- 1Millennium Institute of Oceanography (IMO), University of Concepción, Concepción, Chile
- 2Department of Aquatic Systems, Faculty of Environmental Science, University of Concepción, Concepción, Chile
- 3Patagonian Ecosystems Research Center (CIEP), Coyhaique, Chile
- 4Center for the Study of Multiple-Drivers on Marine Socio-Ecological Systems (MUSELS), University of Concepción, Concepción, Chile
- 5Department of Ecology, Pontifical Catholic University of Chile, Santiago, Chile
- 6UMI 3614 Evolutionary Biology and Ecology of Algae, CNRS, Sorbonne University, Pontificia Universidad Católica de Chile, Universidad Austral de Chile, Station Biologique, Roscoff, France
The interplay of coastal oceanographic processes usually results in partial pressures of CO2 (pCO2) higher than expected from the equilibrium with the atmosphere and even higher than those expected by the end of the century. Although this is a well-known situation, the natural variability of seawater chemistry at the locations from which tested organisms or communities originate is seldom considered in ocean acidification experiments. In this work, we aimed to evaluate the role of the carbonate chemistry dynamics in shaping the response of coastal phytoplankton communities to increased pCO2 levels. The study was conducted at two coastal ecosystems off Chile, the Valdivia River estuary and the coastal upwelling ecosystem in the Arauco Gulf. We characterized the seasonal variability (winter/summer) of the hydrographic conditions, the carbonate system parameters, and the phytoplankton community structure at both sites. The results showed that carbonate chemistry dynamics in the estuary were mainly related to seasonal changes in freshwater discharges, with acidic and corrosive conditions dominating in winter. In the Arauco Gulf, these conditions were observed in summer, mainly associated with the upwelling of cold and high pCO2 (>1,000 μatm) waters. Diatoms dominated the phytoplankton communities at both sites, yet the one in Valdivia was more diverse. Only certain phytoplankton groups in this latter ecosystem showed a significant correlations with the carbonate system parameters. When the impact of elevated pCO2 levels was investigated by pCO2 manipulation experiments, we did not observe any significant effect on the biomass of either of the two communities. Changes in the phytoplankton species composition and abundance during the incubations were related to other factors, such as competition and growth phases. Our findings highlight the importance of the natural variability of coastal ecosystems and the potential for local adaptation in determining responses of coastal phytoplankton communities to increased pCO2 levels.
1. Introduction
Human activities over the last two centuries have triggered changes in the global climate system at a pace unprecedented over the past 300 Myr (Caldeira and Wickett, 2003). On a global scale, the air-sea surface gas exchange has promoted an oceanic uptake of about one third of the recent anthropogenic CO2 emissions (Sabine et al., 2004; Gruber et al., 2019), leading to an alteration of the seawater carbonate chemistry and a reduction in seawater pH of 0.1 units (Hoegh-Guldberg and Bruno, 2010). This process is well-known as ocean acidification (OA, Orr et al., 2005) and is expected to further decrease average oceanic pH between 0.14 and 0.35 units by the end of the century (Caldeira and Wickett, 2003), which will likely have substantial consequences for marine ecosystems (Bopp et al., 2013).
Models predicting future changes in the carbonate chemistry associated to OA can be fairly accurately applied in the open ocean, where the environmental heterogeneity is rather low (Hofmann et al., 2011). Nevertheless, anticipating the synergic effects of OA in coastal ecosystems becomes more complex, owing to the high fluctuations in the physical-chemical properties in short temporal and spatial scales that occurs in these regions (Booth et al., 2012; Waldbusser and Salisbury, 2014). The partial pressure of CO2 (pCO2) in the coastal domain exhibits a higher natural variability related both to biological processes, such as respiration and photosynthesis (Shamberger et al., 2011; Buapet et al., 2013; Saderne et al., 2013), as well as to oceanographic processes including the upwelling of deep waters and the discharge of fresh water from rivers (e.g., Cao et al., 2011; Vargas et al., 2016). The dynamic interplay of these coastal processes may result in pCO2 levels in surface waters higher than expected from the equilibrium with the atmosphere (Hofmann et al., 2011; Wallace et al., 2014). One factor significantly contributing to pCO2 variability in coastal areas is the discharge of riverine waters (Cao et al., 2011; Vargas et al., 2016). These waters are typically more acidic and corrosive than oceanic waters as they present low pH values and a reduced buffering capacity linked to their low alkalinity (Raymond and Cole, 2003; Vargas et al., 2018). In addition, the remineralization of the organic matter transported by rivers by heterotrophic organisms results in high DIC and pCO2 levels. In this regard, estuarine environments constitute one naturally acidified coastal ecosystem, where the pCO2 values can reach several thousands microatmospheres (Borges et al., 2006; Wallace et al., 2014), representing a potential source of CO2 to the atmosphere. The other example is observed in upwelling-influenced coastal zones, where the upwelling-favorable winds promote the transport to the surface of naturally acidic subsurface waters characterized by low pH (<8) and high pCO2 (>600 μatm) levels, resulting in a decrease of the seawater pH in the nearshore area (Feely et al., 2008). However, at the same time, these nutrient-rich upwelled waters stimulate primary production, which lower the concentration of dissolved inorganic carbon (DIC), ultimately reducing the levels of pCO2. Owing to such local acidification processes in the coastal zone, marine organisms inhabiting this areas are constantly exposed to low pH/high pCO2 conditions and, therefore, may be operating at the limit of their physiological tolerances. Further acidification of the water through OA may lead to a synergistic effect (Gruber, 2011) and bring their physiology beyond these thresholds. Conversely, it may also occur that local adaptation to these highly variable environments confers on these organisms a higher resilience capacity to face sudden changes and increases in the pCO2 conditions (e.g., Duarte et al., 2013; Vargas et al., 2017). All these potential effects and consequences are still a mater of investigation.
The impact of high pCO2 levels on the phytoplankton community has been previously characterized in several distinct ecosystems, e.g., the Northern Sea (Burkhardt et al., 2001), equatorial Pacific (Tortell et al., 2002) or Southern ocean (Tortell et al., 2008; Trimborn et al., 2013), among many others. However, these studies have shown contrasting responses of the phytoplankton community structure to increased CO2 levels. For instance, at high pCO2 conditions, Tortell et al. (2002) observed a decrease in the abundance of diatoms and an increase of the non-siliceous groups whereas Tortell et al. (2008) reported an increase in primary production and abundance of the chain-forming diatoms Chaetoceros spp. Hare et al. (2007), in turn, showed that diatoms were replaced by nanoflagellates under these same conditions. Therefore, there is not a clear consensus about which groups will be benefited in a high pCO2 environment. In this light, knowing the carbonate system dynamics of the ecosystems where the phytoplankton communities inhabit may be crucial to correctly interpret the outcome of these investigations (Hofmann et al., 2011; Vargas et al., 2017). Unfortunately, this information is usually lacking in the investigation of the impact of OA.
In the present work, we provide insights into the natural seasonal variability of the carbonate system and the phytoplankton community structure and physiology at two contrasting coastal areas off central Chile: an estuarine ecosystem (Valdivia River estuary), and a coastal upwelling ecosystem influenced by freshwater discharges (Arauco Gulf). In addition, the response of these phytoplankton communities to elevated pCO2 levels is studied using CO2 manipulation experiments. The objective of this study was 2-fold: (1) to evaluate the relationship between the seasonal variability of the seawater chemistry and the phytoplankton community at two contrasting coastal ecosystems, and (2) to study the response of these communities to elevated pCO2 levels. Our hypothesis was that phytoplankton communities inhabiting highly variable, naturally acidic coastal ecosystems, such as estuaries, river-influenced areas and/or coastal upwellings, are adapted to high pCO2 conditions.
2. Methods
2.1. Description of Study Sites
In this study we investigated two coastal ecosystems off Central Chile which experience naturally high and variable pCO2 conditions: The river-influenced coastal upwelling area off Concepción, in the northern part of the Arauco Gulf (36° 42–58′ S, 73°12–17′ W), and the Valdivia River estuarine system (39°46′–53′ S, 73°25′ W) (Figure 1). The Arauco Gulf is an equatorward facing embayment characterized by intense seasonal upwelling events between September and March (austral Spring-Summer) caused by an increase in the southwesterly wind stress (Sobarzo et al., 2007; Letelier et al., 2009). During these events, surface waters over the continental shelf edge are typically supersaturated in CO2, resulting in strong across-shore pCO2 gradients (Torres et al., 2011). In its northern part, the Arauco Gulf is influenced by freshwater discharges from the Biobío River, whose plume typically moves southward within the bay. Average flow rates of this river range between 200 and 3,000 m3 s−1 (Valle-Levinson et al., 2003), with maximum values observed during the rainy season in winter (June-August) and minimum values during summer (January-March). Likewise, Biobío River runoff constitutes a significant source of nutrients (silicate, nitrate and phosphate), trace metals and dissolved organic and inorganic carbon (DOC and DIC) for the adjacent coastal ocean (Pérez et al., 2015; Vargas et al., 2016). The Valdivia River estuary, in turn, constitutes one of the most important estuarine systems from central-southern Chile in terms of mean area and freshwater discharges. Hydrographic conditions reveals that it is a partially mixed type estuary, widely affected by tidal cycles (Pino et al., 1994). It presents a seasonal rainfall regime, with an annual average freshwater discharge of 592 m3 s−1 and maximum and minimum flow rates in austral winter (July) and summer (March), respectively (Garcés-Vargas et al., 2013).
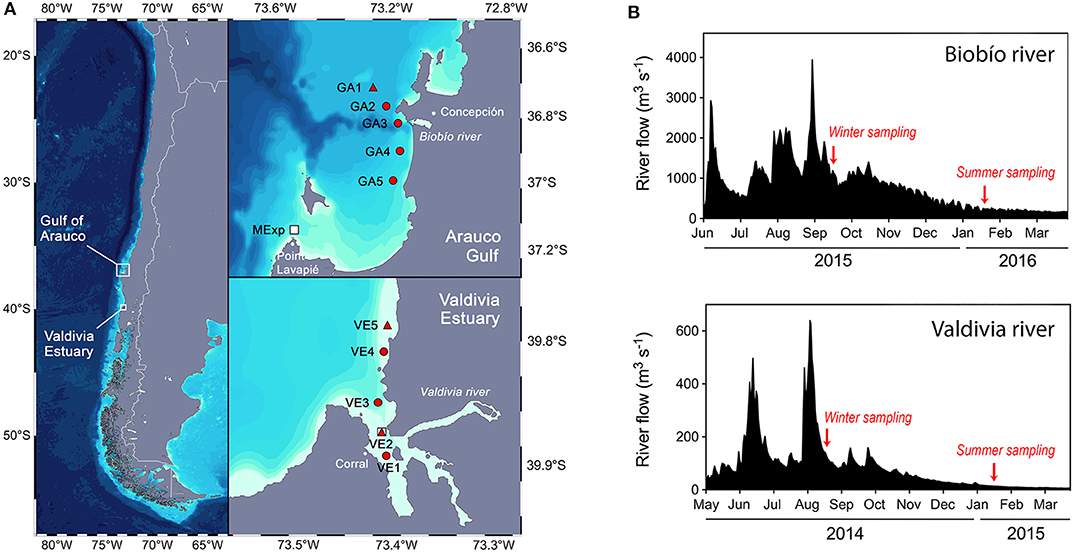
Figure 1. (A) Along-shore transects with the stations position at the two study areas off Central Chile, the Arauco Gulf (GA) and the Valdivia river estuary (VE). Hydrographic and chemical parameters as wells as phytoplankton abundance were measured at all stations. Primary productivity and community respiration were determined at two selected stations in each sampling site (red triangles): one station was located closer to the river mouth, showing a higher influence of fresh water discharges (GA3 and VE2), whereas the other station was located out of this influence (GA1 and VE5). This sampling was repeated in winter and summer at GA (2015–2016) and VE (2014–2015). Squares represent the location where the water was collected for the pCO2- mesocosms experiments, i.e., Lavapié Point (MExp) and VE2. (B) River discharges from the Biobío River (top) and the Valdivia river (bottom) for the year of the corresponding samplings. Red arrows indicates the day of the samplings at each site.
In this study, we conducted four sampling campaigns between 2014 and 2016, both during austral winter and summer; two in the Valdivia River estuary (August 2014 and January 2015) and two in the Arauco Gulf (September 2015 and January 2016). Each sampling was accomplished in 1 day and consisted in an along-shore transect of five stations (Figure 1), covering areas influenced by fresh-water discharges and areas without this influence.
