- 1Department of Biology, Norwegian University of Science and Technology (NTNU), Trondheim, Norway
- 2SINTEF Ocean, Environment and New Resources, Trondheim, Norway
- 3Department Benthic Resources and Processes, Institute of Marine Research, Bergen, Norway
- 4Wageningen Marine Research, Yerseke, Netherlands
- 5Bellona, Oslo, Norway
Integrated multi-trophic aquaculture (IMTA) has the potential of reducing open-cage fish farming impacts on the environment while also introducing new value chains. The aim of this study was to investigate the growth and composition of the kelp Saccharina latissima in salmon-driven IMTA, and to assess the spatial extent of the influence of salmon derived nitrogen in order to evaluate the upscaling potential for IMTA. S. latissima was cultivated 100, 200, and 1,000 m east and 1,000 m west of a 5,000 tons salmon farm in Western Norway from February to September 2013. The proportion of salmon derived nitrogen available for the kelp showed a clear decline with distance from the farm. Accordingly, the kelp cultivated near the salmon cages grew faster during the spring season, and growth rate decreased with increasing distance from the farm. A spatially explicit numerical model system (SINMOD), including compartments for dissolved nutrients and kelp growth, was tuned to the field data and used to investigate the potential for upscaling IMTA production. The model was used to introduce a new metric—the impacted area IA—for the areal effects of IMTA in terms of the increase in production by IMTA. The model showed that a 25 hectare kelp farm in the vicinity of the studied salmon farm could take up 1.6 of the 13.5 tons of dissolved inorganic nitrogen released during kelp cultivation, amounting to almost 12% of the ammonia released during the cultivation period from February to June. The 25 hectare kelp farm would have a production yield of 1,125 tons fresh weight (FW), being 60% more than that of a non-IMTA kelp farm, while a 20% increase of kelp FW could be obtained over a 110 hectar area in salmon-driven IMTA. To achieve an even mass balance, an area of approximately 220 ha−1 would be needed to cultivate enough kelp to fix an equivalent of the nitrogen released by the fish.
Introduction
Norway is the world's largest producer of Atlantic salmon (Salmo salar) with a current production of 1.3 million tons per year (FAO, 2017). Atlantic salmon farming traditionally takes place in open sea cages and involves the use of nutrient rich formulated diets. One of the challenges of this open cage production are the waste discharges from fish excretion and feed loss. Even with a low feed conversion ratio of around 1.1 and relatively low losses of feed (3%), there is a substantial release of metabolic waste products such as feces and excreted inorganic nutrients in salmon aquaculture (Wang et al., 2012, 2013). It is estimated that about 60% of feed nitrogen and 70% of feed phosphorus is released into the environment as metabolic wastes. This means that the aquaculture industry fertilizes the Norwegian coast with 52,000 tons of nitrogen and 10,000 tons of phosphorous annually (Wang et al., 2012). Accumulation of effluents in the marine environment may lead to stimulation of phytoplankton growth and eutrophication of pelagic ecosystems, but this is highly dependent on the assimilative capacity of the area and on local hydrodynamics. The area of influence of effluents in surface water ecosystems is dependent on many factors including the biomass produced at the farm, farming practices, and especially environmental conditions such as local topography, water exchange rates, and water depth (Nordvarg and Håkanson, 2002). A full-scale fertilization experiment has suggested that the production capacity of Norwegian coastal waters is quite high (Olsen et al., 2014), and with the current releases of dissolved nutrients from Norwegian aquaculture, these effluents are unlikely to cause unacceptable degradation of surface water quality on a larger scale (Wang et al., 2012; Taranger et al., 2014). With the aim of increasing food production for a continually growing population, there is projected a five-fold increase in Norwegian salmon production by 2050 (Olafsen et al., 2012). By continuing to produce salmon in open sea cages, this increase in production will also lead to a substantial increase in the amount of dissolved nutrients released. In order to ensure a sustainable increase in production, it is important to determine how the potential influence of dissolved inorganic nutrients released by fish farming can influence pelagic ecosystems, and to find ways to mitigate possible negative impacts.
One of the possible methods to alleviate the effect of increased nutrient loading on the environment is by co-cultivating salmon with species of lower trophic levels. Around two thirds of the nitrogen waste from salmon farming is inorganic ammonia (Wang et al., 2013), which is taken up by primary producers such as phytoplankton and macroalgae. Macroalgae cultivated in the vicinity of salmon farms may utilize the dissolved inorganic nutrients released from salmon farms in open-water integrated multi-trophic aquaculture (IMTA) systems (Buschmann et al., 1996; Chopin et al., 2001; Wang et al., 2012, 2014; Handå et al., 2013). Co-producing salmon and kelp might therefore reduce the ecological influence of dissolved nitrogen released into the environment and at the same time produce valuable kelp biomass. Previous studies have shown that the use of kelp in IMTA with salmon has limited bioremediation potential as the kelp can only take up a part of the dissolved nutrients, but the potential for increased biomass production is substantial (Broch et al., 2013).
In this paper, we assess the potential of salmon driven kelp cultivation in terms of increased production and nutrient extraction efficiency. As a basis for the assessment, kelp growth, and elemental composition along a gradient from a salmon cage farm off the coast of Sogn and Fjordane, Norway, was studied over one kelp growth season from deployment in February to September. A numerical model was used to assess the spatial extent of the influence of salmon derived nitrogen to evaluate the potential for upscaling IMTA.
Materials and Methods
Experimental Design
The cultivation experiment took place at the Marine Harvest salmon farm at Flåtegrunnen (N 61°34.586, E 4°48.942), in the county of Sogn and Fjordane, Norway from February to September 2013 (Figures 1A,B). The farm consisted of eight circular cages of 150 m circumference, of which seven were used for salmon production. The eighth cage was used as one of four experimental stations situated 100 m (100 E; empty fish cage), 200 m (200 E), 1,000 m East (1,000 E), and 1,000 m West (1,000 W) of the farm (away from cage with fish) (Figure 1B). Based on previous literature, 1,000 m was expected to be outside of the impacted area and considered being a reference stations reflecting the natural situation (Merceron et al., 2002; Sanderson et al., 2008; Pitta et al., 2009). Bottom depths at the stations varied between 10 and 40 m, and the water column in the upper 10 m was always mixed due to tidal action.
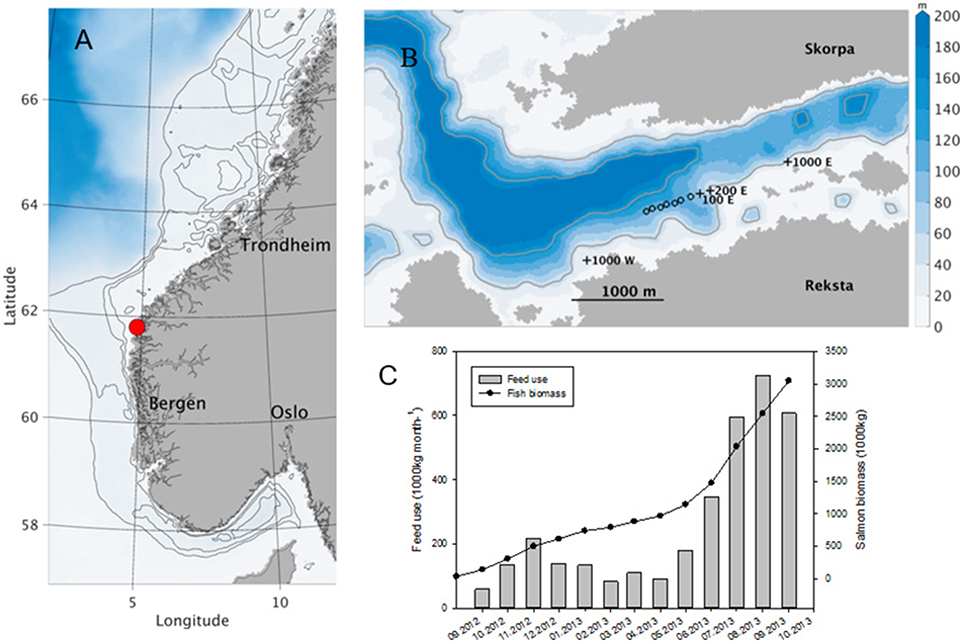
Figure 1. (A) Large scale map showing the approximate position of the fish farm “Flåtegrunnen” at Rekstad, Sogn andFjordane. (B) Detail of the computational domain for the study area in 32 m horisontal resolution showing the positions of the salmon cages and the four experimental stations situated 100 m (100 E), 200 m (200 E) and 1,000 m East (1,000 E), and 1,000 m West (1,000 W). The colors indicate bottom depth, and the gray curves are 50 and 150 m isobaths. (C) Monthly fish biomass and feed used from August 2012 to September 2013.
