- 1Australian Institute of Marine Science, Townsville, QLD, Australia
- 2AIMS@JCU, Australian Institute of Marine Science, College of Marine and Environmental Sciences, James Cook University, Townsville, QLD, Australia
- 3College of Marine and Environmental Sciences, James Cook University, Townsville, QLD, Australia
- 4School of BioSciences, University of Melbourne, Parkville, VIC, Australia
Coral reefs are under major threat from ocean warming. When temperatures become too high corals bleach, expelling their symbiotic, photosynthetic microalgae (Symbiodinium), which they depend on for much of their nutritional requirements. Prolonged bleaching has led to widespread coral mortality and the severity and frequency of bleaching events are predicted to increase in the future. Coral bleaching tolerance is influenced by the thermal tolerance of the Symbiodinium harboured, and these microbial members of the coral holobiont may be able to evolve more rapidly than the coral host itself. Here, we examined the response of replicate cultures of five genetically distinct Symbiodinium strains (A3c, two types of D1, G3, and F1) to increasing temperatures over the course of approximately one year. For three Symbiodinium types (types A3c, G3, and F1), we observed a stable adaptive change at the end of this exposure period, which equated to only 41–69 asexual generations. The long-term selected Symbiodinium culture replicates (SS) showed faster growth rates under short-term, acute heat stress, and in some cases higher photosynthetic efficiencies, compared to wild-type populations (WT). Our results considerably extend the field of experimental evolution in Symbiodinium and with further work into the Symbiodinium-coral association and bleaching response, this approach may become a valuable tool in coral reef conservation and restoration initiatives.
Introduction
Coral reefs are the most biodiverse ecosystem in the marine world, contributing billions of dollars' worth of ecosystem services through tourism, coastal protection and fishing industries, with invaluable cultural and medicinal significance (Moberg and Folke, 1999). However, reef-building corals are under serious threat from rapid ocean warming, driven by global warming, as a consequence of rising anthropogenic carbon emissions. The unprecedented rate of global change is considered too rapid for many marine organisms to keep up, leading to the prediction of mass species extinction by the end of the century (Hoegh-Guldberg and Bruno, 2010; Pereira et al., 2010; Dawson et al., 2011; Pacifici et al., 2015; Urban, 2015). Increases in temperature are one of the main causes of coral bleaching, whereby corals' symbiotic algae, Symbiodinium spp., are lost from coral tissue in a stress response driven by the Symbiodinium and/or the coral (Pandolfi et al., 2011). Corals meet most of their energy requirements from the Symbiodinium living inside their cells (Falkowski et al., 1984) and although corals can survive without their photosymbionts for a limited period of time, prolonged bleaching leads to coral starvation and will ultimately lead to death. Even if corals can re-establish a symbiosis when temperatures return to normal, they may be left prone to disease (e.g., Kushmaro et al., 1997; Harvell et al., 1999; Howells et al., 2016), show reduced growth and can be reproductively compromised (e.g., Baird and Marshall, 2002; Miller et al., 2009)
Rising ocean temperatures have triggered three global mass bleaching events (1998, 2010, and 2014–2017) since records began in the 1980s. Not only have bleaching events become more extreme and widespread, but the time between successive events has declined by 4.6-fold from the early 1980s (Hughes et al., 2017), giving surviving coral less time to recover. Ocean warming is unlikely to be curbed, with models showing only a 5% chance that the global temperature increase since pre-industrial times will be less than 2°C by 2050 (Raftery et al., 2017). To preserve just ≤ 10% of the world's coral reefs, it has been predicted that warming must be limited to below 1.5°C (Frieler et al., 2013). Some marine species may be able to adapt, acclimate or simply move to cooler places in response to rapid ocean warming (Cheung et al., 2009), however for reef-building corals that have relatively long generation times (4–20 years, Babcock, 1991) are already near their physiological thermal limits and are sessile, such options are limited. Therefore, the need to develop measures that enhance the thermal resilience of corals is pressing.
Assisted evolution is the acceleration of naturally occurring evolutionary processes to enhance certain traits; it involves a set of direct interventions and aims to increase coral climate resilience (van Oppen et al., 2015, 2017). One approach that has recently been pioneered is to make use of the comparatively short generation time of the asexually reproducing coral photosymbionts, Symbiodinium, by subjecting them to laboratory thermal selection. Symbiodinium generation times in hospite correspond to between three and 74 days (Muscatine et al., 1984; Hoegh-Guldberg et al., 1987; Wilkerson et al., 1988) and may be restricted by the host through the amount of nutrients provided, host digestion and expulsion (Muscatine and Pool, 1979; Cook et al., 1988; Titlyanovl et al., 1996; Stat et al., 2008; Cantin et al., 2009), which could limit their rate of adaptation. However, in culture, typical doubling times for Symbiodinium are often less than three days (Fitt and Trench, 1983; Kinzie et al., 2001; Taguchi, 2001), and in a laboratory environment, Symbiodinium can be cultured ex-hospite under strong thermal selection conditions that encourage large population sizes and minimise generation times, thus maximising the rate of genetic adaptation (Bromham, 2009). As coral survival is highly dependent on the thermal performance of their symbionts, such research may hold the key to reducing the extent of coral bleaching under thermal stress (van Oppen et al., 2015; Chakravarti et al., 2017).
The genus Symbiodinium currently comprises nine phylogenetic clades (A-I; Pochon and Gates, 2010), and each clade consists of multiple types (e.g., van Oppen et al., 2005b). Corals can host multiple types simultaneously (Mieog et al., 2007; Silverstein et al., 2012; Boulotte et al., 2016) and dominant Symbiodinium types in hospite can vary in relative abundance, depending on coral life-stage (e.g., Little et al., 2004) and environmental factors (e.g., Baker et al., 2004; Rowan, 2004). Most importantly, the association of specific Symbiodinium types might alter the thermal stress tolerance of the holobiont (Berkelmans and van Oppen, 2006; Sampayo et al., 2008; Abrego et al., 2009; Mieog et al., 2009; Stat and Gates, 2011; Howells et al., 2012). Members of Symbiodinium clade D in particular have attracted attention as they have been linked to high bleaching tolerance in corals, where under heat stress, they out-compete other, perhaps more functionally beneficial Symbiodinium (reviewed by Stat et al., 2008). However, Chakravarti et al. (2017) demonstrated that for a laboratory evolved strain of Symbiodinium C1 that had a widened thermal niche, there was limited transfer of heat tolerance when in symbiosis. Such responses could be strain-specific and thus it is important to examine the response of a wide taxonomic diversity of Symbiodinium strains to temperature selection for their potential use in assisting coral evolution.
Here, we carried out a laboratory selection experiment with five different types of Symbiodinium isolated from the Great Barrier Reef (GBR, types A3, two strains of D1, F1, and G3), by exposing cultures to increasing temperatures, up to 34°C, for 1 year. Subsequently, we investigated the thermal tolerances of these selected Symbiodinium (SS) cultures relative to the unselected wild-type (WT) cultures that had been kept under control temperature conditions of 27°C.
Materials and Methods
Symbiodinium Isolation and Culture
Five strains of Symbiodinium were isolated from four species of coral and one species of giant clam from the central and southern Great Barrier Reef (Table 1). Types F1 and A3c were obtained from the University of Technology Sydney (Table 1), and are described in (Suggett et al., 2015) while the remaining strains were isolated and cultured at the Australian Institute of Marine Science. This involved air-brushing the coral tissue, centrifuging (5 min, 1,600 g), decanting, and re-suspending the pellet three times in 0.2 μm filtered seawater. Isolated cells were transferred into sterile culture media, Daigo's IMK for Marine Microalgae (Nihon Pharmaceutical Co., Ltd) containing antibiotics; penicillin, neomycin, streptomycin, nystatin (final concentration 100 μg mL−1), amphotericin (final concentration 2.5 μg mL−1), and GeO2 (final concentration 50 μM, (Beltran et al., 2012)). To minimise bacterial growth, cells were inoculated into fresh IMK+antibiotics monthly, for five months. Each culture was genotyped based on the ITS2 rDNA region and annual sequencing checks confirmed that the cultures did not become contaminated during longer-term laboratory culture. Cultures were maintained at 27°C in an environmental chamber (Steridium, er-rh-500) and 65 ± 10 μmol photons m−2 s−1 (Sylvania FHO24W/T5/865 fluorescent tubes) under a 14:10 light:dark cycle. It is important to note that isolation of symbionts from hosts may not necessarily represent their dominant symbiont type. Additionally, it is possible that the Symbiodinium strains cultured could have either represented free-living cells, perhaps associated with the corals surface, or symbiotic cells harboured within the hosts' tissues. However, in preliminary trials, all five strains were able to infect the coral species Acropora tenuis and A. millepora suggesting that they are true symbionts (LJC, unpubl.).
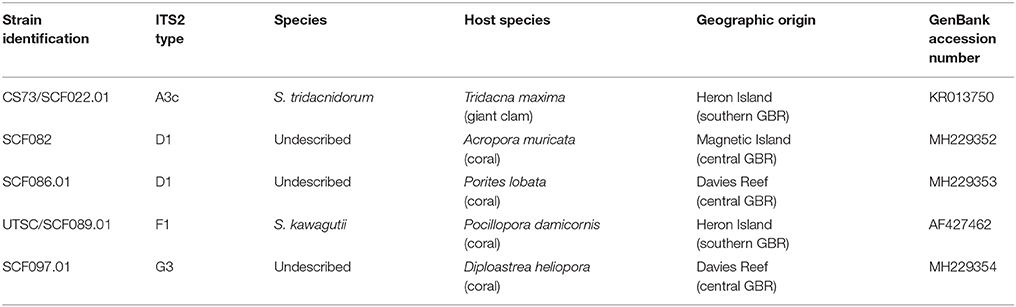
Table 1. Symbiont species, type (ITS2), host species and site of origin for each Symbiodinium strain used in this study.
Symbiodinium Thermal Selection Experiment
Experimental Design
A thermal selection experiment was carried out using a modified ratchet design (Huertas et al., 2011, Figure 1). The ratchet design is a method used to maximise the number of spontaneous mutations arising from asexual cell division, by maintaining large population sizes while increasing levels of thermal selection pressure in a step-wise fashion.