2.2. Sampling and Hydrographic Measurements
The hydrological information on daily river flows were obtained from the Dirección General de Aguas (www.dga.cl) of the Chilean Ministry of Public Work. Seasonal variability in the freshwater discharges of the Biobío and Valdivia Rivers during the sampling years is shown in Figure 1. During each campaign, the water column was characterized through vertical profiles of temperature, salinity and oxygen recorded using a SeaBird SBE-19 plus conductivity-temperature-depth (CTD) equipped with a Westar fluorometer. Water samples for chlorophyll a (Chl a; 200-300 mL), nutrients [, , , and Si(OH)4; 25 mL], DIC and the δ13C isotopic signature of DIC (40 mL), as well as for phytoplankton abundance (250 mL) were collected with 5 L Niskin bottles at 2, 10, 25, and 50 m (only at GA3 and GA5) in the Arauco Gulf, and at 1, 3, and 5 m (plus two additional depths at 8 and 10 m only at VE4 and VE5) in the Valdivia River estuary. In addition, samples for Total Alkalinity (TA) in the Arauco Gulf and for pH in the Valdivia estuary were obtained at the same sampling depths, which were used to estimate both the pCO2 and the saturation state of aragonite (Ωarag) (see below). Chl a samples were directly filtered through GF/F (total Chl a concentration), and stored at −20°C until analysis in the laboratory by fluorometry (Turner Design TD-700) according to Parsons et al. (1984). Water samples for nutrients analysis were pre-filtered (GF/F), frozen (−20°C) and subsequently analyzed following (Strickland and Parsons, 1968).
Gross primary productivity (GPP) and community respiration (CR) was estimated at two stations in each study area (Figure 1), both during winter and summer campaigns. One station was selected at the inner part of the Valdivia River estuary (VE2) and another one offshore in Corral Bay (VE5), at 20 km from the coast. Similarly, in the Arauco Gulf, one station was located inside the Biobío river plume (GA3) at 4 km from the coast, and the other one northward outside the influence of this river plume (GA1), at ~40 km from the coast. In situ incubations to determine GPP and CR were conducted at two depth levels (1 and 3 m) in the Valdivia River estuary and at three depth levels within the euphotic zone (2, 10, and 15 m) in the Arauco Gulf. Discrete-depth measurements of GPP and CR were integrated to 5 and 20 m depths (in Valdivia estuary and the Arauco Gulf, respectively) using the trapezoidal approximation.
Finally, two additional water samplings were carried out to perform the carbonate system manipulation experiments with phytoplankton assemblages from both coastal upwelling and estuarine ecosystems. In the Valdivia River estuary, about 70 L of surface water was collected using 10 L-Niskin bottles at the inner part of the estuary (VE2) in August 2014. Here, the CO2 manipulation experiments were conducted at the Coastal Station of Calfuco (Universidad Austral de Chile, 39° 78′ S, 73° 39′ W). In the Arauco Gulf, the sampling took place in November 2016 at the upwelling center off Lavapié Point (37° 08′ S, 73° 34′ W). A similar water volume (~70 L) was collected using a suction pump in clean plastic containers and was immediately transported to the mesocosm facilities at the Marine Biological Station of Dichato (Universidad de Concepción, 36° 33′ S, 72° 39′ W). Major physical-chemical parameters, such as temperature, salinity, nutrient concentration and CO2 system parameters (i.e., DIC and pH), as well as Chl a concentration and phytoplankton abundance were likewise determined during these field collections in order to have the in situ conditions of the experimental water.
2.3. Analysis and Estimation of the Carbonate System Parameters
All the parameters were analyzed according to Riebesell et al. (2010). Seawater pH was measured potentiometrically in a 25 ml-thermostatted cell at 25 ± 0.1°C using a Metrohm 713 pH meter (input resistance >1,013 Ohm, 0.1 mV sensitivity, and nominal resolution 0.001 pH units) with a glass combined double junction Ag/AgCl electrode (Metrohm model 6.0219.100), calibrated with 8.089 Tris buffer (25°C) according to DOE (1994). pH values are given on the total hydrogen scale (pHT). For DIC and its δ13C isotopic measurements, a subsample of 40 ml was collected with a sterile syringe, filtered through a GF/F filter (previously pre-combusted at 450°C for 4–5 h) directly into 40 ml glass 200 Series I-CHEM® vials and poisoned with a saturated solution of HgCl2 to stop biological activity. The septa of the vials were substituted by butyl rubber septa to prevent diffusion of CO2 (DOE, 1994). Samples were run on an OI Analytical total inorganic carbon-total organic carbon (TIC-TOC) Analyzer (Aurora Model 1030), first run to determine the parts per million carbon organic/inorganic concentration and then for the δ13C isotopic signature. The TIC-TOC analyzer was interfaced with a Finnigan Mat DeltaPlus isotope ration mass spectrometer for analysis by continuous flow. Data were normalized using internal standards. The analytical precision was 2% for the quantitative DIC measurements and ±0.2o for the isotopes. All the analysis were conducted in the G. G. Hatch Stable Isotope Laboratory at the University of Ottawa, Canada. Samples for total alkalinity (TA) were collected in 250 ml- glass bottles, fixed with a saturated solution of HgCl2, and subsequently measured in the laboratory by potentiometric titration with HCl following the closed-cell method described in DOE (1994).
pH and DIC values (Valdivia estuary), and TA and DIC values (Arauco Gulf) were used in the software CO2SYS (Pierrot et al., 2006) to calculate the rest of the carbonate system parameters (i.e., pCO2, TA and pHT in Valdivia estuary and Arauco Gulf, respectively) and the Ωarag. Temperature, salinity, and nutrient [ and Si(OH)4] data were likewise included in these calculations. The carbonic acid dissociation constants (K1 and K2) were taken from Mehrbach et al. (1973) as refit by Dickson and Millero (1987) for marine waters (salinity >30 psu) and those by Millero (2010) for estuarine waters (salinity between 1 and 30 psu). The KHSO4 equilibrium constant determined by Dickson (1990) was applied for all calculations.
2.4. Phytoplankton Community Structure, Gross Primary Production (GPP), and Community Respiration (CR)
Phytoplankton samples for microscopy were fixed with 2% acidic Lugol solution and analyzed following the Utermöhl technique (Utermöhl et al., 1958), with classification until the lowest possible taxonomical level, using an Olympus IX51 microscope. GPP and CR were estimated from changes in the dissolved oxygen (DO) concentrations after incubating BOD bottles at in situ light conditions and in the dark (Strickland, 1960). At all samplings, the incubation time was <12 h and 3–4 replicates of each light condition (i.e., in situ and dark) were run. DO was determined before and after the incubation using the OxyMini® optode system (WPInst.). Net community production (NCP) was calculated as the difference in the DO concentration between light (GPP) and dark (CR) BOD bottles after the incubation.
2.5. Carbonate System Manipulation Experiments
Once in the laboratory, the seawater collected at each sampling site for the experiments was pre-filtered through a 200 μm mesh to eliminate large zooplankton. After acclimation to the corresponding pCO2 treatment for 12 h (Valdivia estuary) or 22 h (Arauco Gulf), seawater was transferred to polycarbonate 20L- bottles to start the experiments. Semi-automatic mesocosm systems for seawater carbonate chemistry manipulation of similar characteristics were employed at the two locations. The different pCO2 treatments were attained by continuously bubbling the seawater with either ambient (low pCO2 treatment) or CO2-enriched air (high pCO2 treatment). Air/CO2 mixtures were produced using a bulk technique, based on mixing filtered, dry air with ultra pure CO2 at a known flow rate using Mass Flow Controllers (MFC; Aalborg Model GFC). In the Valdivia River estuary the low pCO2 level was set at ~380 μm (control level, IPCC 2014), whereas in the Arauco Gulf the low pCO2 level was set at 700 μm corresponding to the in situ value during field sampling (i.e., “control” treatment). For the estuarine community, the high pCO2 treatment was ~900 μm whereas, for the coastal upwelling area, we considered an experimental treatment of 1600 μm pCO2. The upwelled water was already CO2-saturated (700 μm pCO2), and therefore we assumed a higher ΔCO2 of around 900 μatm. In both cases, these high pCO2 levels can naturally occur in these ecosystems (Vargas et al., 2017). Four replicates per treatment were run during the experiments, that lasted for 5 days and were conducted under temperature controlled conditions and 12/12 days/night light regime. The water inside the incubation bottles was gently mixed using rotor-powered blades, in order to prevent sedimentation of the phytoplankton cells. Subsamples for nutrients, carbonate system parameters, chlorophyll a and phytoplankton abundance were collected every day (except nutrients in the Arauco Gulf, which were sampled in situ, and on days 2 and 4 during the experiments) and analyzed as stated above.
2.6. Statistical Analyses
Pairwise comparisons were conducted applying the Student t-test and were considered significant at p < 0.05. The phytoplankton community was characterized using the Shannon-Wiener Diversity index (H'), Margalef's species richness (d), and Pielou's evenness index (J0) at each station and sampling time, using logarithms to the base 2 in the calculation of H′. It should be noted that these indices are not true species-level diversity indexes as some of the organisms could only be identified to the genus level (e.g., Chaetoceros spp. and Thalassiossira spp.). The correlation between environmental and biological variables was addressed by means of the Spearman correlation coefficient, which were considered significant at p < 0.05. Furthermore, a Generalized Linear Model (GLM) was applied to significantly correlated variables to evaluate the dependence between the biological variables and the environmental factors at each study site.
3. Results
3.1. Hydrography
Clear seasonal differences in the hydrographic conditions were observed at the two sampling sites. The Biobío and Valdivia rivers discharges showed a typical annual pattern during the sampling years, with maximum values in winter and minima in summer (Figure 1B). Accordingly, fresh water influence was higher in winter at both areas, although in Valdivia, almost the entire water column at the inner estuarine stations was dominated by riverine waters (VE1-VE3, Figure 2) whereas in the Arauco Gulf this influence was limited to the surface waters in the southernmost stations (GA3–GA5, Figure 3). The water column along the transects was well-mixed and characterized by low temperatures (11–12°C) and oxygenated waters (> 3 ml L−1) during this season. In summer, in contrast, the warming of the surface waters caused the stratification of the water column, with temperatures decreasing 9°C in the upper 5 m in the Valdivia estuary (Figure 2) and 4°C in the upper 20 m in the Arauco Gulf (Figure 3). In the Valdivia estuary, the reduced river flow during this season limited the influence of freshwater to the first 4 m at the inner part of the estuary (VE1-VE3), whereas the influence of more oceanic waters characterized by higher nutrient levels (both + and ) increased. In the Arauco Gulf, the low oxygen values (<1 ml L−1) together with the high concentrations below 20 m showed the influence of an upwelling of oxygen-poor nutrients-rich subsurface waters in summer, especially at stations GA1, GA2, and GA5 (Figure 3). However, + concentration did not follow the same pattern as the concentrations in summer were lower than in winter, which may be attributed to an enhanced nitrogen uptake by phytoplankton during the spring-summer blooms. Si(OH)4 concentrations, in turn, were significantly higher in Valdivia than in the Arauco Gulf (t-test, p < 0.05) but were associated with freshwater inputs at both sites, with maximum values at the Biobío river mouth (GA3) in the Arauco Gulf and at the inner estuarine stations (VE1–VE3) in Valdivia.