The westernmost station served as a reference station in this study. Horizontal carrier lines were aligned in the north-south direction and perpendicular to the alignment of the fish farm (East-West) at each station (Figure 1B).
Seedling Production and Deployment
Non-sporogenous individuals of S. latissima were collected close to the field site in September 2012. Meristematic tissue, stipes, and haptera were cut off and the remaining thalli were transferred to experimental tanks for sorus induction. When the sori were mature, the spores were released and seeded on 1.4 mm string, and to maintain genetic variability, spores from 10 different individual sporophytes were seeded. Seedlings were cultivated in flow-through incubators according to methods in Forbord et al. (2012) and Forbord et al. (2018). After 11 weeks in the hatchery the seeded string was twined onto 14 mm diameter ropes, and 20 ropes of 7 m length were deployed vertically with three meters distance from each horizontal carrier line at each station. The sporophyte length at deployment was on average 1 cm. Sampling of sporophytes throughout the season was predetermined for water depths of 2, 5, and 7 m below the surface based on a previous study showing best growth of kelp at 5 m depth in Mid-Norway (Handå et al., 2013).
Sampling
Two cruises were done to run water samples campaigns for the analysis of dissolved inorganic nitrogen (DIN: NO, NO, NH), one in April when feed use and fish biomass were low and one in September when feed use and fish biomass were high. Water samples were taken in triplicates from 5 m depth with a Niskin water collector. CTD-profiling (SAIV 200) showed that the water body was mixed down up to ~10–40 m (Jansen et al., 2018).
During each sampling month the length and width of seaweed of 15 randomly selected individual sporophytes from each of 6 ropes at each of three 1 m depth horizons at 2, 5, and 7 meters at each station was measured at each sampling (15 sporophytes × 6 ropes × 3 depths = 270 sporophytes per station, 4 stations, 4 samplings). Due to fouling related losses of sporophytes during summer we were only able to measure a minimum of 5 sporophytes per depth horizon and rope in few cases. The 1 m depth horizons 2, 5 and 7 meters relates to 1.5–2.5 m, 4.5–5.5 m, and 6.5–7.5 m below the surface, respectively. These horizons were marked on the ropes before deployment.
For chemical analysis five whole sporophytes were collected from 5 m depth at each of the 6 ropes per station and sampling date. The samples were stored at −80°C until freeze-drying. After freeze drying, the whole 5 sporophytes were milled into a powder to achieve a representative sample for the whole sporophyte and remove individual variability. Subsamples of this powder were used for further analysis of carbon and nitrogen concentrations as well as stable isotope signatures of carbon and nitrogen.
Salmon feed, seston and kelp tissue were sampled for stable isotope analysis when fish biomass was low in April and high in September to determine the contribution of salmon derived and naturally occurring nitrogen during the period of kelp cultivation.
Seston was sampled in triplicate at each of the four stations using a Niskin water collector and filtered on a pre-combusted Whatman GF/F filter. Salmon feed was sampled from the respective feed batch in use in April and September. Samples were frozen with dry ice, freeze dried, and stored at −80°C under nitrogen atmosphere until analysis. Stable isotope data was used to calculate one-isotope, two-source linear mixing models (reviewed by Boecklen et al., 2011).
Chemical Analysis
The concentration of total ammonia nitrogen (TAN) and nitrate in the water samples were determined in parallel with a Fluorescence Detector (DFL-10) (Kérouel and Aminot, 1997) and a flow analyser with O.I. Analytical cartridge Part A002603, respectively, according to Jansen et al. (2018). Samples of kelp, salmon feed, and filters with seston were analyzed at the Colorado Plateau Stable Isotope Laboratory at the Northern Arizona University (USA) for content of carbon and nitrogen and stable isotope ratios of δ13C and δ15N. The samples were weighed and packed in tin capsules before analyzing with a Carlo Erba NC2100 elemental analyser coupled with a Thermo-Electron Delta V Advantage isotope ratio mass spectrometer (IRMS), configured through a CONFLO III. Isotope ratios were calculated as follows:
where X = 13C or 15N and R is 13C/12C ratio for carbon and 15N/14N for nitrogen. IAEA standards were used for carbon isotope nitrogen calibration.
Statistical Analyses
One-way ANOVA followed by a Holm-Sidak post- hoc tests were run on growth, % carbon, % nitrogen and C/N data using SigmaPlot 12.0 with a significance level of 0.05. To assess the influence of the fish farm on kelp growth rates we calculated the specific growth rate (μ). As maximum size of the kelp occurred at different sampling dates, we calculated the growth rate from deployment to maximum size. The influence of salmon farming on the relative contribution of inorganic nitrogen in kelp stemming from salmon farming or from natural sources was assessed by ANOVA followed by Holm-Sidak post-hoc test using the arcsin transformed proportion of salmon derived nitrogen calculated in isotope mixing models as the dependent variable. To assess the influence of distance from the farm on specific kelp growth rate and the proportion of natural and salmon derived nitrogen we fitted a 2-parameter hyperbolic decay model to the data, using salmon derived nitrogen from the isotope mixing models as the dependent variable and distance from the farm as the independent variable.
Stable Isotope Mixing Models
The relative contribution of nitrogen from natural sources and from salmon in the kelp was calculated using δ15N signatures of seston and salmon feed as sources and of kelp from the four stations as mixture following Phillips and Gregg (2001). The stable isotope signature of nitrogen excreted by consumers is typically depleted compared to the ingested food sources and 1%0 was subtracted from the salmon feed δ15N signatures (Steele and Daniel, 1978; Minagawa and Wada, 1984).
where δ15NM, δ15NA, and δ15NB represent the mean nitrogen signatures of the mixture M and sources A and B, respectively, and fA and fB are the proportions of A and B in M. Errors are expressed as variance. Due to heavy degradation of kelp in September and virtually no kelp growth throughout the summer, we omitted September from the analysis.
Mathematical Modeling of S. latissima Growth and Production Upscaling
The nested, coupled 3D hydrodynamic-ecological model system SINMOD (Wassmann et al., 2006; Broch et al., 2013) was used to simulate the general environmental conditions around Flåtegrunnen from February to June 2013. The model includes variables for concentrations of phytoplankton (mmol N m−3) and nutrients (NO, NH-N) and is coupled with a growth model for S. latissima. The system can thus simulate dispersal and dilution as well as uptake of nutrients by phytoplankton and kelp. A model setup for the region around the farm at Flåtegrunnen in 32 m horizontal resolution was established (Figure 1). The nesting technique, forcing data and coupling of the models are detailed in Broch et al. (2013, 2017). Release rates of TAN (no distinction between NH and NH3 was made) from the fish farm were calculated from reported figures on feed usage (Figure 1C), using a conversion factor of 0.025 kg TAN-N released (kg dry fish feed used)−1 (adapted from Wang et al., 2012; see also Broch et al., 2013). The S. latissima growth model (Broch and Slagstad, 2012; Broch et al., 2013) was updated and tuned to differentiate between uptake and use of NO and NH (see Appendix).