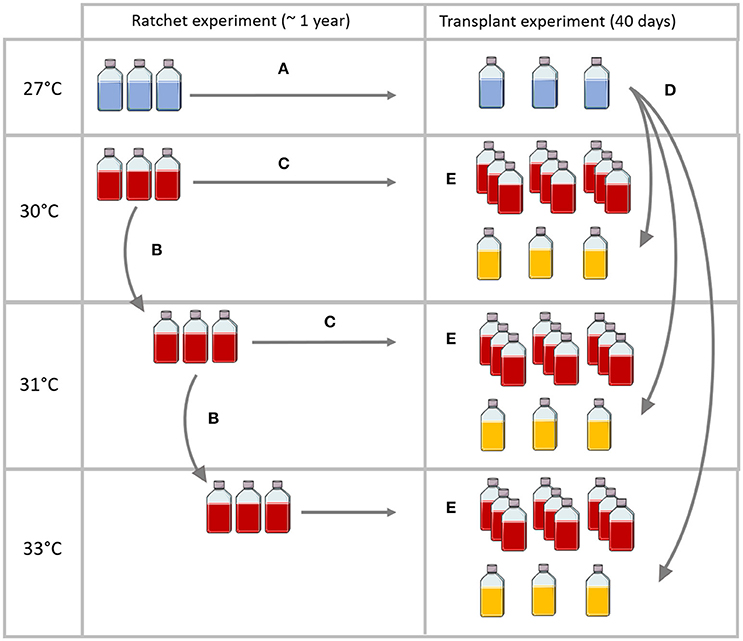
Figure 1. Simplified experimental design to show long-term thermal selection (ratchet experiment) ending in an acute heat stress transplantation experiment. (A) Control replicate cultures were kept at 27°C throughout the duration of the long-term selection experiment. (B) Replicate populations were moved to the next, elevated temperature treatment if after six weeks they exhibited positive growth. (C) For populations that were eligible to be transferred to the next temperature treatment, an aliquot was also kept at the same temperature conditions throughout the experiment. (D) After one year, control replicate cultures were transferred to each of the elevated temperature treatments that the temperature-selected populations had achieved (E) after one year of thermal selection, thermally selected populations were split into three replicate cultures and maintained in their own temperature conditions. A 40-day transplant experiment commenced.
Our ratchet experiment involved five temperature treatments starting at control temperature conditions of 27°C (27.04°C ± 0.002, n = 54275) and 30°C (30.06°C ± 0.0008, n = 54347), with further 1–2°C increments; 31°C (31.17°C ± 0.002, n = 48123), 33°C (32.78°C ± 0.006, n = 35702) and 34°C (34.04°C ± 0.001, n = 14045), under the same light conditions as their prior culture (65 ± 10 μmol photons m−2 s 14:10 light:dark cycle) and in five temperature-controlled environmental chambers (Steridium, er-rh-500). Temperature measurements were recorded every 10 min, using a data logger (HOBO Pendant®). To begin the ratchet experiment, replicate control cultures (n = 3) of each strain were inoculated into fresh media at 100,000 cells mL−1 to give a final volume of 20 mL of culture and kept at 27°C (control) and the first treatment temperature of 30°C (Figure 1). This jump in temperature from 27°C to 30°C was chosen based on previous observations showing most Symbiodinium strains can survive 30°C. Importantly, once the experiment started, each “replicate” culture at 30°C (or subsequent temperature treatments) was no longer considered a replicate but an independent evolutionary unit in which different random mutations could arise (Huertas et al., 2011). The first ratchet level consisted of populations (both control and treatment) being cultured for a three-week pre-acclimation period before being inoculated into fresh media at the same starting concentration of 100,000 cells mL−1 and being cultured for a further three weeks before being transferred to fresh media again. Every three weeks, aliquots of each population were taken to determine the growth rate, which was used to calculate the number of generations each replicate had gone through (see section Growth Rate and Number of Cell Generations for calculation of growth rate number of generations).
At the end of the six-week ratchet, if an individual population at 30°C showed positive growth, the population was transferred into the next temperature treatment (31°C), into fresh media at 100,000 cells mL−1. If a population did not display positive growth it remained at the same temperature until the next ratchet cycle when growth performance was assessed again. The experiment continued in this way, with populations being transferred to incremental temperature increases every six weeks, where appropriate (Figure 1).
Our ratchet experimental design differed from that of Huertas et al. (2011) in three ways. Firstly, we allowed six weeks before assessing the growth of each population and deciding to move a population(s), whereas microalgal growth was assessed after only 15–20 days in Huertas et al. (2011). Six weeks at each treatment temperature was important in our experiment because we observed that for one strain (type D1, SCF086) the full, negative effects of elevated temperature on growth were cumulative and not observed before three weeks, which may have led to an uninformed decision on which populations were suitable to transfer to the next ratchet temperature. Alternatively, we did not want to mistake an initial, positive plastic response for a stable adaptive change. Secondly, we transferred cells into the next selecting temperature if they exhibited any positive growth, whereas in Huertas et al. (2011) only if a population exhibited growth equal to, or greater than, the control population, was it transferred; our decision was made based on a previous experiment (Chakravarti et al., 2017), where a laboratory selected SS strain of Symbiodinium (type C1) that had been cultured for approximately 80 generations at 31°C was not able to match the growth rate of its WT counterpart at the control temperature, but exceeded the growth of the WT transferred short-term into elevated temperature. Thirdly, even if a population was transferred to the next temperature treatment, a copy was also always maintained at the previous temperature treatment(s). Previous temperatures may have been sub-optimal for a population, even if they were able to maintain some growth, thus more generations of culture could have allowed a (further) adaptive response to occur.
Testing for a Stable Adaptive Response
To test whether a stable adaptive response had occurred after ~1 year of thermal selection in the ratchet experiment, we carried out a transplantation experiment. This involved control or wild-type (WT) populations being both kept at 27°C (WT@27, 26.84°C ± 0.005, n = 4394) and also transferred to the elevated temperature treatments e.g., WT@30 (29.89°C ± 0.0004, n = 54347), WT@31 (31.02°C ± 0.021, n = 4322), WT@33 (32.93°C ± 0.016, n = 4409) and/or WT@34 (34.08°C ± 0.0009, n = 4322). Growth rates and photophysiological measurements of the selected Symbiodinium populations (SS) already at elevated temperature (SS@30, SS@31 etc.) were compared to those from WT cultures at control temperature moved to elevated temperatures.
Each WT population was transferred to fresh media at a cell density of 200,000 cells mL−1 and pre-acclimated for two weeks to each temperature treatment that their counterpart SS resided in. SS populations were also transferred to fresh media and cultured for two weeks at their appropriate temperature conditions. The pre-acclimated cells were then transferred into fresh media at the same starting concentration to give three replicate cultures (n = 3) for each population in each temperature treatment.
On days 3, 10, 17, and 26 after inoculation, 100 μL aliquots of homogenised culture from each WT and SS replicate was transferred into a black, clear-bottom 96-well culture plate (Costar, Corning; Sigma-Aldrich) for photophysiology and growth rate measurements.
Photophysiology
Culture aliquots were transferred to multi-well plates during the end of the dark period and were dark-adapted for a minimum of a further 30 min at their appropriate temperature treatments. The maximum quantum yield of PSII (Fv/Fm = (Fm-Fo)/Fm) was measured on dark-adapted samples using an imaging PAM (iPAM) with the measuring light set at 10 and gain and damping at two. The cultures were then subjected to 6 min of actinic light of two (PAR = 20 μmol photons m−2 s−1) before measuring the effective quantum yield of PSII (ΔF/Fm' = ((Fm' – F')/ Fm'). Cells were then fixed in the well-plates by adding 3.4 μL of 25% glutaraldehyde for later cell counts and calculation of growth rate.
Growth Rate and Number of Cell Generations
To measure growth rate, cell density was determined on day 17 post-acclimation in each replicate by triplicate haemocytometer counts. The specific growth rate (μ, doubling/day) was calculated as
where N0 is the cell density at the start (200,000 cells/mL) of the experiment and N1 is the cell density at day 17 and t is the duration of culture (17) in days. To estimate the number of generations each population had been through during long-term selection, the doubling time (or generation time) was calculated, according to the equation
Where d is the number of days of growth and N0 is the initial inoculation cell density (i.e., 100,000 cells mL−1 during the ratchet experiment and 200,000 cells mL−1 during the transplant experiment). Subsequently, the number of generations was calculated as
Where N is the number of days of culture and T is the doubling time.
Statistical Analyses
To test whether the selected Symbiodinium (SS) populations had mounted an adaptive response as a result of long-term thermal selection, we compared the growth and photosynthetic performance of the three SS populations (a-c) at each temperature treatment that they had reached (e.g., SS@30a/b/c, 31, 33, and/or 34) with the performance of the control population, maintained at 27°C long-term (e.g., WT@27) and the WT population transferred into the elevated temperature treatment (e.g., WT@30, WT@31, WT@33 and/or WT@34). This was done for each Symbiodinium type using linear models with “population” (i.e., WT@27, WT@30, SS@30a, SS@30b, SS@30c) as a fixed factor for both growth rate and photosynthetic efficiencies and with “time” as an additional fixed factor for the latter. All analyses were conducted in R v. 3.4.1 (R Core Team, 2016). Data Sheets 1 and 2 include all raw data.
Results
Long-Term Culture
One strain of Symbiodinium, D1 (SCF082), was not able to grow at the first ratchet temperature of 30°C, despite two, six-week attempts of culture and was therefore could not be included in the subsequent transplant experiment. Symbiodinium A3c, D1 (SCF086.01), F1 and G3 all exhibited positive growth at 30°C and were cultured for between 25 and 71 generations compared with 47–73 generations at 27°C (Table 2). Only two Symbiodinium types, F1 and G3, were able to grow at the next ratchet temperature of 31°C with all three SS populations of both types cultured at 31°C for up to 69 and 53 generations, respectively. At 33°C and 34°C only Symbiodinium F1 was able to grow, with the three SS populations having gone through 41–46 generations at this temperature by the end of the ratchet experiment. At the highest ratchet temperature, F1 was cultured for between 16 and 17 generations, before the transplant experiment was carried out.
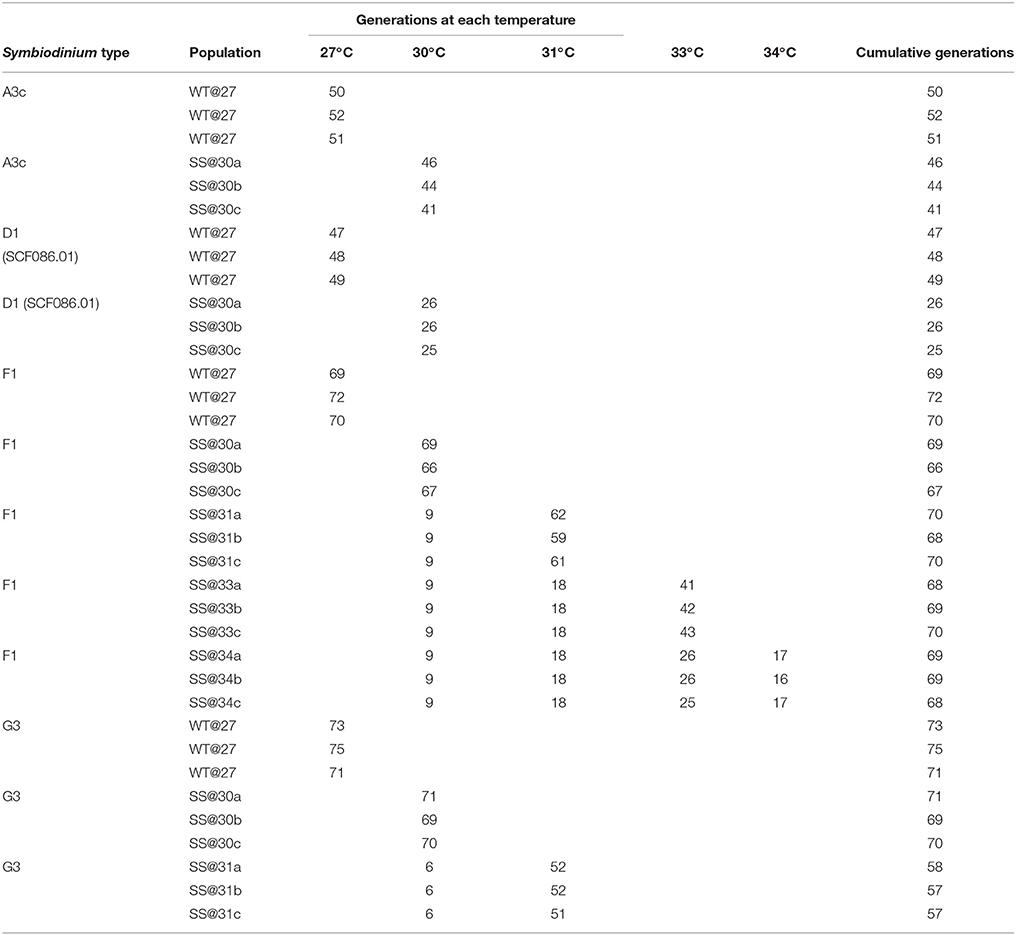
Table 2. Number of generations that each population spent at each temperature condition during the ~1-year ratchet experiment.
