- 1Leibniz Institute for Baltic Sea Research, Rostock, Germany
- 2GEOMAR Helmholtz Centre for Ocean Research Kiel, Kiel, Germany
- 3NIOZ Royal Netherlands Institute for Sea Research, Utrecht University, Texel, Netherlands
- 4Leibniz Institute of Freshwater Ecology and Inland Fisheries, Berlin, Germany
- 5Leibniz Institute for Plasma Science and Technology, Greifswald, Germany
The dissolved organic phosphorus (DOP) pool in marine waters contains a variety of different compounds. Knowledge of the distribution and utilization of DOP by phyto- and bacterioplankton is limited, but critical to our understanding of the marine phosphorus cycle. In the Baltic Sea, detailed information about the composition of DOP and its turnover is lacking. This study reports the concentrations and uptake rates of DOP compounds, namely, adenosine triphosphate (dATP), deoxyribonucleic acid (dDNA), and phospholipids (dPL), in the Baltic Proper and in Finnish coastal waters in the summers of 2011 and 2012. Both areas differed in their dissolved inorganic phosphorus (DIP) concentrations (0.16 and 0.02–0.04 μM), in the C:P (123–178) and N:P (18–27) ratios, and in abundances of filamentous cyanobacteria and of autotrophic and heterotrophic picoplankton. The mean concentrations of dATP-P, dDNA-P, and dPL-P were 4.3–6.4, 0.05–0.12, and 1.9–6.8 nM, respectively, together contributing between 2.4 and 5.2% of the total DOP concentration. The concentrations of the compounds varied between and within the investigated regions and the distribution patterns of the individual components are not linked to each other. DIP was taken up at rates of 10.1–380.8 nM d-1. dATP-P and dDNA-P were consumed simultaneously with DIP at rates of 6.9–24.1 and 0.09–0.19 nM d-1, respectively, with the main proportion taken up by the size fraction <3 μm and with DIP to be the dominant source. Groups of hydrographical and biological parameters were identified in the multiple regression analysis to impact the concentrations and uptake rates. It points to the complexity of the regulation. Our results indicate that the investigated DOP compounds, particularly dATP-P, can make significant contributions to the P nutrition of microorganisms and their use seems to be not intertwined. Therefore, more detailed knowledge of all DOP components including variation of concentrations and the utilization is required to understand the roles of DOP in marine ecosystems.
Introduction
In the marine phosphorus (P) cycle, dissolved inorganic phosphorus (DIP), particulate inorganic (PIP) and organic phosphorus (POP) and dissolved organic phosphorus (DOP) are the main P pools. The transformations between the various pools have been described in several studies, e.g., Jauzein et al. (2010), White et al. (2012), Karl (2014), however, a complete understanding of the P cycle is lacking. DIP is consumed by phyto- and bacterioplankton and incorporated into biomass, forming POP, and following cell death and remineralization is partly released as DOP into the surrounding water. DOP acts as an additional P source to microbial organisms, especially when it dominates the dissolved P pool, as observed in oligotrophic regions of the ocean (Cavender-Bares et al., 2001) and in marine ecosystems where DIP is seasonally depleted (Mather et al., 2008; Lomas et al., 2010).
Dissolved organic phosphorus concentrations are reported to range between 0.06 and 0.54 μM in ocean surface waters (Karl and Björkman, 2015), but can be elevated in coastal waters due to enhanced productivity, as reported by Lin et al. (2012) for the St. Louis estuary (Gulf of Mexico), where DOP levels ranged from 0.16 to 0.67 μM. In the Baltic Sea, investigations of DOP are sparse and mostly limited to measurements of concentrations, with few studies on the bioavailable fraction. Concentrations of DOP in coastal regions of the Baltic Sea reached up to 0.98 μM, while in the central Baltic Sea the observed concentration range was 0.2–0.52 μM (Hoikkala et al., 2015). The DOP pool has been characterized in terms of its molecular size and compound groups. DOP includes low and high molecular weight compounds (LMW-DOP < 10 kDa and HMW-DOP > 10 kDa) (Ridal and Moore, 1990; Clark et al., 1999; Young and Ingall, 2010). The DOP fraction < 10 kDa comprises 50–80% of the DOP pool in open ocean surface waters (Benitez-Nelson, 2000) and 54–76% in the Bay of Tokyo (Suzumura et al., 1998). Concerning compound groups, the DOP pool includes phosphoesters (mono- and di-esters) and phosphonates (Kolowith et al., 2001; Monbet et al., 2009; Repeta et al., 2016). The majority of the DOP pool consists of phosphoesters (Young and Ingall, 2010) including sugar phosphates, vitamins, nucleotides such as adenosine mono- and tri-phosphate (AMP, ATP) and nucleic acids, i.e., deoxyribonucleic (DNA) and ribonucleic acid (RNA) (Karl and Björkman, 2015). All these substrates are assumed to be bioavailable to the microbial community after enzymatic degradation. Phospholipids (PL) are hydrophobic compounds assumed to have a relatively low bioavailability (Björkman and Karl, 1994; Suzumura and Ingall, 2004). Phosphonates are considered to form part of the recalcitrant DOP fraction (Suzumura et al., 1998). However, several bacteria and cyanobacteria are reported to be genetically equipped for phosphonate utilization (Dyhrman et al., 2006; Feingersch et al., 2012; Repeta et al., 2016). Siuda and Chrost (2001) showed that P is hydrolyzed through enzymatic processes from DOP compounds to various degrees, with the highest proportion of P released from nucleotides.
ATP has been used as a proxy for the labile DOP fraction (Casey et al., 2009), with radioactive [33P]-labeled ATP used to quantify labile DOP uptake by organisms. The uptake of ATP is influenced by nutrient conditions, besides other factors like temperature. However, Casey et al. (2009) and Michelou et al. (2011) observed taxon-specific differences in the degree of ATP uptake under identical environmental conditions. In the North Pacific subtropical gyre, the ATP uptake is realized by heterotrophic bacteria rather than by pigmented picoplankton (Björkman et al., 2012). Accordingly, there seems to be a broad range of utilization of DOP compounds by microorganisms in marine waters.
In semi-enclosed waters such as the Baltic Sea, knowledge of the DOP composition and turnover of specific DOP components is still lacking. However, determining the DOP composition is the first step toward understanding the dynamics of the DOP pool and its functions, which is the basis for a comprehensive understanding of the P cycle. For this purpose, we studied the concentrations of the DOP compounds ATP, PL, and DNA, as well as their spatial and temporal variations and the utilization of ATP and DNA in relation to DIP, in the summer season, when DOP usually forms the main dissolved P source for microorganisms (Nausch et al., 2004, 2008). We aimed to understand the contribution of these compounds to the DOP pool and to the P nutrition of phyto- and bacterioplankton in the Baltic Sea.
Materials and Methods
Study Sites and Hydrographical Conditions
Dissolved organic phosphorus compounds in the central Baltic Sea were investigated during a cruise with RV ELISABETH-MANN-BORGESE along a north-south transect through the Baltic Proper (Figure 1) from 25 to 28 July 2011. Weak to moderate winds were observed, varying from the south-southwest to the west-northwest directions. The surface temperature of 16.5 ± 0.5°C and salinity of 7.10 ± 0.07 at the southern stations (TF 222–TF 263) differed from those at more northern stations (TF272-CS_LL19), which had an observed temperature of 17.6 ± 0.4°C and a salinity of 6.92 ± 0.21, indicating warmer water with a slightly lower salinity (Figure 1 and Table 1).
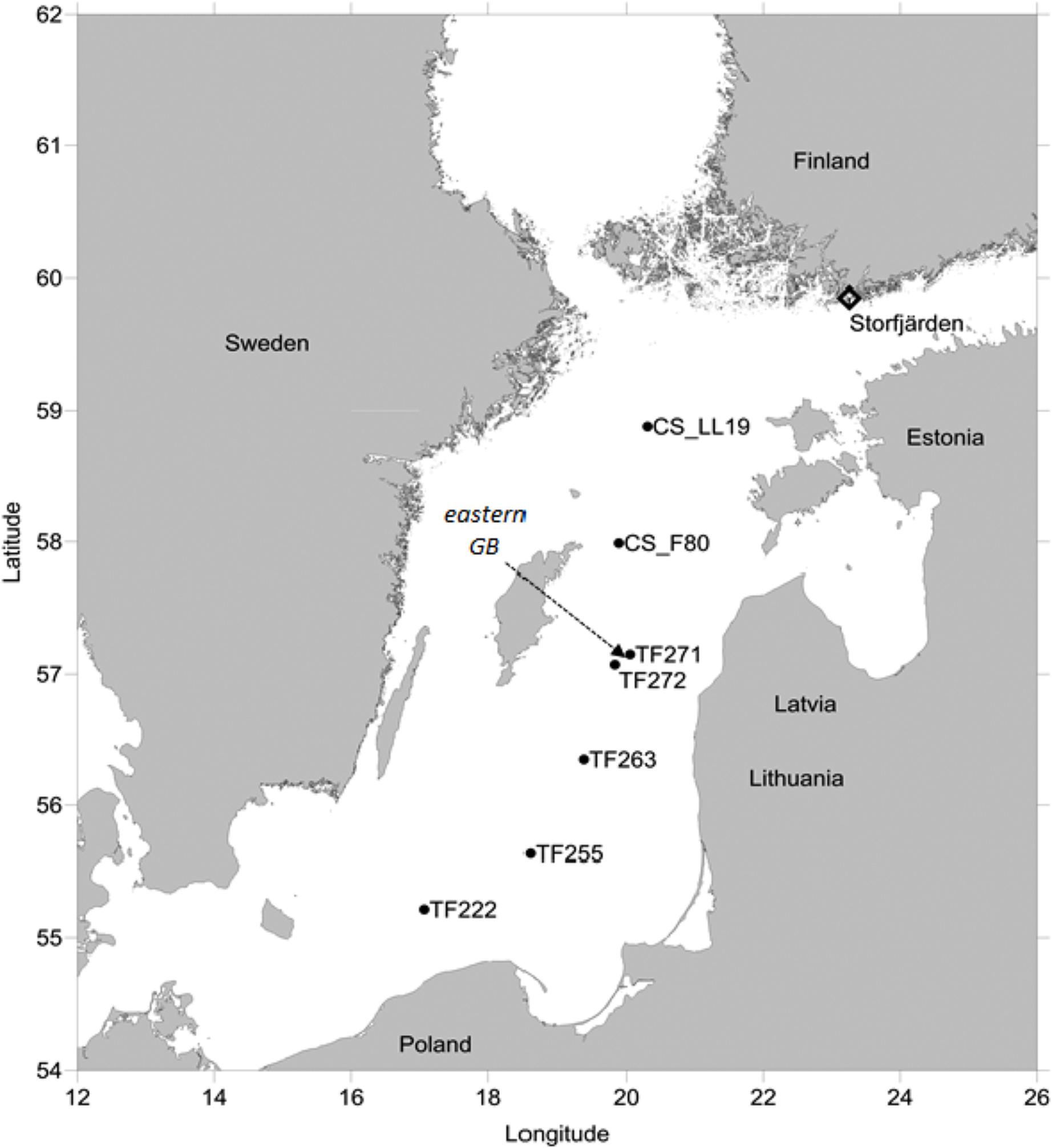
FIGURE 1. Stations in the Baltic Proper and in the Storfjärden where investigations were undertaken in the summers 2011 and 2012, respectively. The arrow points to the station in the eastern Gotland Basin (GB) where time series measurements were conducted in summer 2012 concurrent with the measurements in the Storfjärden.
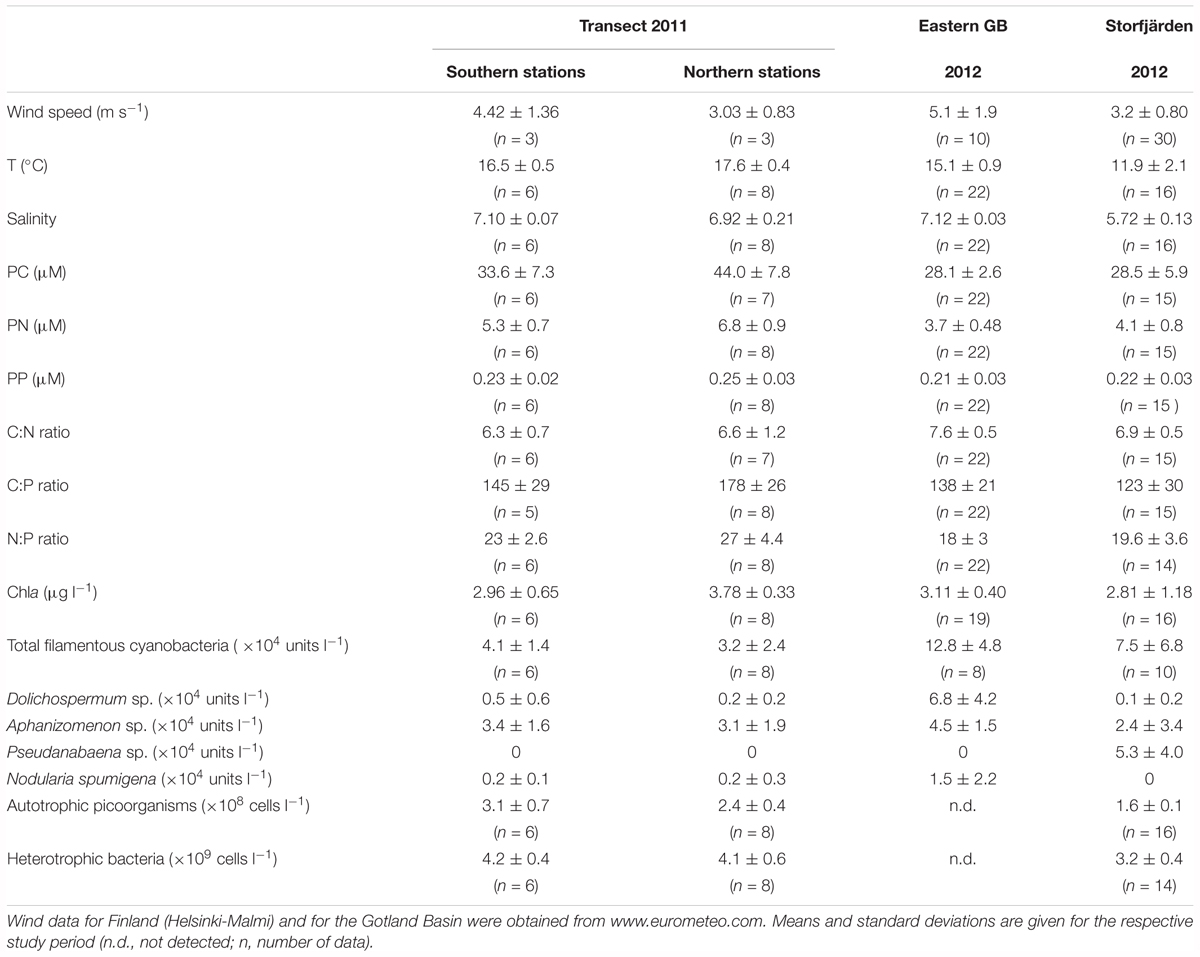
TABLE 1. Wind, hydrographical, and biological conditions in both sub-regions of the transect in the Eastern Gotland Basin (GB) and in the Storfjärden.