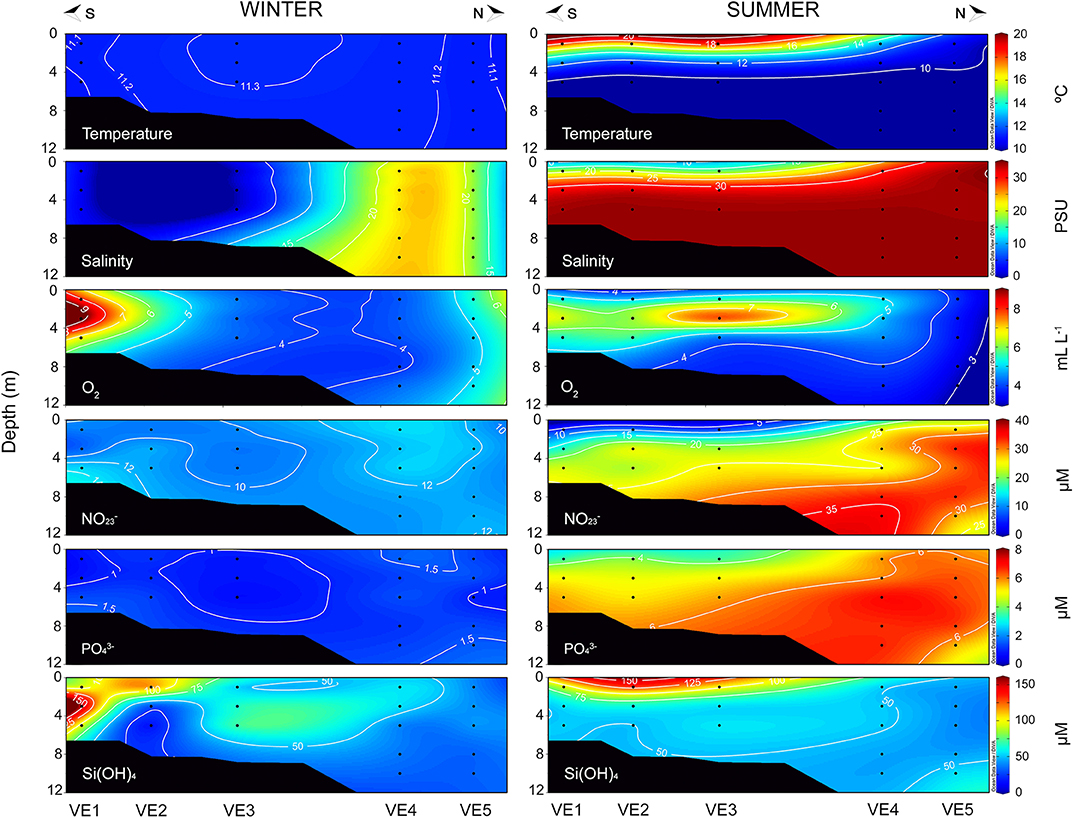
Figure 2. Hydrographic characterization of temperature, salinity, oxygen (O2), and nutrients [ plus , , and Si(OH)4] along the transects sampled at the Valdivia river estuary during winter 2014 (left) and summer 2015 (right).
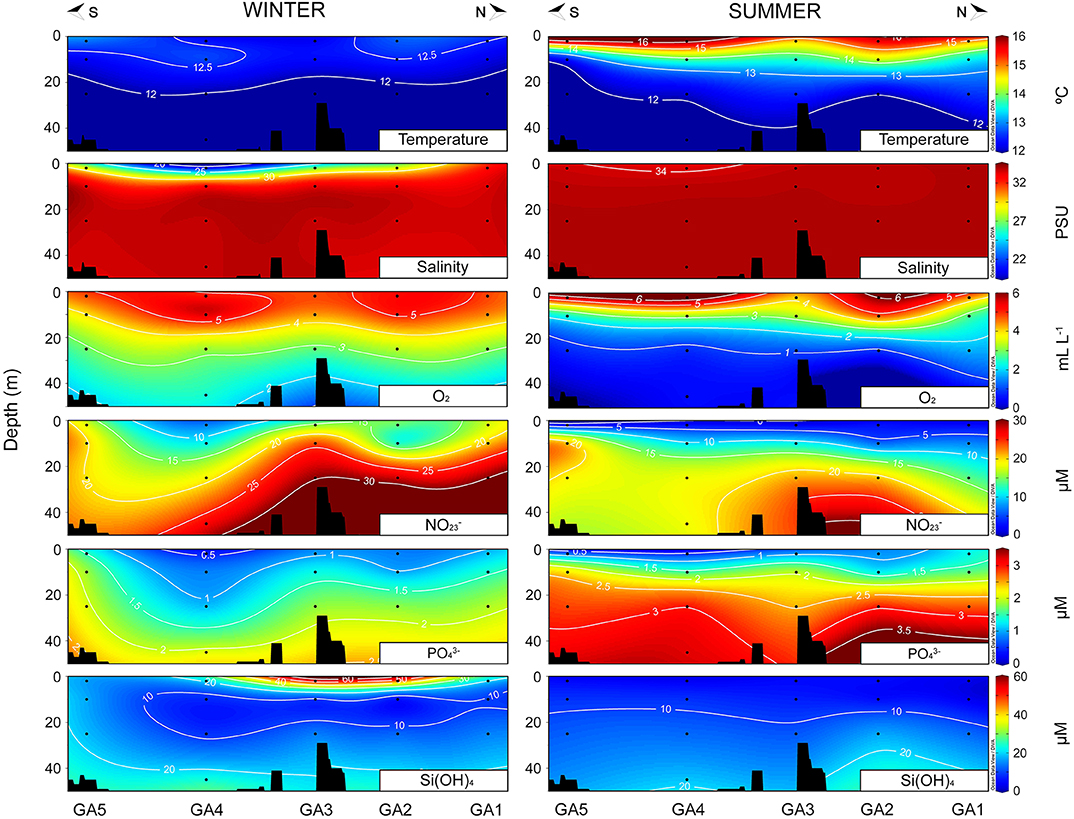
Figure 3. Hydrographic characterization of temperature, salinity, oxygen (O2), and nutrients [ plus , , and Si(OH)4] along the transects sampled at the Arauco Gulf during winter 2015 (left) and summer 2016 (right).
3.2. Carbonate System Dynamics at Two Coastal Ecosystems
As with the hydrographic conditions, carbonate system parameters showed marked seasonal differences. The dynamics of the carbonate system in the Valdivia estuary were driven by the interplay between predominant freshwater discharges in winter and the influence of more oceanic waters in summer (Figure 4). In winter, the water column at the inner part of the estuary (VE1-VE3) was characterized by low DIC concentrations (<1,250 μmol kg−1), low TA (<1,700 μmol kg−1), low pCO2 levels (<300 μatm), and corrosive conditions (Ωarag <1), due to low alkalinity/salinity conditions associated to high freshwater inputs from the Valdivia river (Figure 1B). Depleted δ13CDIC values indicated the contribution of riverine DIC to the total pool of DIC in this estuarine portion. The pHT values showed intermediate values during the winter campaign, ranging between 7.60 and 7.99 units. In summer, however, the pHT ranged from as low as 7.48 at the stations outside of the river plume (i.e., VE4 and VE5) to as high as 8.03 at the surface waters of VE1 and VE3 stations. The influence of oceanic waters extended from the coastal stations to the inner portion of the estuary during this season, being characterized by low pHT, and high DIC concentrations (>1,700 μmol kg−1), TA values (>2,000 μmol kg−1), and pCO2 levels (>700 μatm) (Figure 4).
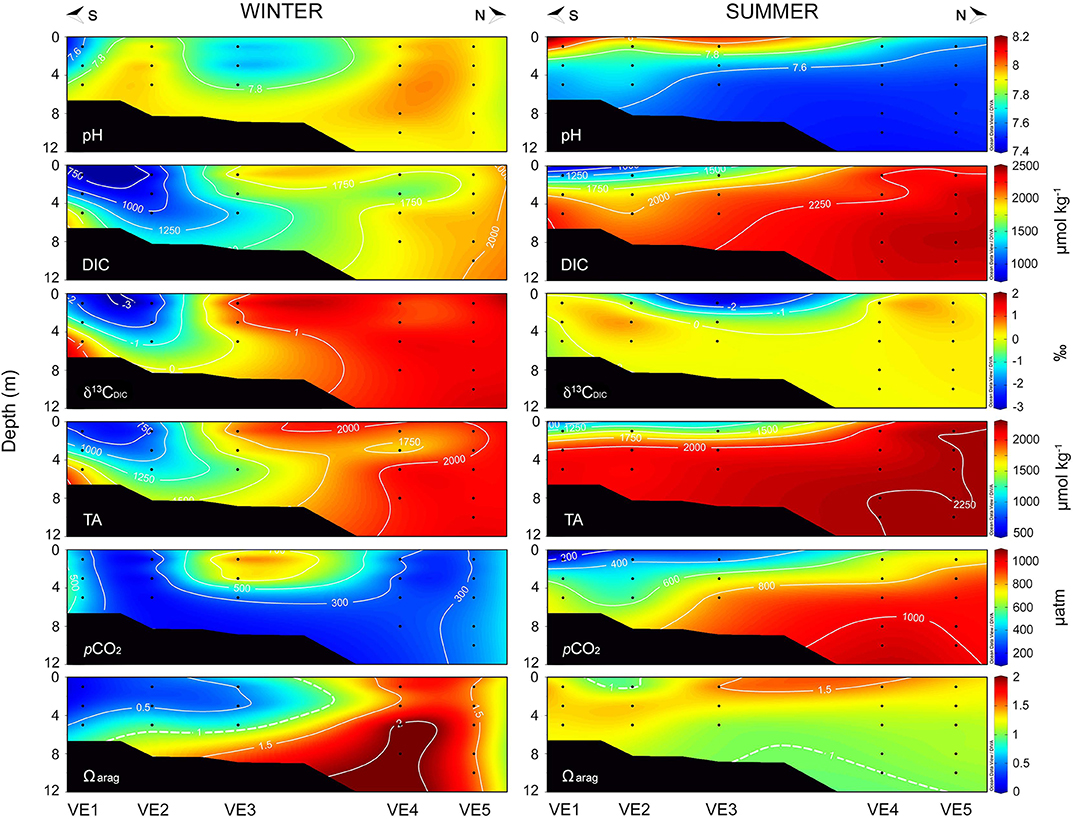
Figure 4. Carbonate system parameters along the transect conducted in the Valdivia river estuary in winter (left) and summer (right). From the top to the bottom: pH, dissolved inorganic carbon (DIC), its δ13C isotope (δ13CDIC), total alkalinity (TA), partial pressure of CO2 (pCO2), and the saturation station of aragonite (Ωarag). Dashed line in Ωarag plot represents the isopleth of 1.
In the Arauco Gulf, the highest pHT values were recorded at the surface waters in winter, ranging between 8.19 and 8.56 units (Figure 5), while the lowest DIC concentrations (1535 μmol kg−1) and TA levels (1,731 μmol kg−1) for this area were determined at the river mouth station GA3, associated with higher freshwater inputs from the Biobío river during this season (Figure 1B). However, the riverine DIC contribution to the DIC pool in the Arauco Gulf was not evident from the δ13CDIC values. The pCO2 levels remained relatively low (<300 μatm) throughout most of the transect in winter and Ωarag values revealed supersaturation conditions with respect to aragonite. In summer, in contrast, all the carbonate system parameters (except the TA, which showed a relatively constant value of 2,298 ± 8 μmol kg−1 along the transect) reflected the upwelling of acidic and corrosive subsurface waters mainly at stations GA1, GA2, and GA5 (Figure 5). These subsurface waters were also characterized by low pH values (<7.9 units), high DIC concentration (>2,100 μmol kg−1), high pCO2 levels (>700 μatm), and Ωarag undersaturation conditions (<1), as compared to other stations (i.e., GA3 and GA4) and season (i.e., winter). δ13CDIC values also evidenced the influence of fractionated DIC pool (<1o) associated to low oxygen and corrosive subsurface waters.
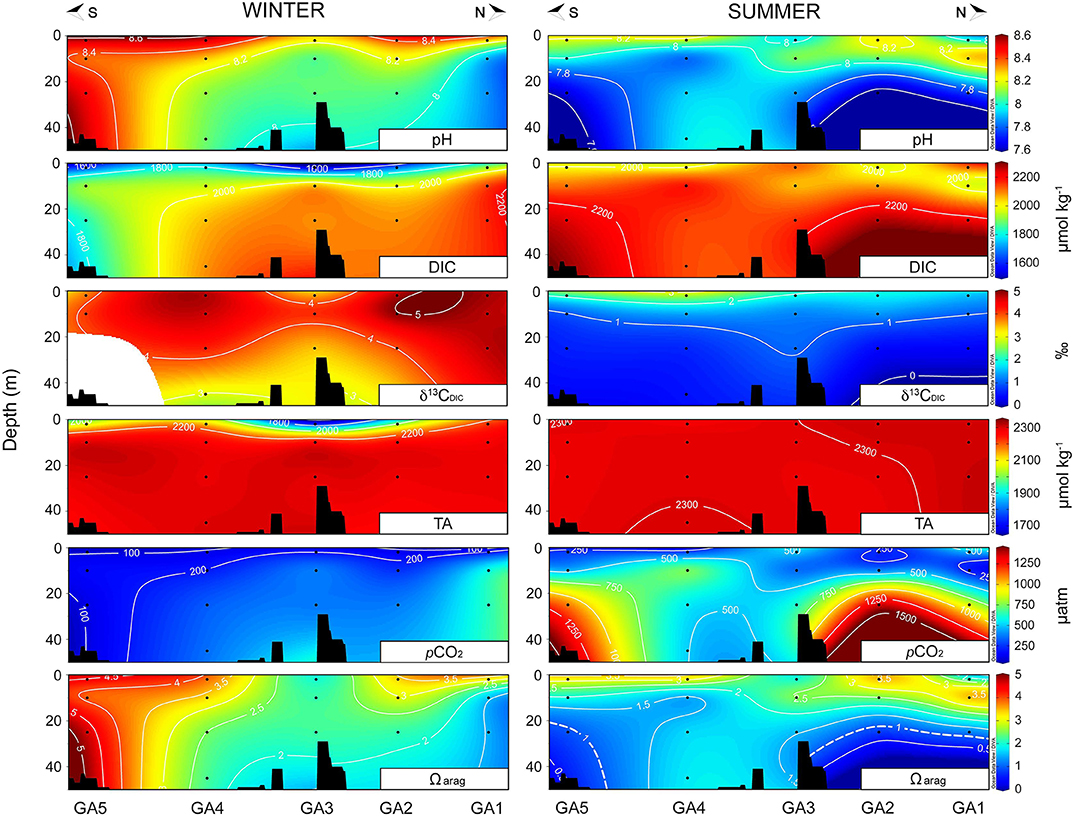
Figure 5. Carbonate system parameters along the transect conducted in the Arauco Gulf in winter (left) and summer (right). From the top to the bottom: pH, dissolved inorganic carbon (DIC), its δ13C isotope (δ13CDIC), total alkalinity (TA), partial pressure of CO2 (pCO2), and the saturation station of aragonite (Ωarag). Dashed line in Ωarag plot represents the isopleth of 1.
