anthozoa) in particular consideration of the ectodermal pigments,” in Verhandlungen der Gesellschaft für Ökologie, ed J. Pfadenhauer (Heidelberg: Spektrum Akademischer Verlag), 497–503.
Wiedenmann, J., Schenk, A., Röcker, C., Girod, A., Spindler, K. D., and Nienhaus, G. U. (2002). A far-red fluorescent protein with fast maturation and reduced oligomerization tendency from Entacmaea quadricolor (Anthozoa, Actinaria). Proc. Natl. Acad. Sci. U.S.A. 99, 11646–11651. doi: 10.1073/pnas.182157199
Wijgerde, T., van Melis, A., Silva, C. I., Leal, M. C., Vogels, L., Mutter, C., et al. (2014). Red light represses the photophysiology of the Scleractinian coral Stylophora pistillata. PLoS ONE 9:e92781. doi: 10.1371/journal.pone.0092781
Keywords: coral color, green fluorescent protein, photoprotection, color polymorphism, balancing selection, light stress, coral bleaching
Citation: Quick C, D'Angelo C and Wiedenmann J (2018) Trade-Offs Associated with Photoprotective Green Fluorescent Protein Expression as Potential Drivers of Balancing Selection for Color Polymorphism in Reef Corals. Front. Mar. Sci. 5:11. doi: 10.3389/fmars.2018.00011
Received: 12 April 2017; Accepted: 16 January 2018;
Published: 06 February 2018.
Edited by:
Daniel Wangpraseurt, University of Cambridge, United KingdomReviewed by:
Anya Salih, Western Sydney University, AustraliaAnthony William Larkum, University of Technology Sydney, Australia
Aldo Cróquer, Simón Bolívar University, Venezuela
Copyright © 2018 Quick, D'Angelo and Wiedenmann. This is an open-access article distributed under the terms of the Creative Commons Attribution License (CC BY). The use, distribution or reproduction in other forums is permitted, provided the original author(s) and the copyright owner are credited and that the original publication in this journal is cited, in accordance with accepted academic practice. No use, distribution or reproduction is permitted which does not comply with these terms.
*Correspondence: Jörg Wiedenmann, am9lcmcud2llZGVubWFubkBub2Muc290b24uYWMudWs=