Testing for a Stable Adaptive Response (Transplantation Experiment)
Symbiodinium A3c
Two of the A3c SS populations (SS@30a and SS@30c) were able to grow significantly faster than the WT@30 population (max t2 = 5.28, P < 0.01, Table S1) by up to 49% (±8.80%) after long-term culture at 30°C, with the growth rate of population SS@30a being significantly faster than the WT@27 (t2 = 3.51, P = 0.04, Table S1), by 28% (±7.53% Figure 2A). Mean effective yields for the three SS@30 populations, were between 10.68% (±1.45%) and 21.75% (±2.61%) lower than the controls throughout the experiment, however they remained stable over the 26 days period (min t = −1.79, P = 0.71, Figure 2B). In contrast, the WT@30 population, showed a significant decline of 17.19% (±4.75%) in mean effective quantum yield by the end of the experiment with a final mean value that was significantly lower than that of the three SS@30 populations (max t = 5.56, P < 0.01, Table S1) by up to 23.92% (±4.75%, Figure 2B). The maximum quantum yield values followed a similar trend (Figure 2C), although the drop in WT@30 mean yield value by the end of the experiment was not as marked.
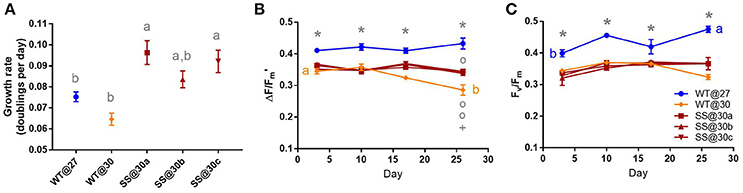
Figure 2. Symbiodinium A3c. Results of transplant experiment; comparison of mean (±SEM) growth rates (A), effective quantum yields (B) and maximum quantum yields (C) between the wild-type (WT) Symbiodinium type A3c population at 27°C (WT@27), WT transferred into 30°C (WT@30) and the three selected Symbiodinium A3c populations (SS@30a, b, c). Populations' were pre-acclimated for 14 days and growth rates were measured following 17 more days and quantum yield measurements over the following 26 days. Statistically significant differences among populations at a given time point are represented by different letters (A) or symbols above/below the mean points (B,C). Statistically significant differences over time (between D3 and D26) are represented by different letters (B,C, Tukey's post-hoc tests; P < 0.05), n = 3 for all means. Where error bars are not visible, they are small and hidden by the symbols.
Symbiodinium D1
Long-term culture at 30°C did not improve the mean growth of any D1 SS population. In fact, one population, SS@30b grew 43.8% (±15.2%) slower than the WT@30 (t2 = −3.84, P = 0.02, Figure 3A, Table S2). There was some variation in growth rates among the three SS populations, with SS@30b growing 43.1% (±15.4%) and significantly slower than SS@30c (t2 = 3.73, P = 0.03, Table S2). In general, the SS@30 populations displayed a trend of both lower effective quantum yield values and maximum quantum yield values compared to both the WT@27 and WT@30 populations during the transplant experiment (Figures 3B,C), despite long-term thermal exposure.
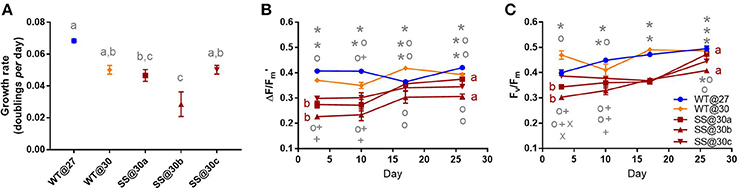
Figure 3. Symbiodinium D1. Results of transplant experiment; comparison of mean (±SEM) growth rates (A), effective quantum yields (B) and maximum quantum yields (C) between the wild-type (WT) Symbiodinium type D1 population at 27°C (WT@27), WT transferred into 30°C (WT@30) and the three selected Symbiodinium D1 populations (SS@30a, b, c). Populations' were pre-acclimated for 14 days and growth rates were measured following 17 more days and quantum yield measurements over the following 26 days. Statistically significant differences among populations at a given time point are represented by different letters (A) or symbols above/below the mean points (B,C). Statistically significant differences over time (between D3 and D26) are represented by different letters (B,C, Tukey's post-hoc tests; P < 0.05), n = 3 for all means. Where error bars are not visible, they are small and hidden by the symbols.
Symbiodinium f1
After long-term thermal selection, one population, SS@30b, grew 24.84% (±0.95%) slower compared to the WT@27 (t2 = −3.810, P = 0.02, Table S3) and 23.80% (±0.96%) slower compared to the WT@30 (t2 = −3.60, P = 0.03, Figure 4A, Table S3), while the remaining two SS@30 populations (a and c), maintained mean growth rates that were similar to the WT@27 and WT@30 (min t2 = −2.37, P = 0.20). By day 17 and 26, WT@27 and WT@30 effective quantum yield values were reduced by as much as 24.74% (±1.25%), compared to all three SS populations, which had statistically similar yields (min. t = −1.62, P = 0.98, Figure 4B). Similarly, maximum quantum yield values became reduced for the WT@27 and WT@30 populations at day 26; by up to 19.56% (±0.40%) compared with the SS populations (±0.14%, min t = −8.12, P < 0.01, Figure 4C, Table S3).
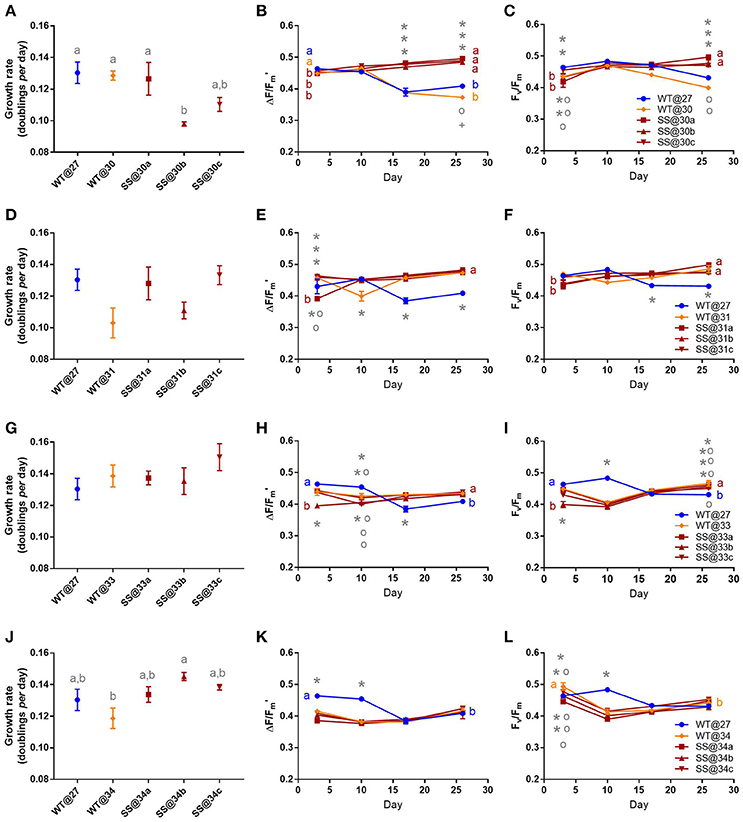
Figure 4. Symbiodinium F1. Results of transplant experiment; comparison of mean (±SEM) growth rates (A,D,G,J), effective quantum yields (B,E,H,K) and maximum quantum yields (C,F,I,L) between the wild-type (WT) Symbiodinium type F1 population at 27°C (WT@27), WT transferred into elevated temperature (WT@30, WT@31, WT@33, or WT@34) and the three selected Symbiodinium F1 populations (e.g., SS@34a, b, c). Populations' were pre-acclimated for 14 days and growth rates were measured following 17 more days and quantum yield measurements over the following 26 days. Statistically significant differences among populations at a given time point are represented by different letters (A) or symbols above/below the mean points (B,C). Statistically significant differences over time (between D3 and D26) are represented by different letters (B,C, Tukey's post-hoc tests; P < 0.05), n = 3 for all means. Where error bars are not visible, they are small and hidden by the symbols.
Long-term culture at 31°C did not significantly affect the growth rates of the F1 SS populations nor did the short-term transfer of WT cells into 31°C (WT@31, max t2 = 2.73, P = 0.12, Figure 4D). During the latter half of the experiment, the three SS@31 populations and the WT@31 population displayed mean effective quantum yield values that were up to 17.26% (±1.93%) greater than the mean values for the WT@27 control (max t = 5.90, P < 0.01, Figure 4E, Table S4). Similar trends were apparent for the mean maximum quantum yield values (Figure 4F), with the WT@27 having significantly lower values than the remaining treatments by up to 13.50% (±0.94%) at day 26 (max t = 5.98, P < 0.01, Table S4).
Similarly to the 31°C experiment, no significant differences in growth rate existed between any F1 population in the 33°C transplant experiment (max t2 = 2.00, P = 0.33, Figure 4G). Furthermore, differences in both mean effective quantum yields and maximum quantum yields by the end of the experiment, were minimal (Figures 4H,I, Table S5).
After long-term selection for F1 populations at 34°C there were no significant differences in mean growth rates between the three populations (max t = −1.65, P = 0.50), however, the SS@34b population exhibited a mean growth rate that was 22% (±2.10%) faster than the WT@34 population (t2 = 3.81, P = 0.02, Figure 4J, Table S6). Effective quantum yield values between all F1@34 populations, by the end of the experiment, were similar (max t = 0.20, P = 1.00, Figure 4K). Further, maximum quantum yield values were not significantly different to each other between the populations by the end of the experiment (max t = −2.14, P = 0.82 Figure 4L).
Symbiodinium G3
There were no significant differences in growth rate between any of the G3 populations in the 30°C transplant experiment (max t2 = 1.71, P = 0.47, Figure 5A). There were also no notable differences in effective quantum yield values by the end of the experiment (max t2 = 0.03, P = 1.00, Figure 5B, Table S7) or maximum quantum yield values (max t2 = 1.445, P = 0.10, Figure 5C, Table S7).