In general terms, the more acidic waters (low pH/ high pCO2) were observed in summer at both the Arauco Gulf and the Valdivia estuary, caused by the influence of subsurface upwelled waters and coastal oceanic waters, respectively. However, in contrast to the Arauco Gulf, the more corrosive conditions Ωarag(< 1) in the water column at the Valdivia estuary were recorded in winter, coinciding with the larger freshwater inputs, which are characterized by a reduced buffering capacity associated to the low TA values (Figure 5, Figure S1).
3.3. Temporal and Spatial Variations of the Phytoplankton Community
Seasonal differences in the Chl a concentration and the abundance of the dominating phytoplankton groups were larger than spatial variations along the transects at both samplings sites. Vertical profiles in the Valdivia estuary showed low Chl a concentrations (<2.5 μg L−1) in winter at all stations and depths (Figure 6), with integrated values (over the first 5 m) ranging between 5.3 and 8.4 mg m−2 (Table 1). In summer, this concentration increased at all stations but mainly at the inner estuarine section (VE1–VE3), where it achieved maximum values between 7 and 18 μg L−1 at subsurface waters and integrated values in the water column of 44.6–65.5 mg m−2 (Table 1). Similarly, the cell abundance of the different phytoplankton groups was about one order of magnitude larger in summer (Figure 6), even though the community was more diverse and the species richness was higher in winter (Table 1). Diatoms were the most abundant group throughout the year, contributing between 50 and 94% to the total abundance (Table S1), although there was a seasonal change in the dominant species. In winter, Aulacoseira granulata and Thalassionema nitzschioides accounted for most of the phytoplankton abundance, whereas in summer Chaetoceros spp. and Skeletonema costatum dominated the water column. A distinct distribution of less abundant groups was also observed along the transect. For instance, chlorophytes were only collected at the inner estuarine stations (Figure 6), where they were relatively abundant in winter (14–29%, Table S1). Other groups, such as dinoflagellates were present throughout the entire water column, at all stations and samplings, but their relative contribution to the total community changed likely associated to the influence of more oceanic waters as it was higher both at VE4 and VE5 stations and in summer all along the transect (between 10 and 36%, Table S1).
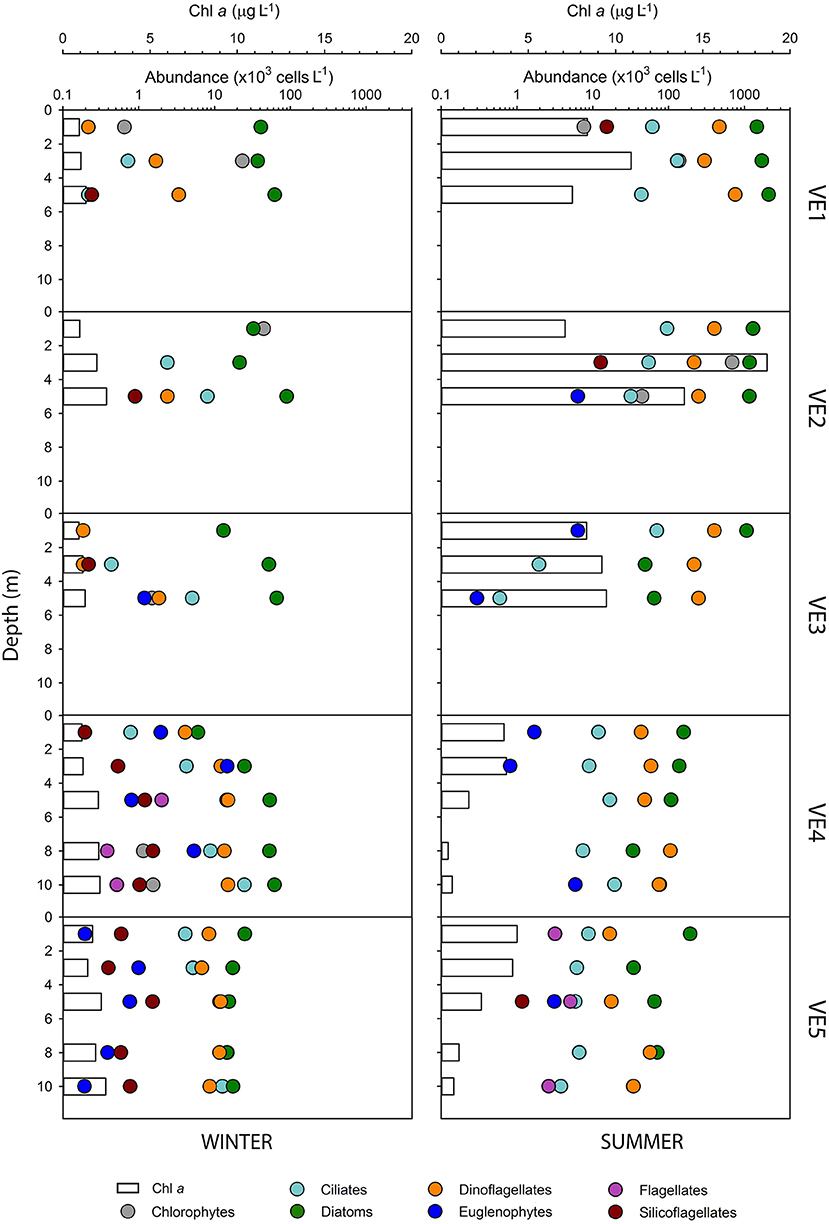
Figure 6. Cell abundance of the phytoplankton groups (dots) dominating the community in the Valdivia river estuary during winter (left) and summer (right). Note that the abundance is given in log-scale. The bars represents the chlorophyll a concentration at each station and during each seasonal sampling. The legend at the bottom applies to all the panels.
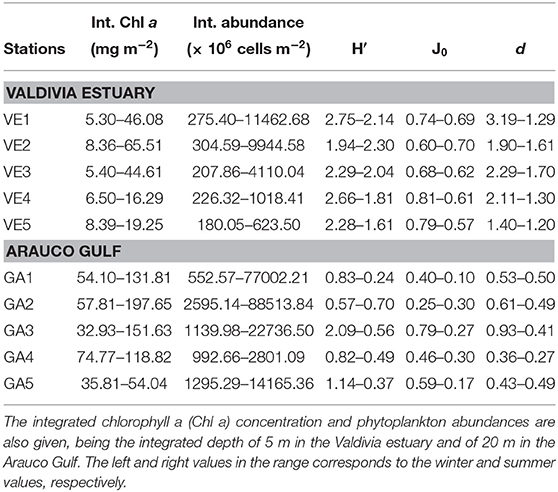
Table 1. Phytoplankton community biodiversity and species richness through the Shannon-Wiener diversity index (H′), Pielou's evenness index (J0), and Margalef's species richness (d) at both study areas.
In the Arauco Gulf the seasonal patterns of Chl a concentration and the cell abundance were similar as in Valdivia, being higher in summer than in winter (Figure 7). However, the latter was one order of magnitude higher in the Arauco Gulf during the same season, with maximum values reaching more than 3 × 106 cells L−1 at surface waters at GA2 (Figure 7) and integrated values (over the first 20 m) of 88.5 × 109 cells m−2 (Table 1). According to the diversity and species richness indexes (Table 1), the plankton community in this coastal ecosystem was less diverse and showed lower species richness in summer, but it was strongly dominated by diatoms throughout the year (relative abundance between 91 and 99%, Table S2). As in Valdivia, there was a seasonal change in the species composition at each station. Chaetoceros spp. was the most abundant group at stations GA1 and GA2 in winter (80–86%), whereas the same genus dominated the phytoplankton community at stations GA4 and GA5 in summer (88–82%, Table S2). The northernmost stations (GA1–GA3) were dominated by the chain-forming diatom Leptocylindrus danicus in summer. It is noteworthy that GA3, the station located at the Biobío river mouth (Figure 1), presented the highest diversity and species richness values in winter, not showing a clear dominance of any species or genera (Table S2). The contribution of dinoflagellates was low in this area both in winter and summer, ranging from 0.2 to 8.9%, with maximum integrated cell abundances of 35.6 × 107 cells m−2 at GA3.
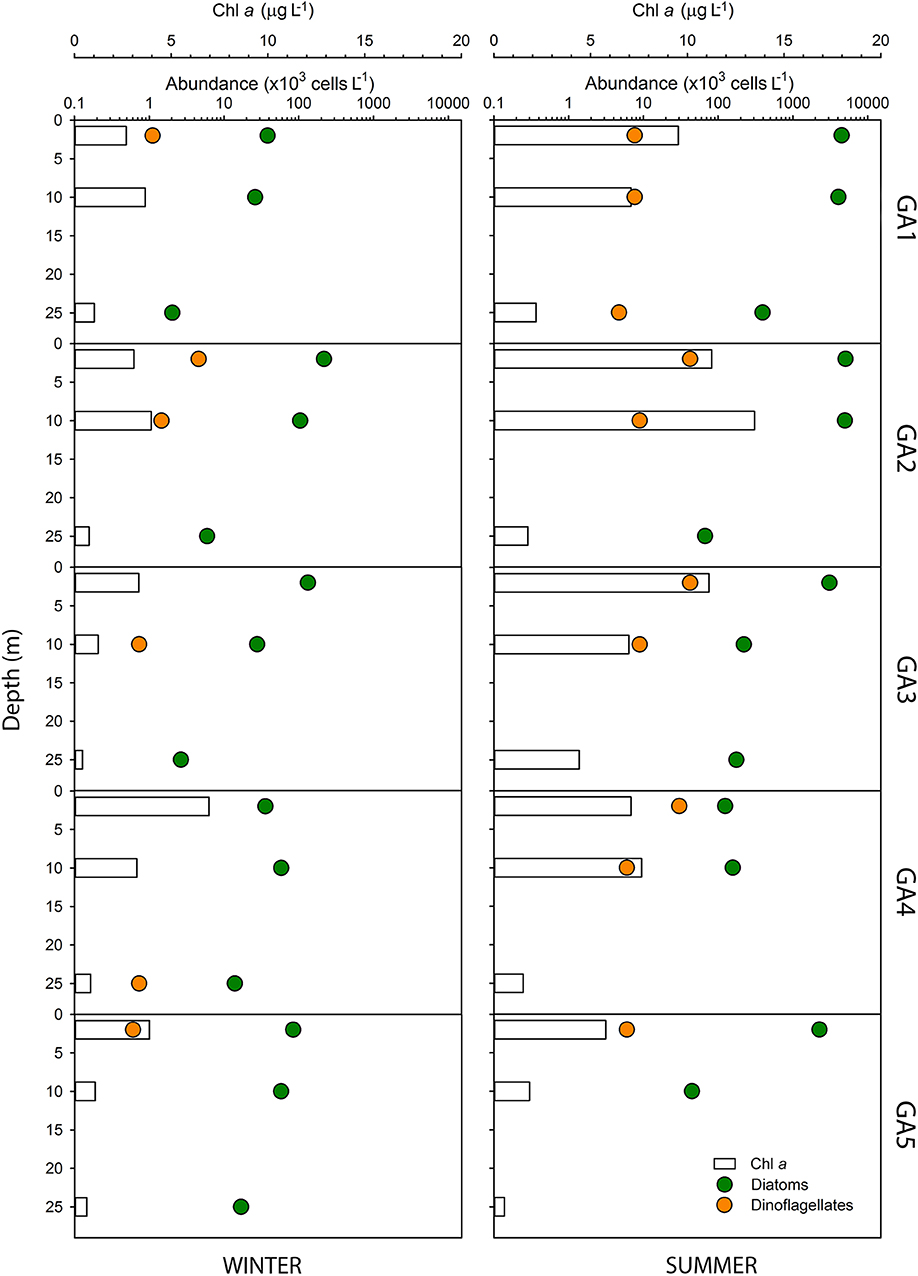
Figure 7. Cell abundance of the phytoplankton groups (dots) dominating the community in the Arauco Gulf estuary during winter (left) and summer (right). Note that the abundance is given in log-scale. The bars represents the chlorophyll a concentration at each station and during each seasonal sampling. The legend in the lower panel applies to all the panels.