Time series data were collected in the eastern Gotland Basin (GB) and in Storfjärden (northwestern Gulf of Finland) (Figure 1). In the eastern GB (station TF 271: 57°19.2′ N, 20°30.0′ E), sampling was conducted onboard RV METEOR from 7 to 20 July 2012, with a water temperature of 15.1 ± 0.9°C and a salinity of 7.12 ± 0.03 in the upper mixed surface layer. In Storfjärden, the study was undertaken between 19 June and 24 July 2012 in the proximity of the Tvärminne Zoological Station (59°51.5′ N, 23°15.5′ E). The station was influenced by wind-driven upwelling events of varying intensities that caused variations in water temperatures, which ranged from 8.1 to 15.9°C (mean 11.9 ± 2.1°C) and with salinities ranging between 5.46 and 5.97 (Paul et al., 2015).
Water Sampling
Central Baltic Sea
On-board the research vessels, water was sampled using a rosette sampler (HydroBios) equipped with 5-L free flow bottles from 1 and 10 m depth to cover the surface mixed layer above the thermocline. The combined Seabird SBE911 probe was equipped with sensors for conductivity, temperature, depth, and chlorophyll fluorescence. Water from the bottles was collected in pre-cleaned (HCl- and deionized water) polyethylene containers that had been pre-rinsed with sample water. From the containers, subsamples were taken for each parameter and for uptake rate measurements immediately after sampling.
Storfjärden
For the DOP, other P-fractions, chlorophyll and picoplankton analyses, samples were collected every second day over a depth of 17 m using a depth-integrating water sampler (IWS HydroBios) that was lowered slowly on a cable by hand from a small boat (see Nausch et al., 2016 for further details). For the DOP compounds, samples were taken every fourth day.
Analytical Methods
Chlorophyll a and Particulate Carbon
Water (300–800 ml) for Chlorophyll a (Chla) analysis was filtered (200 mbar pressure difference) through glass fiber filters (GF/F, Whatman, diameter 25 mm) with a nominal pore size of 0.7 μm. The filters were flash frozen in liquid nitrogen and stored at -80°C until extraction with 96% ethanol for at least 3 h (Wasmund et al., 2006). Chla fluorescence was measured with a TURNER fluorometer (10-AU-005) at 450 nm excitation and 670 nm emission (Welschmeyer, 1994). The Chla extraction procedure for the Storfjärden samples was different. For these samples, the GF/F filters were extracted with 90% acetone after homogenization in a cell mill for 5 min (Paul et al., 2015). According to Wasmund et al. (2006), both methods provide comparable results.
Particulate carbon (PC) was analyzed from 300 to 800 ml samples filtered (200 mbar pressure difference) onto pre-combusted (450°C, 6 h) GF/F filters (Whatman, diameter 25 mm) and stored frozen at -20°C until analysis. Samples from the cruises were measured by flash combustion using a Carlo Erba EA 1108 at 1020°C in the IOW and using a EuroEA elemental analyzer coupled with a Conflo II interface to a Finnigan DeltaPlus mass spectrometer for samples from Storfjärden.
Filamentous Cyanobacteria and Picoplankton
Water samples for the microscopic analyses were preserved with acetic Lugol (KI/I2) (1% v/v final concentration) and settled in an Utermöhl sedimentation chamber for 24 h. The species composition and abundance were assessed with an inverted microscope (ZEISS or Leica) at 100× magnification. Filaments were enumerated as units; one unit corresponds to a filament length of 100 μm.
Picoplankton was counted using a FACS Calibur flow cytometer (BD Biosciences) equipped with a 488 nm laser. The detection of autotrophic picoplankton was based on their autofluorescence and side-scatter properties, whereas bacteria were stained with SYBRGreen I (Molecular Probes) before counting. SYBR green fluorescence was plotted versus the side-scatter traits. Populations were clustered using the software program “Cell Quest Pro” (Gasol and Del Giorgio, 2000).
Inorganic and Organic Phosphorus
Dissolved inorganic phosphorus was determined colorimetrically in an autoanalyzer (Alliance Instruments) according to Grasshoff et al. (1983) onboard the research vessels during the cruises. The detection limit was 0.02 μM. During the Storfjärden time-series, we used a segmented continuous-flow analyzer coupled with a liquid-waveguide capillary flow-cell (LWCC) of 2 m length to determine DIP with a detection limit of 0.8 nM (Patey et al., 2008). These measurements were conducted at the Tvärminne Zoological Station.
Total phosphorus (TP) and dissolved phosphorus (DP) were analyzed from 40 ml unfiltered and filtered (pre-combusted GF/F-filters, 450°C, 6 h) water samples, respectively, stored at -20°C until further treatment. During the analysis, TP and DP samples were oxidized to DIP in a laboratory microwave equipped with 40 Teflon tubular vessels in an alkaline medium (Grasshoff et al., 1983). The resulting DIP concentration was measured in a 10 cm cuvette with a precision of 0.01 μM. DOP was calculated as the difference between the DP and DIP concentrations.
Particulate phosphorus (PP) was calculated as the difference between TP and DP. The microwave digestion does not discriminate between organic and inorganic P because all P is transformed into DIP. However, due to the correlation with Chla, we assumed that PP consisted predominantly of POP.
DOP Compounds
The samples for DOP compound analysis were filtered through pre-combusted (6 h, 450°C) GF/F filters, followed by filtration through 0.2 μm cellulose acetate filters (Sartorius Stedim). Subsamples of the filtrate were prepared for storage according to the specific method used for each compound. The subsamples were analyzed as described by Unger et al. (2013); each compound is described below by its P content.
Dissolved adenosine triphosphate (dATP)
Dissolved adenosine triphosphate (dATP) was determined according to the method of Björkman and Karl (2001), which was adapted by Unger et al. (2013) to Baltic Sea conditions. A Mg(OH)2 precipitate was prepared by the addition of 1 M NaOH (0.5% v/v) to 200 ml of the filtered seawater samples. The precipitate was concentrated by centrifugation at 1680 g for 1.5 h. The resulting pellet was dissolved by dropwise addition of 5 M HCl; the pH was adjusted to 7.2 using TRIS buffer (pH 7.4, 20 mM). The dATP concentrations were detected by bioluminescence flash analysis in a Sirius luminometer (Bertold) after addition of firefly latern extract (FLE-250, Sigma-Aldrich) with a detection limit of 2.5 pM. A standard series of adenosine 5′-triphosphate disodium salt hydrate (Sigma-Aldrich) was applied to each sample set. The P content of the dATP (ATP-P) was calculated by assuming that 1 mol ATP was equivalent to 3 moles P.
Dissolved phospholipids (dPL)
The concentration of phosphate bound to dPL (dPL-P) was analyzed according to Suzumura and Ingall (2004). To do so, 400 ml of the filtered water was stored at -20°C. Prior to analysis, samples were thawed and extracted twice with 100 ml of chloroform. The chloroform phase was concentrated in a rotary evaporator, transferred into microwave tubes and completely evaporated in a 60°C water bath. After addition of 20 ml of deionized water, the samples were digested in a microwave in alkaline medium, as done for the TP and DP analyses. A set of standard concentrations of L-phosphatidyl-DL-glycerol sodium salt (Sigma Aldrich) prepared in Baltic Sea water and chloroform as blank were analyzed in the same way as the samples. The detection limit of the concentrated samples was 0.8 nM.
Dissolved DNA (dDNA)
The concentration of dDNA was measured according to Karl and Bailiff (1989). A total of 200 ml of the filtered sample was gently mixed with equivalent amounts of ethylene-diamine-tetraacetic acid (EDTA, 0.1 M, pH 9.3, Merck) plus 4 ml of cetyltrimethyl-ammonium bromide (CTAB, Sigma-Aldrich) and stored at -20°C for at least 24 h. After thawing the samples, the precipitate was filtered onto pre-combusted (450°C, 6 h) GF/F filters (25 mm diameter) and stored at -80°C. DNA was measured using a fluorescence-spectrophotometer (excitation 404 nm, emission 502 nm) with a detection limit of 20 ng. DNA standards (Sigma Aldrich D3779) were prepared in seawater and processed as described above. The P content of the DNA was calculated by multiplying the measured DNA concentration by 2.06 nmol P∗μg dDNA-1 detected by alkaline digestion of DNA-sodium salt from calf thymus (SIGMA-ALDRICH) (Trinkler, 2009).
Uptake rates of DIP, ATP, and DNA
To measure the DIP uptake rates, [33P]PO4 (specific activity: ∼110 TBq mmol-1) was added to 50 ml of water sample at a final concentration of 50 pM. Isotope-labeled samples were incubated under laboratory light conditions and in situ temperatures. For the controls, formaldehyde was added at a final concentration of 1% before radiotracer addition. During the incubation period of 2–2.5 h, 5 ml subsamples were harvested every 30 min. and amended with 100 μM non-radioactive PO4 to halt the [33P]PO4 incorporation (Thingstad et al., 1993), which was followed by immediate filtration on polycarbonate filters (Millipore, 0.2 and 3 μm pore size) that had been pre-soaked with 1 mM KH2PO4.
The [33P]PO4 uptake was measured in a Perkin Elmer liquid scintillation counter using IrgaSafe as scintillation cocktail. The [33P]PO4 uptake rate (% h-1) is the consumed fraction from the added [33P]PO4 per time calculated from the linear slope of increasing counts on the filters (Nausch et al., 2012). The gross uptake rate was calculated from the [33P]PO4 uptake rate and the in situ DIP concentration. Turnover times (Tt) were calculated by the equation Tt = t/-ln(1-r), where t = incubation time, r = fraction of added [33P]PO4 consumed after the incubation time (Thingstad et al., 1993).
Filters of 0.2 μm filters and 3 μm pore size were used to discriminate the uptake rates of the total community (>0.2 μm) and the size fractions >3 μm, which mostly contained phytoplankton and particle associated bacteria. The uptake into the size fraction <3 μm, which contained autotrophic and heterotrophic picoplankton, was calculated by the difference of the radioactivity on the 0.2 and 3 μm filters.
The method for ATP uptake measurements was derived from the protocol of Ammerman (1993) developed for the detection of ATP hydrolysis and the uptake of hydrolysed P. Here only the uptake of hydrolysed ATP were measured by addition of [33P]ATP (specific activity: 111 TBq mmol-1) to 10 ml samples and to formaldehyde-treated (1%) controls at a final concentration of 50 pM. The samples were incubated in the dark at in situ temperature for 1 h. The uptake was halted by addition of 200 μl of a cold 20 mM ATP solution to the samples, which were then processed and calculated as described for the PO4 uptake measurements.
To measure DNA uptake rates, radiolabeled DNA was prepared using the Amersham Nick Translation Kit (GE Healthcare Life Sciences). According to the manufacturer instructions, 3.7 MBq of [33P]dCTP (specific activity > 111 TBq mmol-1) was paired with 1 μg DNA (Lovdal et al., 2007). The specific activity of the produced [33P]DNA was about 2 × 107 cpm μg-1. About 0.3 μg L-1[33P]DNA (0.6 nM nucleotide P) were added to the samples and handled as described for the [33P]ATP uptake rate measurements.
Statistical Analysis
Spearman rank correlations, ANOVA and t-tests were performed using the online program http://www.socscistatistics.com/tests/studentttest/Default.aspx. The t-test was applied to check whether the differences between the northern and the southern part of the transect were significant. ANOVA was used to test whether the differences in the turnover times of DIP, dATP-P, and dDNA-P in each part of the transect was significant. The correlation of the DOP compounds with Chla or with other parameters was examined using the Spearman rank correlation. The multiple regression analysis, performed with Excel 2013, was used to determine which factors influenced the variations in DOP and in the measured compounds. The significance level of p < 0.05 was used in all applications. Additionally, principal component analysis (PCA), performed with the computer program SPSS statistics 22, was applied to identify the parameter groups influencing the DOP compound distribution patterns.
Results
Transect in 2011
DOP Concentrations and Compounds
There were no significant differences in the TP, DOP, and DIP concentrations between the southern and the northern stations of the transect through the central Baltic Sea (Tables 2, 3). DIP concentrations of 0.02 and 0.03 μM were near the detection limit of the analytical method, and >88% of the DP pool was DOP. The DOP concentrations varied marginally between the stations (Figure 2A), with a maximum difference of 0.05 μM between the lowest and the highest value. Multiple regression analysis showed that none of the single parameters, e.g., Chla, PC concentrations, C:P ratios, temperature or bacteria abundances, had a significant influence on the DOP concentrations (Table 5 and Supplementary Figure 1). Together, the single parameters explained 99% of the variation, and the sum of the parameters PP, DIP, POC, PON, the C:N ratio and the C:P ratio explained approximately 88% of the variation.