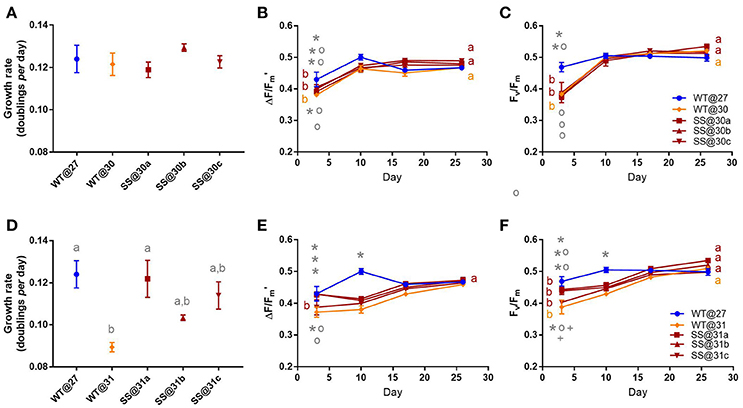
Figure 5. Symbiodinium G3. Results of transplant experiment; comparison of mean (±SEM) growth rates (A,D), effective quantum yields (B,E) and maximum quantum yields (C,F) between the wild-type (WT) Symbiodinium type G3 population at 27°C (WT@27), WT transferred into 30°C or 31°C (WT@30 or WT@31) and the three selected Symbiodinium G3 populations (e.g., SS@30a, b, c). Populations' were pre-acclimated for 14 days and growth rates were measured following 17 more days and quantum yield measurements over the following 26 days. Statistically significant differences among populations at a given time point are represented by different letters (A) or symbols above/below the mean points (B,C). Statistically significant differences over time (between D3 and D26) are represented by different letters (B,C, Tukey's post-hoc tests; P < 0.05), n = 3 for all means. Where error bars are not visible, they are small and hidden by the symbols.
Wild-type (WT) G3 cells transferred into 31°C grew 27.9% (±1.78%) slower, compared to the WT@27 controls (t2 = −4.23, P = 0.01, Table S8). For two of the SS populations (b and c) growth rates were intermediate to that of WT@31 and WT@27, and not significantly different (min t2 = −2.26, P = 0.24). However, for the remaining SS@31a population, the mean growth rate was similar to the WT@27 cells (t2 = −2.260, P = 0.999) and significantly greater, by 36% (±9.82%), than the WT@31 cells (t2 = 3.97, P = 0.02, Figure 5D, Table S8). The mean effective quantum yield for the WT@31 population was significantly lower by up to 13.47% (±3.74%) compared to the WT@27, SS@31a and SS@31b populations at three days post-acclimation (max t = −3.92, P = 0.04, Table S8). By the end of the experiment, however, mean effective quantum yields were similar among all populations (min t = −0.57, P = 1.00, Figure 5E). Again, at three days post-acclimation the WT@31 population had the lowest maximum quantum yield values compared to the WT@27, SS@31a and SS@31b populations (max t = −4.06, P = 0.02, Figure 5F, Table S2), by up to 17.27% (±4.62%), but by the end of the mean values were similar among all populations (min. t −0.57 = , P = 1.00, Figure 5F).
Discussion
The thermal tolerance response to long-term exposure to increasing temperatures of five Symbiodinium wild-types (WT) representing four distinct phylogenetic clades exhibited considerable variation. After one year of culture at step-wise increases in temperature, three of the five Symbiodinium types showed signs of a stable adaptive change; two thermally-derived populations of type A3c at 30°C, one of type G3 at 31°C and one of type F1 at 34°C after only 41–69 generations at elevated temperature showed increased performance at elevated temperatures compared to the respective WT cultures.
Symbiodinium A3c
The acute heat stress (i.e., transplant) experiment of the WT A3c cells into the first elevated temperature treatment of 30°C resulted in a 17% decrease in growth rate and declines in photosynthetic efficiencies, suggesting a relatively low natural thermal tolerance for this strain. This is perhaps surprising as the literature describes most Symbiodinium clade A strains as thermally tolerant (Robison and Warner, 2006; Reynolds et al., 2008; Kemp et al., 2014) and typically prevalent in shallow-water cnidarians, where high light and temperature co-occur (LaJeunesse, 2002). This has generally been attributed to enhanced and alternative photosynthetic electron pathways such as cyclic electron transport and light harvesting complex dissociation mechanisms as well as photoprotection processes (Reynolds et al., 2008). In contrast, two of the SS A3c populations in our study that were cultured for up to 46 generations at 30°C, were able to grow 43–49% faster than the WT@30 and up to 28% faster than the WT at the control temperature. Furthermore, throughout the transplant experiment, the photosynthetic efficiencies of the WT@30 significantly decreased over time, while the SS populations were able to maintain high photosynthetic efficiencies. These results suggest a stable, adaptive change having occurred after only up to 46 generations at 30°C.
Current evidence on the value of members of clade A to the coral host is varied. Clade A Symbiodinium have been associated with the fast colonisation of health-compromised corals (Toller et al., 2001), low transfer of photosynthate compared to C1 (Stat et al., 2008) and high competitive ability in mixed in vitro cultures (Rowan, 1998). Furthermore, there have been reports of wide phenotypic and physiological diversity of clade A (Robison and Warner, 2006; Takahashi et al., 2009; Ragni et al., 2010; Krämer et al., 2012). These factors have led to the suggestion that members of clade A are more parasitic in nature and suboptimal as symbionts (Stat et al., 2008; Mieog et al., 2009). However, other studies have suggested their ecological value; type A3c was associated with juvenile GBR Acropora tenuis that had lower mortality than when harbouring other types of Symbiodinium (Quigley et al., 2016) and early larval uptake of clade A in Acropora yongei was associated with a higher post-settlement survival rate (Suzuki et al., 2013). These studies suggest that some members of clade A could be valuable in experimental evolution studies aimed at enhancing the tolerances of the early life stages of coral. Thus, the stable enhanced thermal tolerance we observed here is promising, particularly because we observed this in two of three SS populations.
Symbiodinium D1
We experimented with two clade D strains of Symbiodinium that are genetically distinct based on ITS2 sequences. Surprisingly, neither were particularly thermally tolerant, considering members of clade D are widely described as being heat resistant in the literature. The D1 strains, SCF082 and SCF086.01, were not able to grow at, or above 30°C, respectively; for Symbiodinium type D1 (SCF086.01), SS populations managed to grow for only 25–26 generations at 30°C over a one-yr period, compared to the 47–49 generations of the WT@27. Furthermore, long-term culture at 30°C had a negative effect on the photosynthetic efficiencies of the SS populations and a 44% decrease in growth of one population. In contrast, Symbiodinium clade D has often been found to dominate corals on reefs that are exposed to heat stress or have had a history of bleaching (Glynn et al., 2001; Toller et al., 2001; Baker et al., 2004; Rowan, 2004; van Oppen et al., 2005a; Jones et al., 2008; LaJeunesse et al., 2009; Pettay et al., 2015) and has been shown to enhance the thermal tolerance of the coral Acropora millepora, a common Indo-Pacific species, by 1.0–1.5°C through the shuffling of symbiont types (Berkelmans and van Oppen, 2006). Other studies have shown high abundances of Symbiodinium clade D in corals at 32°C in Thailand (LaJeunesse et al., 2010), 31.8°C in Palau (Fabricius et al., 2004) and >33°C in the Persian Gulf (Baker et al., 2004; Mostafavi et al., 2007). This suggests that there is either wide variation in thermal tolerance between clade D types and/or thermal tolerances are different in free-living Symbiodinium cultures compared to in hospite populations.
Symbiodinium F1
Symbiodinium type F1 was the most thermally tolerant strain in this study. In the transplant experiments, growth rates of the WT transferred to 30, 31, 33, and 34°C did not significantly change, while photosynthetic efficiencies remained similar. This agrees with previous in vitro studies on other members in this clade; between 25°C and 33°C, a different strain of Symbiodinium F1 did not show any differences in growth or photosynthetic efficiencies over two weeks, while expressing significantly higher levels of antioxidant defense enzymes at 33°C aimed to scavenge reactive oxygen species (ROS) produced as a by-product of photosynthesis and cellular respiration (Krueger et al., 2014). The ability to mitigate damage caused by excess ROS was also shown by Symbiodinium F2 after 12 days at 29–31°C (McGinty et al., 2012), while the same F2 isolate showed the greatest capacity for both photo-acclimation and growth at 32°C compared with isolates belonging to clades A and B (Robison and Warner, 2006).
Long-term culture in this study did not significantly affect the ability of F1 to grow or its photosynthetic efficiency under elevated temperature, apart from two cases. Firstly, 66–67 generations at 30°C resulted in a significant drop in growth rate for two SS populations (SS@30b and c), with SS@30b being statistically lower than the WT@30 transplant by 23.80% and WT@27 control by 24.84%. Despite this, the photosynthetic efficiencies of all three SS populations were greater than the WT@27 and WT@30 across much of the transplant experiment and remained stable. Secondly, long-term culture at 34°C enhanced the growth rate of one SS population (SS@34b) such that it grew 18% faster than the WT@34.
Symbiodinium type F1 is rarely described in the literature, in symbiosis or as free-living populations. Clade F made up only 0.9% of Symbiodinium sequences recovered from 10 coral species, sediments and water samples across contrasting thermal environments in American Samoa (Cunning et al., 2015). In the Indian Ocean, 47 ITS2 Symbiodinium types were characterised from over 600 samples that ranged over 50 host genera and only one was found to harbour a member of clade F, an anemone species (LaJeunesse et al., 2010). Only one of 16 coral species, Goniastrea sp., around the Arabian Peninsula harboured clade F (F4.3) and at >5% abundance (Ziegler et al., 2017). In the GBR, eastern Pacific and Caribbean 114 coral species revealed no Symbiodinium clade F (van Oppen et al., 2005b). Such a lack of biogeographic prevalence and abundance is surprising given this clade's ability to cope with heat stress. This calls for the need to study clade F's efficacy as a symbiont.
Symbiodinium G3
After 57 generations at 31°C, a population of Symbiodinium type G3 was able to grow 36% faster than the WT@31, with small but apparent improvements in photosynthetic efficiencies. These results suggest a stable adaptive change had occurred after only one year at elevated temperature for at least one of the SS G3 populations.
Clade G has been found to associate with a wide variety of hosts and environments including sponges (Schönberg and Loh, 2005; Granados et al., 2008; Schönberg et al., 2008; Hill et al., 2011), soft corals (van Oppen et al., 2005a) and foraminiferans (Pochon et al., 2001), and is also known to occur in the water column and in benthic sediments (Takabayashi et al., 2012). Occasionally, members of clade G have been found to associate with scleractinian corals including Porites lobata in Hawaii (Stat et al., 2013) and low frequencies (>0.09% of all sequences) have been observed in Orbicella annularis in US Virgin Islands (Edmunds et al., 2014; Pochon et al., 2014) and Acropora in Western Australia (Thomas et al., 2014).
Although members in this clade do not seem to be common symbionts of reef-building corals, our study strain, extracted from a scleractinian coral species Diploastrea heliopora in the GBR, adds to the growing body of evidence of clade G being able to form a symbiosis with scleractinian corals. Its ability to exhibit a stable adaptive change after one year of culture at 31°C, is promising for evolutionary experiments aimed to develop heat resistant Symbiodinium.