As expected, the largest gross primary production rates (GPP) were recorded during summer campaigns at both study sites (Table 2), with values being one order of magnitude higher (>4,000 mg C m−2 d−1) in the Arauco Gulf than in the Valdivia River estuary. Seasonal differences in the community respiration (CR) were observed between sites as well. In Valdivia, the highest CR levels were determined at the inner estuarine stations in winter. In the Arauco Gulf, in contrast, the highest values were measured in summer at the more oceanic station (GA1), coinciding with the highest GPP rates supported by the upwelling of nutrient-rich subsurface waters (Figure 3). The GPP/CR ratio showed a similar trend at the two coastal ecosystems (Table 2), with significantly lower values recorded during winter campaigns (t-test, p < 0.05).
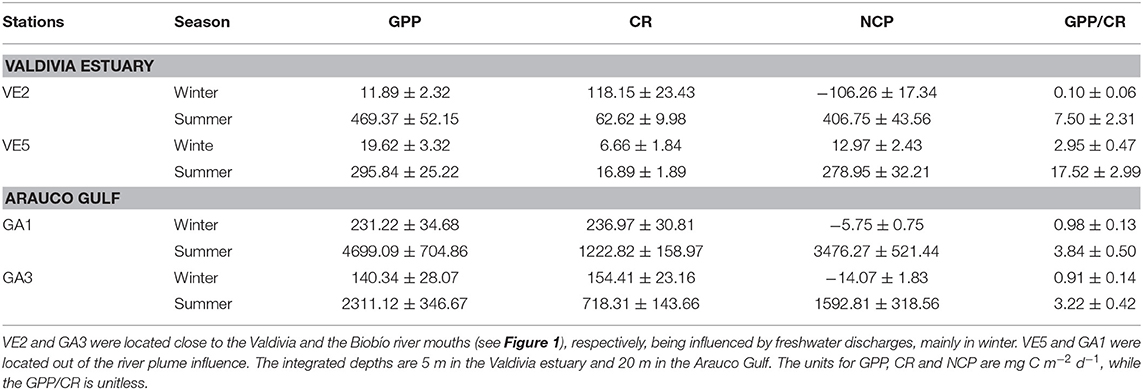
Table 2. Integrated gross primary productivity (GPP), community respiration (CR), net community production (NCP), and the GPP/CR ratio at two station of the Valdivia estuary and the Arauco Gulf during winter and summer.
3.4. Relationship Between Environmental and Biological Variables
Several significant correlations were obtained between the structure and physiological rates of phytoplankton communities and the abiotic factors, such as the hydrographic properties and the carbonate system parameters. Tables 3, 4 includes these correlations as well as the results of the GLM model applied to elucidate the role of each environmental factor in predicting the biological variables in Valdivia and the Arauco Gulf, respectively. We observed distinct relationship of the environmental and biological factors between the two sites.
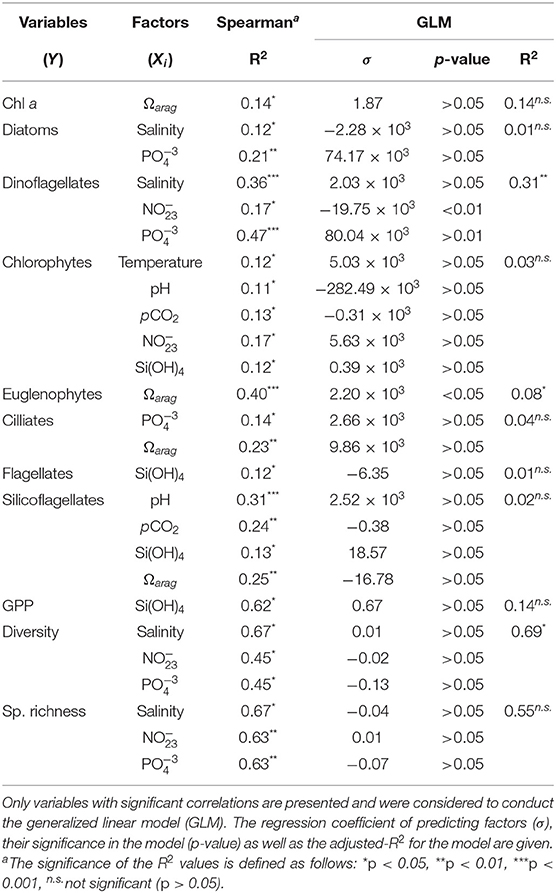
Table 3. Relationship between biological variables (Y) and environmental factors (Xi) at the Valdivia river estuary.
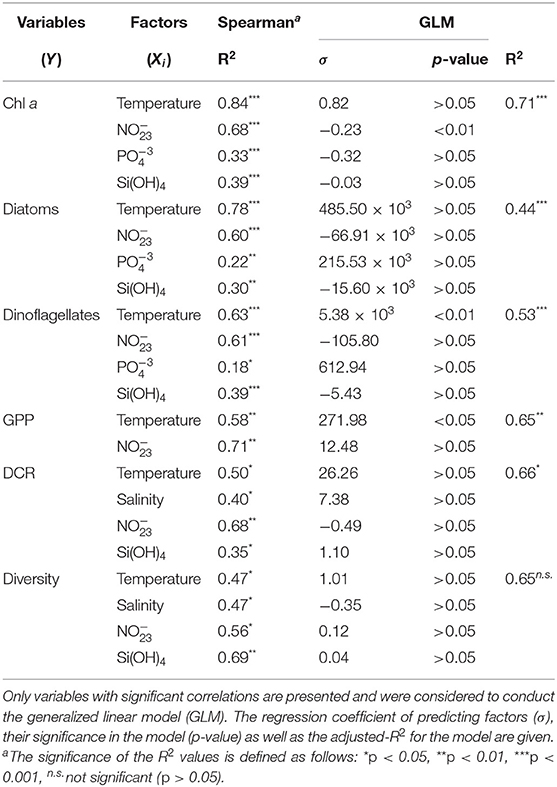
Table 4. Relationship between biological variables (Y) and environmental factors (Xi) at the Arauco Gulf.
In the Arauco Gulf, the variability of the Chl a concentration, the abundance of the different taxonomic groups and the physiological rates were largely explained (44–71%) by variations in temperature and nutrients concentration (Table 4). None of the carbonate system parameters was significantly correlated to the considered biological variables in this ecosystem. In contrast, in the Valdivia estuary, the carbonate chemistry in the water column showed a significant role in modulating the abundance of certain taxonomic groups. For instance, change in the Ωarag values explained 14% of the variance in the Chl a concentration and 23–40% of the seasonal variability observed in the abundance of euglenophytes, cilliates and silicoflagellates (Table 3). The abundance of diatoms and dinoflagellates, in turn, was correlated to the salinity and nutrients concentration, even though the multivariate linear approach considering these factors was only able to explain significantly the variance in dinoflagellates (31%).
3.5. Response of Coastal Phytoplankton Communities to Short-Term Exposure to Elevated pCO2 Levels
Seawater parameters during field collection (i.e., in situ) and for the experimental set up are given in Figures 8, 9. It is noteworthy that the pH/pCO2 levels established for the two experimental treatments (low/high) were maintained constant over the 5-days experiments, as reflected by the continuous monitoring of pH and pCO2 values. Natural assemblages of planktonic communities from estuarine and coastal upwelling ecosystems showed distinct responses during the experimental period. In the Valdivia River estuary, the in situ values of pH and pCO2 were of 7.93 and 162 μatm (VE2 in Figure 5, winter sampling), respectively. For the experiment, however, we decided to set the low pCO2/high pH treatment (hereinafter “low treatment”) at 380 μatm, a typical and recommended value for control treatments in CO2 manipulation experiments (IPCC, 2014) and the high pCO2/low pH treatment (hereinafter “high treatment”) at 900 μatm. Both the Chl a concentration and the abundance of phytoplankton groups in general showed an exponential increase during this experiment (Figure 8). Thus, Chl a values in the low treatment, for instance, increased from as low as 1 μg L−1 at initial conditions to as high as 15 μg L−1 in 5 days. No significant differences were detected between the low and high treatments at the end of the incubation (t-test, p > 0.05). The fast increase in the phytoplankton growth rates was reflected in the nutrient ratios (Figure 8). While the Si/N ratio decreased steadily (from ca. 11 to 9), the N/P ratio increased toward the end of the experiments in both treatments (from ca. 9 to 25). In this regard, there was no significant differences in the N uptake rates between the low and high treatments. The phytoplankton community in these estuarine waters was mainly dominated by diatoms (65% of the total abundance), in agreement to that observed under natural conditions (Figure 6). Most of the plankton groups increased their abundance during the incubation at both treatments, except silicoflagellates, which were only present at the beginning of the experiment. Euglenophytes and dinoflagellates showed a moderate increase in both treatments, whereas diatoms and flagellates exhibited the largest increase and were the most abundant groups at the end. When the effect of the elevated pCO2 level was tested after the 5-days incubation, we only observed significant differences (t-test, p < 0.01) in the abundance of diatoms and flagellates, being higher in the low treatment. A detailed examination of the species composition of diatoms revealed that the contribution of less abundant species (<1% of the total abundance, grouped as “Other diatoms,” Figure 8), which corresponded mostly to freshwater diatoms, as well as of Chaetoceros spp. decreased toward the end of the experiment. Conversely, taxa, such as Thalassiosira spp. and Skeletonema costatum increased, mainly at the high pCO2 treatment.
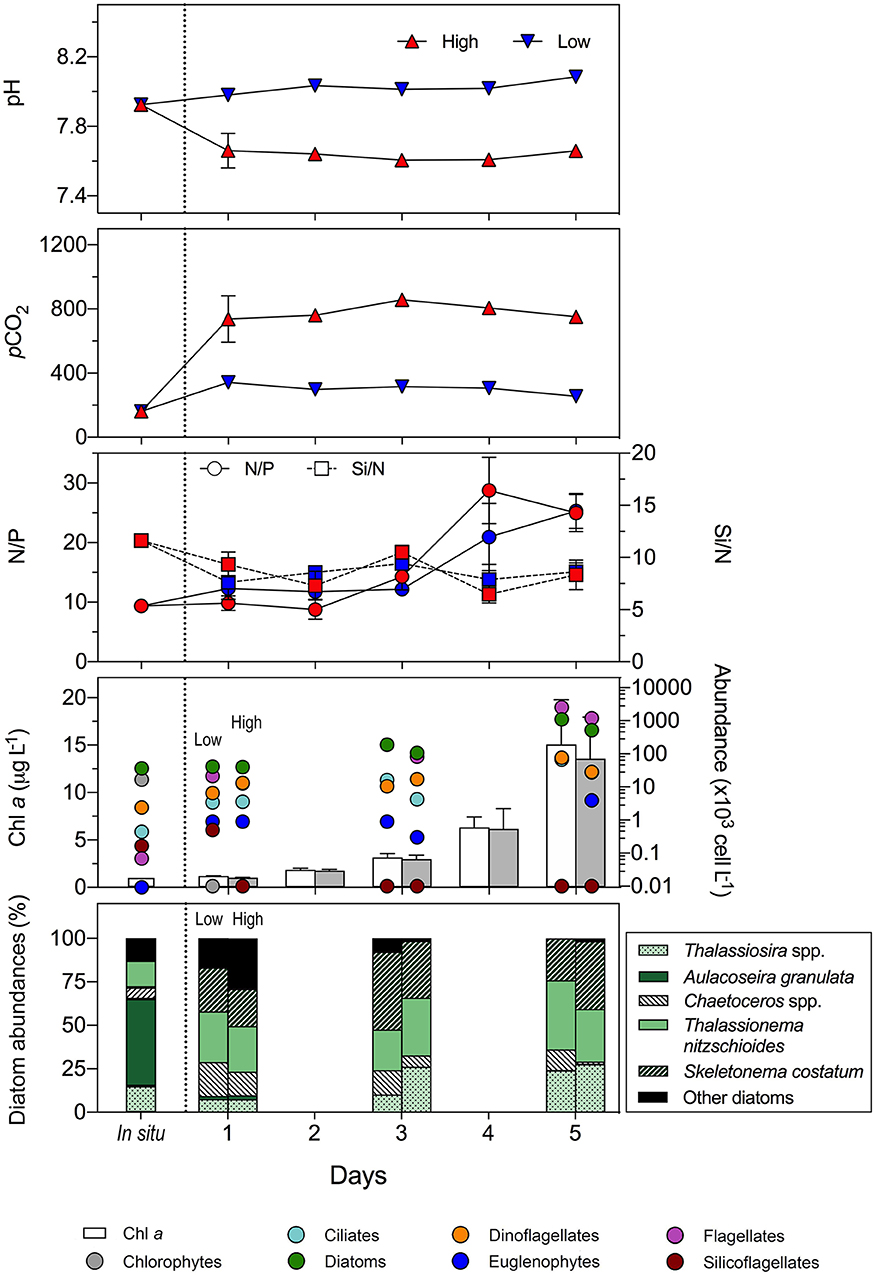
Figure 8. Temporal development of the pH, pCO2 levels, nutrient ratios (N/P and Si/N), chlorophyll a concentration (Chl a), and phytoplankton groups abundance over the course of the mesocosms experiment conducted with the plankton community from the Valdivia estuary (January 2015). The relative contribution of the most abundant (>5%) diatom species is also given. The low and high pCO2 treatments were of 380 μatm (control level) and 900 μatm, respectively. Average values for four independent replicates are given; error bars stand for the standard deviation. The vertical dashed line separates the in situ and the experimental conditions.