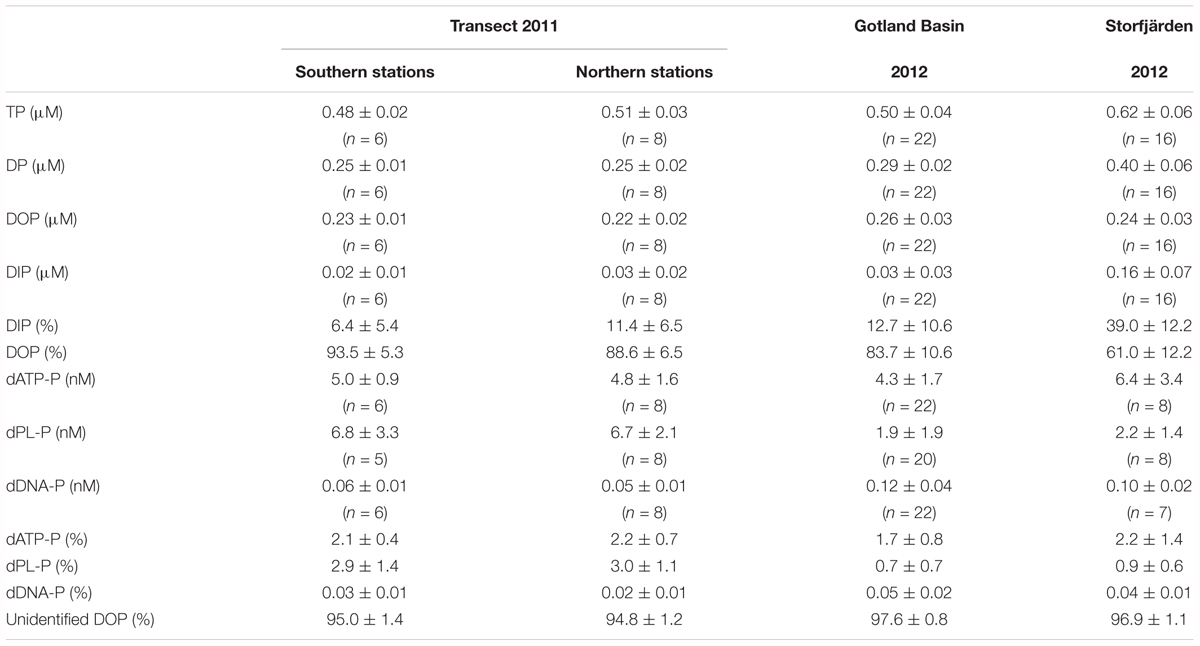
TABLE 2. Mean concentrations and standard deviation of P-pool sizes, its contribution to total dissolved phosphorus (DP), and the contribution of single components (dATP-P, dPL-P, dDNA-P) to the DOP pool in three studies conducted in the Baltic Sea (n, number of data).
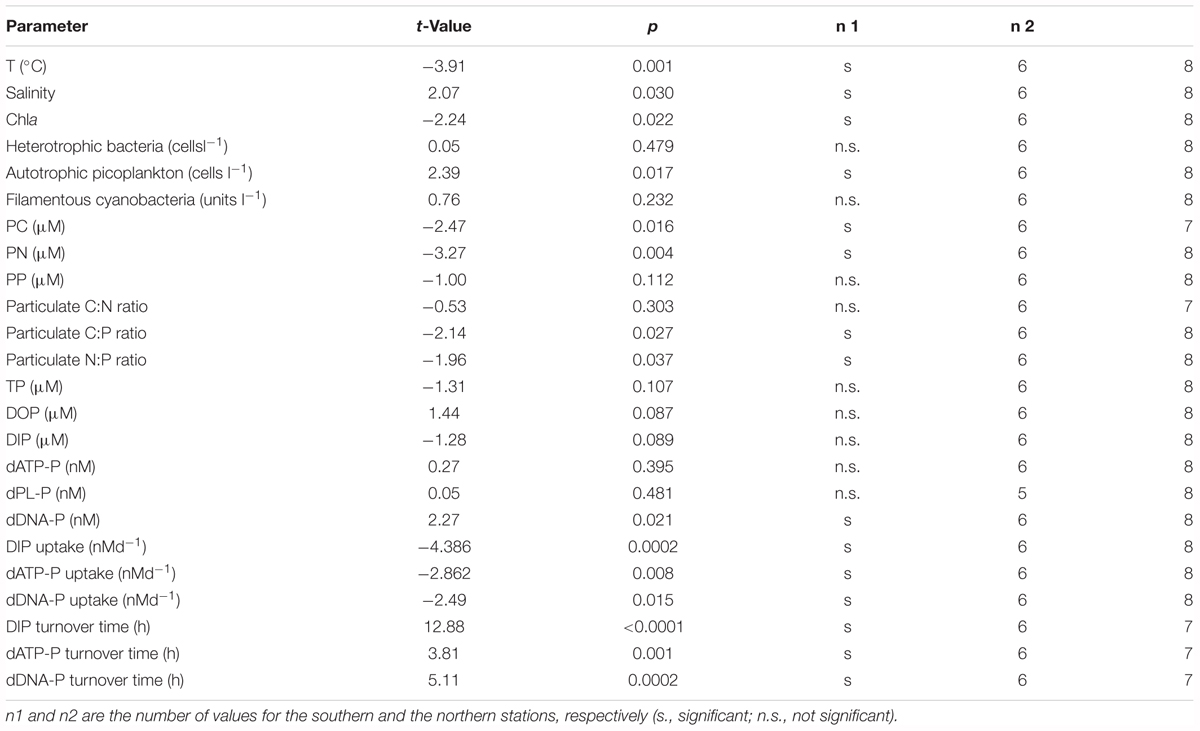
TABLE 3. Results of the t-test to validate if the differences between the southern and the northern stations of the transect investigated in summer 2011 were significant at a level p < 0.05.
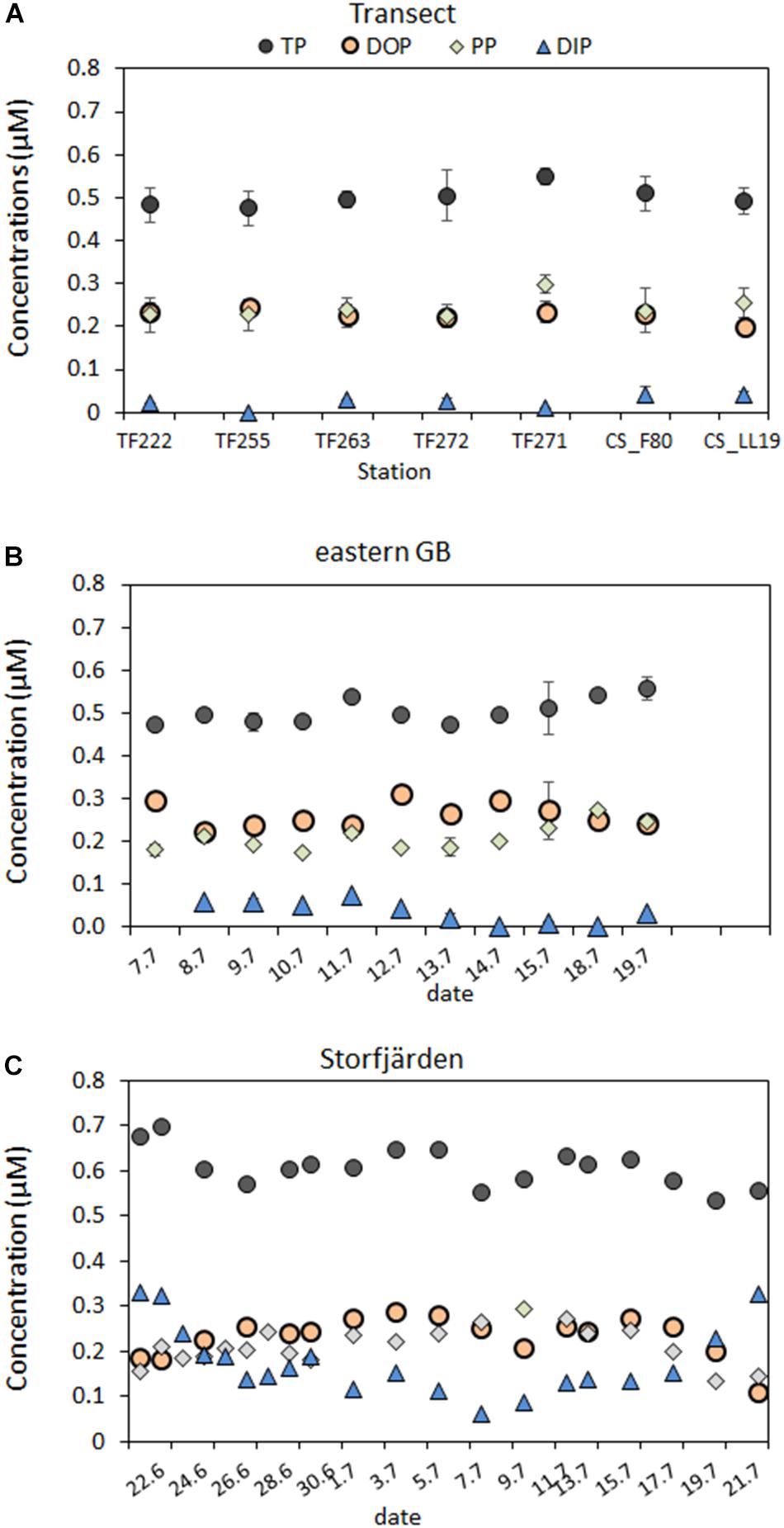
FIGURE 2. P-pool sizes (in μM) for the mixed surface layer above the thermocline along the transect through the Baltic Proper (A) and of the time series in the eastern GB (B) and in the Storfjärden (C). Means of the 1 and 10 m are used for the transect and the eastern GB and for a depth integrated sample in the Storfjärden.
The dATP-P, dPL-P, and dDNA-P concentrations were in the nanomolar range (Figure 3 and Table 2) and together constituted only 2.4–5.2% (mean 4.1%, n = 14) of DOP. The other > 95% of the DOP remained unidentified in our study (Table 2). The dATP-P concentrations varied between 2.6 and 7.4 nM along the whole transect, and there were no significant differences between the southern and the northern parts of the transect (Tables 2, 3). However, the dATP-P concentration range was greater at the northern than at the southern stations, with both lower and higher concentrations (Figure 3A).
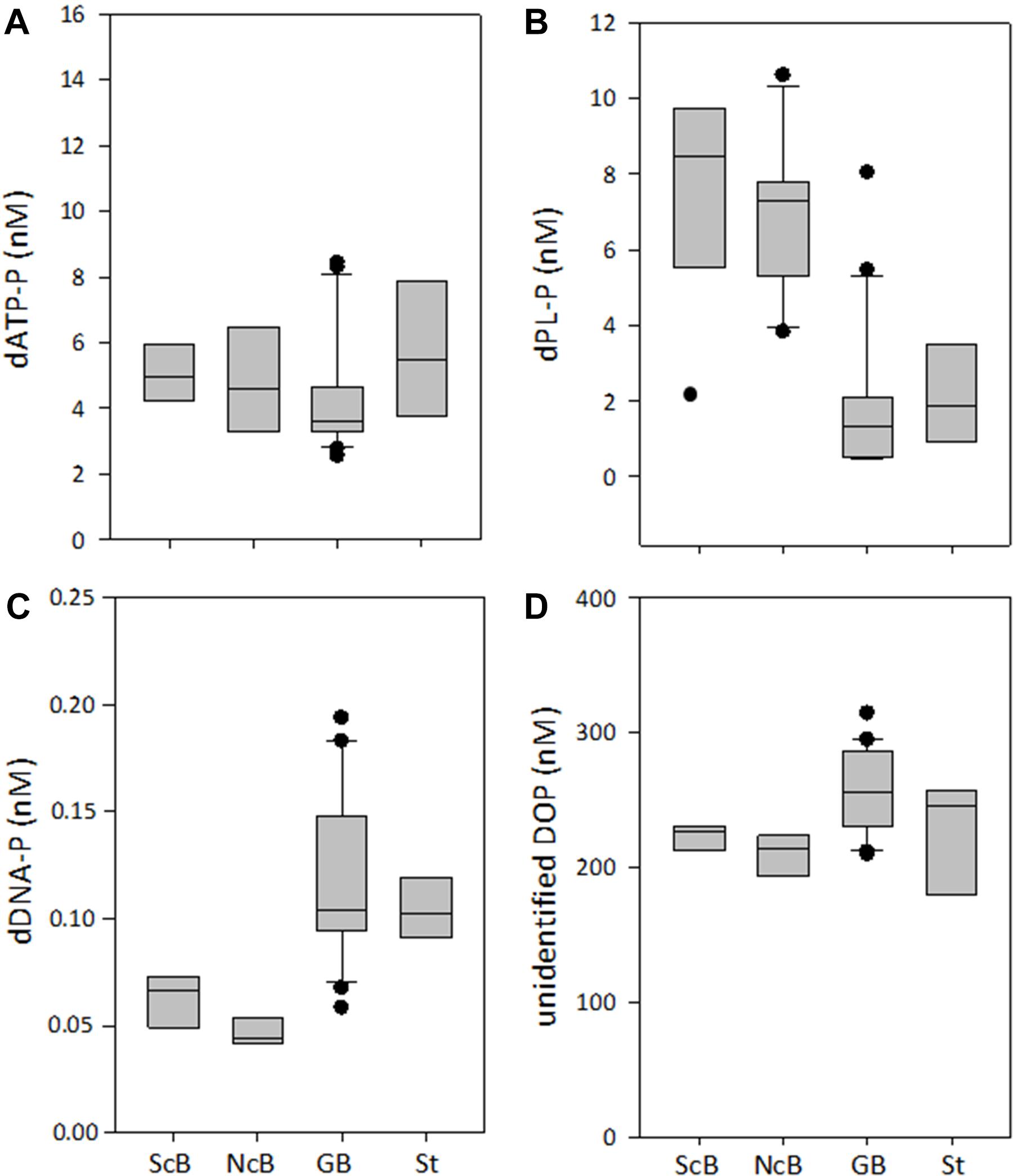
FIGURE 3. Concentrations of the DOP compounds dATP-P (A), dPL-P (B), dDNA-P (C), and of the unidentified DOP (D) in the two sub-regions of the transect [southern part (ScB); northern part (NcB)], in the eastern Gotland Basin (GB) and in the Storfjärden (St). The line inside the box represents the median value; the box is edged by the 25 and 75% percentile and the error bars indicate the 10 and 90% percentile.