Experimental Evolution in Microalgae and Mechanisms Underlying Adaptive Changes
To our knowledge, there have been five experimental evolution studies involving other species of marine microalgae that have subjected cells to long-term elevated temperature (Table 3). Growth rate is used as the universal indicator of fitness, allowing for comparisons between experiments. These studies indicate that after 20–460 generations (from 45 days to two years) there have been stable increases in growth of between 16 and 60% for elevated-temperature-derived strains compared to ancestral populations placed at acute elevated temperature; results comparable with our findings of increases in growth rate of 22–49%. Evolutionary experiments involving Symbiodinium have been carried out on only three different strains, which are reported in two studies (Table 3). After only 55 generations (Huertas et al., 2011) to 80 generations (Chakravarti et al., 2017) Symbiodinium were able to grow at elevated temperatures of 30°C and 31°C, respectively, where previously there had been no growth at these temperatures.
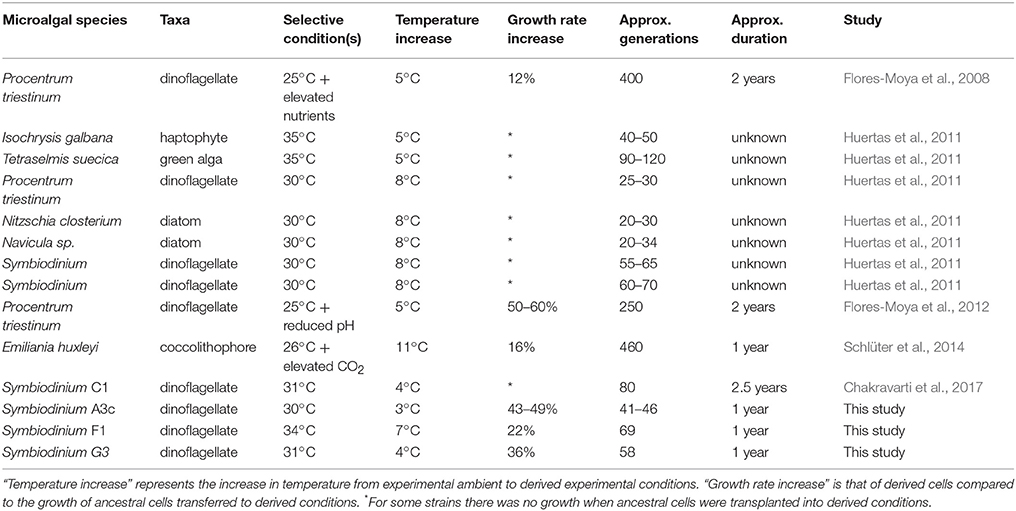
Table 3. Experimental evolution studies on marine microalgae that show an adaptive response to elevated temperature.
The current study adds three more strains of Symbiodinium that provide evidence of a stable adaptive change to increased temperature. These stable, physiological improvements could have resulted from either genetic adaptation through selection on random, beneficial mutations and/or acclimatisation processes that can be heritable across generations, via epigenetic modifications of gene expression via, for example, DNA methylation, small RNAs and chromatin modifications (Bird, 2002; Greer and Shi, 2012; Castel and Martienssen, 2013). Under experimental heat stress a warmer water population of Symbiodinium C1 significantly up-regulated ROS scavenging and molecular chaperone gene expression, vital for repairing damaged proteins, compared to a cooler water population of Symbiodinium C1, despite both being identical in ITS1 and ITS2 sequences and cultured under “ambient” conditions for four years prior to experiments (Levin et al., 2016). Gene expression responses such as these can result from genetic adaptation or epigenetic processes where historical stress induced modifications that are inherited across generations (Bonduriansky et al., 2012); either (or both) could be mechanisms for observed enhanced thermal tolerances in our study. It is possible that the historical, laboratory conditions, particularly the comparatively low light conditions that the strains were cultured under could have had some influence on their physiological responses to elevated temperature.
It is important to note that most long-term evolutionary studies on microalgae have inoculated experimental replicates with a single cell to ensure each replicate started with only one genotype (e.g., Huertas et al., 2011; Lohbeck et al., 2012). In our study, cultures A3c and F1 originated from a single cell, however G3 and clades D did not. It is therefore possible that either selection on standing genetic variation and/or selection on random, beneficial mutations could have occurred in our ratchet experiment. Additionally, it is possible that for those originally monoclonal isolates, genetic diversity arising from random somatic mutations before carrying out our study may have been enough genetic variation for selection to act upon when our experiment commenced. Furthermore, our study and other evolutionary experiments on microalgae assume mitotic, asexual cell divisions produce genetic adaptations or induce heritable acclimatisation responses across generations. However, the discovery of meiosis-specific genes in the Symbiodinium genome indicates that sexual reproduction is possible in Symbiodinium (Chi et al., 2014; Rosic et al., 2015; Levin et al., 2016). This has led to the hypothesis that a switch from asexual to sexual reproduction (i.e., inducing recombination) may be a response mechanism to environmental stress in Symbiodinium (Chi et al., 2014; Wilkinson et al., 2015), although it has not yet been observed.
Considerations for Symbiodinium Experimental Evolution as a Tool for Coral Assisted Evolution
We show that Symbiodinium types F1 and G3 are the most naturally thermally tolerant strains in this study, able to survive and grow at 34 and 31°C, respectively, even without long-term thermal selection. Despite their heat resistance, members of clades F and G are some of the least prevalent in corals, with clades A, B, C, and D most commonly described as being associated with scleractinians (van Oppen et al., 2009). Symbiodinium types in clade D, the least thermally tolerant strains in this study, with one strain unable to grow at the lowest selecting temperature and the other exhibiting a supressed photosynthetic response after thermal selection, are the most prevalent in corals after bleaching events. Our findings highlight the complex relationship between symbiont identity, symbiont thermal tolerance ex hospite and holobiont bleaching tolerance (Bhagooli and Hidaka, 2003; Goulet et al., 2005; Chakravarti et al., 2017; Gabay et al., 2018). Here, the continuum of parasitism to mutualism of photosymbionts (Baker et al., 2018) is an important consideration in Symbiodinium thermal selection experiments whereby the relative contribution of a symbiont to the host should be considered, for example, the amount of photosynthate produced and released, in addition to a strains ability to be taken up by the host and retained under heat stress.
It is important to consider the traits that are assessed during Symbiodinium evolutionary experiments. Here we use growth rate as well as photosynthetic efficiencies as key traits. However, additional, or alternative traits may be required to better predict the fitness of corals inoculated with laboratory-evolved Symbiodinium strains. For example, fast growth rate, although an important indicator of fitness, may not be advantageous to a symbiont, or its host, in hospite. Additionally, in terms of photophysiology, a recent study showed that corals containing type D1a were able to retain a symbiosis at 33°C despite significant losses in photosynthetic function, while those harbouring type C3 also experienced photodamage but in contrast, they lost 99% of their symbionts (Silverstein et al., 2017).
It is also important that for temperature-selected Symbiodinium in evolutionary experiments, we test their contribution to the host under heat stress, as well as their symbiotic performance at ambient temperatures—not just their ability to infect and be retained. For instance, following a bleaching event on the GBR in 2006, bleached Acropora millepora colonies harbouring clade C were able to recover lipid levels to those of unbleached C colonies, however clade D colonies that had remained unbleached had 26% lower stored lipids compared to C colonies with 28% smaller eggs and did not recover pre-bleaching lipid levels for nine months (Jones and Berkelmans, 2011). In such cases, perhaps factors such as the extracellular production of ROS by Symbiodinium or lack of photosynthate release contributed the fitness of the holobiont. Studies investigating Symbiodinium carbon fixation, release and incorporation into host tissue though radioactive 14C labelling and stable isotopic 13C and 15N labelling (Biel et al., 2007; Stat et al., 2008; Cantin et al., 2009; Baker et al., 2013, 2018; Pernice et al., 2015; Matthews et al., 2017) together with newer technologies such as nanoscale secondary ion mass spectrometry (NanoSIMS) (e.g., Wangpraseurt et al., 2016) will be crucial for understanding the relative function of the symbiont within the host. This, along with measuring other physiological parameters relating to coral fitness response when harbouring laboratory-evolved Symbiodinium as well as gene expression studies (e.g., DeSalvo et al., 2010; Pinzon et al., 2015; Traylor-Knowles et al., 2017), will thus be informative.
Finally, inoculation of aposymbiotic corals with a mix of experimentally evolved Symbiodinium types remains an important consideration. For example, it is possible that the host environment and/or nutritional needs of corals change throughout their life history such that different Symbiodinium confer different advantages depending on its hosts' life stage (Abrego et al., 2008; Jones et al., 2008; Quigley et al., 2016). Furthermore, absolute specificity is rare in corals (e.g., Silverstein et al., 2012; Quigley et al., 2014) and thus infecting corals with a cocktail of thermally selected strains could allow the host to retain those most beneficial under thermal stress through symbiont community shuffling (see Chen et al., 2005; Berkelmans and van Oppen, 2006; Thornhill et al., 2006; Mieog et al., 2007), aiding in a flexible response to environmental change. Additionally, it is important that thermal tolerance of ex hospite Symbiodinium is not overlooked. Eighty five per cent of scleractinian coral species are broadcast spawners, of which 80% acquire symbionts horizontally, from free-living populations (Baird et al., 2009). It is therefore critical that the free-living Symbiodinium pool remains thermally tolerant, as an exogenous source for coral larvae and recruits as well as bleached colonies (Boulotte et al., 2016). Our results show that rapid adaptation is indeed possible in free-living Symbiodinium populations undergoing directed selection and we show that this is possible across multiple genetically distinct strains of Symbiodinium.
Conclusions And Next-Steps
After only one year of thermal selection and as few as 41 generations, we were already able to observe stable adaptive responses to elevated temperature in three of the five strains of Symbiodinium included in this study. The observed increases in growth rates are comparable with evolutionary experiments in other microalgae, where selected populations have been exposed to elevated temperatures for up to 460 generations. A further year of thermal selection and we would expect to see the divergence in thermal tolerance of selected populations compared with ancestral ones to expand.
Important next-steps are to thoroughly investigate the infectivity of these strains under different temperature conditions, their stability in hospite, their effect on holobiont bleaching tolerance and health across different coral life stages, and to understand if and why thermal tolerance differs ex- and in hospite. Such experiments will provide insight into which clades and types of Symbiodinium will be better sources for evolutionary experiments and which traits should be targeted in directed selection experiments that aim to develop symbionts with the ability to increase coral bleaching tolerance.
Author Contributions
LC and MvO contributed to conception and design of the study; LC performed the experiments and analyses. LC wrote the first draft of the manuscript with input from MvO.
Conflict of Interest Statement
The authors declare that the research was conducted in the absence of any commercial or financial relationships that could be construed as a potential conflict of interest.
The reviewer CO and handling Editor declared their shared affiliation.
Acknowledgments
We thank Victor Beltran for extracting and culturing three of the strains in this study, David Suggett for providing the remaining two strains used in this study and Carlos Alvarez-Roa for ITS2 sequencing the strains used in this study. We also thank the Reviewers and Editor for their comments on the manuscript. The study was funded by Paul G. Allen Family Foundation and the Australian Institute of Marine Science. LC acknowledges receipt of a James Cook University Post-Graduate Research Scholarship (JCUPRS). Study organisms were collected under GBRMPA permit no G12/35236.1.