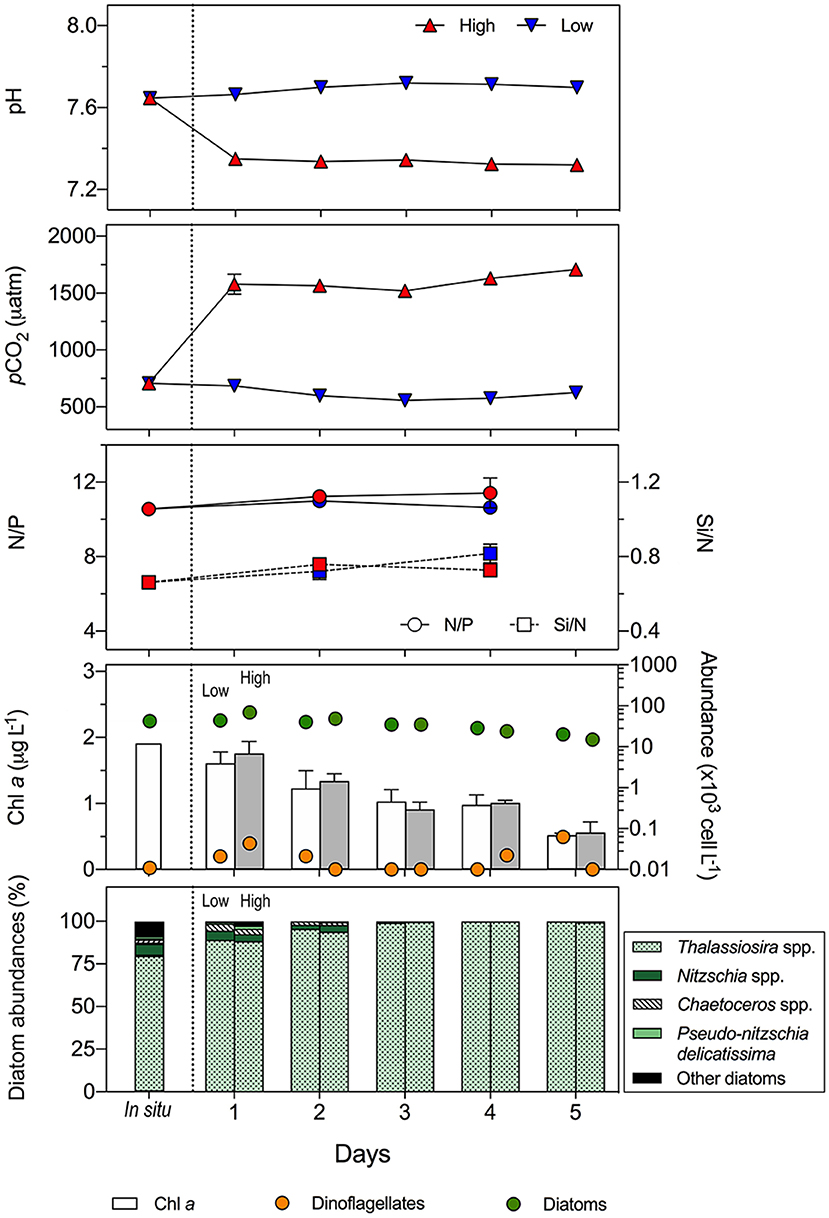
Figure 9. Temporal development of the pH, pCO2 levels, nutrient ratios (N/P and Si/N), chlorophyll a concentration (Chl a), and phytoplankton groups abundance over the course of the mesocosms experiment conducted with the plankton community from the Arauco Gulf (November 2016). The relative contribution of the most abundant (>1%) diatom species is also given. The low and high pCO2 treatments were of 700 μatm (in situ pCO2 level during the sampling) and 1,600 μatm, respectively. Average values for four independent replicates are given; error bars stand for the standard deviation. The vertical dashed line separates the in situ and the experimental conditions.
In the Arauco Gulf, the in situ pH and pCO2 levels were 7.65 and 706 μatm, respectively (Figure 8). These values were considered for the low treatment as a measurement of control conditions in upwelling waters, whereas the high treatment was set at 1,600 μatm of pCO2, considering a Δ pCO2 of 900 μatm with respect to the control conditions. Nutrient ratios N/P and Si/N remained constant at ca. 11 and 0.73, respectively, indicating no significant uptake of N by phytoplankton and no nutrient limitation during the experiment. Likewise, no significant effect of pCO2 levels was observed in the concentration of Chl a, although it decreased from about 2 μg L−1 at in situ and initial values to 0.6 μg L−1 at the end of the incubation. Similarly, the abundance of diatoms decreased with time at both the high and low treatments, although this decrease was two times higher at high pCO2 conditions than at low pCO2 conditions (13.5 × 103 cells L−1 d−1 vs. 5.9 × 103 cells L−1 d−1). This decrease in the cell abundance coincided with an increase in the aggregates formation, as revealed by visual observations of the incubation bottles. The abundance of dinoflagellates, in contrast, did not show any influence of elevated pCO2 levels nor a decrease with time. In this experiment, the diatom community was dominated by Thalassiosira spp. As in the estuarine ecosystem, the less abundant diatom species decreased toward the end of the experiment irrespective of the treatment.
4. Discussion
4.1. The Natural Environmental Variability of Hydrography and Carbonate System for Estuarine and Coastal-Upwelling Phytoplankton Communities
A frequent research gap in OA studies is the lack of information about the natural variability of the seawater chemistry at the locations where the organisms are collected. However, it is increasingly more evident that this knowledge is critical to correctly interpret the outcome of these investigations (e.g., Boyd et al., 2016; Vargas et al., 2017), particularly in studies conducted at highly variable and/or naturally acidic coastal ecosystems. In the present work, we have described the physical-chemical properties of the water column (in terms of temperature, salinity, dissolved oxygen, nutrients concentration and carbonate system parameters) at each study area both during winter and summer, as we expected to record different carbonate chemistry conditions associated to the distinct oceanographic processes dominating the water column during these two seasons.
The seasonal variability in the hydrographic conditions and the phytoplankton community in the Valdivia river estuary and the adjacent coastal area were mostly associated to changes in the extent of the river plume along the transect, which was primarily linked to seasonal changes in the freshwater discharges, as previously described for this area (Vargas et al., 2003; Giesecke et al., 2017). In winter, the low + and concentrations typical for this system (Pérez et al., 2015) together with the limited solar irradiance characteristic at this latitude (Iriarte et al., 2007; González et al., 2010) limited the phytoplankton growth and favored a net heterotrophic system, where the CR exceeded GPP (Table 2). This high CR was likely associated to the consumption of the organic matter transported by the river. Furthermore, as the relative abundance of the microheterotrophic components of the plankton community was not significantly higher during this time (Table S1), the enhanced respiration could be attributed to an increase in the pico- and nanoplankton community associated to freshwater discharges (Iriarte et al., 2018; Cuevas et al., 2019). In summer, the reduction of the river runoff led to a strong stratification in this tidal estuary, with considerable implications for the distribution of Chl a in the water column. The surface waters of lower salinity showed low phytoplankton biomass, increasing within the pycnocline and below (Figure 6), which is a typical pattern observed in other estuaries and fjord areas (Alldredge et al., 2002; Jouenne et al., 2007). Phytoplankton composition along the transect shifted from a freshwater-water species dominated community during winter toward a marine species dominated community during summer, in agreement to that reported by Giesecke et al. (2017).
The hydrographic features in the Arauco Gulf follow a seasonal pattern, with higher upwelling events during austral spring/summer (Valle-Levinson et al., 2003; Sobarzo et al., 2007) and with a considerable influence of freshwater discharges from the Biobío River in winter (Vargas et al., 2016). Furthermore, water column stratification in this region results from the influence of both temperature and salinity (Sobarzo et al., 2007). Accordingly, our results showed that thermal stratification dominated the water column in summer (associated with an increase in the solar irradiance) whereas salinity stratification was stronger in winter, particularly at those stations influenced by the river plume (GA3–GA5, Figure 3). The transport of nutrient-rich subsurface waters to the euphotic zone in summer promoted the phytoplankton growth, reaching Chl a values (>10 μg L−1) common for this coastal region (Iriarte and Bernal, 1990; Vargas et al., 2007). Likewise, the nutrient concentrations measured during our samplings are within the range of previously reported values (Vargas et al., 2013; Pérez et al., 2015). The relatively low + values (as compared to the values in winter), together with the high phytoplankton biomass, suggest that our summer sampling took place after an intense upwelling episode. The composition of the phytoplankton taxa found in this study in the Arauco Gulf was in good agreement with that already described for this ecosystem (Anabalón et al., 2007; González et al., 2007) as well as for other upwelling areas (Kudela et al., 2005). On the other hand, the high GPP values found in this (1.4–4.7 g C m2 d−1) and previous studies (e.g., Daneri et al., 2000; Vargas et al., 2007) highlights the importance of the Humboldt current system off central Chile as one of the most productive systems in the world.
Carbonate chemistry dynamics at both sampling sites were likewise associated to the major local oceanographic forcing's dominating the water column. In Valdivia, the more corrosive conditions were observed at the inner estuarine section in winter, associated with the larger river freshwater discharges (Figure 4). This low salinity water was characterized by low DIC concentration and TA (Figure S1), which limited the buffering capacity of the system and lead to Ωarag <1. Similar undersaturation conditions have been reported for other estuarine and river plume environments (e.g., Cao et al., 2011; Wallace et al., 2014). In summer, the subsurface intrusion of more oceanic waters, characterized by high pCO2 and low pH values, was responsible of the undersaturation conditions at the offshore stations (Figure 4). The high pH and low pCO2 levels observed at the surface waters of the inner estuarine portion was most likely caused by the high photosynthetic rates observed during this season (Table 2) (Hinga, 2002). In the Arauco Gulf, the more corrosive conditions were registered in summer and were associated to the upwelling episode that apparently happened before our sampling. The subsurface waters were characterized by low pH (<7.6) and high pCO2 (>1,000 μatm) levels, which led to undersaturation conditions with respect to Ωarag. This is a common feature observed in this (Vargas et al., 2016) and other upwelling ecosystems (e.g., Feely et al., 2008; Hofmann et al., 2011). In winter, the larger influence of freshwater discharges caused the low DIC and TA values determined at the river mouth station (GA3) (Vargas et al., 2016). Overall, the values of the carbonate system parameters determined in this study for both sites are comparable to those included in recent seasonal analysis of the carbonate chemistry conducted in the same areas by Pérez et al. (2015) and Vargas et al. (2016, 2017). In summary, both sites represent ecosystems which naturally experience very large ranges in carbonate system parameters, despite the important differences in the hydrographical causes of this variability and the particular combinations of carbonate system and other chemical parameters that result.