Four simulations were run using the kelp-ecosystem model. The first two (one with and one without a fish farm effluent) were run in order to determine the spatial extent of the IMTA-effect. The last two (one with and the other without a fish farm effluent) were run in order to assess the increased growth and uptake potential of a large scale (25 ha) kelp installation west of the fish farm, with the same kelp densities as in the main simulations in Broch et al. (2013). The simulations were run from February to June as the fouling of the sporophytes until mid-June was negligible (Førde et al., 2016). After this point, the sporophytes were too heavily fouled and are therefore omitted from this study. For comparison with the simulation results, the frond area of the kelp was calculated as follows (Broch et al., 2013):
where A is the frond area, and L and W are the length and width of the kelp frond, respectively.
Quantification of the Spatiotemporal Influence of IMTA
A new metric, IAP (Integrated Area, unit: area), was used to quantify the spatial extent of the influence of IMTA at a location. This term is applicable to any open IMTA aquaculture system. IAP is the total area of the region where cultivation in IMTA leads to at least a production increase of P% in biomass or another variable of interest (e.g., accumulated carbon or nitrogen) compared with monoculture not associated to a salmon farm. The IAP changes with time, location and cultivation variables. This metric is reminiscent of the area-effect curves presented e.g., in Zeldis et al. (2011), but relates to improved effect due to IMTA rather than effects on basic ecosystem variables. For example, an IA50 = 10 ha for dry weight in April for S. latissima cultures near a salmon farm, means that an area of 10 ha will experience a potential increase in dry weight of at least 50% in April relative to cultivation at the same location, but without the fish farm effluent. Based on the simulation results (see previous subsection), the IAP was calculated as a function of P and time for the kelp-salmon IMTA system studied here.
Results
Environmental Variables
During the experimental period from February to September 2013, the net increase in total salmon biomass at the farm was 2,250 tons, reaching a maximum value of 3,000 tons in September. The monthly feed use increased steadily over the summer and reached a peak value of 700 tons in August (Figure 1C). The surface water temperature ranged from 4°C in April to 15°C in September.
The NH concentrations at 5 m depth ranged from 0.3 to 0.5 mmol m−3 in April and 1.5–3.4 mmol m−3 in September (Figure 2). While there were no differences in NH concentrations between the stations in April, the concentrations found in September were generally higher, and significantly higher at the 100 E station (3.4 mmol m−3) compared to the other stations (<2.0 mmol m−3).
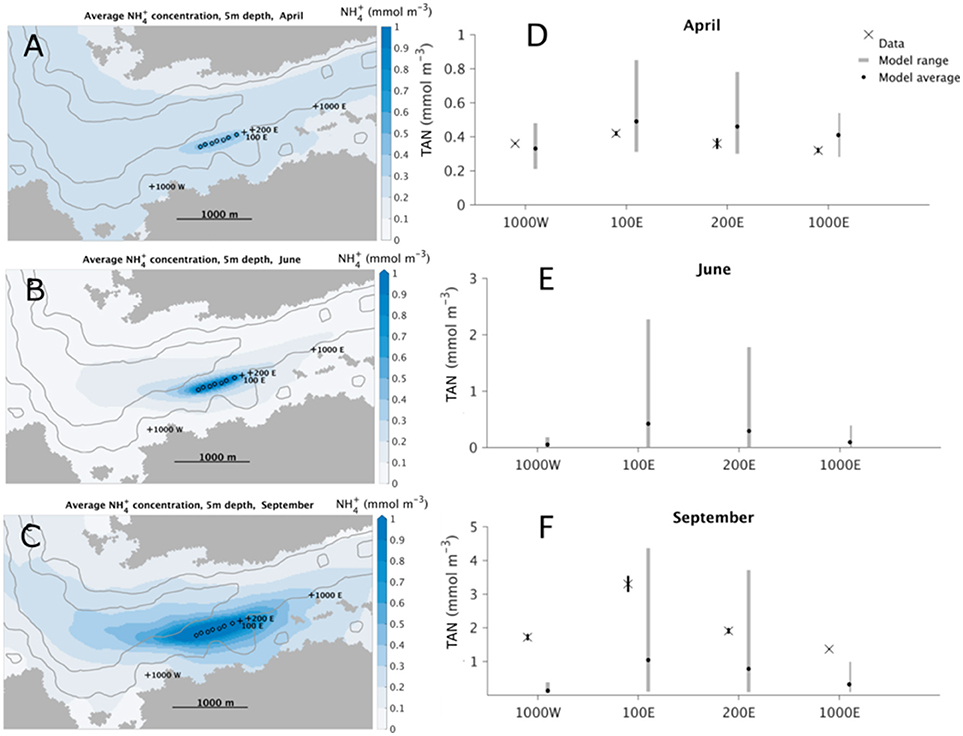
Figure 2. Measure and modeled concentrations of TAN (mmol m−3) in seawater at different stations in April, June and September 2013 (mean±se, n = 3). (A–C) Simulated spatiotemporal extent of the effluent and background NH around the fish farm at Flåtegrunnen. (D–F) Simulated and measured NH-concentrations at all the stations at 5 m depth for April (D) and September (F), and simulated concentrations in June (E). Black xs: measured concentrations; black dots: monthly mean simulated concentrations. Dark gray bars: range of simulated mean daily minimum to mean daily maximum concentrations without fish farm effluents. Light gray bars: range of simulated mean daily minimum to mean daily maximum concentrations with fish farm effluents.
The average simulated pattern of dispersal and dilution (including uptake by phytoplankton) of released NH from the fish farm was similar in April, June, and September, but with different average concentrations (Figure 2). A clearly defined ellipsoidal plume of nutrients around the fish farm is apparent, indicating transport of NH in both easterly and westerly directions. The average daily ranges of simulated NH concentrations at the four stations were underestimated in September compared with the measured concentrations (Figure 2). The measured values were within the average simulated range at all stations in April and at the 100 E and 200 E stations in September.
Growth
Kelp growth did not differ between the three depths (2, 5, and 7 m). S. latissima sporophytes grew significantly faster (P < 0.05) at the 100 E station than at the other stations from February to June, and the sporophytes reached approximately twice the length and width compared to other stations at all depths (Figure 3), corresponding to a three times higher frond area. While the sporophyte length at the 100 E station decreased due to heavy fouling during the summer at all depths, kelp from the 200 E station kept growing, and grew significantly (P < 0.05) faster than kelp from both the 1,000 m stations, and in August reached comparable sporophyte lengths as the 100 E kelpdid in June. The algae grown at the 1,000 m stations never reached comparable sizes. The reduction in plant size from August onwards was caused by heavy fouling by bryozoans, leading to almost complete losses of fronds by September. There was a clear gradient of decreasing growth rates with increasing distance from the farm (Figure 4), suggesting the main influence of the farm to be in the first 200 m from the farm.
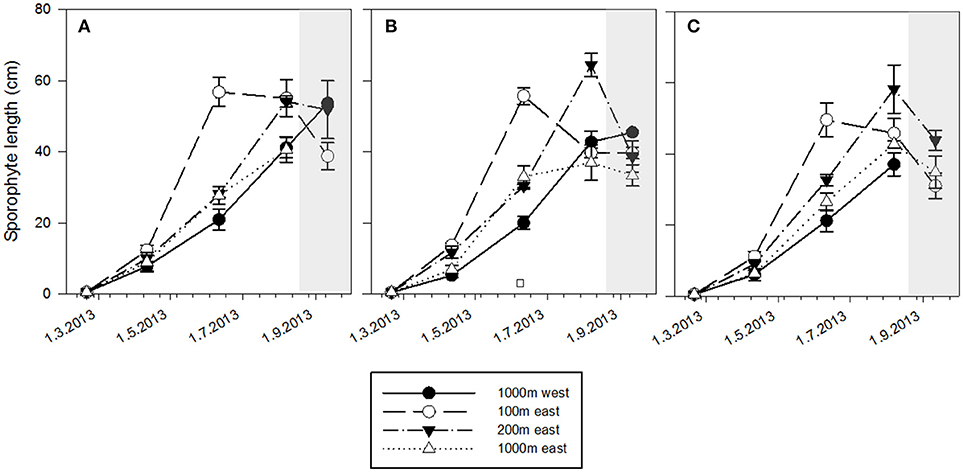
Figure 3. Seasonal development of sporophyte length at 2 m (A), 5 m (B), and 7 m (C) depths at the four experimental stations (mean+-standard error, n = 6). Gray shaded area indicates period of high bio-founling.