Supplementary Material
The Supplementary Material for this article can be found online at: https://www.frontiersin.org/articles/10.3389/fmars.2018.00227/full#supplementary-material
References
Abrego, D., Ulstrup, K. E., Willis, B. L., and van Oppen, M. J. (2008). Species-specific interactions between algal endosymbionts and coral hosts define their bleaching response to heat and light stress. Proc. Biol. Sci. 275, 2273–2282. doi: 10.1098/rspb.2008.0180
Abrego, D., Van Oppen, M. J., and Willis, B. L. (2009). Onset of algal endosymbiont specificity varies among closely related species of Acropora corals during early ontogeny. Mol. Ecol. 18, 3532–3543. doi: 10.1111/j.1365-294X.2009.04276.x
Babcock, R. C. (1991). Comparative demography of three species of scleractinian corals using age- and size-dependent classifications. Ecol. Monogr. 61, 225–244. doi: 10.2307/2937107
Baird, A. H., Guest, J. R., and Willis, B. L. (2009). Systematic and biogeographical patterns in the reproductive biology of scleractinian corals. Annu. Rev. Ecol. Evol. Syst. 40, 551–571. doi: 10.1146/annurev.ecolsys.110308.120220
Baird, A. H., and Marshall, P. A. (2002). Mortality, growth and reproduction in scleractinian corals following bleaching on the Great Barrier Reef. Mar. Ecol. Prog. Ser. 237, 133–141. doi: 10.3354/meps237133
Baker, A. C., Starger, C. J., McClanahan, T. R., and Glynn, P. W. (2004). Coral reefs: corals' adaptive response to climate change. Nature 430, 741–741. doi: 10.1038/430741a
Baker, D. M., Andras, J. P., Jordán-Garza, A. G., and Fogel, M. L. (2013). Nitrate competition in a coral symbiosis varies with temperature among Symbiodinium clades. ISME 7, 1248–1251. doi: 10.1038/ismej.2013.12
Baker, D. M., Freeman, C. J., Wong, J. C. Y., Fogel, M. L., and Knowlton, N. (2018). Climate change promotes parasitism in a coral symbiosis. ISME J. 12, 921–930. doi: 10.1038/s41396-018-0046-8
Beltran, V. H., Dunlap, W. C., and Long, P. F. (2012). “Comparison of the photosynthetic bleaching response of four coral species common to the central GBR,” in Proceedings of the 12th International Coral Reef Symposium (Townsville, QLD: James Cook University), 1–6.
Berkelmans, R., and van Oppen, M. J. (2006). The role of zooxanthellae in the thermal tolerance of corals: a “nugget of hope” for coral reefs in an era of climate change. Proc. Biol. Sci. 273, 2305–2312. doi: 10.1098/rspb.2006.3567
Bhagooli, R., and Hidaka, M. (2003). Comparison of stress susceptibility of in hospite and isolated zooxanthellae among five coral species. J. Exp. Mar. Bio. Ecol. 291, 181–197. doi: 10.1016/S0022-0981(03)00121-7
Biel, K. Y., Gates, R. D., and Muscatine, L. (2007). Effects of free amino acids on the photosynthetic carbon metabolism of symbiotic dinoflagellates. Russ. J. Plant Physiol. 54, 171–183. doi: 10.1134/S1021443707020033
Bird, A. (2002). DNA methylation patterns and epigenetic memory. Genes Dev. 16, 6–21. doi: 10.1101/gad.947102
Bonduriansky, R., Crean, A. J., and Day, T. (2012). The implications of nongenetic inheritance for evolution in changing environments. Evol. Appl. 5, 192–201. doi: 10.1111/j.1752-4571.2011.00213.x
Boulotte, N. M., Dalton, S. J., Carroll, A. G., Harrison, P. L., Putnam, H. M., Peplow, L. M., et al. (2016). Exploring the Symbiodinium rare biosphere provides evidence for symbiont switching in reef-building corals. ISME J. 10, 2693–2701. doi: 10.1038/ismej.2016.54
Bromham, L. (2009). Why do species vary in their rate of molecular evolution? Biol. Lett. 5, 401–404. doi: 10.1098/rsbl.2009.0136
Cantin, N. E., van Oppen, M. J. H., Willis, B. L., Mieog, J. C., and Negri, A. P. (2009). Juvenile corals can acquire more carbon from high-performance algal symbionts. Coral Reefs 28, 405–414. doi: 10.1007/s00338-009-0478-8
Castel, S. E., and Martienssen, R. A. (2013). RNA interference in the nucleus: roles for small RNAs in transcription, epigenetics and beyond. Nat. Rev. Genet. 14, 100–112. doi: 10.1038/nrg3355
Chakravarti, L. J., Beltran, V. H., and van Oppen, M. J. H. (2017). Rapid thermal adaptation in photosymbionts of reef-building corals. Glob. Chang. Biol. 23, 4675–4688. doi: 10.1111/gcb.13702
Chen, C. A., Wang, J.-T., Fang, L.-S., and Yang, Y.-W. (2005). Fluctuating algal symbiont communities in Acropora palifera (Scleractinia: Acroporidae) from Taiwan. Mar. Ecol. Prog. Ser. 295, 113–121. doi: 10.3354/meps295113
Cheung, W. W. L., Lam, V. W. Y., Sarmiento, J. L., Kearney, K., Watson, R., and Pauly, D. (2009). Projecting global marine biodiversity impacts under climate change scenarios. Fish Fish. 10, 235–251. doi: 10.1111/j.1467-2979.2008.00315.x
Chi, J., Parrow, M. W., and Dunthorn, M. (2014). Cryptic sex in Symbiodinium (Alveolata, Dinoflagellata) is supported by an inventory of meiotic genes. J. Eukaryot. Microbiol. 61, 322–327. doi: 10.1111/jeu.12110
Cook, C. B., D'Elia, C. F., and Muller-Parker, G. (1988). Host feeding and nutrient sufficiency for zooxanthellae in the sea anemone Aiptasia pallida. Mar. Biol. 98, 253–262.
Cunning, R., Yost, D. M., Guarinello, M. L., Putnam, H. M., and Gates, R. D. (2015). Variability of Symbiodinium communities in waters, sediments, and corals of thermally distinct reef pools in American Samoa. PLoS ONE 10:e0145099. doi: 10.1371/journal.pone.0145099
Dawson, T. P., Jackson, S. T., House, J. I., Prentice, I. C., and Mace, G. M. (2011). Beyond predictions: biodiversity conservation in a changing climate. Science 332, 53–58. doi: 10.1126/science.1200303
DeSalvo, M. K., Sunagawa, S., Voolstra, C. R., and Medina, M. (2010). Transcriptomic responses to heat stress and bleaching in the elkhorn coral Acropora palmata. Mar. Ecol. Prog. Ser. 402, 97–113. doi: 10.3354/meps08372
Edmunds, P. J., Pochon, X., Levitan, D. R., Yost, D. M., Belcaid, M., Putnam, H. M., et al. (2014). Long-term changes in Symbiodinium communities in Orbicella annularis in St. John, US Virgin Islands. Mar. Ecol. Prog. Ser. 506, 129–144. doi: 10.3354/meps10808
Fabricius, K. E., Mieog, J. C., Colin, P. L., Idip, D., and van Oppen, M. J. (2004). Identity and diversity of coral endosymbionts (zooxanthellae) from three Palauan reefs with contrasting bleaching, temperature and shading histories. Mol. Ecol. 13, 2445–2458. doi: 10.1111/j.1365-294X.2004.02230.x
Falkowski, P. G., Dubinsky, Z., Muscatine, L., and Porter, J. W. (1984). Light and the bioenergetics of a symbiotic coral. Bioscience 34, 705–709. doi: 10.2307/1309663
Fitt, W. K., and Trench, R. K. (1983). The relation of diel patters of cell division to diel patterns of motlity in the symbiotic dinoflagellate Symbiodinium microadriaticum freudenthal in culture. New Phytol. 94, 421–432. doi: 10.1111/j.1469-8137.1983.tb03456.x
Flores-Moya, A., Costas, E., and López-Rodas, V. (2008). Roles of adaptation, chance and history in the evolution of the dinoflagellate Prorocentrum triestinum. Naturwissenschaften 95, 697–703. doi: 10.1007/s00114-008-0372-1
Flores-Moya, A., Rouco, M., García-Sánchez, M. J., García-Balboa, C., González, R., Costas, E., et al. (2012). Effects of adaptation, chance, and history on the evolution of the toxic dinoflagellate Alexandrium minutum under selection of increased temperature and acidification. Ecol. Evol. 2, 1251–1259. doi: 10.1002/ece3.198
Frieler, K., Meinshausen, M., Golly, A., Mengel, M., Lebek, K., Donner, S. D., et al. (2013). Limiting global warming to 2 °C is unlikely to save most coral reefs. Nat. Clim. Chang. 3, 165–170. doi: 10.1038/nclimate1674
Gabay, Y., Weis, V. M., and Davy, S. K. (2018). symbiont identity influences patterns of symbiosis establishment, host growth, and asexual reproduction in a model cnidarian-dinoflagellate symbiosis. Biol. Bull. 234, 1–10. doi: 10.1086/696365
Glynn, P. W., Maté, J. L., Baker, A. C., and Calderón, M. O. (2001). Coral bleaching and mortality in panama and ecuador during the 1997–1998 El Niño–Southern Oscillation Event: spatial/temporal patterns and comparisons with the 1982–1983 event. Bull. Mar. Sci. 69, 79–109.
Goulet, T. L., Cook, C. B., and Goulet, D. (2005). Effect of short-term exposure to elevated temperatures and light levels on photosynthesis of different host-symbiont combinations in the Aiptasia pallidal Symbiodinium symbiosis. Limnol. Oceanogr. 50, 1490–1498. doi: 10.4319/lo.2005.50.5.1490
Granados, C., Camargo, C., Zea, S., and Sánchez, J. A. (2008). Phylogenetic relationships among zooxanthellae (Symbiodinium) associated to excavating sponges (Cliona spp.) reveal an unexpected lineage in the Caribbean. Mol. Phylogenet. Evol. 49, 554–560. doi: 10.1016/j.ympev.2008.07.023
Greer, E. L., and Shi, Y. (2012). Histone methylation: a dynamic mark in health, disease and inheritance. Nat. Rev. Genet. 13, 343–357. doi: 10.1038/nrg3173
Harvell, C. D., Kim, K., Burkholder, J. M., Colwell, R. R., Epstein, P. R., Dme, A., et al. (1999). Emerging marine diseases-climate links and anthropogenic factors. Science 285, 1505–1510.