Among the three analyzed DOP compounds, dPL-P had the highest concentrations ranging between 2.1 and 10.6 nM along the whole transect (Figure 3B). The concentrations of dDNA-P (0.04–0.07 nM) were two orders of magnitude lower than those of the other compounds (Figure 3C). Both dPL-P and dDNA-P showed slightly higher concentrations at the southern stations than at the northern stations. However, in the t-test, the differences between the two parts of the transect were significant only for dDNA-P (Table 3). Although dATP-P and dPL-P were not related to any other of the investigated parameters in the Spearman rank correlation test (Table 4), dDNA-P was inversely related to DIP, PON and the C:P ratio. As observed for DOP, the multiple regression analysis (Table 5 and Supplementary Figure 1) did not indicate that any other single parameter had a significant influence on the variations in the three DOP compounds. However, together they explained up to 91% of the dDNA-P variation, 98% of the dATP-P variation and 78% of the dPL-P variation. The sum of the Chla, PP, DIP, PC, PN, C:N ratio and C:P ratio could explain 89% of the dDNA-P variation, 27% of the dATP-P variation and 41% of the dPL-P variation.
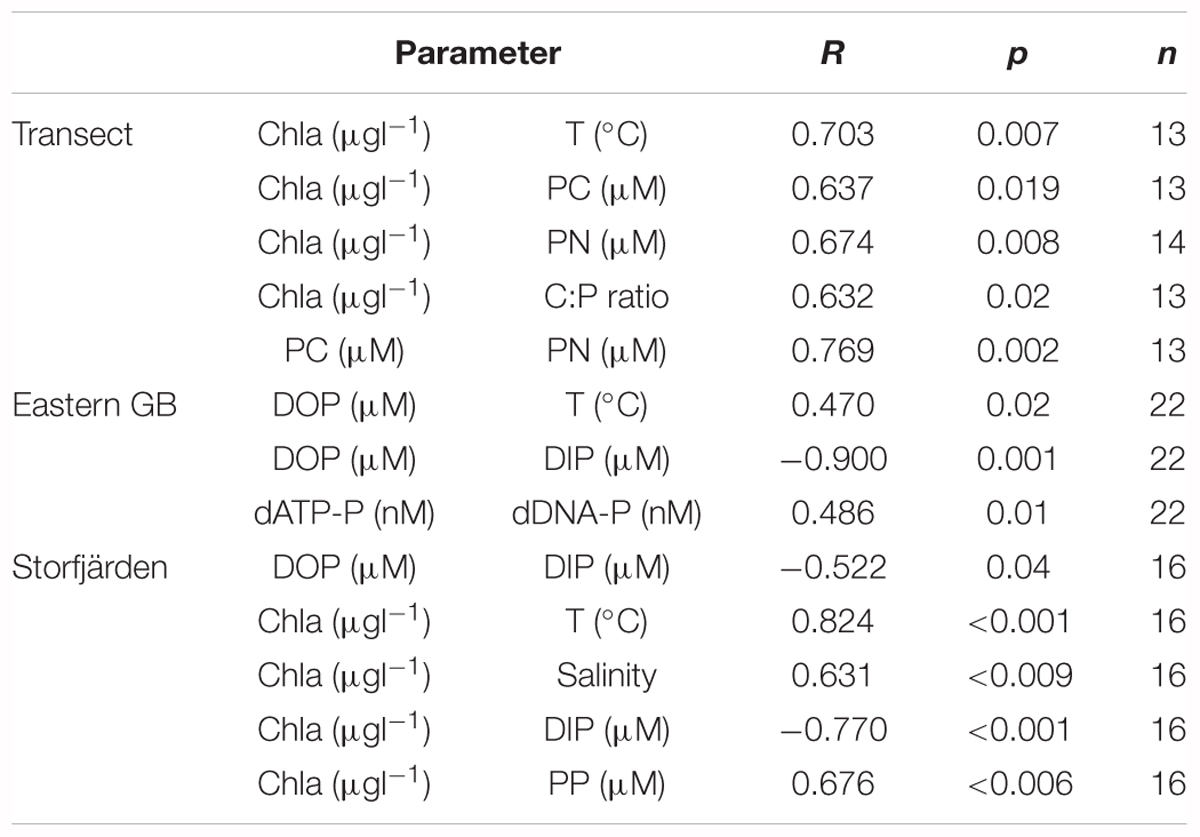
TABLE 4. Relationships between DOP, dATP-P and Chla and other parameters that were significant in the Spearman Rank correlation test.
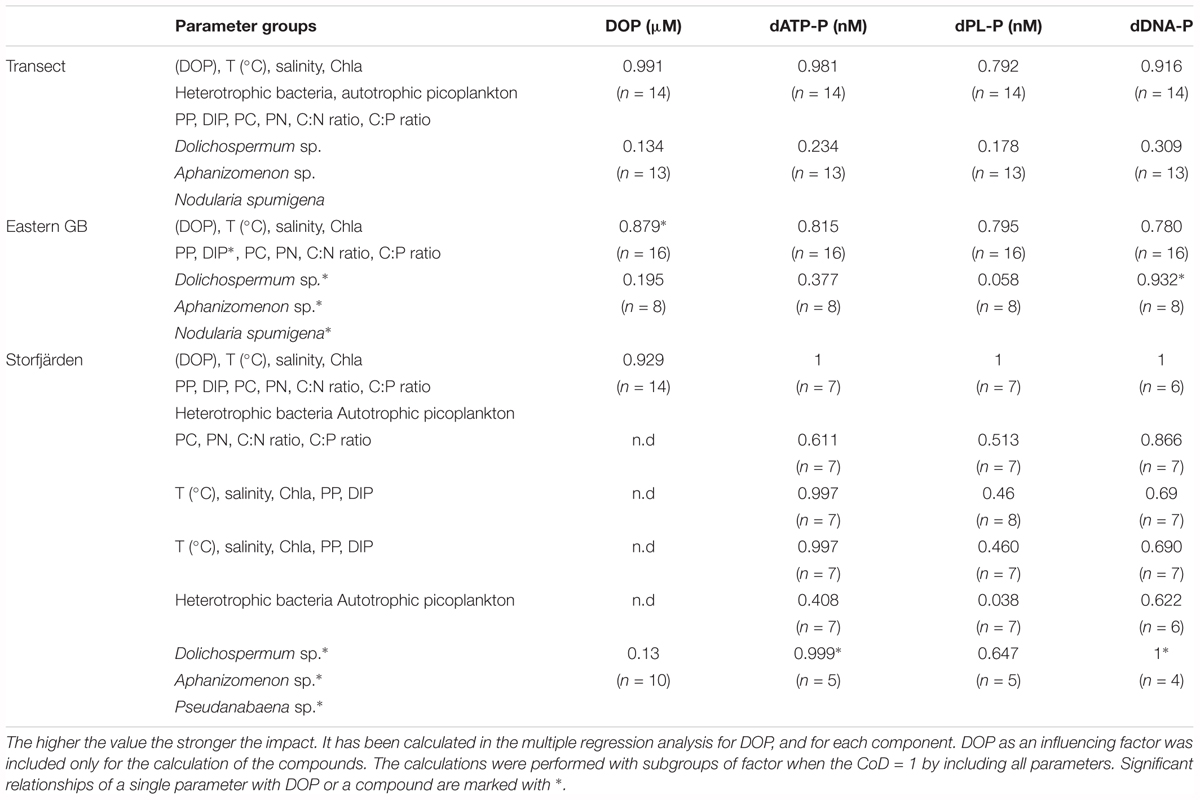
TABLE 5. Coefficients of determination (CoD) to describe the impact of parameter groups on concentrations of DOP, dATP-P, dPL-P, and dDNA-P.
Phytoplankton and Bacteria
The average Chla concentration at the three southern stations, 3.0 ± 0.7 μg l-1 (n = 6), was significantly lower than that at the northern stations, 3.8 ± 0.3 μg l-1 (n = 8) (Tables 1, 3). The abundances of filamentous cyanobacteria were comparable for both areas, and picoplankton counts were significantly higher in the southern than in the northern area; therefore, these components do not explain the observed difference in Chla concentrations (Tables 1, 3). The diazotrophic filamentous cyanobacteria were numerically dominated by Aphanizomenon sp. along the whole transect, representing 73–96% of the total counts. Nodularia spumigena contributed 2.2–11.0% of the total counts and was abundant in observable numbers only at the northernmost station CS_LL19. Apart from that, the abundances of N. spumigena did not differ between the southern and northern parts of the transect (Table 1).
The Chla concentrations correlated positively with PC and PN along the transect but not with PP (Table 4). The average PC concentrations were 33.6 ± 7.3 μM in the southern and 44.0 ± 7.8 μM in the northern part of the transect. PN concentrations of 5.3 ± 0.7 and 6.8 ± 0.9 μM were observed in the southern and the northern parts, respectively (Table 1). The PC and PN concentrations correlated with each other (Table 4), resulting in similar C:N ratios of 6.3 ± 0.7 and 6.6 ± 1.2 in both parts of the transect (Table 1). The average C:P ratios of the northern stations were significantly higher than those of the southern stations (178 ± 26 and 145 ± 29, respectively) and reached values > 200 at three stations in the 1 m but not in 10 m depth.
Uptake Rates of DIP, dATP-P, and dDNA-P
The turnover times of DIP (TtDIP), dATP-P (TtdATP-P), and dDNA-P (TtdDNA-P) were the longest at the southern stations of the transect (Figures 5A–C and Table 6). At these stations, the TtDIP and TtdATP-P were comparable (17 and 15 h, respectively), while the TtdDNA-P was as high as 107 h. At the northern stations, the turnover times differed from each other (DIP < dATP-P < dDNA-P) (ANOVA: F = 51.14; p < 0.0001), and the turnover times of dDNA-P (average 22 h) were considerably shorter than those at the southern stations (Table 3).
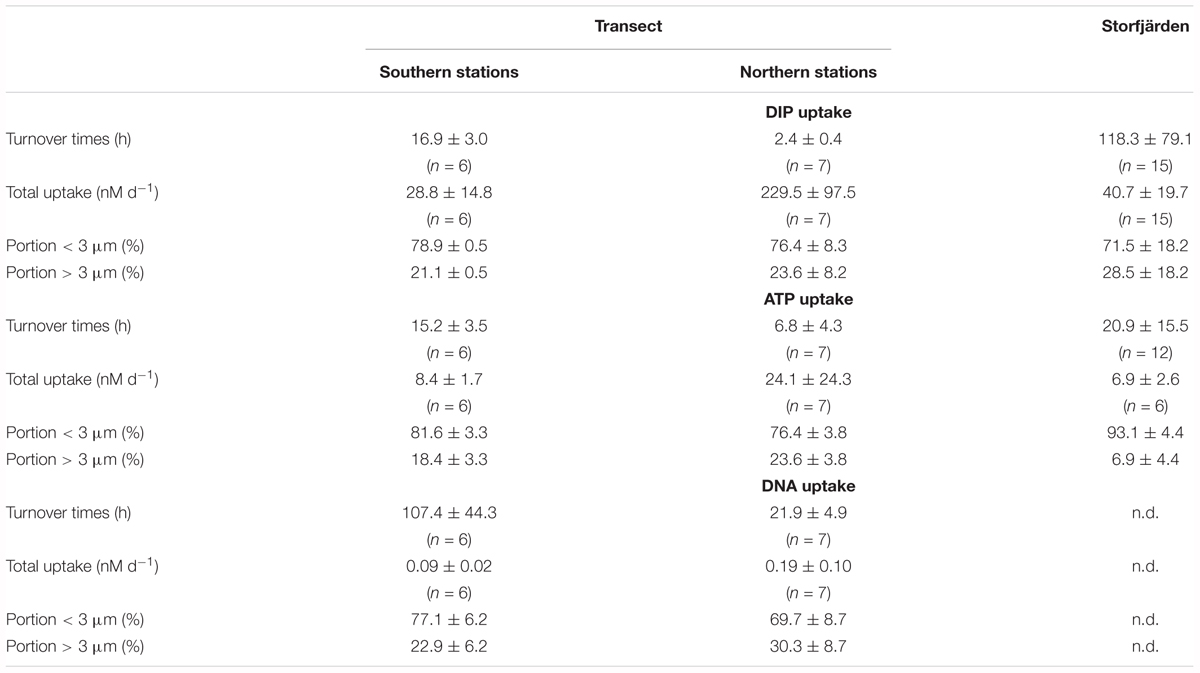
TABLE 6. Mean values and standard deviation of turnover times, uptake rates of DIP, dATP-P, and dDNA-P and its distribution into size fractions.
The uptake rates show that DIP was always the preferred P source, followed by dATP-P (Figures 5F–H and Table 6). The uptake rates differed between the two parts of the transect by a factor of 8 for DIP and by a factor of approximately 3 and 2 for dATP-P and dDNA-P, respectively; the differences were significant in the t-test for DIP, but not for dATP-P and dDNA-P (Table 3). The P uptake from DIP, dATP-P, and dDNA-P resulted in a total P uptake of 37.3 ± 14.4 nM d-1 in the southern part and 248.5 ± 118.2 nM d-1 which is about 30 and 11%, respectively, more than from DIP alone. The contribution of dDNA-P was generally small (maximum 0.38 nM d-1).
More than two-thirds of the DIP and each DOP compound were taken up by the size-fraction < 3 μm (Table 6).
Multiple regression analysis including subgroup analysis (Table 7) revealed that the sum of the temperature, DOP, DIP, Chla, PC, PN, PP, C:N ratio, and C:P ratio could explain 95.4–97.7% of the variation. Among these parameters, the temperature and DIP concentrations had the strongest effects on the dATP-P and dDNA-P-uptake rates (Supplementary Figure 2). The effects of the autotrophic picoplankton and heterotrophic bacteria seemed to be of minor importance.