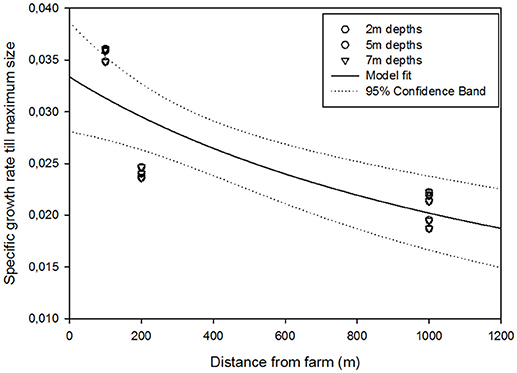
Figure 4. Specific growth rate till maximum kelp size as a function of distance from the farm. Model fitted to data is a hyperbolic decay, 2 parameters, R2 = 0.80.
Simulated frond areas at the four stations showed less spatial variation than the measured values (Equation 4), especially in April (Figure 5). When the simulated frond sizes were presented as ranges over an interval of time (±1 week around the sampling date), the model revealed much the same spatiotemporal growth pattern as the field estimates, especially in June (Figure 5). The simulated frond areas gradually decreased from the 100 E to the 200 E and 1,000 E stations, while the measurements of frond areas at 200 E and 1,000 E were approximately the same.
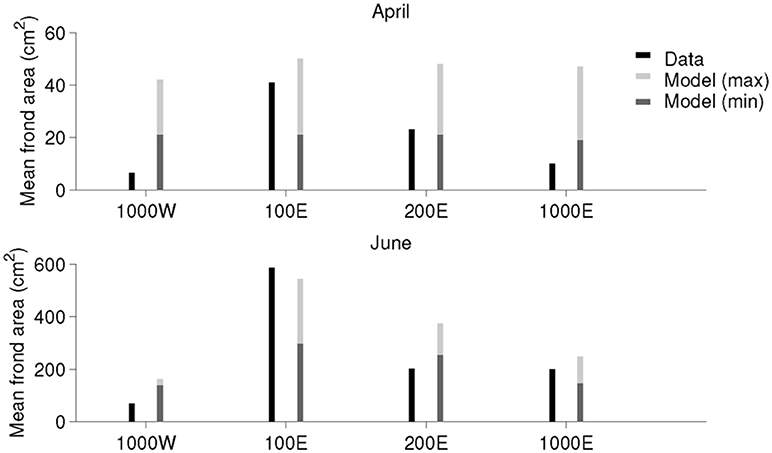
Figure 5. Comparison between simulated and measured frond area values at the four stations (ordered from West to East) on 11th of April (Top) and 11th of June (Bottom). The black stems to the left represent the values estimated from measured frond length and width. The dark gray stems to the right represent the spatial average (3 model grid cells, representing the spatial extent of a kelp long line) of simulated frond areas at 5 m depth 7 days before the sampling dates (4 April and 4 June). The light gray stems represent the same spatial averages 7 days after the sampling dates (18 April and 18 June).
The IA30-region for dry weight (DW) of individuals in June had a shape reminiscent of the contours of NH-concentrations (Figure 2 and Figure 6). The IA30-region is the region in which the simulated kelp dry weight (DW) yield increased by at least 30% from the scenario without fish farm effluents included to the one with effluents included. The region stretched further in a westerly than an easterly direction from the fish cages, covering an area of ~50 ha, including the fish farm.
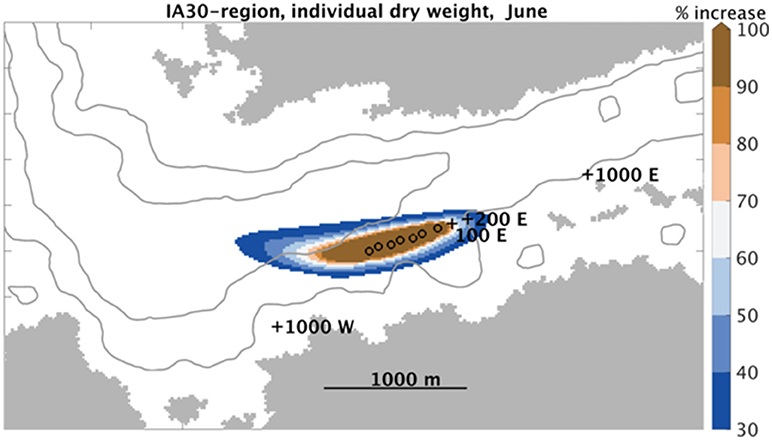
Figure 6. IA30 for dry weight. Spatial extent of the region with a 30% (or greater) increase in individual sporophyte dry weight yields from a simulation scenario without to one with salmon farm effluents. Each model grid cell represents an area of 32 m × 32 m. IA30 = 27 ha in this case.
The plots of the areas of the IAP regions for different times and different values of P (the percentage increase) illustrate the increasing importance of the contribution of NH from the fish farm as the natural background NO was being depleted (Figure 7). In April the area of the region of 20% or higher increase in DW by IMTA (the IA20-region) was almost negligible, while in June this area covered 60–110 ha.
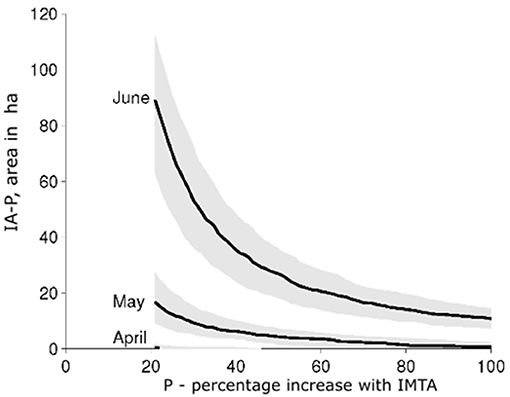
Figure 7. IAP for dry weight as a function of P (%) for April 11th, May 11th, and June 11th (black curves). P (%) is the relative increase in dry weight compared to the same system without salmon farming activities, while IAP is the areal extent of the region experiencing at least this relative increase with IMTA. The shaded regions around the black curves represent the range of IAP values for 1 week before and after the specified dates.
The total simulated biomass production of the hypothetical 25 ha IMTA kelp farm from February to June was about 1,125 tons FW, with a production ha−1 ranging from about 30 tons FW ha−1 furthest away to 130 tons FW ha−1 closest by the fish farm (Table 1). There was a simulated net uptake of 1.6 tons DIN-N, including TAN-N from the farm and TAN-N and NO from the ambient waters. By comparison, the effluent from the fish farm was ~13.5 tons TAN-N from February to June, so that the kelp culture extracted about 12% of the N-effluent during the cultivation period. The 25 ha IMTA farm had a 50% higher average nitrogen uptake, and a 60% higher DW yield compared to an identical farm without fertilization from a fish farm, while the FW yield was 100% higher in the IMTA than the non-IMTA case (Table 1). We also simulated the area necessary to take up 13.5 tons of nitrogen, which equals the estimated N release from the farm during the same period. To achieve an even mass balance, an area of ~220 ha−1 would be needed to cultivate enough kelp to fix an equivalent of the nitrogen released by the fish.
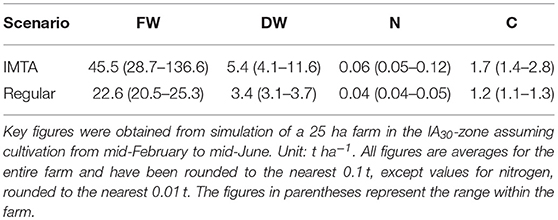
Table 1. Saccharina latissima fresh weight (FW) and dry weight (DW) yields and nitrogen (N) and carbon (C)-uptake with and without IMTA.