Hill, M., Allenby, A., Ramsby, B., Schönberg, C., and Hill, A. (2011). Symbiodinium diversity among host clionaid sponges from Caribbean and Pacific reefs: Evidence of heteroplasmy and putative host-specific symbiont lineages. Mol. Phylogenet. Evol. 59, 81–88. doi: 10.1016/j.ympev.2011.01.006
Hoegh-Guldberg, O., and Bruno, J. F. (2010). The impact of climate change on the world's marine ecosystems. Science 328, 1523–1528. doi: 10.1126/science.1189930
Hoegh-Guldberg, O., McCloskey, L. R., and Muscatine, L. (1987). Expulsion of zooxanthellae by symbiotic cnidarians from the Red Sea. Coral Reefs 5, 201–204. doi: 10.1007/BF00300964
Howells, E. J., Beltran, V. H., Larsen, N. W., Bay, L. K., Willis, B. L., and van Oppen, M. J. H. (2012). Coral thermal tolerance shaped by local adaptation of photosymbionts. Nat. Clim. Chang. 2, 116–120. doi: 10.1038/nclimate1330
Howells, E. J., Ketchum, R. N., Bauman, A. G., Mustafa, Y., and Watkins, K. D. (2016). Species-specific trends in the reproductive output of corals across environmental gradients and bleaching histories. Mar. Pollut. Bull. 105, 532–539. doi: 10.1016/j.marpolbul.2015.11.034
Huertas, I. E., Rouco, M., Lopez-Rodas, V., and Costas, E. (2011). Warming will affect phytoplankton differently: evidence through a mechanistic approach. Proc. R. Soc. B Biol. Sci. 278, 3534–3543. doi: 10.1098/rspb.2011.0160
Hughes, T. P., Kerry, J. T., Álvarez-Noriega, M., Álvarez-romero, J. G., Anderson, K. D., Baird, A. H., et al. (2017). Global warming and recurrent mass bleaching of corals. Nature 543, 373–377. doi: 10.1038/nature21707
Jones, A. M., and Berkelmans, R. (2011). Tradeoffs to thermal acclimation: energetics and reproduction of a reef coral with heat tolerant symbiodinium Type-D. J. Mar. Biol. 2011:185890. doi: 10.1155/2011/185890
Jones, A. M., Berkelmans, R., van Oppen, M. J., Mieog, J. C., and Sinclair, W. (2008). A community change in the algal endosymbionts of a scleractinian coral following a natural bleaching event: field evidence of acclimatization. Proceedings. Biol. Sci. 275, 1359–1365. doi: 10.1098/rspb.2008.0069
Kemp, D. W., Hernandez-Pech, X., Iglesias-Prieto, R., Fitt, W. K., and Schmidt, G. W. (2014). Community dynamics and physiology of Symbiodinium spp. before, during, and after a coral bleaching event. Limnol. Oceanogr. 59, 788–797. doi: 10.4319/lo.2014.59.3.0788
Kinzie, R. A., Takayama, M., Santos, S. R., and Coffroth, M. A. (2001). The adaptive bleaching hypothesis: experimental tests of critical assumptions. Biol. Bull. 200, 51–58. doi: 10.2307/1543084
Krämer, W. E., Caamaño-Ricken, I., Richter, C., and Bischof, K. (2012). Dynamic regulation of photoprotection determines thermal tolerance of two phylotypes of Symbiodinium clade A at two photon fluence rates. Photochem. Photobiol. 88, 398–413. doi: 10.1111/j.1751-1097.2011.01048.x
Krueger, T., Becker, S., Pontasch, S., Dove, S., Hoegh-Guldberg, O., Leggat, W., et al. (2014). Antioxidant plasticity and thermal sensitivity in four types of Symbiodinium Sp. Phycol. Soc. Am. 50, 1035–1047. doi: 10.1111/jpy.12232
Kushmaro, A., Rosenberg, E., Fine, M., and Loya, Y. (1997). Bleaching of the coral Oculina patagonica by Vibrio AK-1. Mar. Ecol. Prog. Ser. 147, 159–165. doi: 10.3354/meps147159
LaJeunesse, T. (2002). Diversity and community structure of symbiotic dinoflagellates from Caribbean coral reefs. Mar. Biol. 141, 387–400. doi: 10.1007/s00227-002-0829-2
LaJeunesse, T. C., Pettay, D. T., Sampayo, E. M., Phongsuwan, N., Brown, B., Obura, D. O., et al. (2010). Long-standing environmental conditions, geographic isolation and host-symbiont specificity influence the relative ecological dominance and genetic diversification of coral endosymbionts in the genus Symbiodinium. J. Biogeogr. 37, 785–800. doi: 10.1111/j.1365-2699.2010.02273.x
LaJeunesse, T. C., Smith, R. T., Finney, J., and Oxenford, H. (2009). Outbreak and persistence of opportunistic symbiotic dinoflagellates during the 2005 Caribbean mass coral “bleaching” event. Proc. Biol. Sci. 276, 4139–4148.
Levin, R. A., Beltran, V. H., Hill, R., Kjelleberg, S., McDougald, D., Steinberg, P. D., et al. (2016). Sex, scavengers, and chaperones: Transcriptome secrets of divergent Symbiodinium thermal tolerances. Mol. Biol. Evol. 33, 2201–2215. doi: 10.1093/molbev/msw119
Lohbeck, K. T., Riebesell, U., and Reusch, T. B. H. (2012). Adaptive evolution of a key phytoplankton species to ocean acidification. Nat. Geosci. 5, 346–351. doi: 10.1038/ngeo1441
Little, A. F., van Oppen, M. J. H., and Willis, B. L. (2004). Flexibility in algal endosymbioses shapes growth in reef corals. Science 304, 1492–1494. doi: 10.1126/science.1095733
Matthews, J. L., Crowder, C. M., Oakley, C. A., Lutz, A., Roessner, U., Meyer, E., et al. (2017). Optimal nutrient exchange and immune responses operate in partner specificity in the cnidarian-dinoflagellate symbiosis. Proc. Natl. Acad. Sci. U.S.A. 114, 13194–13199. doi: 10.1073/pnas.1710733114
McGinty, E. S., Pieczonka, J., and Mydlarz, L. D. (2012). Variations in reactive oxygen release and antioxidant activity in multiple Symbiodinium types in response to elevated temperature. Microb. Ecol. 64, 1000–1007. doi: 10.1007/s00248-012-0085-z
Mieog, J. C., Olsen, J. L., Berkelmans, R., Bleuler-Martinez, S. A., Willis, B. L., and van Oppen, M. J. (2009). The roles and interactions of symbiont, host and environment in defining coral fitness. PLoS ONE 4:e6364. doi: 10.1371/journal.pone.0006364
Mieog, J. C., van Oppen, M. J. H., Cantin, N. E., Stam, W. T., and Olsen, J. L. (2007). Real-time PCR reveals a high incidence of Symbiodinium clade D at low levels in four scleractinian corals across the Great Barrier Reef: implications for symbiont shuffling. Coral Reefs 26, 449–457. doi: 10.1007/s00338-007-0244-8
Miller, J., Muller, E., Rogers, C., Waara, R., Atkinson, A., Whelan, K. R. T., et al. (2009). Coral disease following massive bleaching in 2005 causes 60% decline in coral cover on reefs in the US Virgin Islands. Coral Reefs 28, 925–937. doi: 10.1007/s00338-009-0531-7
Moberg, F., and Folke, C. (1999). Ecological goods and services of coral reef ecosystems. Ecol. Econ. 29, 215–233. doi: 10.1016/S0921-8009(99)00009-9
Mostafavi, P. G., Fatemi, S. M. R., Shahhosseiny, M. H., Hoegh-Guldberg, O., and Loh, W. K. W. (2007). Predominance of clade D Symbiodinium in shallow-water reef-building corals off Kish and Larak Islands (Persian Gulf, Iran). Mar. Biol. 153, 25–34. doi: 10.1007/s00227-007-0796-8
Muscatine, L., Falkowski, P. G., Porter, J. W., and Dubinsky, Z. (1984). Fate of photosynthetic fixed carbon in light- and shade-adapted colonies of the symbiotic coral Stylophora pistillata. Proc. R. Soc. B Biol. Sci. 222, 181–202. doi: 10.1098/rspb.1984.0058
Muscatine, L., and Pool, R. R. (1979). Regulation of numbers of intracellular algae. Proc. R. Soc. London. Ser. B Biol. Sci. 204, 131–139. doi: 10.1098/rspb.1979.0018
Pacifici, M., Foden, W. B., Visconti, P., Watson, J. E. M., Butchart, S. H. M., Kovacs, K. M., et al. (2015). Assessing species vulnerability to climate change. Nat. Clim. Chang. 5, 215–224. doi: 10.1038/nclimate2448
Pandolfi, J. M., Connolly, S. R., Marshall, D. J., and Cohen, A. L. (2011). Projecting coral reef futures under global warming and ocean acidification. Science 333, 418–422. doi: 10.1126/science.1204794
Pereira, H. M., Leadley, P. W., Proença, V., Alkemade, R., Scharlemann, J. P., Fernandez-Manjarrés, J. F., et al. (2010). Scenarios for global biodiversity in the 21st century. Science 330, 1496–1501. doi: 10.1126/science.1196624
Pernice, M., Dunn, S. R., Tonk, L., Dove, S., Domart-Coulon, I., Hoppe, P., et al. (2015). A nanoscale secondary ion mass spectrometry study of dinoflagellate functional diversity in reef-building corals. Environ. Microbiol. 17, 3570–3580. doi: 10.1111/1462-2920.12518
Pettay, D. T., Wham, D. C., Smith, R. T., Iglesias-Prieto, R., Lajeunesse, T. C., and Moran, N. A. (2015). Microbial invasion of the Caribbean by an Indo-Pacific coral zooxanthella. Proc. Natl. Acad. Sci. U.S.A. 112, 7513–7518. doi: 10.1073/pnas.1502283112
Pinzon, J. H., Kamel, B., Burge, C. A., Harvell, C. D., Medina, M., Weil, E., et al. (2015). Whole transcriptome analysis reveals changes in expression of immune-related genes during and after bleaching in a reef-building coral. R. Soc. Open Sci. 2, 140214–140214. doi: 10.1098/rsos.140214
Pochon, X., and Gates, R. D. (2010). A new Symbiodinium clade (Dinophyceae) from soritid foraminifera in Hawai'i. Mol. Phylogenet. Evol. 56, 492–497. doi: 10.1016/J.YMPEV.2010.03.040
Pochon, X., Gates, R. D., Vik, D., and Edmunds, P. J. (2014). Molecular characterization of symbiotic algae (Symbiodinium spp.) in soritid foraminifera (Sorites orbiculus) and a scleractinian coral (Orbicella annularis) from St John, US Virgin Islands. Mar. Biol. 161, 2307–2318. doi: 10.1007/s00227-014-2507-6
Pochon, X., Pawlowski, J., Zaninetti, L., and Rowan, R. (2001). High genetic diversity and relative specifcity among Symbiodinium-like endosymbiotic dinoflagellates in soritid foraminiferans. Mar. Biol. 139, 1069–1078. doi: 10.1007/s002270100674
Quigley, K. M., Davies, S. W., Kenkel, C. D., Willis, B. L., Matz, M. V., and Bay, L. K. (2014). Deep-sequencing method for quantifying background abundances of Symbiodinium types: exploring the rare Symbiodinium biosphere in reef-building corals. PLoS ONE 9:e94297. doi: 10.1371/journal.pone.0094297
Quigley, K. M., Willis, B. L., and Bay, L. K. (2016). Maternal effects and Symbiodinium community composition drive differential patterns in juvenile survival in the coral Acropora tenuis. R. Soc. Open Sci. 3:160471. doi: 10.1098/rsos.160471