Previous studies have thus reported the dynamics of the hydrography, carbonate chemistry and phytoplankton community structure separately at the two study sites. However, to our knowledge, this is the first time that the relationship between abiotic factors including the carbonate system parameters and the phytoplankton is investigated along the Chilean coast. We observed significant negative correlations between carbonate system parameters and cell abundances only in certain phytoplankton taxa at the estuarine community (Table 3), e.g., silicoflagellates in which changes in the pH and Ωarag values explained about one forth of the changes in their cell abundance. Other phytoplankton groups, such as diatoms and dinoflagellates, were mainly correlated to nutrient concentrations and to other abiotic factors, such as salinity, in the estuarine ecosystem, and temperature in the coastal upwelling ecosystem.
4.2. Influence of Elevated pCO2 on Contrasting Phytoplankton Assemblages
A principal aim of the present study was to investigate how phytoplankton communities that experience high carbonate system variability and naturally acidified conditions respond to increases in the pCO2 levels. This study and the previous studies cited show that the annual range of pCO2 variation is higher in the Arauco Gulf (~200–1,600 μatm) than in the Valdivia River estuary (~150–1,000 μatm). These differences were taken into account in the design of the tested experimental conditions, adjusting the tested CO2 conditions to be more comparable relative to what the communities naturally experience.
Prior to evaluating the effect of increased pCO2, an overall look at the response of the communities investigated here showed that they exhibited opposite behavior during the incubation, despite that both were dominated by diatoms and presented similar initial values of Chl a and total cell abundance. Regardless of the pCO2 treatment, the community from the Valdivia River estuary showed an exponential increase of both the Chl a concentration and the abundance of most of the plankton groups (Figure 8), while the phytoplankton community from the Arauco Gulf presented a decreasing trend of the same biological variables toward the end of the incubation (Figure 9). Since the two experiments were conducted under nutrient-sufficient conditions, we argue that the reason for this contrasting response is that the two communities were at different growth phases when they were collected from the field, which is a common situation when conducting experiments with naturally-phytoplankton assemblages. In general, the phytoplankton from the Valdivia river estuary was more diverse and was actively growing as reflected by the increase in the N/P ratio (Figure 9), which evidenced an enhanced N uptake to support the high growth rates. The phytoplankton from the Arauco Gulf, however, was in a stationary growth phase, according to the constant N/P and Si/N ratios measured during the experiment, and were largely dominated (>75%) by one single diatom genera, Thalassiosira spp. These results suggest that this latter community was currently in a post-bloom phase. During this phase, diatoms increase the production of transparent exopolymeric particles (TEP) which enhances cells stickiness and contributes to the subsequent formation of aggregates (Alldredge et al., 1995; Kioerboe et al., 1996; Thornton, 2002) as observed during our experiments. This aggregates formation is likely the cause of the observed cell abundance decrease in both pCO2 treatments during the experiment with this coastal-upwelling community (Figure 9). In any case, considering that the purpose of this work was to evaluate and compare the response of two distinct coastal phytoplankton communities to rising pCO2, this premise was fulfilled, as the results showed that they differed not only in their taxonomic composition but also in their growth phase.
Owing to the key role of phytoplankton in aquatic food webs as major primary producers, the impact of high pCO2 conditions on these organisms has been the focus of intense research over the last two decades. These investigations, however, have yielded contrasting results. For instance, some studies with natural phytoplankton assemblages have reported a stimulating effect of elevated pCO2 on growth rates and primary productivity (Kim et al., 2006; Riebesell et al., 2007; Tortell et al., 2008). Hare et al. (2007), in turn, reported constant primary production under elevated CO2 in the Bering Sea but a decrease in the diatom contribution to the phytoplankton community. On the other hand, an inhibitory effect has been observed in the formation of the calcareous plankton skeletons, like that of coccolithophorids (Riebesell et al., 2000; Delille et al., 2005), and more recently, other studies have likewise reported negative effects of increased CO2 in non-calcifying organisms (Feng et al., 2010; Yoshimura et al., 2010; Hama et al., 2011). In the present work, we observed no detectable impact of increased CO2 on Chl a concentration in none of the two phytoplankton communities studied (Figures 8, 9), which aligns well with results from previous micro- and mesocosm OA experiments conducted with diatom-dominated phytoplankton assemblages under the same nutrient sufficient conditions (e.g., Engel et al., 2014; Bach et al., 2017).
Although there was no significant impact on total phytoplankton biomass, there were some possible effects on particular phytoplankton functional groups or even specific taxa within functional groups, particularly in the estuarine community. For example, the total numerical abundances of flagellates, diatoms, and dinoflagellates, was about 25–50% lower under the high pCO2 treatment. This also suggests that the Chl a per cell must have on average been higher under this treatment, to account for the lack of an effect on total Chl a concentrations, although we have no direct data at the cellular level for this. Increases in Chl a per cell in response to high pCO2 have been previously reported in multiple dinoflagellates (e.g., Eberlein et al., 2016; Hennon et al., 2017). However, Chl a per cell in diatoms has been reported to increase modestly (Crawfurd et al., 2011) to stay the same (e.g., Hennon et al., 2017) or decrease (Jacob et al., 2017) under high pCO2. Within the diatoms, the genera Skeletonema and Thalassiosira appeared to benefit more than others. Although in situ the community was dominated by freshwater diatoms, such as A. granulata (which represented 50% of the numerical abundance), after 5 days these were completely replaced by marine groups. The freshwater diatoms thus probably represent a component that is not growing locally but is advected in, along with other particulate and dissolved organic carbon contributed from the river. Chaetoceros spp. also showed modest decrease toward the end.
The mechanisms underlying differences in the response of distinct phytoplankton to elevated pCO2 can be complex to unravel. On the one hand, a key factor in determining the success of specific functional groups, like diatoms, is their carbon concentrating mechanisms (CCM, Burkhardt et al., 2001; Cassar et al., 2004). Free CO2 in the ocean is at limiting levels for RuBisCO, the primary carboxilating enzyme used in photosynthesis (Beardall and Raven, 2004). To overcome this constraint, many phytoplankton species have developed an intracellular mechanisms that converts bicarbonate ions (HCO3−) to CO2 at the active site of this enzyme, thereby supporting higher carbon fixation rates than what would be possible if photosynthesis only relied on diffusive CO2 uptake (Giordano et al., 2005). The proportion at which both processes operate (i.e., direct CO2 uptake vs. HCO3− uptake) varies largely among species (e.g., Reinfelder, 2011; Matsuda and Kroth, 2014), as they differ in the efficiency and regulation of their CCMs (Burkhardt et al., 2001; Rost et al., 2003; Trimborn et al., 2008). Increases in the seawater CO2 may directly benefit phytoplankton species primarily relying on diffusive CO2 uptake or those with inefficient CCMs. Species that rely on a resource-intensive CCM, in turn, could also potentially benefit from a downregulation of the CCM in the future, which will allow them to optimize the energy and resources allocation (Rost et al., 2008; Eggers et al., 2014). Nevertheless, this CCM downregulation at increased pCO2 has been observed to occur primarily under nutrient-limitation conditions (Taucher et al., 2015), and depends on the capacity of each species to switch between carbon acquisition mechanisms. On the other hand, low pH can potentially affect key metabolic rates, such as the ones involved in the maintenance of intracellular homeostasis, which could ultimately counteract the positive effect of CO2 fertilization (Wu and Gao, 2010; Bach and Taucher, 2019).
The response of the phytoplankton community from the coastal-upwelling ecosystem in the Arauco Gulf showed no significant effect of short-term exposure to increased pCO2 in the abundance of either of the two groups that integrated the community, i.e., diatoms and dinoflagellates (Figure 9). This finding support our initial hypothesis that exposure to high CO2 conditions will not challenge phytoplankton communities inhabiting highly variable and acidic habitats. In agreement with our results, a recent review by Bach and Taucher (2019) showed that diatom communities in oceanic environments responded more frequently to rising pCO2, with negative effects, than in coastal, estuarine, or benthic environments. It is currently beyond doubt that environmental variability can play a key role in promoting local adaptation of organisms so that they tolerate larger range of pH and pCO2 levels, which are usually characteristics of coastal ecosystems in both temporal and spatial scales (Duarte et al., 2013; Vargas et al., 2017). One study that reflects the importance of local adaptation was the meta-analysis conducted by Vargas et al. (2017) along the Chilean coast. These authors showed that organisms inhabiting areas with high mean pCO2 and high pCO2 variability exhibited lower negative effects on physiological traits to elevated pCO2 conditions, suggesting that local adaptation can play a key role in setting sensitivity of species to changes in pCO2. In agreement to that reported by these authors for other marine organisms, such as copepods, gastropods or mussels, our results suggest that phytoplankton communities inhabiting the coastal upwelling ecosystem in the Gulf of Arauco are locally adapted to the naturally occurring high pCO2 levels (Figure 5; Vargas et al., 2017), so that short-term exposure to elevated pCO2 levels does not challenge them.
5. Conclusions
Our results have shown that changes in the carbonate chemistry in the coastal water may have distinct implications for the phytoplankton communities inhabiting an estuarine and a coastal upwelling systems. Increases of pCO2 can modify the structure of the estuarine phytoplankton community by decreasing the cell abundance of specific phytoplankton groups, which could have potential bottom-up effects for higher trophic levels. Contrarily, the phytoplankton community from the coastal upwelling ecosystem appears to be locally adapted to changes in the pCO2 levels. Given the high CO2 values naturally occurring in this coastal ecosystem, other environmental drivers, such as eutrophication and/or warming may have a larger impact on this phytoplankton assemblage in the future. Overall, these findings highlight the importance of knowing the habitat-specific natural variability of seawater chemistry to better understand the potential impact of elevated pCO2 conditions at the individual, community and ecosystem levels.
Data Availability Statement
The datasets generated for this study are available on request to the corresponding author.
Author Contributions
CV and BJ contributed the concept and design of the study. LL-M, BJ, and PC collected the samples during field samplings, conducted the experiments, and analyzed the samples. NO, PC, and CV organized the database and analyzed the datasets. NO drafted the manuscript with contributions from CV and PD. All authors contributed to the manuscript revision, read, and approved the submitted version.
Funding
The present work was fully funded by FONDECYT Project 1170065 to CV and 1181614 to PD. NO was supported by CONICYT Post-doctoral Project 3190365.
Conflict of Interest
The authors declare that the research was conducted in the absence of any commercial or financial relationships that could be construed as a potential conflict of interest.
Acknowledgments
The authors also acknowledge support from the Millennium Science Initiative from Chile's Ministry of Economy, Development, and Tourism, through both the Millennium Nucleus MUSELS MINECON NC120086 and the Millennium Institute of Oceanography (IMO), MINECON IC120019.