Isotope Mixing Models
The relative contribution of nitrogen released through salmon farming and natural inorganic N sources differed significantly between the stations (ANOVA, p < 0.05), with the highest contribution (~40% of salmon derived N) at the nearest station to the farm (100 m) being different from the two stations farthest from the farm (1,000 m, ~10%), with the 200 m station falling in between (29%), not being different from either the 100 m or the 1,000 m stations (Figure 8A). More important than differences between individual stations is the general pattern that the relative contribution of salmon derived nitrogen utilized by the kelp decreased with increasing distance from the salmon cages (Figure 8B). Seston was only sampled in April and September, allowing calculating one-isotope, two-source linear mixing models for these 2 months only. However, the kelp sporophytes were heavily fouled and degraded in September and no considerable growth took place at least from August to September. A meaningful use of stable isotope data as an indicator for the origin of nitrogen in kelp is based on incorporation of nitrogen, i.e., positive growth rates.
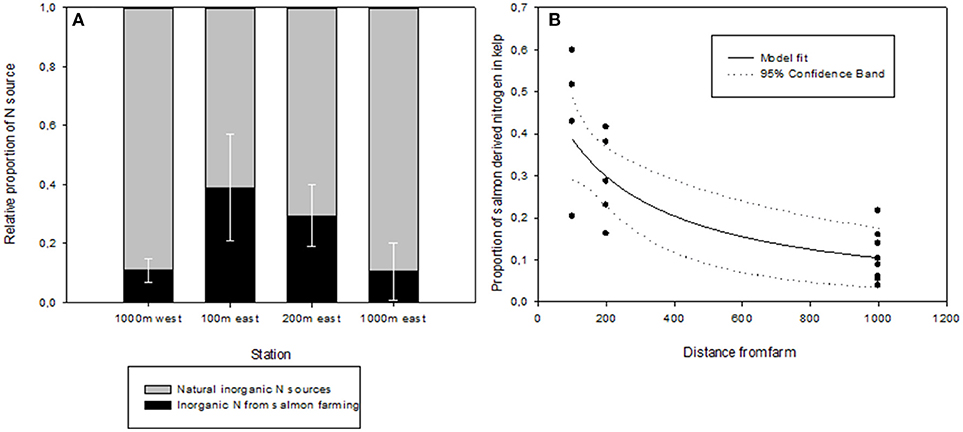
Figure 8. (A) Relative contribution of inorganic nitrogen supplied from either salmon farming or from natural sources in S. latissima based on stable isotope mixing models. Error bars express standard deviation. (B) Relative contribution of inorganic nitrogen supplied from either salmon farming or from natural sources in S. latissima based on stable isotope mixing models as a function of distance from the farm. Model fitted to data is a hyperbolic decay, 2 parameters, R2 = 0.57.
Composition
The fertilizing effect of the farm generally kept the elemental composition of the kelp more stable. The carbon concentration of the kelp tissue varied between 23 and 28% of the dry weight between the stations and within season (Figure 9), with significant differences between stations (p < 0.05). Within the period of positive growth, the 100 E station exhibited the lowest carbon content. The nitrogen contents in the kelp varied from 1 to 2% of the dry weight, reflecting spatiotemporal variability in nitrogen availability (Figure 9). All stations displayed a decrease in nitrogen contents until June, with a less pronounced decrease at the 100 E station (p < 0.05), indicating a less severe nitrogen limitation and the subsidizing role of the fish farm in a period with little or no nitrogen available from natural sources. The lower C/N ratios at the farm (Figure 9) were a result of lower carbon contents as well as higher nitrogen contents. The peak C/N ratios in June, showing the highest values at the 1,000 W station and lowest near the farm, again reflected the low nitrogen content at that time and the potential of nitrogen fertilization of the fish farm.
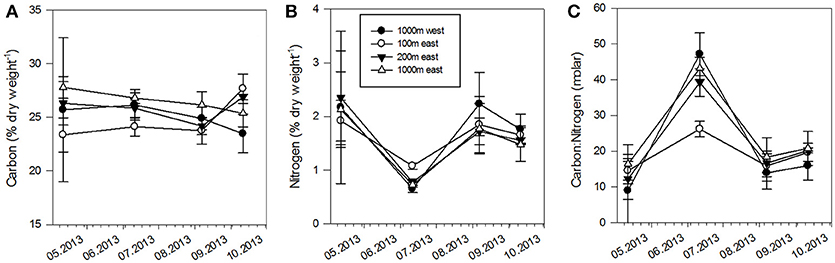
Figure 9. Seasonal percentage of (A) nitrogen, (B) carbon of dry weight, and (C) C:N ratio of kelp tissues at 5 m depth at the four experimental stations (mean, n = 5).
Discussion
The present study examined the feasibility of IMTA combining S. latissima and S. salar in an open net cage system in western Norway. S. latissima sporelings were deployed at several distances away from a salmon farm and algal growth and composition were monitored over one cultivation season from February until September. Additionally, ammonia concentrations were measured and the contribution of salmon derived nitrogen to the total nitrogen pool of the plants was estimated. Finally, the potential increase of kelp production related to the nitrogen input from a fish farm was modeled to quantify the potential for upscaling of kelp (S. latissima) cultivation in salmon-driven IMTA.
The Norwegian coastal ecosystem is characterized by strong seasonal changes in light availability, temperature and nutrient availability. Typically a phytoplankton spring bloom, usually occurring in late March, early April in this part of Norway (Ibrahim et al., 2014), depletes dissolved inorganic nutrients. Such decreases in nitrogen availability can be traced in both micro- and macroalgae by a relative increase in cellular carbon contents and a decrease in nitrogen contents, and consequently increases in C:N ratios in kelp biomass (Gevaert et al., 2001). In the present study, a similar trend with reduced N-contents in kelp tissues farmed 1,000 m away from the salmon farm compared to that farmed closer to the salmon farm, illustrated the nitrogen subsidiary role of fish farming. The nitrogen contents of the sporophytes analyzed in April did not reflect the low DIN concentrations in the surrounding waters. This can be explained by surplus storage of nitrogen in the kelp tissue before the spring bloom when DIN concentrations are still high (Gevaert et al., 2001). As DIN concentrations change quickly in a bloom setting and the timing of the onset of the bloom may vary up to around 20 days from 1 year to another (Vikebø et al., 2012), ambient DIN concentrations were most likely higher just prior to our first sampling on the 8th of April. The nitrogen content of the kelp varied least at the 100 E station despite high growth rates, again suggesting a more constant nitrogen supply than at the other stations. Similar results have been reported by Sanderson et al. (2008), Handå et al. (2013), and Freitas et al. (2016).
Kelp size, elemental, and biochemical composition changes during the growth season (Lüning, 1990). Sharma et al. (2018) reported that sugars, which could serve to produce biofuels peaked earlier in the season, while a later harvest contained the higher proportions of amino acids, minerals, and phenolic compound. Schiener et al. (2015) also reported seasonal variability, where the highest yields of lamianarin and mannitol coincided with the lowest yields in ash, protein, moisture and polyphenols. Clearly, the harvest strategy must be adjusted to the product the kelp shall be converted to (human consumption, biochemicals, biomass). However, there always is a trade-off between little biomass and early harvest. This trade-off might be tipped toward an early harvest if the price of the product counterbalances the potential biomass loss due to early harvest. Potential higher value products might be young kelp sporophytes for human consumption, which tends to have a more tender and favorable texture matching consumer preference. In our study, the nitrogen concentrations showed less variation with season, documenting the fertilizing effect, meaning IMTA could provide more stable and reliable and ultimately predictable product quality, which would add an extra benefit to seaweed culture next to solely increased productivity.
Stable nitrogen isotopes have proved to be useful indicators for the detection of farm derived nitrogen in macroalgae, as they are incorporated into plant tissue (Carballeira et al., 2013). Costanzo et al. (2004), for example, showed a clear decrease of the influence of a shrimp farm in North East Australia with distance from the farm using stable nitrogen isotopes of mangrove leaves. The gradient of the δ15N signature of mangroves along the creek transporting the effluent of the shrimp farm clearly showed not only a dilution effect, but also that nitrogen originating from the shrimp farming operation was taken up along the creek, and hence were incorporated in plant tissue. This suggests that a precise positioning of macroalgae downstream of the nitrogen source in open-water IMTA systems can enable recycling of a part of the dissolved nutrients released from fish farms into the surrounding environment.