Raftery, A. E., Zimmer, A., Frierson, D. M. W., Startz, R., and Liu, P. (2017). Less than 2 °C warming by 2100 unlikely. Nat. Clim. Chang. 7, 637–641. doi: 10.1038/nclimate3352
Ragni, M., Airs, R. L., Hennige, S. J., Suggett, D. J., Warner, M. E., and Geider, R. J. (2010). PSII photoinhibition and photorepair in Symbiodinium (Pyrrhophyta) differs between thermally tolerant and sensitive phylotypes. Mar. Ecol. Erogress Ser. 406, 57–70. doi: 10.3354/meps08571
R Core Team (2016). R Core Team. R Foundation Statistical Computing. Available online at: https://www.r-project.org/
Reynolds, J. M., Bruns, B. U., Fitt, W. K., and Schmidt, G. W. (2008). Enhanced photoprotection pathways in symbiotic dinoflagellates of shallow-water corals and other cnidarians. Proc. Natl. Acad. Sci. U.S.A. 105, 13674–13678. doi: 10.1073/pnas.0805187105
Robison, J. D., and Warner, M. E. (2006). Differential impacts of photoacclimation and thermal stress on the photobiology of four different phylotypes of Symbiodinium (Phyrrhophyta). J. Phycol. 42, 568–579. doi: 10.1111/j.1529-8817.2006.00232.x
Rosic, N., Ling, E. Y., Chan, C. K., Lee, H. C., Kaniewska, P., Edwards, D., et al. (2015). Unfolding the secrets of coral–algal symbiosis. ISME J. 9, 844–856. doi: 10.1038/ismej.2014.182
Rowan, R. (1998). Diversity and ecology of zooxanthellae on coral reefs. J. Phycol. 34, 407–417. doi: 10.1046/j.1529-8817.1998.340407.x
Rowan, R. (2004). Coral bleaching: thermal adaptation in reef coral symbionts. Nature 430, 742. doi: 10.1038/430742a
Sampayo, E. M., Ridgway, T., Bongaerts, P., and Hoegh-Guldberg, O. (2008). Bleaching susceptibility and mortality of corals are determined by fine-scale differences in symbiont type. Proc. Natl. Acad. Sci. U.S.A. 105, 10444–10449. doi: 10.1073/pnas.0708049105
Schlüter, L., Lohbeck, K. T., Gutowska, M. A., Gröger, J. P., Riebesell, U., and Reusch, T. B. H. (2014). Adaptation of a globally important coccolithophore to ocean warming and acidification. Nat. Clim. Chang. 4, 1024–1030. doi: 10.1038/nclimate2379
Schönberg, C. H. L., and Loh, W. K. W. (2005). Molecular identity of the unique symbiotic dinoflagellates found in the bioeroding demosponge Cliona orientalis. Mar. Ecol. Prog. Ser. 299, 157–166. doi: 10.3354/meps299157
Schönberg, C. H. L., Suwa, R., Hidaka, M., and Loh, W. K. W. (2008). Sponge and coral zooxanthellae in heat and light: preliminary results of photochemical efficiency monitored with pulse amplitude modulated fluorometry. Mar. Ecol. 29, 247–258. doi: 10.1111/j.1439-0485.2007.00216.x
Silverstein, R. N., Correa, A. M., and Baker, A. C. (2012). Specificity is rarely absolute in coral-algal symbiosis: implications for coral response to climate change. Proc. Biol. Sci. 279, 2609–2618. doi: 10.1098/rspb.2012.0055
Silverstein, R. N., Cunning, R., and Baker, A. C. (2017). Tenacious D:Symbiodiniumin clade D remain in reef corals at both high and low temperature extremes despite impairment. J. Exp. Biol. 220, 1192–1196. doi: 10.1242/jeb.148239
Stat, M., and Gates, R. D. (2011). Clade D Symbiodinium in scleractinian corals: a “nugget” of hope, a selfish opportunist, an ominous sign, or all of the above? J. Mar. Biol. 2011, 1–9. doi: 10.1155/2011/730715
Stat, M., Morris, E., and Gates, R. D. (2008). Functional diversity in coral-dinoflagellate symbiosis. Proc. Natl. Acad. Sci. U.S.A. 105, 9256–9261. doi: 10.1073/pnas.0801328105
Stat, M., Pochon, X., Franklin, E. C., Bruno, J. F., Casey, K. S., Selig, E. R., et al. (2013). The distribution of the thermally tolerant symbiont lineage (Symbiodinium clade D) in corals from Hawaii: correlations with host and the history of ocean thermal stress. Ecol. Evol. 3, 1317–1329. doi: 10.1002/ece3.556
Suggett, D. J., Goyen, S., Evenhuis, C., Szab,ó, M., Pettay, D. T., Warner, M. E., et al. (2015). Functional diversity of photobiological traits within the genus Symbiodinium appears to be governed by the interaction of cell size with cladal designation. New Phytol. 208, 370–381. doi: 10.1111/nph.13483
Suzuki, G., Yamashita, H., Kai, S., Hayashibara, T., Suzuki, K., Iehisa, Y., et al. (2013). Early uptake of specific symbionts enhances the post-settlement survival of Acropora corals. Mar. Ecol. Prog. Ser. 494, 149–158. doi: 10.3354/meps10548
Taguchi, S., and Kinzie, I. I. I.R. A. (2001). Growth of zooxanthellae in culture with two nitrogen sources. Mar. Biol. 138, 149–155. doi: 10.1007/s002270000435
Takabayashi, M., Adams, L. M., Pochon, X., and Gates, R. D. (2012). Genetic diversity of free-living Symbiodinium in surface water and sediment of Hawai‘i and Florida. Coral Reefs 31, 157–167. doi: 10.1007/s00338-011-0832-5
Takahashi, S., Whitney, S. M., and Badger, M. R. (2009). Different thermal sensitivity of the repair of photodamaged photosynthetic machinery in cultured Symbiodinium species. Proc. Natl. Acad. Sci. U.S.A. 106, 3237–3242. doi: 10.1073/pnas.0808363106
Thomas, L., Kendrick, G. A., Kennington, W. J., Richards, Z. T., and Stat, M. (2014). Exploring Symbiodinium diversity and host specificity in Acropora corals from geographical extremes of Western Australia with 454 amplicon pyrosequencing. Mol. Ecol. 23, 3113–3126. doi: 10.1111/mec.12801
Thornhill, D. J., LaJeunesse, T. C., Kemp, D. W., Fitt, W. K., and Schmidt, G. W. (2006). Multi-year, seasonal genotypic surveys of coral-algal symbioses reveal prevalent stability or post-bleaching reversion. Mar. Biol. 148, 711–722. doi: 10.1007/s00227-005-0114-2
Titlyanovl, E. A., Titlyanova, T. V., Leletkin, V. A., Tsukahara, J., Van Woesik, R., and Yamazato, K. (1996). Degradation of zooxanthellae and regulation of their density in hermatypic corals. Marime Ecol. Prog. Ser. 139, 167–178. doi: 10.3354/meps139167
Toller, W. W., Rowan, R., and Knowlton, N. (2001). Repopulation of Zooxanthellae in the Caribbean corals Montastraea annularis and M. faveolata following experimental and disease-associated bleaching. Biol. Bull. 201, 360–373. doi: 10.2307/1543614
Traylor-Knowles, N., Rose, N. H., Sheets, E. A., and Palumbi, S. R. (2017). Early transcriptional responses during heat stress in the coral Acropora hyacinthus. Biol. Bull. 232, 91–100. doi: 10.1086/692717
Urban, M. C. (2015). Climate change. Accelerating extinction risk from climate change. Science 348, 571–573. doi: 10.1126/science.aaa4984
van Oppen, M. J. H., Baker, A. C., Coffroth, M. A., and Willis, B. L. (2009). “Bleaching resistance and the role of algal endosymbionts,” in Coral Bleaching: Patterns, Processes, Causes and Consequences, Ecological Studies, eds M. J. H. van Oppen and J. M. Lough (Berlin; Heidelberg: Springer-Verlag), 83–102.
van Oppen, M. J. H., Gates, R. D., Blackall, L. L., Cantin, N., Chakravarti, L. J., Chan, W. Y., et al. (2017). Shifting paradigms in restoration of the world's coral reefs. Glob. Chang. Biol. 23, 3437–3448. doi: 10.1111/gcb.13647
van Oppen, M. J. H., Mahiny, A. J., and Done, T. J. (2005a). Geographic distribution of zooxanthella types in three coral species on the Great Barrier Reef sampled after the 2002 bleaching event. Coral Reefs 24, 482–487. doi: 10.1007/s00338-005-0487-1
van Oppen, M. J., Mieog, J. C., Sanchez, C. A., and Fabricius, K. E. (2005b). Diversity of algal endosymbionts (zooxanthellae) in octocorals: the roles of geography and host relationships. Mol. Ecol. 14, 2403–2417. doi: 10.1111/j.1365-294X.2005.02545.x
van Oppen, M. J., Oliver, J. K., Putnam, H. M., and Gates, R. D. (2015). Building coral reef resilience through assisted evolution. Proc. Natl. Acad. Sci. U.S.A. 112, 2307–2313. doi: 10.1073/pnas.1422301112
Wangpraseurt, D., Pernice, M., Guagliardo, P., Kilburn, M. R., Clode, P. L., Polerecky, L., et al. (2016). Light microenvironment and single-cell gradients of carbon fixation in tissues of symbiont-bearing corals. ISME J. 10, 788–792. doi: 10.1038/ismej.2015.133
Wilkerson, F. P., Kobayashi, D., and Muscatine, L. (1988). Mitotic index and size of symbiotic algae in Caribbean Reef corals. Coral Reefs 7, 29–36. doi: 10.1007/BF00301979
Wilkinson, S. P., Fisher, P. L., Jh Van Oppen, M., and Davy, S. K. (2015). Intra-genomic variation in symbiotic dinoflagellates: recent divergence or recombination between lineages? BMC Evol. Biol. 15:46. doi: 10.1186/s12862-015-0325-1
Keywords: Symbiodinium, adaptation, acclimation, symbiosis, ocean warming, climate change, assisted evolution
Citation: Chakravarti LJ and van Oppen MJH (2018) Experimental Evolution in Coral Photosymbionts as a Tool to Increase Thermal Tolerance. Front. Mar. Sci. 5:227. doi: 10.3389/fmars.2018.00227
Received: 23 April 2018; Accepted: 12 June 2018;
Published: 03 July 2018.
Edited by:
Christopher Edward Cornwall, Victoria University of Wellington, New ZealandReviewed by:
Clinton Alexander Oakley, Victoria University of Wellington, New ZealandChristopher Bennett Wall, Hawaii Institute of Marine Biology, University of Hawaii at Manoa, United States
Copyright © 2018 Chakravarti and van Oppen. This is an open-access article distributed under the terms of the Creative Commons Attribution License (CC BY). The use, distribution or reproduction in other forums is permitted, provided the original author(s) and the copyright owner(s) are credited and that the original publication in this journal is cited, in accordance with accepted academic practice. No use, distribution or reproduction is permitted which does not comply with these terms.
*Correspondence: Leela J. Chakravarti, bGVlbGFqY2hha3JhdmFydGlAZ21haWwuY29t