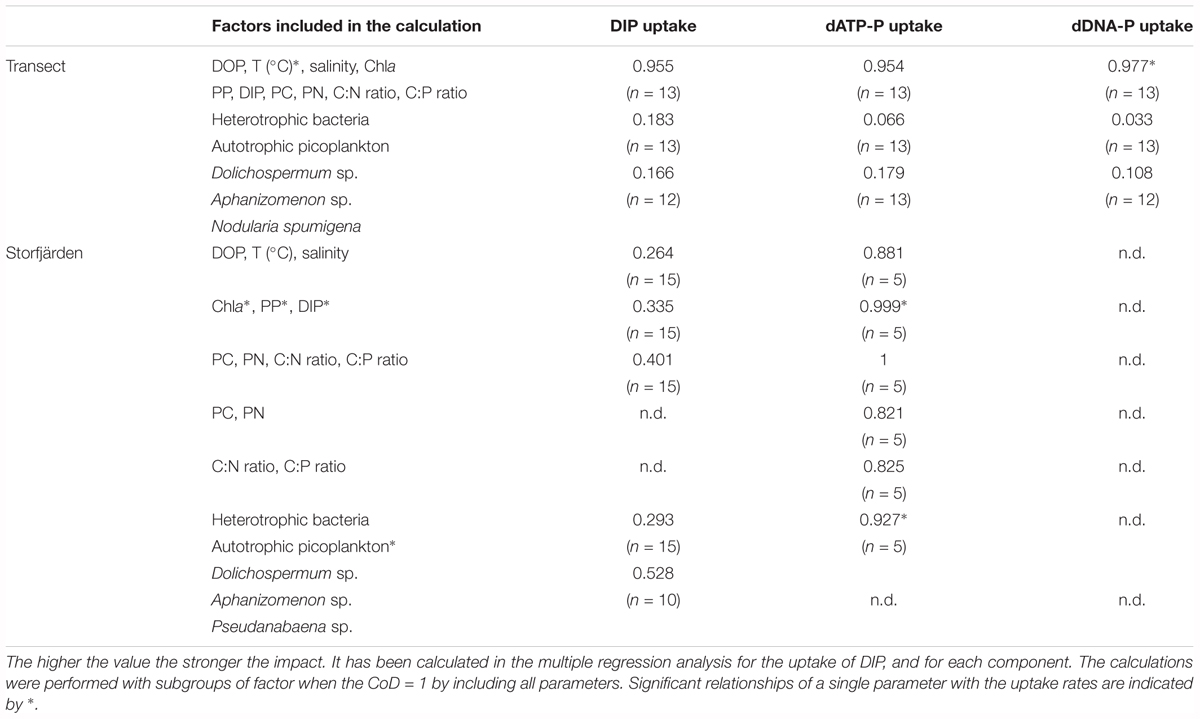
TABLE 7. Coefficents of determination (CoD) to describe the impact of parameter groups on uptake rates of DIP, dATP-P, and dDNA-P.
Time Series Measurements in 2012
DOP Concentration and Compounds
Dissolved organic phosphorus concentrations of 0.26 ± 0.03 μM in the Eastern Gotland Basin were relatively consistent during the investigation period (Figure 2B) and contributed to ∼84% of the DP (Table 2). The DIP concentrations were 0.06–0.08 μM during the first 6 days and 0.02–0.04 μM after day 6. The average DIP concentration for the whole period was 0.04 ± 0.03 μM (Figure 2B). DOP was positively correlated with temperature and inversely correlated with DIP (Table 4). In the multiple regression analysis, 85% of the DOP variation could be explained by the sum of Chla, DIP, PP, PC, PN, and the C:N and C:P ratios and ∼19% could be explained by the filamentous cyanobacteria (Table 5). The highest t-value was obtained for DIP, indicating that DIP might have the strongest effect.
Together, the three DOP compounds (dATP-P, dPL-P, and dDNA-P) constituted just 2.4 ± 0.9% of the total DOP pool (Table 2). The dATP-P concentrations ranged between 2.5 and 8.4 nM (mean: 4.3 ± 1.7 nM) (Figure 3A and Table 2). The dPL-P concentrations ranged between 0.4 and 8.0 nM (mean 1.9 ± 1.9 nM), but the values of 5.5 and 8.0 nM were outside the 95 percentile and could be outliers (Figure 3B). The average dDNA-P concentrations of 0.12 ± 0.04 nM (Figure 3C) were again the lowest among the three investigated compounds.
The temporal dynamics of the three compounds differed (Figure 4). Nevertheless, the Spearman rank correlation indicated a similarity between dATP-P and dDNA-P concentrations (Table 4), as both compounds had high concentrations at the beginning and at the end of the investigation period. The multiple regression (Table 5 and Supplementary Figure 1) analysis again showed that no single parameter alone could explain the variation in each of the three components. All parameters together could explain 98% of the variation in dATP-P, 79% of the variation in dPL-P and 92% of the variation in dDNA-P, and 78–81% of the variations could be explained by the sum of the hydrographical and biological parameters (Table 5). The variation in the t-values (Supplementary Figure 1) was within a small range, so it was difficult to identify a dominating factor.
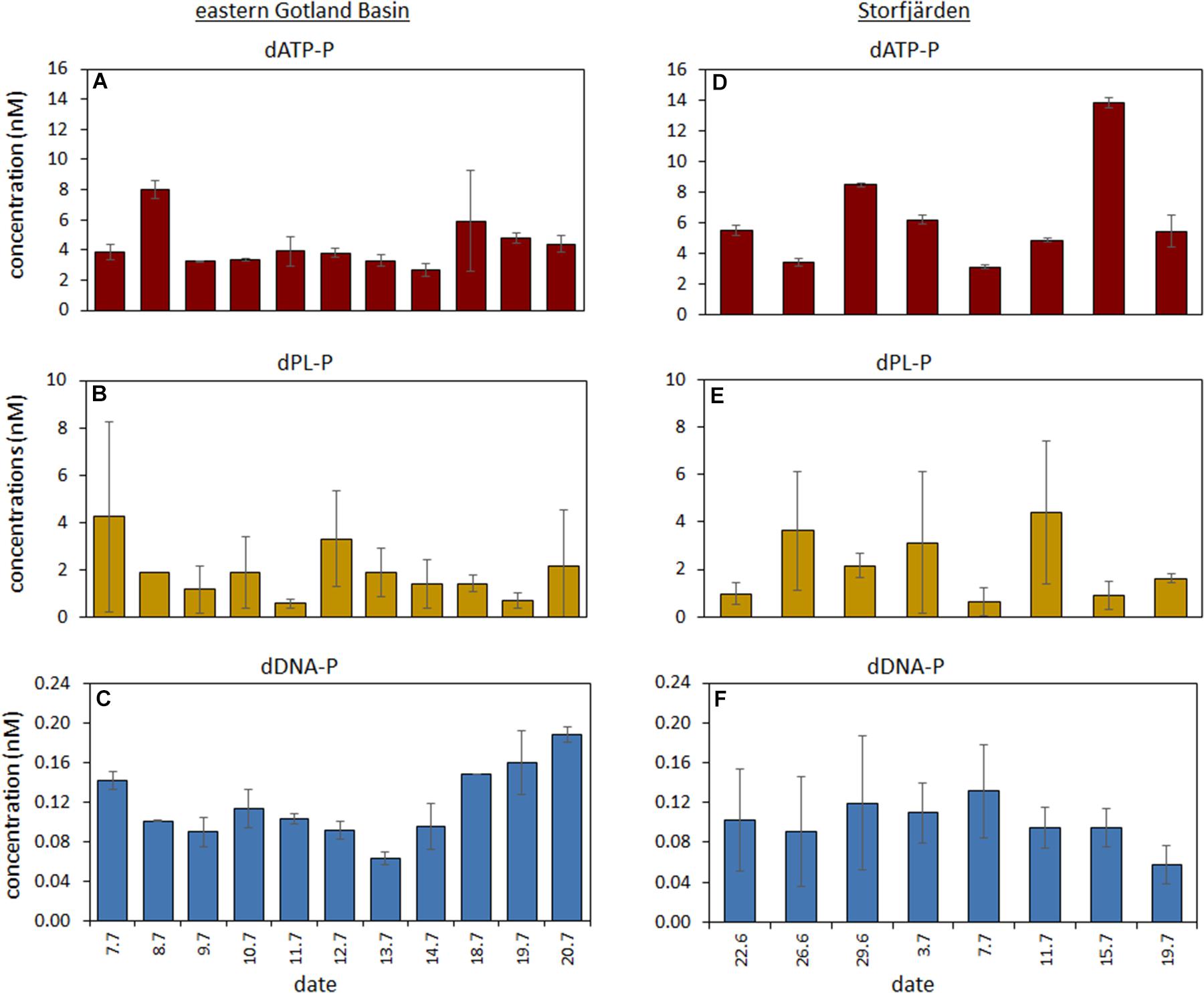
FIGURE 4. Temporal variation of the concentrations of the DOP compounds during the time series in the eastern Gotland Basin (A–C) and in the Storfjärden (D–F). Mean values of the 1 and 10 m are used for the eastern GB and for a depth integrated sample in the Storfjärden.
The averaged TP concentrations (0.61 ± 0.06 μM) sampled in Storfjärden exceeded those measured in parallel in the eastern GB (0.50 ± 0.04 μM) (Table 2). The average DIP concentration of 0.16 ± 0.07 μM accounted for 39% of DP, whereas the average DOP concentration of 0.24 ± 0.03 μM accounted for 61% of DP. DOP increased from 0.18 μM on day 0 to 0.29 μM on day 11 and thereafter decreased to 0.11 μM (Figure 2C), thus correlating inversely with DIP (Table 4). Approximately 93% of the DOP variation could be explained by the sum of all 11 parameters, including temperature, salinity, DIP, Chla, particulate matter and its ratios, in the multiple regression analysis and was most strongly influenced by temperature, heterotrophic bacteria and PC (Table 5).
The dATP-P concentration of 6.4 ± 3.4 nM was the highest among the three measured compounds and accounted for 2.2 ± 1.4% of DOP (Table 2). The dPL-P and dDNA-P concentrations of 2.2 ± 1.4 and 0.10 ± 0.02 nM, respectively, accounted for 0.9 ± 0.6 and 0.04 ± 0.01% of DOP, respectively. No correlation was detected between the DOP compounds and any other parameter. The multiple regression analysis showed strong influences of filamentous cyanobacteria, especially on variations in dATP-P and dDNA-P (Table 5). However, the variations in the DOP components can also be explained by other parameter combinations, as evident by the sum of temperature, salinity, Chla, DIP, and PP explaining nearly 100% of the dATP-P variation, 46% of the dPL-P variation and 69% of the dDNA-P variation. Within this parameter set, the t-values (Supplementary Figure 1) indicated that PP and DIP had the strongest influences on the variation in dATP-P, whereas no single parameter had a greater influence than the others for dPL-P and dDNA-P.
Phytoplankton
The Chla concentrations in Storfjärden were comparable to those in the eastern GB (Table 1). However, the phytoplankton composition differed between both stations due to the occurrence of filamentous cyanobacteria. The species Dolichospermum sp., Aphanizomenon sp. were abundant in both regions of investigation, but their abundances differed. N. spumigena occurred only in low abundances in the eastern GB, while Pseudanabaena sp. was found only in Storfjärden where it was the predominant filamentous species (Table 1).
The Chla concentrations in Storfjärden varied synchronously with changes in temperature, salinity and DIP, as indicated by significant Spearman rank correlations (Table 4); the PC and PN concentrations (28.5 ± 5.9 and 4.1 ± 0.8 μM, respectively) did not correlate with Chla. The C:P ratios ranged from 91 to 128, except for during the first 8 days, when the C:P ratios were between 146 and 190 (Table 1).
Similar PC and PN concentrations and a similar range of C:P ratios were observed in the eastern GB (Table 1). Thus, both stations seemed to have a comparable particulate matter biogeochemistry.
Uptake Rates of DIP, dATP-P, and dDNA-P
In Storfjärden, the average TtDIP was 118 ± 79 h (Figure 5D and Table 6) and decreased to approximately 30 h during 2 days in the middle of the time series when DIP concentrations decreased to 0.06 μM (Figure 2A); the TtdATP-P of 21 ± 16 h (Figure 5E) was significantly shorter than that of DIP. Approximately 50% DIP was uptaken by the size fraction < 3 μm. During the other times, on average, 71.5% DIP was uptaken the size fraction < 3 μm at a rate of 40.7 ± 19.7 nM d-1 (Table 6 and Figure 5I). This size fraction realized 93.1 ± 4.4% of the dATP-P uptake (Table 6 and Figure 5J). Both the DIP and dATP-P uptake (6.9 ± 2.6 nM d-1) together resulted in a total uptake of 23.7–59.0 nM d-1 P which averaged 17% more than by the use of DIP alone.