In the present study, salmon derived nitrogen was traceable in the kelp tissue by means of stable nitrogen isotopes, and the relative contribution decreased with increasing distance from the fish farm early in the season. Stable isotope analysis samples were taken only in April and September but not during the summer months. The kelp sporophytes were heavily fouled by September, and virtually no growth took place for at least 4 weeks prior the last sampling. Using mixing models to reveal the relative contribution of nitrogen derived from either natural or farming sources to the nitrogen assimilated by kelp in September therefore provided little information. Non-growing organisms are unlikely to isotopically reflect their surroundings, as no or very little new tissue is generated, hence no new materials are incorporated. To further complicate the issue, isotopic ratios change drastically in starving organisms (e.g., Oelbermann and Scheu, 2002; Haubert et al., 2005; Aberle and Malzahn, 2007), and may therefore not reflect the nutrient sources anymore, hence violating one of the basic assumptions behind the use of stable isotopes as tracers for nutrient sources (Hobson et al., 1993).
The decreasing trend in salmon derived nitrogen in the sporophytes raises the question to which extend an aquaculture operation fertilizes the system. Surprisingly, we found a small, but detectable contribution of salmon derived nitrogen 1,000 m away from the farm. The literature suggests rather a more limited spatial influence. Marinho et al. (2015) showed increased production and N uptake in kelp (S. latissima) production 100–500 m away from a in a rainbow trout/blue mussel IMTA system compared to the reference site, which was 2,000 m away from the fish cages in a Danish fjord. However, they note that the fish did not release enough nitrogen to increase N concentrations near the fish cage above background levels. Sanderson et al. (2008) studied ammonium concentrations around salmon cages in north-west Scotland. The authors concluded that generally the N fertilization impact of a fish farm did not exceed 200–300 m and used station a 400 m away from the nutrient source as reference stations. Pitta et al. (2009) reported similar spatial trends in the Aegean Sea, where they concluded that nutrient effects were not visible anymore 500 m away from fish cages. Troell et al. (1997) found higher growth rates of a red algae cultured 10 m away from salmon cages in Chile, than algae grown 150 m and 1,000 m away from the fish farm. As growth and composition of kelp in that study did not differ between 150 and 1,000 m the authors concluded that in their study the spatial impact of the fish farm was small. Even smaller influenced areas were reported by Merceron et al. (2002) who noticed a spatial influence <50 m of a trout cage in Cherbourg, France. However, Sanderson (2006) found indications for fish farm derived nitrogen 1,000 m away from a fish farm in wild kelp. Similar findings were reported by Abreu et al. (2009), who also found a fertilizing effect of a salmon farm in southern Chile. The authors report that Gracialaria chilensis utilized nitrogen derived from salmon farming at least 800 m away from the farm. Nordvarg and Johansson (2002) even showed increased system productivity related to fish farms of up to 8 km2, however, the latter study mainly dealt with semi-closed bays with limited water exchange. Clearly, the extent of the influence of a fish farm depends on the amount of nutrients released and the hydrographic conditions the fish farm is placed at, and the term “influenced” is not well-defined and can span from “traces of fertilizing effects can be found,” to “substantial fertilizing effect useful for IMTA.”
The measured field data was used to update the kelp model to quantify the potential for upscaling of kelp (S. latissima) cultivation in salmon-driven IMTA. The simulation results showed a development in S. latissima frond size similar to that found by field measurements, though with a less pronounced difference between the stations (Figure 4). The simulations further indicated a greater potential for increased biomass in IMTA in the western than the eastern direction from the fish farm. The introduction of the IA-metric (Figure 6) is useful in this context because it focuses on the influenced area, independent of any specific current direction. The IA-metric integrates all factors like background nutrient availability, light intensity, temperature, water current speed, and the feeding intensity of the fed species (in this case Atlantic salmon) over time and is one measure of the potential efficiency of an IMTA system.
An efficiency measure in IMTA related to this is the increase in production by implementing IMTA compared to a monoculture system. The total fresh biomass production for a 25 ha virtual kelp farm in the IA30 zone was 100% higher in the IMTA case than in monoculture without nutrients from salmon farming. By comparison, Sanderson et al. (2012) reported a 26% increase in L. saccharina productivity when grown in IMTA. However, the latter study reported a production capacity of about 220 t ha−1 y−1, which exceeds an average production of 45 t ha−1 y−1 modeled in our study. Troell et al. (1997) calculated similar high potential yields in an salmon/kelp-IMTA with a production potential of about 250 t wet weight ha−1 y−1. Clearly the increase in production due to IMTA and the production capacity depends on the trophic state of the system, where a lower natural nutrient background leads to higher increased in productivity.
From a bioremediation and mass balance perspective, the simulations indicated that a 25 ha kelp farm could take up almost 12% of the ammonia released during the cultivation period from February to June. This equals 0.47% taken up per ha, which corresponds well with the 0.41% per ha obtained in a previous study (Broch et al., 2013). Other model exercises (Hadley et al., 2016) revealed higher N extraction capacities of kelp IMTA systems, where the authors report an uptake potential of 30–80%, albeit without any specific mention of the area covered by the seaweed cultures. However, in the current study the yield would be larger if there would be a better temporal overlap in feed use in aquaculture (being high in the second half of a year) and the main kelp growth period. In general, the correct timing of seeding and harvest is of utmost importance for a successful extraction of fish farm released nitrogen, as stressed in several studies (e.g., Broch et al., 2013; Freitas et al., 2016; Buck et al., 2018).
Conclusions
The results suggest that direct uptake of nutrients in close vicinity to salmon farms can enhance growth and biomass production of S. latissima in Norwegian coastal waters. Macroalgae cultivated in IMTA contained more nitrogen suggesting higher protein content. Over the main growth season for macroalgae (February-June), a 25 ha S. latissima farm positioned close to a salmon farm could take up 12% of the 13.5 tons of inorganic nitrogen released from the salmon. The farm would have a potential average yield of 45.5 tons of S. latissima per hectare compared to a kelp farm monoculture of 22.6 tons. Co-cultivating salmon and kelp in IMTA therefore has potential to produce more biomass, however, has also limited bioremediation effects when it comes to the uptake of released dissolved inorganic nutrients from salmon farms. This is especially an effect of the temporal mismatches between the release by fish and uptake rates by kelp.
Author Contributions
JF, SF, AH, HJ, MB, HF, and AF were involved in planning, execution of the experiment, and writing of the manuscript. OB ran the modeling exercises and contributed to writing. JS and YO were involved in planning, and writing. AM was analyzing data and writing the manuscript.
Conflict of Interest Statement
The authors declare that the research was conducted in the absence of any commercial or financial relationships that could be construed as a potential conflict of interest.
Acknowledgments
The present research is a contribution to the EXPLOIT (project no. 216201/E40) and MACROSEA (project no. 254883/E40) projects funded by the Research Council of Norway. We are grateful to Marine Harvest for kindly providing research facilities and vessels and for all practical support. We thank NOTUR (project no. 2967k) and the HPC center at UiT / The Arctic University of Norway for computational and human resources.
Supplementary Material
The Supplementary Material for this article can be found online at: https://www.frontiersin.org/articles/10.3389/fmars.2018.00418/full#supplementary-material
References
Aberle, N., and Malzahn, A. M. (2007). Inter-specific and nutrient-dependent variations in stable isotope fractionation: experimental studies simulating pelagic multi-trophic systems. Oecologia 154, 291–303. doi: 10.1007/s00442-007-0829-5
Abreu, M. H., Varela, D. A., Henríquez, L., Villarroel, A., Yarish, C., Sousa-Pinto, I., et al. (2009). Traditional vs. integrated multi-trophic aquaculture of Gracilaria chilensis C. J. Bird, J. McLachlan & E. C. Oliveira: productivity and physiological performance. Aquaculture 293, 211–220. doi: 10.1016/j.aquaculture.2009.03.043
Ahn, O., Petrell, R. J., and Harrison, P. J. (1998). Ammonium and nitrate uptake by Laminaria saccharina and Nereocystis luetkeana originating from a salmon sea cage farm. J. Appli. Phycol. 10, 333–340. doi: 10.1023/A:1008092521651
Boecklen, W. J., Yarnes, C. T., Cook, B. A., and James, A. C. (2011). On the use of stable isotopes in trophic ecology. Annu. Rev. Ecol. Evol. Syst. 42, 411–440. doi: 10.1146/annurev-ecolsys-102209-144726
Broch, O. J., Daae, R. L., Ellingsen, I. H., Nepstad, R., Bendiksen, E. Å., Reed, J. L., et al. (2017). Spatiotemporal dispersal and deposition of fish farm wastes: a model study from central Norway. Front. Marine Sci. 4:199. doi: 10.3389/fmars.2017.00199
Broch, O. J., Ellingsen, I. H., Forbord, S., Wang, X., Volent, Z., Alver, M. O., et al. (2013). Modelling the cultivation and bioremediation potential of the kelp Saccharina latissima in close proximity to an exposed salmon farm in Norway. Aquacul. Environ. Inter. 4, 187–206. doi: 10.3354/aei00080
Broch, O. J., and Slagstad, D. (2012). Modelling seasonal growth and composition of the kelp Saccharina latissima. J. Appli. Phycol. 24, 759–776. doi: 10.1007/s10811-011-9695-y
Buck, B. H., Troell, M. F., Krause, G., Angel, D. L., Grote, B., and Chopin, T. (2018). State of the art and challenges for offshore integrated multi-trophic aquaculture (IMTA). Front. Marine Sci. 5:165. doi: 10.3389/fmars.2018.00165
Buschmann, A. H., Troell, M., Kautsky, N., and Kautsky, L. (1996). Integrated tank cultivation of salmonids and Gracilaria chilensis (Gracilariales, Rhodophyta). Hydrobiologia 326–327, 75–82. doi: 10.1007/BF00047789
Carballeira, C., Viana, I. G., and Carballeira, A. (2013). δ15N values of macroalgae as an indicator of the potential presence of waste disposal from land-based marine fish farms. J. Appl. Phycol. 25, 97–107. doi: 10.1007/s10811-012-9843-z
Chapman, A. R. O., Markham, J. W., and Lüning, K. (1978). Effects of nitrate concentration on the growth and physiology of Laminaria saccharina (Phaeophyta) in culture. J. Phycol. 14, 195–198. doi: 10.1111/j.1529-8817.1978.tb02448.x
Chopin, T., Buschmann, A. H., Halling, C., Troell, M., Kautsky, N., Neori, A., et al. (2001). Integrating seaweeds into marine aquaculture systems: a key toward sustainability. J. Phycol. 37, 975–986. doi: 10.1046/j.1529-8817.2001.01137.x
Costanzo, S. D., O'Donohue, M. J., and Dennison, W. C. (2004). Assessing the influence and distribution of shrimp pond effluent in a tidal mangrove creek in north-east Australia. Mar. Pollut. Bull. 48, 514–525. doi: 10.1016/j.marpolbul.2003.09.006
Falkowski, P. G., and Raven, J. A. (2007). Aquatic Photosynthesis. Princeton, NJ; Oxford: Princeton University Press.
FAO (2017). Global Aquaculture Production 1950-2016 [Online]. Fisheries Department, Fishery Information, Data and Statistics Unit, Rome Italy. (Accessed June, 2018).
Forbord, S., Skjermo, J., Arff, J., Handå, A., Reitan, K. I., Bjerregaard, R., et al. (2012). Development of Saccharina latissima (Phaeophyceae) kelp hatcheries with year-round production of zoospores and juvenile sporophytes on culture ropes for kelp aquaculture. J. Appl. Phycol. 24, 393–399. doi: 10.1007/s10811-011-9784-y
Forbord, S., Steinhovden, K., Kjølbo-Rød, K., Handå, A., and Skjermo, J. (2018). “Cultivation protocol for Saccharina latissima,” in Protocols for Macroalgae Research, 1st Edn, eds B. Charrier, T. Wichard, and C. R. K. Reddy (Boca Raton, FL; London; New York, NY: CRC Press), 37–59.
Førde, H., Forbord, S., Handå, A., Fossberg, J. L., Arff, J., Johnsen, G., et al. (2016). Development of bryozoan fouling on cultivated kelp (Saccharina latissima) in Norway. J. Appl. Phycol. 28, 1225–1234. doi: 10.1007/s10811-015-0606-5
Freitas, J. R. C., Morrondo, J. M. S., and Ugarte, J. C. (2016). Saccharina latissima (Laminariales, Ochrophyta) farming in an industrial IMTA system in Galicia (Spain). J. Appl. Phycol. 28, 377–385. doi: 10.1007/s10811-015-0526-4
Gevaert, F., Davoult, D., Creach, A., Kling, R., Janquin, M. A., Seuront, L., et al. (2001). Carbon and nitrogen content of Laminaria saccharina in the eastern English channel: biometrics and seasonal variations. J. Marine Biol. Assoc. UK 81, 727–734. doi: 10.1017/S0025315401004532
Hadley, S., Wild-Allen, K., Johnson, C., and Macleod, C. (2016). Quantification of the impacts of finfish aquaculture and bioremediation capacity of integrated multi-trophic aquaculture using a 3D estuary model. J. Appli. Phycol. 28, 1875–1889. doi: 10.1007/s10811-015-0714-2
Handå, A., Forbord, S., Wang, X. X., Broch, O. J., Dahle, S. W., Storseth, T. R., et al. (2013). Seasonal- and depth-dependent growth of cultivated kelp (Saccharina latissima) in close proximity to salmon (Salmo salar) aquaculture in Norway. Aquaculture 414, 191–201. doi: 10.1016/j.aquaculture.2013.08.006
Haubert, D., Langel, R., Scheu, S., and Ruess, L. (2005). Effects of food quality, starvation and life stage on stable isotope fractionation in Collembola. Pedobiologia 49, 229–237. doi: 10.1016/j.pedobi.2004.11.001
Hobson, K. A., Alisauskas, R. T., and Clark, R. G. (1993). Stable-nitrogen isotope enrichment in avian tissues due to fasting and nutritional stress: implications for isotopic analysis of diet. Condor 95, 388–394. doi: 10.2307/1369361
Ibrahim, A., Olsen, A., Lauvset, S., and Rey, F. (2014). Seasonal variations of the surface nutrients and hydrography in the Norwegian Sea. Int. J. Environ. Sci. Dev. 5, 496–505. doi: 10.7763/IJESD.2014.V5.534
Jansen, H. M., Broch, O. J., Bannister, R., Cranford, P., Handå, A., Husa, V., et al. (2018). Spatial-temporal dynamics in the dissolved nutrient waste plume from Norwegian salmon cage aquaculture. Aquacul. Environ. Inter. 10, 385–399. doi: 10.3354/aei00276
Kérouel, R., and Aminot, A. (1997). Fluorometric determination of ammonia in sea and estuarine waters by direct segmented flow analysis. Marine Chem. 57, 265–275. doi: 10.1016/S0304-4203(97)00040-6
Lüning, K. (1990). Seaweeds: Their Environment, Biogeography, and Ecophysiology. New York, NY; Chichester; Brisbane; Toronto; Singapore: Wiley.