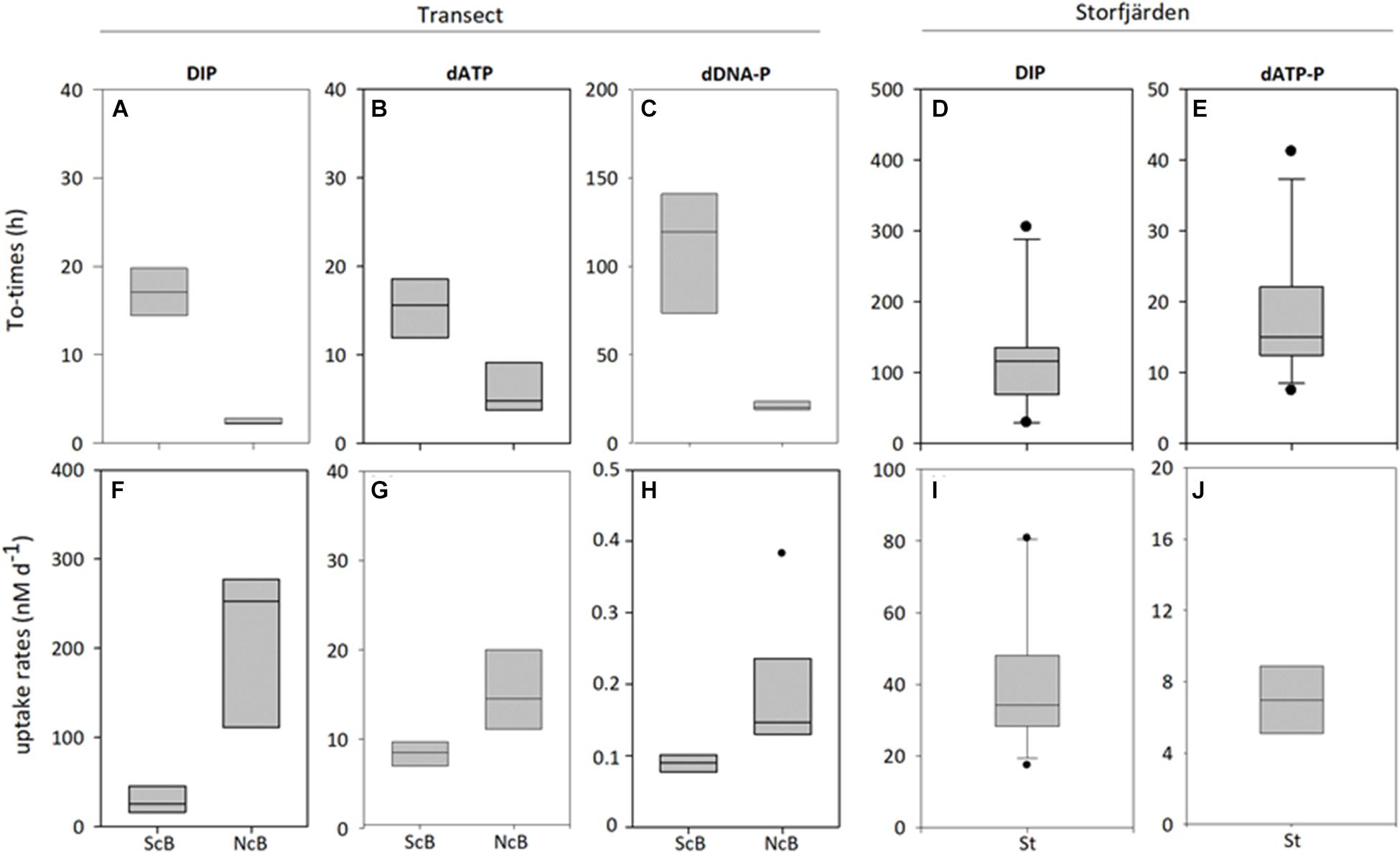
FIGURE 5. Turnover times (A–E) and uptake rates (F–J) of DIP, dATP-P and dDNA-P at the two parts of the transect (southern part -ScB; northern part-NcB, and in the Storfjärden (St). The line inside the box represents the median value; the box is edged by the 25 and 75% percentile and the error bars indicate the 10 and 90% percentile.
In the multiple regression analysis (Table 7) for the uptake rates in Storfjärden, groups of 2–4 parameters had differing impacts on the uptake of DIP and dATP-P. Chla, PP, and DIP seemed to have a strong influence (∼100%) on the uptake of dATP-P, but the influence of this group on the uptake of DIP was only 33.5%. In addition, other parameter combinations, e.g., DOP, temperature, and salinity (88%) or bacteria and autotrophic picoplankton (99%) could explain the uptake of dATP-P to a high degree (88%).
Discussion
Concentrations of DOP and DOP Compounds
The overall mean DOP concentration of 0.23 μM detected in the Baltic Sea has also been reported in other oceanic regions, including coastal waters (Björkman and Karl, 2003; Lomas et al., 2010; Karl and Björkman, 2015), suggesting that DOP is a relatively constant pool in marine ecosystems. However, DOP contains a number of different compounds that are unknown, how significant they are and how they behave. Knowledge of the individual components is necessary to understand the marine DOP pool. This study provides warranted insight into the internal dynamic of the DOP pool in the Baltic Sea.
Our study shows that DOP compounds, namely, dATP, dDNA and dPL, comprised a small share of the total DOP pool in the Baltic Sea. Nevertheless, they made a significant contribution to the nutrition of microorganisms, with differences in the contribution of dATP-P and dDNA-P. The individual components were not obviously regulated by single factors but were rather controlled by the simultaneous action of sets of factors.
The mean dATP-P concentrations of 4.3–6.4 nM in our study are comparable with those reported for the productive Brainsfield Strait (Antarctica) (Nawrocki and Karl, 1989), whereas they are an order of magnitude higher than those reported for the oligotrophic North Pacific (0.2–0.8 nM) (Karl and Björkman, 2015) where higher concentrations in summer than in winter occurred (Björkman and Karl, 2005). Thus, it seems that the dATP-P concentrations increase when the productivity of the water is higher, evident in the seasonal variation as well as in ecosystems of different eutrophication levels. The multiple regression analysis showed that the combination of several parameters could explain the distribution of all three compounds to a high degree in the Baltic Sea. The sum of temperature, DIP, Chla, particulate matter and their ratios and the abundance of heterotrophic bacteria (Table 4) seemed to control the dATP-P concentrations in all three regions. Within this parameter group, Chla, heterotrophic bacteria and the C:N ratio had the strongest effect on the dATP-P concentrations along the transect but not in the eastern GB and Storfjärden. In Storfjärden, DIP and PP were chief among these factors. Here, filamentous cyanobacteria had much stronger effects on dATP-P (Table 5), which contrasted among species. Dolichospermum sp. had a positive relation with dATP-P, while Aphanizomenon sp. and Pseudanabaena sp. had a negative relation with dATP-P. This different relation of the individual cyanobacteria species to dATP-P was also observed for DOP compounds dPL-P and dDNA-P especially in the time series studies (Supplementary Figure 1) where it can be assumed that the plankton community remained relatively unchanged. It indicates that the single cyanobacteria species obviously behaved differently in its impact on the DOP compounds. We observed a negative relation between cyanobacteria and the C:N ratio along the transect. This could mean that nitrogen fixation (reducing the C:N ratios) and dATP-P concentrations are related to each other. The dATP-P concentration seemed to be higher when the nitrogen fixation was high. So it may be that the release of fixed nitrogen (Gilbert and Bronk, 1994; Mulholland et al., 2004; Wannicke et al., 2009) was accompanied by dATP-P release. The dATP-P release interacted with its utilization by heterotrophic bacteria, as deduced from their negative relationship to dATP-P. However, such relationships were not observed in the eastern GB. We have no explanation for this observation. Under marginal nitrogen fixation at sufficient nitrate concentrations in Storfjärden (Paul et al., 2016), the abundance of the cyanobacteria became more significant as a regulatory factor. Release of dATP-P from growing cyanobacteria seems to be possible as found for other organisms. So, diatoms that form blooms in spring, have been found to release dATP-P (Riemann (1979). In culture experiments, Mempin et al. (2013) found that heterotrophic bacteria were able to release dATP during growth. Alternatively, Nawrocki and Karl (1989) suggested grazing by micro and macrozooplankton is the dominant dATP source in Antarctic waters. Viral lysis is general accepted to be a source for dissolved organic matter (DOM) (Tuomi and Kuuppo, 1999; Middelboe and Jorgensen, 2006; Anderson et al., 2012) including DOP. To assess the significance of viral lysis on dATP-release we made the assumption that virus induced mortality is 12–18% (Weinbauer et al., 2003) and that the death cells release their total ATP content of 0.5–6.9 × 10-9 μg cell-1 (3–40 amol P cell-1) (Hamilton and Holm-Hansen, 1967). Thus bacteria abundances of 4.2 × 109 cells L-1 estimated in the central Baltic Sea (Table 2) could release about 2–25 nM P. It becomes evident that viral lysis can significantly contribute to the release of dATP-P in the Baltic Sea although this value is overestimated, since it is unlikely that the entire ATP is released simultaneously and parts of the released dATP can be consumed immediately as found for DOM (Anderson et al., 2012).
The concentrations of dPL-P (1.7–6.8 nM) were similar to those observed in other semi-enclosed systems, such as in Tokyo Bay (Suzumura and Ingall, 2004). Within this concentration range, differences were observed between studies performed along the transect in 2011 and in the eastern GB in 2012, despite similar hydrographical and biogeochemical conditions. Both investigation sites were located in the central Baltic Sea and had similar low DIP concentrations (0–0.06 μM along the transect, 0–0.08 μM in the eastern GB); therefore, it can be assumed that DOP is used as a P source by phyto- and bacterio-plankton. The only significant difference between the two studies was the abundance of filamentous cyanobacteria (Table 1). The negative relationship between dPL-P and the species of filamentous cyanobacteria in the multiple regression (Table 5) and in the PCA analysis (Supplementary Figure 3) indicated that the cyanobacteria were possibly consumers of dPL-P in our study. Filamentous cyanobacteria are known to express a high amount of alkaline phosphatase activity under DIP-depleted (<0.05 μM) conditions (Endres et al., 2013; Unger et al., 2013) and can constitute the major fraction (42%) of the total activity in summer when filamentous cyanobacteria occurred in high abundances while the contribution of bacterial APA is rather low (3% in the size fraction <0.8 μm) (Nausch et al., 2004). In a certain proportion of phospholipids, P is coupled via ester linkage to the lipid part of the molecule. This portion can be degraded via phosphodiesterase and phosphatase (Liu et al., 1998) and is therefore bioavailable. Therefore, it is conceivable that the higher abundance of cyanobacteria was related to the lower dPL-P concentration in the eastern GB in 2012. The eastern GB and Storfjärden investigated in the same year, had similar dPL-P concentrations (Figures 3, 4) despite their differences in DIP concentrations. This indicates that DIP availability did not influence the level of dPL-P concentrations. It can be deduced from the multiple regression analysis that the combination of hydrographic and stock parameters obviously had a stronger effect on dPL-P than the filamentous cyanobacteria. In the PCA analysis, no factor was shown to have a significant influence on dPL-P concentrations (Supplementary Figure 4). Goutx et al. (2009) assumed eukaryotic picophytoplankton and zooplankton were sources of dPL in the Mediterranean Sea. In Storfjärden, we could not find any relationship between eukaryotic nano-phytoplankton and the dPL-P concentrations (data not shown). Zooplankton were not considered in our study.
Using the method of Karl and Bailiff (1989) for determination the Baltic Sea dDNA-P concentrations of 0.05–0.12 nM were extremely low compared to other oceanic regions, e.g., Karl and Bailiff (1989), and Weinbauer et al. (1995). To better compare our results with previous results, we applied the factor 2.06 nmol P per μg DNA (Trinkler, 2009) to convert the data reported by these authors into [nM] dDNA-P. The dDNA-P concentrations were then 2.1–43.4 nM in the coastal regions of Hawaii (Karl and Bailiff, 1989), 4–31 nM in Tampa Bay (Gulf of Mexico), approximately 2.5 nM in the surface layer of the HOT station, and 21–26 nM in the Northern Adriatic Sea (Weinbauer et al., 1995). Thus, marine eutrophic waters seemingly have higher dDNA-P concentrations than oligotrophic regions. Furthermore, phytoplankton seems the main regulator of seasonal and diel variations (Paul et al., 1988). In contrast to the dPL-P concentrations, higher dDNA-P concentrations occurred in the Storfjärden and the eastern GB than in the transect (Table 2) indicating that probably the cyanobacteria had a higher release than uptake of dDNA-P inversely to dPL-P. This interpretation is not supported by earlier observations in experiments with N. spumigena, namely, that no release of dDNA-P after uptake occurred in contrast to dATP-P and dPL-P (Unger et al., 2013). Note however that in our field experiments, N. spumigena occurred only in marginal abundances and Aphanizomenon sp., was the dominant species. It cannot be excluded that Aphanizomenon sp. uses different DOP compounds than N. spumigena.
Our results confirm that the regulation of DOP constituents is very complex as they are constantly subject to uptake and release. The concentrations seem to be predominantly affected by the abundance or nitrogen fixing activity of filamentous cyanobacteria. Single species can have contrasting effects that can interact with heterotrophic bacteria as evident for dATP-P under DIP-depleted conditions.
Uptake and Turnover Times of DIP and DOP Compounds
The mean DIP uptake rates of 28.8–229.5 nM d-1 measured in the Baltic Sea in this study are comparable to those reported for the Atlantic Ocean (Casey et al., 2009; Lomas et al., 2010; Sohm and Capone, 2010), but with higher minimum values. Calculated from depth-integrated data, DIP uptake rates of 1.4–8.2 nM d-1 in the oligotrophic subtropical North and Southeastern Pacific (Björkman et al., 2012; Duhamel et al., 2017) were lower than those in the Baltic Sea. The mean dATP-P uptake rates in the Baltic Sea (6.9–24.11 nM d-1) exceeded those estimated in the Pacific and Atlantic Ocean (Casey et al., 2009; Lomas et al., 2010; Björkman et al., 2018) by a factor of 10–220, probably due to the higher plankton production in the Baltic Sea. Nausch et al. (2004) reported that >90% of the hydrolysed P were immediately taken up in summer while only about 20% were used in spring (Nausch et al., 2004). Thus, the importance of dATP-P for the nutrition of phyto- and bacterio-plankton in summer might be high in the Baltic Sea, while the P-uptake from dDNA might be relatively low (about 100 times lower than that of dATP-P). However, for the DNA uptake in the open ocean there is little information available. Phyto- and bacterio-plankton develop probably a higher affinity to all three forms (dATP-P, dDNA-P, and DIP) simultaneously when DIP is depleted (Lovdal et al., 2007); it can explain why the uptake rates of dATP-P and dDNA-P along the transect in our study showed the same trend as the uptake of DIP. Hence, the organisms seemingly developed a strategy to acquire P from all or from many of the DOP compounds; the efficiency of P delivery varies depending on the bioavailability. According to the multiple regression analysis (Table 7), all three uptake rates along the transect were regulated by a combination of hydrographical and biological factors. Among these factors, the temperature and DIP concentration had the strongest effects (Table 7). Temperature and DIP can directly affect the uptake rates, while the influence of other factors (e.g., C:P ratios) is indirectly via the P deficiency of organisms. Lovdal et al. (2007) estimated that, under P deficiency, the 0.2–1 μm size fraction is the first to develop a higher affinity for DIP, dATP-P, and dDNA-P. Subsequently, as the P limitation increases, the dATP-P and dDNA-P affinity of the larger size fraction increases too.
The use of dATP-P as a P source persists, even under DIP-replete conditions in Storfjärden. However, within the DIP range of 0.06–0.32 μM, the uptake of dATP-P decreased with increasing DIP concentrations (negative t-values in Supplementary Figure 2) indicating the lower importance of the dATP-P for the plankton nutrition when more DIP is available. PP and Chla were also strongly related with the dATP uptake, negatively with Chla and positively with PP and with autotrophic picoplankton (Table 7). It could be interpreted that more dATP-P can be released at increasing cellular P and that filamentous cyanobacteria which might account for a high Chla proportion also uses organic P in parallel with DIP under DIP repleted conditions. The simultaneous positive and negative relation of the individual parameters to the dATP-P uptake shows the complexity of the regulation of a single DOP components. The size fractionation of the uptake rates of dATP-P and dDNA-P (Table 6) along the transect and the Storfjärden (Table 6) reflects the findings by Lovdal et al. (2007). The proportion of dATP-P and dDNA-P taken up by the <3 and >3 μm size fractions shifted to the >3 μm size fraction under P-limitation. This trend was not very strong. Further studies should divide phytoplankton into several other size classes to elucidate differences.
Turnover times of DIP (TtDIP) are often used as indicators of the P deficiency or the P demand of organisms (Sohm and Capone, 2010), especially when DIP concentrations are near the detection limit (Moutin et al., 2008). In our study, we observed a relationship between the TtDIP and DIP concentrations in Storfjärden, where a DIP gradient occurred. No relationship was found along the transect, where all DIP concentrations ranged between 0.01 and 0.06 μM. These observations are comparable with findings of Tanaka et al. (2006), who found a linear relationship only for DIP concentrations > 0.1 μM. In previous studies performed in the Baltic Sea the TtDIP shortened from 179 to 1 h when the DIP concentrations declined from 0.1 to 0.01 μM (Nausch et al., 2004). It seems that DIP concentrations have a high impact on turnover times which is visible when a gradient in concentrations (Supplementary Figure 6 and Figure 2C) occurs. At low DIP concentrations (Figure 2A) increases in biomass (Sohm and Capone, 2010) or the induction of a higher P demand, e.g., by a nitrogen and carbon surplus (Tanaka et al., 2006; Lovdal et al., 2007), could induce shortening of the DIP turnover times. Along the transect, small but significant increases in the temperature, biomass parameters (Chla, PC, PN) and particulate C:P ratios (Table 3) were monitored; these factors could be the driving factors underlying shorter TtDIP in the northern stations compared to the southern stations and an indication for a strengthening of P limitation. Van Den Broeck et al. (2004) reported that “long DIP turnover times are a result of enhanced concentrations and slow assimilation rates, while short turnover times are due to low DIP concentrations and more rapid fluxes.” We can now specify that turnover times are related to the ratio between concentrations of DIP and DOP compounds and respective uptake rates, and vice versa, the uptake rates are related to the ratio between turnover times and concentrations (Supplementary Figure 5). These findings can also be applied to the uptake of dATP-P and dDNA-P.
In the Baltic Sea, the dATP-P and dDNA-P pools showed longer turnover times when concentrations were higher, as is the case in other regions of the ocean (Nawrocki and Karl, 1989; Björkman and Karl, 2005; Björkman et al., 2012) and longer turnover times were associated with lower uptake rates (Table 6). That is not the case with DIP when the Störfjärden and the transect were compared. The mean DIP uptake rate in Storfjärden was lower than that in the northern part congruent with the longer turnover times. However, the DIP uptake in the Storfjärden exceeded the uptake in the southern part despite sevenfold longer turnover times (Table 6). So it seems that the long DIP turnover in the Storfjärden were compensated by the DIP concentrations to a certain degree resulting in higher uptake rates than expected from the turnover times.
One question that must be answered is whether differences in substrate concentrations caused the differences in the turnover times of DIP, dATP-P, and dDNA-P in our study. The shorter TtdATP-P than TtDIP in Storfjärden seems to be due to the relatively low dATP-concentrations in this area. A similar finding was reported for the Southeast subtropical Pacific Ocean (Duhamel et al., 2017). However, this relationship between substrates and turnover times was not observed along the transect. Possibly, the affinity of organisms to DIP (Lovdal et al., 2007) increased more than the affinity of organisms to dATP-P under P-depleted conditions such as those observed.
The relationship between turnover times and P concentrations cannot be applied when dDNA-P and dATP-P are considered. The TtdDNA-P was longer than the TtdATP-P despite much lower DNA concentrations. The reason for this can be found in the complex DNA structure requires a set of different enzymes for degradation and P release. Siuda and Güde (1996) therefore assumed enzymatic hydrolysis is the rate limiting step in DNA conversion. Lovdal et al. (2007), however, considered the diffusive transport of DNA to the cells as the limiting step for the lower intake. Our investigations show that the relation between concentrations and turnover times is valid only for a limiting number of situations; many other factors can influence the turnover times.
The TtDIP values in the Baltic Sea were within the range of those reported in other regions of the ocean, e.g., the North Subtropical Pacific Gyre, Southeast Pacific Gyre (Moutin et al., 2008), and the tropical North Atlantic (Sohm and Capone, 2010). TtdATP-P values of 1–2 days were reported for the HOT-station in the North Pacific Subtropical Gyre (Björkman and Karl, 2005). The Storfjärden characterized by upwelling of cold water from below the thermocline had similar TtdATP-P as observed in the eastern GB in spring 2001 (Nausch et al., 2004). TtdATP-P of about 1 day or longer do not represent a typical summer situation. In summer, the TtdATP-P can be less than 1 day, which emphasizes the importance of dATP-P for the nutrition of organisms in the Baltic Sea in this season.
Conclusion
Our study shows that the dissolved DOP compounds (dATP-P, dPL-P, dDNA-P) are significant P sources for the nutrition of phyto- and bacterio-plankton, despite their low concentrations. DIP remained the dominant P source in the Baltic Sea under both DIP depleted and replete conditions. The variations in the dATP-P, dPL-P, and dDNA-P concentrations and how they are influenced by different combinations of underlying factors imply that these DOP compounds are regulated independently of each other. The observed filamentous cyanobacteria seemed to possess regulatory functions. However, the DOP functioning could not be clarified completely in this study due to the complexity of interactions. Therefore, further detailed knowledge of the DOP pool, including the fraction that was not identified in our study, is necessary to explain its ecological function in marine ecosystems.
Author Contributions
All authors listed have made a substantial, direct and intellectual contribution to the work, and approved it for publication.
Funding
The studies in both years were funded by the BMBF project BIOACID II (FKZ 03F06550). The publication of this article was funded by the Open Access Fund of the Leibniz Association.
Conflict of Interest Statement
The authors declare that the research was conducted in the absence of any commercial or financial relationships that could be construed as a potential conflict of interest.
The handling Editor declared a shared affiliation, though no other collaboration, with several of the authors EPA, LTB, UR, and AS at time of review.
Acknowledgments
We would like to thank the staff of the RV “Elisabeth-Mann Borgese” for the support during the cruise, cruise leader Maren Voss for the cruise management and Birgit Sadkowiak for the analysis of inorganic nutrients on board. In particular, we would like to thank Pia Steinrücken for sampling during the METEOR-cruise.
We are grateful to the KOSMOS team for their invaluable help with the logistics and maintenance of the investigations in Storfjärden. In particular, we sincerely thank Andrea Ludwig for organizing and coordinating the campaign. We appreciate the assistance of Jehane Ouriqua in the inorganic nutrient analysis and the assistance of many other participants who carried out the samplings. Hans-Peter Grossart and Thomas Hornick are owed our special thanks for providing the heterotrophic bacteria data. Finally, we thank Jana Woelk for analyzing the phosphorus samples in the Leibniz Institute for Baltic Sea Research.
We would also like to acknowledge the staff of the Tvärminne Zoological Station for their hospitality and support, for allowing us to use the experimental facilities, and for providing CTD data for the summers of 2008–2011. The study was performed in the framework of the BMBF project BIOACID (FKZ 03F06550) and the Leibniz Science Campus Phosphorus Research Rostock.
Supplementary Material
The Supplementary Material for this article can be found online at: https://www.frontiersin.org/articles/10.3389/fmars.2018.00386/full#supplementary-material
References
Ammerman, J. W. (1993). “Microbial cycling of inorganic and organic phosphorus in the water column,” in Handbook of Methods in Aquatic Microbial Ecology, eds P. F. Kemp, B. F. Sherr, E. B. Sherr, and J. J. Cole (Boca Raton, FL: Lewis Publishers), 649–660.
Anderson, R., Winter, C., and Jurgens, K. (2012). Protist grazing and viral lysis as prokaryotic mortality factors at Baltic Sea oxic-anoxic interfaces. Mar. Ecol. Prog. Ser. 467, 1–14. doi: 10.3354/meps10001
Benitez-Nelson, C. R. (2000). The biogeochemical cycling of phosphorus in marine systems. Earth Sci. Rev. 51, 109–135. doi: 10.1016/S0012-8252(00)00018-10
Björkman, K., Duhamel, S., and Karl, D. M. (2012). Microbial group specific uptake kinetics of inorganic phosphate and adenosine-5 ’-triphosphate (ATP) in the North Pacific Subtropical Gyre. Front. Microbiol. 3:189. doi: 10.3389/fmicb.2012.00189
Björkman, K., and Karl, D. M. (1994). Bioavailability of inorganic and organic phosphorus compounds to natural assemlages of microorganisms in Hawaiian coastal waters. Mar. Ecol. Prog. Ser. 111, 265–273. doi: 10.3354/meps111265
Björkman, K. M., Duhamel, S., Church, M. J., and Karl, D. M. (2018). Spatial and temporal dynamics of inorganic phosphate and adenosine-5’-triphosphate in the North Pacific Ocean. Front. Mar. Sci. 5:235. doi: 10.3389/fmars.2018.00235
Björkman, K. M., and Karl, D. M. (2001). A novel method for the measurement of dissolved adenosine and guanosine triphosphate in aquatic habitats: applications to marine microbial ecology. J. Microbiol. Methods 47, 159–167. doi: 10.1016/S0167-7012(01)00301-303
Björkman, K. M., and Karl, D. M. (2003). Bioavailability of dissolved organic phosphorus in the euphotic zone at station ALOHA, North Pacific Subtropical Gyre. Limnol. Oceanogr. 48, 1049–1057. doi: 10.4319/lo.2003.48.3.1049
Björkman, K. M., and Karl, D. M. (2005). Presence of dissolved nucleotides in the North Pacific Subtropical Gyre and their role in cycling of dissolved organic phosphorus. Aquat. Microb. Ecol. 39, 193–203. doi: 10.3354/ame039193
Casey, J. R., Lomas, M. W., Michelou, V. K., Dyhrman, S. T., Orchard, E. D., Ammerman, J. W., et al. (2009). Phytoplankton taxon-specific orthophosphate (Pi) and ATP utilization in the western subtropical North Atlantic. Aquat. Microb. Ecol. 58, 31–44. doi: 10.3354/Ame01348
Cavender-Bares, K. K., Karl, D. M., and Chisholm, S. W. (2001). Nutrient gradients in the western North Atlantic Ocean: relationship to microbial community structure and comparison to patterns in the Pacific Ocean. Deep Sea Res. Oceanogr. Res. Pap. 48, 2373–2395. doi: 10.1016/S0967-0637(01)00027-29
Clark, L. L., Ingall, E. D., and Benner, R. (1999). Marine organic phosphorus cycling: novel insights from nuclear magnetic resonance. Am. J. Sci. 299, 724–737. doi: 10.2475/ajs.299.7-9.724
Duhamel, S., Björkman, K. M., Repeta, D. J., and Karl, D. M. (2017). Phosphorus dynamics in biogeochemically distinct regions of the southeast subtropical Pacific Ocean. Prog. Oceanogr. 151, 261–274. doi: 10.1016/j.pocean.2016.12.007
Dyhrman, S. T., Chappell, P. D., Haley, S. T., Moffett, J. W., Orchard, E. D., Waterbury, J. B., et al. (2006). Phosphonate utilization by the globally important marine diazotroph Trichodesmium. Nature 439, 68–71. doi: 10.1038/nature04203
Endres, S., Unger, J., Wannicke, N., Nausch, M., Voss, M., and Engel, A. (2013). Response of Nodularia spumigena to pCO(2) - part 2: exudation and extracellular enzyme activities. Biogeosciences 10, 567–582. doi: 10.5194/bg-10-567-2013
Feingersch, R., Philosof, A., Mejuch, T., Glaser, F., Alalouf, O., Shoham, Y., et al. (2012). Potential for phosphite and phosphonate utilization by Prochlorococcus. ISME J. 6, 827–834. doi: 10.1038/ismej.2011.149
Gasol, J. M., and Del Giorgio, P. A. (2000). Using flow cytometry for counting natural planktonic bacteria and understanding the structure of planktonic bacterial communities. Sci. Mar. 64, 197–224. doi: 10.3989/scimar.2000.64n2197
Gilbert, J. M., and Bronk, D. A. (1994). Release of dissolved organic nitrogen by marine diazotrophoccyanobacteria, Trichodesmium spp. Appl. Environ. Microbiol. 60, 3996–4000.
Goutx, M., Guigue, C., Aritio, D., Ghiglione, J. F., Pujo-Pay, M., Raybaud, V., et al. (2009). Short term summer to autumn variability of dissolved lipid classes in the Ligurian sea (NW Mediterranean). Biogeosciences 6, 1229–1246. doi: 10.5194/bg-6-1229-2009
Grasshoff, K., Ehrhardt, M., and Kremling, K. (1983). Methods of Seawater Analysis. Weinheim: Verlag Chemie.