Marinho, G. S., Holdt, S. L., Birkeland, M. J., and Angelidaki, I. (2015). Commercial cultivation and bioremediation potential of sugar kelp, Saccharina latissima, in Danish waters. J. Appli. Phycol. 27, 1963–1973. doi: 10.1007/s10811-014-0519-8
Merceron, M., Kempf, M., Bentley, D., Gaffet, J.-D., Le Grand, J., and Lamort-Datin, L. (2002). Environmental impact of a salmonid farm on a well flushed marine site: I. current water quality. J. Appl. Ichthyol. 18, 40–50. doi: 10.1046/j.1439-0426.2002.00306.x
Minagawa, M., and Wada, E. (1984). Stepwise enrichment of 15N along food chains: further evidence and the relation between δ15N and animal age. Geochim. Cosmochim. Acta 48, 1135–1140. doi: 10.1016/0016-7037(84)90204-7
Nordvarg, L., and Håkanson, L. (2002). Predicting the environmental response of fish farming in coastal areas of the Åland archipelago (Baltic Sea) using management models for coastal water planning. Aquaculture 206, 217–243. doi: 10.1016/S0044-8486(01)00719-0
Nordvarg, L., and Johansson, T. (2002). The effects of fish farm effluents on the water quality in the Åland archipelago, Baltic Sea. Aquacul. Eng. 25, 253–279. doi: 10.1016/S0144-8609(01)00088-7
Oelbermann, K., and Scheu, S. (2002). Effects of prey type and mixed diets on survival, growth and development of a generalist predator, Pardosa lugubris (Araneae: Lycosidae). Basic Appl. Ecol. 3, 285–291. doi: 10.1078/1439-1791-00094
Olafsen, T., Winther, U., Olsen, Y., and Skjermo, J. (2012). Value Created from Productive Oceans in 2050. Royal Norwegian Society of Sciences and Letters (DKNVS) and the Norwegian Academy of Technological Sciences (NTVA). 82 pp.
Olsen, Y., Reinertsen, H., Sommer, U., and Vadstein, O. (2014). Responses of biological and chemical components in North East Atlantic coastal water to experimental nitrogen and phosphorus addition - A full scale ecosystem study and its relevance for management. Sci. Total Environ. 473, 262–274. doi: 10.1016/j.scitotenv.2013.12.028
Phillips, D. L., and Gregg, J. W. (2001). Uncertainty in source partitioning using stable isotopes. Oecologia 127:171. doi: 10.1007/s004420100723
Pitta, P., Tsapakis, M., Apostolaki, E. T., Tsagaraki, T., Holmer, M., and Karakassis, I. (2009). “Ghost nutrients” from fish farms are transferred up the food web by phytoplankton grazers. Mar. Ecol. Prog. Series 374, 1–6. doi: 10.3354/meps07763
Sanderson, J. C. (2006). Reducing the Environmental Impacts of Sea-Cage Fish Farming Through Cultivation of Seaweed. Ph D. Thesis. The Open University.
Sanderson, J. C., Cromey, C. J., Dring, M. J., and Kelly, M. S. (2008). Distribution of nutrients for seaweed cultivation around salmon cages at farm sites in north–west Scotland. Aquaculture 278, 60–68. doi: 10.1016/j.aquaculture.2008.03.027
Sanderson, J. C., Dring, M. J., Davidson, K., and Kelly, M. S. (2012). Culture, yield and bioremediation potential of Palmaria palmata (Linnaeus) Weber & Mohr and Saccharina latissima (Linnaeus) C.E. Lane, C. Mayes, Druehl & G.W. Saunders adjacent to fish farm cages in northwest Scotland. Aquaculture 354–355, 128–135. doi: 10.1016/j.aquaculture.2012.03.019
Schiener, P., Black, K. D., Stanley, M. S., and Green, D. H. (2015). The seasonal variation in the chemical composition of the kelp species Laminaria digitata, Laminaria hyperborea, Saccharina latissima and Alaria esculenta. J. Appli. Phycol. 27, 363–373. doi: 10.1007/s10811-014-0327-1
Sharma, S., Neves, L., Funderud, J., Mydland, L. T., Overland, M., and Horn, S. J. (2018). Seasonal and depth variations in the chemical composition of cultivated Saccharina latissima. Algal Res. Biomass Biofuels Bioprod. 32, 107–112. doi: 10.1016/j.algal.2018.03.012
Steele, K. W., and Daniel, R. M. (1978). Fractionation of nitrogen isotopes by animals: a further complication to the use of variations in the natural abundance of 15N for tracer studies. J. Agri. Sci. 90, 7–9. doi: 10.1017/S002185960004853X
Taranger, G. L., Karlsen, Ø., Bannister, R. J., Glover, K. A., Husa, V., Karlsbakk, E., et al. (2014). Risk assessment of the environmental impact of Norwegian Atlantic salmon farming. ICES J. Marine Sci. 72, 997–1021. doi: 10.1093/icesjms/fsu132
Troell, M., Halling, C., Nilsson, A., Buschmann, A. H., Kautsky, N., and Kautsky, L. (1997). Integrated marine cultivation of Gracilaria chilensis (Gracilariales, Rhodophyta) and salmon cages for reduced environmental impact and increased economic output. Aquaculture 156, 45–61.
Vikebø, F. B., Korosov, A., Stenevik, E. K., Husebø, Å., and Slotte, A. (2012). Spatio-temporal overlap of hatching in Norwegian spring-spawning herring and the spring phytoplankton bloom at available spawning substrata. ICES J. Mar. Sci. 69, 1298–1302. doi: 10.1093/icesjms/fss083
Wang, X., Andresen, K., Handå, A., Jensen, B., Reitan, K. I., and Olsen, Y. (2013). Chemical composition and release rate of waste discharge from an Atlantic salmon farm with an evaluation of IMTA feasibility. Aqua. Environ. Inter. 4, 147–162. doi: 10.3354/aei00079
Wang, X., Broch, O. J., Forbord, S., Handa, A., Skjermo, J., Reitan, K. I., et al. (2014). Assimilation of inorganic nutrients from salmon (Salmo salar) farming by the macroalgae (Saccharina latissima) in an exposed coastal environment: implications for integrated multi-trophic aquaculture. J. Appl. Phycol. 26, 1869–1878. doi: 10.1007/s10811-013-0230-1
Wang, X., Olsen, L. M., Reitan, K. I., and Olsen, Y. (2012). Discharge of nutrient wastes from salmon farms: environmental effects, and potential for integrated multi-trophic aquaculture. Aqua. Environ. Inter. 2, 267–283. doi: 10.3354/aei00044
Wassmann, P., Reigstad, M., Haug, T., Rudels, B., Carroll, M. L., Hop, H., et al. (2006). Food webs and carbon flux in the Barents Sea. Prog. Oceanograp. 71, 232–287. doi: 10.1016/j.pocean.2006.10.003
Keywords: nitrogen, bioremediation, stable isotope, numerical modeling, Norway
Citation: Fossberg J, Forbord S, Broch OJ, Malzahn AM, Jansen H, Handå A, Førde H, Bergvik M, Fleddum AL, Skjermo J and Olsen Y (2018) The Potential for Upscaling Kelp (Saccharina latissima) Cultivation in Salmon-Driven Integrated Multi-Trophic Aquaculture (IMTA). Front. Mar. Sci. 5:418. doi: 10.3389/fmars.2018.00418
Received: 18 June 2018; Accepted: 19 October 2018;
Published: 09 November 2018.
Edited by:
Liping Liu, Shanghai Ocean University, ChinaReviewed by:
Yi Zhou, Institute of Oceanology (CAS), ChinaBrett W. Molony, Department of Primary Industries and Regional Development of Western Australia (DPIRD), Australia
Alejandro H. Buschmann, University of Los Lagos, Chile
Copyright © 2018 Fossberg, Forbord, Broch, Malzahn, Jansen, Handå, Førde, Bergvik, Fleddum, Skjermo and Olsen. This is an open-access article distributed under the terms of the Creative Commons Attribution License (CC BY). The use, distribution or reproduction in other forums is permitted, provided the original author(s) and the copyright owner(s) are credited and that the original publication in this journal is cited, in accordance with accepted academic practice. No use, distribution or reproduction is permitted which does not comply with these terms.
*Correspondence: Ole Jacob Broch, b2xlLmphY29iLmJyb2NoQHNpbnRlZi5ubw==