Hamilton, R. D., and Holm-Hansen, O. (1967). Adenosine triphosphate content of marine bacteria. Limnol. Oceanogr. 12, 319–324. doi: 10.4319/lo.1967.12.2.0319
Hoikkala, L., Kortelainen, P., Soinne, H., and Kuosa, H. (2015). Dissolved organic matter in the Baltic Sea. J. Mar. Syst. 142, 47–61. doi: 10.1016/j.jmarsys.2014.10.005
Jauzein, C., Labry, C., Youenou, A., Quere, J., Delmas, D., and Collos, Y. (2010). Growth and phosphorus uptake by the toxic Dinoflagellate Alexandrium catenella (Dinophyceae) in response to phosphate limitation1. J. Phycol. 46, 926–936. doi: 10.1111/j.1529-8817.2010.00878.x
Karl, D., and Björkman, K. (2015). “Dynamics of dissolved organic phosphorus,” in Biogeochemistry of Dissolved Organic Matter, 2nd Edn, eds D. A. Hansell and C. A. Carlson (London: Academic Press), 234–334.
Karl, D. M. (2014). Microbially mediated transformations of phosphorus in the sea: new views of an old cycle. Annu. Rev. Mar. Sci. 6, 279–337. doi: 10.1146/annurev-marine-010213-135046
Karl, D. M., and Bailiff, M. D. (1989). The measurements of dissolved nucleotides in aquatic environments. Limnol. Oceanogr. 34, 543–558. doi: 10.4319/lo.1989.34.3.0543
Kolowith, L. C., Ingall, E. D., and Benner, R. (2001). Composition and cycling of marine organic phosphorus. Limnol. Oceanogr. 46, 309–320. doi: 10.4319/lo.2001.46.2.0309
Lin, P., Chen, M., and Guo, L. D. (2012). Speciation and transformation of phosphorus and its mixing behavior in the Bay of St. Louis estuary in the northern Gulf of Mexico. Geochim. Cosmochim. Acta 87, 283–298. doi: 10.1016/j.gca.2012.03.040
Liu, Q. J., Parrish, C. C., and Helleur, R. (1998). Lipid class and carbohydrate concentrations in marine colloids. Mar. Chem. 60, 177–188. doi: 10.1016/S0304-4203(97)00103-105
Lomas, M. W., Burke, A. L., Lomas, D. A., Bell, D. W., Shen, C., Dyhrman, S. T., et al. (2010). Sargasso Sea phosphorus biogeochemistry: an important role for dissolved organic phosphorus (DOP). Biogeosciences 7, 695–710. doi: 10.5194/bg-7-695-2010
Lovdal, T., Tanaka, T., and Thingstad, T. F. (2007). Algal-bacterial competition for phosphorus from dissolved DNA, ATP, and orthophosphate in a mesocosm experiment. Limnol. Oceanogr. 52, 1407–1419. doi: 10.4319/lo.2007.52.4.1407
Mather, R. L., Reynolds, S. E., Wolff, G. A., Williams, R. G., Torres-Valdes, S., Woodward, E. M. S., et al. (2008). Phosphorus cycling in the North and South Atlantic Ocean subtropical gyres. Nat. Geosci. 1, 439–443. doi: 10.1038/Ngeo232
Mempin, R., Tran, H., Chen, C. N., Gong, H., Ho, K. K., and Lu, S. W. (2013). Release of extracellular ATP by bacteria during growth. BMC Microbiol 13:301. doi: 10.1186/1471-2180-13-301
Michelou, V. K., Lomas, M. W., and Kirchman, D. L. (2011). Phosphate and adenosine-5 ’-triphosphate uptake by cyanobacteria and heterotrophic bacteria in the Sargasso Sea. Limnol. Oceanogr. 56, 323–332. doi: 10.4319/lo.2011.56.1.0323
Middelboe, M., and Jorgensen, N. O. G. (2006). Viral lysis of bacteria: an important source of dissolved amino acids and cell wall compounds. J. Mar. Biolog. Assoc. U.K. 86, 605–612. doi: 10.1017/S0025315406013518
Monbet, P., McKelvie, I. D., and Worsfold, P. J. (2009). Dissolved organic phosphorus speciation in the waters of the Tamar estuary (SW England). Geochim. Cosmochim. Acta 73, 1027–1038. doi: 10.1016/j.gca.2008.11.024
Moutin, T., Karl, D. M., Duhamel, S., Rimmelin, P., Raimbault, P., Van Mooy, B. A. S., et al. (2008). Phosphate availability and the ultimate control of new nitrogen input by nitrogen fixation in the tropical Pacific Ocean. Biogeosciences 5, 95–109. doi: 10.5194/bg-5-95-2008
Mulholland, M. R., Bronk, D. A., and Capone, D. G. (2004). Dinitrogen fixation and release of ammonium and dissolved organic nitrogen by Trichodesmium IMS101. Aquat. Microb. Ecol. 37, 85–94. doi: 10.3354/ame037085
Nausch, M., Bach, L. T., Czerny, J., Goldstein, J., Grossart, H. P., Hellemann, D., et al. (2016). Effects of CO2 perturbation on phosphorus pool sizes and uptake in a mesocosm experiment during a low productive summer season in the northern Baltic Sea. Biogeosciences 13, 3035–3050. doi: 10.5194/bg-13-3035-2016
Nausch, M., Nausch, G., Mohrholz, V., Siegel, H., and Wasmund, N. (2012). Is growth of filamentous cyanobacteria supported by phosphate uptake below the thermocline? Estuar. Coast. Shelf Sci. 99, 50–60. doi: 10.1016/j.ecss.2011.12.011
Nausch, M., Nausch, G., and Wasmund, N. (2004). Phosphorus dynamics during the transition from nitrogen to phosphate limitation in the central Baltic Sea. Mar. Ecol. Prog. Ser. 266, 15–25. doi: 10.3354/Meps266015
Nausch, M., Nausch, G., Wasmund, N., and Nagel, K. (2008). Phosphorus pool variations and their relation to cyanobacteria development in the Baltic Sea: a three-year study. J. Mar. Syst. 71, 99–111. doi: 10.1016/j.jmarsys.2007.06.004
Nawrocki, M. P., and Karl, D. M. (1989). Dissolved ATP turnover in the Bransfield Strait, Antarctica during a spring bloom. Mar. Ecol. Prog. Ser. 57, 35–44. doi: 10.3354/meps057035
Patey, M. D., Rijkenberg, M. J. A., Statham, P. J., Stinchcombe, M. C., Achterberg, E. P., and Mowlem, M. (2008). Determination of nitrate and phosphate in seawater at nanomolar concentrations. Trac Trends Anal. Chem. 27, 169–182. doi: 10.1016/j.trac.2007.12.006
Paul, A. J., Achterberg, E. P., Bach, L. T., Boxhammer, T., Czerny, J., Haunost, M., et al. (2016). No observed effect of ocean acidification on nitrogen biogeochemistry in a summer Baltic Sea plankton community. Biogeosciences 13, 3901–3913. doi: 10.5194/bg-13-3901-2016
Paul, A. J., Bach, L. T., Schulz, K.-G., Boxhammer, T., Czerny, J., Achterberg, E. P., et al. (2015). Effect of elevated CO2 on organic matter pools and fluxes in a summer, post spring-bloom Baltic Sea plankton community. Biogeosciences 12, 6181–6203. doi: 10.5194/bg-12-6181-2015
Paul, J. H., DeFlaun, M. F., Jeffrey, W. H., and David, A. W. (1988). Seasonal and diel variability in dissolved DNA and in microbial biomass and activity in a subtropical estuary. Appl. Environ. Microbiol. 54, 718–727.
Repeta, D. J., Ferrón, S., Sosa, O. A., Johnson, C. G., Repeta, L. D., Acker, M., et al. (2016). Marine methane paradox explained by bacterial degradation of dissolved organic matter. Nat. Geosci. 9, 884–887. doi: 10.1038/ngeo2837
Ridal, J. J., and Moore, R. M. (1990). A Re-examination of the measurement of dissolved organic phosphorus in seawater. Mar. Chem. 29, 19–31. doi: 10.1016/0304-4203(90)90003-U
Riemann, B. (1979). The occurrence and ecological significance of dissolved ATP in fresh water. Freshw. Biol. 9, 481–490. doi: 10.1111/j.1365-2427.1979.tb01532.x
Siuda, W., and Chrost, R. J. (2001). Utilization of selected dissolved organic phosphorus compounds by bacteria in lake water under non-limiting orthophosphate conditions. Pol. J. Environ. Stud. 10, 475–483.
Siuda, W., and Güde, H. (1996). Evaluation of dissolved DNA and nucleotides as potential sources of phosphorus for plankton organisns in Lake Constance. Arch. Hydrobiol. Spec. Issues Advanc. Limnol. 48, 155–162.
Sohm, J. A., and Capone, D. G. (2010). Zonal differences in phosphorus pools, turnover and deficiency across the tropical North Atlantic Ocean. Glob. Biogeochem. Cycle 24, 1–9. doi: 10.1029/2008gb003414
Suzumura, M., and Ingall, E. D. (2004). Distribution and dynamics of various forms of phosphorus in seawater: insight from field observation in the Pacific Ocean an a laboratory experiment. Deep Sea Res. Oceanogr. Res. Pap. 51, 1113–1130. doi: 10.1016/j.dsr.2004.05.001
Suzumura, M., Ishikawa, K., and Ogawa, H. (1998). Characterization of dissolved organic phosphorus in coastal seawater using ultrafiltration and phosphohydrolytic enzymes. Limnol. Oceanogr. 43, 1553–1564. doi: 10.4319/lo.1998.43.7.1553
Tanaka, T., Henriksen, P., Lignell, R., Olli, K., Seppala, J., Tamminen, T., et al. (2006). Specific affinity for phosphate uptake and specific alkaline phosphatase activity as diagnostic tools for detecting phosphorus-limited phytoplankton and bacteria. Estuar. Coasts 29, 1226–1241. doi: 10.1007/Bf02781823
Thingstad, T. F., Skoldal, E. F., and Bohne, R. A. (1993). Phosphorus cycling and algal-bacterial competition in Sandsfjord, western Norway. Mar. Ecol. Prog. Ser. 99, 239–259. doi: 10.3354/meps099239
Trinkler, S. (2009). Charakterisierung Verschiedener KOMPONENTEN des Pools Gelöster Organischer Phosphorverbindungen in der Ostsee. Rostock: Universität Rostock, 92.
Tuomi, P., and Kuuppo, P. (1999). Viral lysis and grazing loss of bacteria in nutrient- and carbon-manipulated brackish water enclosures. J. Plankton Res. 21, 923–937. doi: 10.1093/plankt/21.5.923
Unger, J., Endres, E., Wannicke, N., Engel, A., Voss, M., Nausch, G., et al. (2013). Response of Nodularia spumigena to pCO2 – Part 3: turnover of phosphorus compounds. Biogeosciences 10, 1483–1499. doi: 10.5194/bg-10-1483-2013
Van Den Broeck, N., Moutin, T., Rodier, M., and Le Bouteiller, A. (2004). Seasonal variations of phosphate availability in the SW Pacific Ocean near New Caledonia. Mar. Ecol. Prog. Ser. 268, 1–12. doi: 10.3354/meps268001
Wannicke, N., Koch, B. P., and Voss, M. (2009). Release of fixed N-2 and C as dissolved compounds by Trichodesmium erythreum and Nodularia spumigena under the influence of high light and high nutrient (P). Aquat. Microb. Ecol. 57, 175–189. doi: 10.3354/ame01343
Wasmund, N., Topp, I., and Schories, D. (2006). Optimizing the storage and extraction of chlorophyll samples. Oceanologia 48, 125–144.
Weinbauer, M. G., Brettar, I., and Hofle, M. G. (2003). Lysogeny and virus-induced mortality of bacterioplankton in surface, deep, and anoxic marine waters. Limnol. Oceanogr. 48, 1457–1465. doi: 10.4319/lo.2003.48.4.1457
Weinbauer, M. G., Fuks, D., Puskaric, S., and Peduzzi, P. (1995). Diel, seasonal, and depth-related variability of viruses and dissolved DNA in the Northern Adriatic Sea. Microb. Ecol. 30, 25–41. doi: 10.1007/BF00184511
Welschmeyer, N. A. (1994). Fluorometric analysis of chlorophylla in the presence of chlorophyllb and phaeopigments. Limnol. Oceanogr. 39, 1985–1992. doi: 10.4319/lo.1994.39.8.1985
White, A. E., Watkins-Brandt, K. S., Engle, M. A., Burkhardt, B., and Paytan, A. (2012). Characterization of the rate and temperature sensitivities of bacterial rem ineralization of dissolved organic phosphorus compounds by natural populations. Front. Microbiol. 3:276. doi: 10.3389/fmicb.2012.00276
Keywords: phosphorus cycle, Baltic Sea, dissolved organic phosphorus (DOP), DOP compounds, adenosine triphosphate, DNA, phospholipids, phosphorus uptake rates
Citation: Nausch M, Achterberg EP, Bach LT, Brussaard CPD, Crawfurd KJ, Fabian J, Riebesell U, Stuhr A, Unger J and Wannicke N (2018) Concentrations and Uptake of Dissolved Organic Phosphorus Compounds in the Baltic Sea. Front. Mar. Sci. 5:386. doi: 10.3389/fmars.2018.00386
Received: 01 June 2018; Accepted: 02 October 2018;
Published: 10 December 2018.
Edited by:
Wolfgang Koeve, GEOMAR Helmholtz Centre for Ocean Research Kiel (HZ), GermanyReviewed by:
Karin M. Björkman, University of Hawaii, United StatesJulia M. Diaz, Skidaway Institute of Oceanography, United States
Copyright © 2018 Nausch, Achterberg, Bach, Brussaard, Crawfurd, Fabian, Riebesell, Stuhr, Unger and Wannicke. This is an open-access article distributed under the terms of the Creative Commons Attribution License (CC BY). The use, distribution or reproduction in other forums is permitted, provided the original author(s) and the copyright owner(s) are credited and that the original publication in this journal is cited, in accordance with accepted academic practice. No use, distribution or reproduction is permitted which does not comply with these terms.
*Correspondence: Monika Nausch, bW9uaWthLm5hdXNjaEBpby13YXJuZW11ZW5kZS5kZQ==