Supplementary Material
The Supplementary Material for this article can be found online at: https://www.frontiersin.org/articles/10.3389/fmars.2020.00323/full#supplementary-material
References
Alldredge, A. L., Cowles, T. J., MacIntyre, S., Rines, J. E. B., Donaghay, P. L., Greenlaw, C. F., et al. (2002). Occurrence and mechanisms of formation of a dramatic thin layer of marine snow in a shallow Pacific fjord. Mar. Ecol. Prog. Ser. 233, 1–12. doi: 10.3354/meps233001
Alldredge, A. L., Gotschalk, C. C., Passow, U., and Riebesell, U. (1995). Mass aggregtion of diatom blooms: insights from a mesocosm study. Deep Sea Res. II Top. Stud. Oceanogr. 42, 9–27. doi: 10.1016/0967-0645(95)00002-8
Anabalón, V., Morales, C. E., Escribano, R., and Angélica Varas, M. (2007). The contribution of nano- and micro-planktonic assemblages in the surface layer (0–30 m) under different hydrographic conditions in the upwelling area off Concepción, central Chile. Prog. Oceanogr. 75, 396–414. doi: 10.1016/j.pocean.2007.08.023
Bach, L. T., Alvarez-Fernandez, S., Hornick, T., Stuhr, A., and Riebesell, U. (2017). Simulated ocean acidification reveals winners and losers in coastal phytoplankton. PLoS ONE 12:e0188198. doi: 10.1371/journal.pone.0188198
Bach, L. T., and Taucher, J. (2019). CO2 effects on diatoms: a synthesis of more than a decade of ocean acidification experiments with natural communities. Ocean Sci. 15, 1159–1175. doi: 10.5194/os-15-1159-2019
Beardall, J., and Raven, J. A. (2004). The potential effects of global climate change on microalgal photosynthesis, growth and ecology. Phycologia 43, 26–40. doi: 10.2216/i0031-8884-43-1-26.1
Booth, J. A. T., McPhee-Shaw, E. E., Chua, P., Kingsley, E., Denny, M., Phillips, R., et al. (2012). Natural intrusions of hypoxic, low pH water into nearshore marine environments on the California coast. Cont. Shelf Res. 45, 108–115. doi: 10.1016/j.csr.2012.06.009
Bopp, L., Resplandy, L., Orr, J. C., Doney, S. C., Dunne, J. P., Gehlen, M., et al. (2013). Multiple stressors of ocean ecosystems in the 21st century: projections with CMIP5 models. Biogeosciences 10, 6225–6245. doi: 10.5194/bg-10-6225-2013
Borges, A. V., Schiettecatte, L.-S., Abril, G., Delille, B., and Gazeau, F. (2006). Carbon dioxide in European coastal waters. Estuar. Coast. Shelf Sci. 70, 375–387. doi: 10.1016/j.ecss.2006.05.046
Boyd, P. W., Cornwall, C. E., Davison, A., Doney, S. C., Fourquez, M., Hurd, C. L., et al. (2016). Biological responses to environmental heterogeneity under future ocean conditions. Glob. Change Biol. 22, 2633–2650. doi: 10.1111/gcb.13287
Buapet, P., Gullström, M., and Björk, M. (2013). Photosynthetic activity of seagrasses and macroalgae in temperate shallow waters can alter seawater pH and total inorganic carbon content at the scale of a coastal embayment. Mar. Freshw. Res. 64, 1040–1049. doi: 10.1071/MF12124
Burkhardt, S., Amoroso, G., Riebesell, U., and Sültemeyer, D. (2001). CO2 and HCO3-uptake in marine diatoms acclimated to different CO2 concentrations. Limnol. Oceanogr. 46, 1378–1391. doi: 10.4319/lo.2001.46.6.1378
Caldeira, K., and Wickett, M. E. (2003). Anthropogenic carbon and ocean pH. Nature 425:365. doi: 10.1038/425365a
Cao, Z., Dai, M., Zheng, N., Wang, D., Li, Q., Zhai, W., et al. (2011). Dynamics of the carbonate system in a large continental shelf system under the influence of both a river plume and coastal upwelling. J. Geophys. Res. 116:G02010. doi: 10.1029/2010JG001596
Cassar, N., Laws, E. A., Bidigare, R. R., and Popp, B. N. (2004). Bicarbonate uptake by Southern Ocean phytoplankton. Glob. Biogeochem. Cycles 18, 1–10. doi: 10.1029/2003GB002116
Crawfurd, K. J., Raven, J. A., Wheeler, G. L., Baxter, E. J., and Joint, I. (2011). The response of thalassiosira pseudonana to long-term exposure to increased CO2 and decreased pH. PLoS ONE 6:e0026695. doi: 10.1371/journal.pone.0026695
Cuevas, L. A., Tapia, F. J., IRIARTE, J. L., González, H. E., Silva, N., and Vargas, C. A. (2019). Interplay between freshwater discharge and oceanic waters modulates phytoplankton size-structure in fjords and channel systems of the Chilean Patagonia. Prog. Oceanogr. 173, 103–113. doi: 10.1016/j.pocean.2019.02.012
Daneri, G., Dellarossa, V., Quiñones, R., Jacob, B., Montero, P., and Ulloa, O. (2000). Primary production and community respiration in the Humboldt Current System off Chile and associated oceanic areas. Mar. Ecol. Prog. Ser. 197, 41–49. doi: 10.3354/meps197041
Delille, B., Harlay, J., Zondervan, I., Stephan, J., Chou, L., Wollast, R., et al. (2005). Response of primary production and calcification to changes of pCO2 during experimental blooms of the coccolithophorid Emiliania huxleyi. Glob. Biogeochem. Cycles 19:GB2023. doi: 10.1029/2004GB002318
Dickson, A. G. (1990). Thermodynamics of the dissociation of boric acid in synthetic seawater from 273.15 to 318.15 K. Deep Sea Res. A Oceanogr. Res. Pap. 37, 755–766. doi: 10.1016/0198-0149(90)90004-F
Dickson, A. G., and Millero, F. J. (1987). A comparison of the equilibrium constants for the dissociation of carbonic acid in seawater media. Deep Sea Res. A Oceanogr. Res. Pap. 34, 1733–1743. doi: 10.1016/0198-0149(87)90021-5
DOE (1994). Handbook of Methods for the Analysis of the Various Parameters of the Carbon Dioxide System in Seawater, version 2, 74. Washington, DC: ORNL/CDIAC.
Duarte, C. M., Hendriks, I. E., Moore, T. S., Olsen, Y. S., Steckbauer, A., Ramajo, L., et al. (2013). Is ocean acidification an open-ocean syndrome? Understanding anthropogenic impacts on seawater pH. Estuar. Coasts 36, 221–236. doi: 10.1007/s12237-013-9594-3
Eberlein, T., Van de Waal, D. B., Brandenburg, K. M., John, U., Voss, M., Achterberg, E. P., et al. (2016). Interactive effects of ocean acidification and nitrogen limitation on two bloom-forming dinoflagellate species. Mar. Ecol. Prog. Ser. 543, 127–140. doi: 10.3354/meps11568
Eggers, S. L., Lewandowska, A. M., Barcelos e Ramos, J., Blanco-Ameijeiras, S., Gallo, F., and Matthiessen, B. (2014). Community composition has greater impact on the functioning of marine phytoplankton communities than ocean acidification. Glob. Change Biol. 20, 713–723. doi: 10.1111/gcb.12421
Engel, A., Piontek, J., Grossart, H.-P., Riebesell, U., Schulz, K. G., and Sperling, M. (2014). Impact of CO2 enrichment on organic matter dynamics during nutrient induced coastal phytoplankton blooms. J. Plankton Res. 36, 641–657. doi: 10.1093/plankt/fbt125
Feely, R. A., Sabine, C. L., Hernandez-Ayon, J. M., Ianson, D., and Hales, B. (2008). Evidence for upwelling of corrosive ‘acidified’ water onto the continental shelf. Science 320, 1490–1492. doi: 10.1126/science.1155676
Feng, Y., Hare, C. E., Rose, J. M., Handy, S. M., DiTullio, G. R., Lee, P. A., et al. (2010). Interactive effects of iron, irradiance and CO2 on Ross Sea phytoplankton. Deep Sea Res. I 57, 368–383. doi: 10.1016/j.dsr.2009.10.013
Garcés-Vargas, J., Ruiz, M., Pardo, L. M., Nuñez, S., and Pérez-Santos, I. (2013). Hydrographic features of Valdivia river estuary south-central Chile. Latin Am. J. Aquat. Res. 41, 113–125. doi: 10.3856/vol41-issue1-fulltext-9
Giesecke, R., Vallejos, T., Sanchez, M., and Teiguiel, K. (2017). Plankton dynamics and zooplankton carcasses in a mid-latitude estuary and their contributions to the local particulate organic carbon pool. Cont. Shelf Res. 132, 58–68. doi: 10.1016/j.csr.2016.07.020
Giordano, M., Beardall, J., and Raven, J. A. (2005). Concentrating mechanisms in algae: mechanisms, environmental modulation, and evolution. Annu. Rev. Plant Biol. 56, 99–131. doi: 10.1146/annurev.arplant.56.032604.144052
González, H. E., Calderón, M. J., Castro, L., Clement, A., Cuevas, L. A., Daneri, G., et al. (2010). Primary production and plankton dynamics in the Reloncaví Fjord and the Interior Sea of Chiloé, Northern Patagonia, Chile. Mar. Ecol. Prog. Ser. 402, 13–30. doi: 10.3354/meps08360
González, H. E., Menschel, E., Aparicio, C., and Barría, C. (2007). Spatial and temporal variability of microplankton and detritus, and their export to the shelf sediments in the upwelling area off Concepción, Chile (~36°S), during 2002–2005. Prog. Oceanogr. 75, 435–451. doi: 10.1016/j.pocean.2007.08.025
Gruber, N. (2011). Warming up, turning sour, losing breath: ocean biogeochemistry under global change. Philos. Trans. R. Soc. A 369, 1980–1996. doi: 10.1098/rsta.2011.0003
Gruber, N., Clement, D., Carter, B. R., Feely, R. A., van Heuven, S., Hoppema, M., et al. (2019). The oceanic sink for anthropogenic CO2 from 1994 to 2007. Science 363, 1193–1199. doi: 10.1126/science.aau5153
Hama, T., Kawashima, S., Shimotori, K., Satoh, Y., Omori, Y., Wada, S., et al. (2011). Effect of ocean acidification on coastal phytoplankton composition and accompanying organic nitrogen production. J. Oceanogr. 68, 183–194. doi: 10.1007/s10872-011-0084-6
Hare, C. E., Leblanc, K., DiTullio, G. R., Kudela, R. M., Zhang, Y., Lee, P. A., et al. (2007). Consequences of increased temperature and CO2 for phytoplankton community structure in the Bering Sea. Mar. Ecol. Prog. Ser. 352, 9–16. doi: 10.3354/meps07182
Hennon, G. M. M., Hernndez Limn, M. D., Haley, S. T., Juhl, A. R., and Dyhrman, S. T. (2017). Diverse CO2-induced responses in physiology and gene expression among eukaryotic phytoplankton. Front. Microbiol. 8:2547. doi: 10.3389/fmicb.2017.02547
Hinga, K. R. (2002). Effects of pH on coastal marine phytoplankton. Mar. Ecol. Prog. Ser. 238, 281–300. doi: 10.3354/meps238281
Hoegh-Guldberg, O., and Bruno, J. F. (2010). The impact of climate change on the world's marine ecosystems. Science 328, 1523–1528. doi: 10.1126/science.1189930
Hofmann, G. E., Smith, J. E., Johnson, K. S., Send, U., Levin, L. A., Micheli, F., et al. (2011). High-frequency dynamics of ocean pH: a multi-ecosystem comparison. PLoS ONE 6:e28983. doi: 10.1371/journal.pone.0028983
Iriarte, J. L., and Bernal, P. (1990). Vertical distribution of diatoms and thecate dinoflagellates in the gulf of Arauco: species composition, relative abundance, and the chloropyll maximum layer. Sci. Mar. 54, 389–399.
Iriarte, J. L., Cuevas, L. A., Cornejo, F., Silva, N., González, H. E., Castro, L., et al. (2018). Low spring primary production and microplankton carbon biomass in Sub-Antarctic Patagonian channels and fjords (50–53°S). Arctic Antarctic Alpine Res. 50, 1–14. doi: 10.1080/15230430.2018.1525186
Iriarte, J. L., González, H. E., Liu, K.-K., Rivas, C., and Valenzuela, C. (2007). Spatial and temporal variability of chlorophyll and primary productivity in surface waters of southern Chile (41.5–43°S). Estuar. Coast. Shelf Sci. 74, 471–480. doi: 10.1016/j.ecss.2007.05.015
Jacob, B. G., von Dassow, P., Salisbury, J. E., Navarro, J. M., and Vargas, C. A. (2017). Impact of low pH/high pCO2 on the physiological response and fatty acid content in diatom Skeletonema pseudocostatum. J. Mar. Biol. Assoc. U. K. 97, 225–233. doi: 10.1017/S0025315416001570
Jouenne, F., Lefebvre, S., Véron, B., and Lagadeuc, Y. (2007). Phytoplankton community structure and primary production in small intertidal estuarine-bay ecosystem (eastern English Channel, France). Mar. Biol. 151, 805–825. doi: 10.1007/s00227-006-0440-z
Kim, J.-M., Lee, K., Shin, K., Kang, J.-H., Lee, H.-W., Kim, M., et al. (2006). The effect of seawater CO2 concentration on growth of a natural phytoplankton assemblage in a controlled mesocosm experiment. Limnol. Oceanogr. 51, 1629–1636. doi: 10.4319/lo.2006.51.4.1629
Kioerboe, T., Hansen, J., Alldredge, A. L., Jackson, G. A., Passow, U., Dam, H. G., et al. (1996). Sedimentation of phytoplankton during a diatom bloom: rates and mechanisms. J. Mar. Res. 54, 1123–1148. doi: 10.1357/0022240963213754
Kudela, R., Pitcher, G., Probyn, T., Figueiras, F., Moita, T., and Trainer, V. (2005). Harmful algal blooms in coastal upwelling systems. Oceanography 18, 184–197. doi: 10.5670/oceanog.2005.53
Letelier, J., Pizarro, O., and Nuñez, S. (2009). Seasonal variability of coastal upwelling and the upwelling front off central Chile. J. Geophys. Res. Oceans 114, 1–16. doi: 10.1029/2008JC005171
Matsuda, Y., and Kroth, P. G. (2014). “Carbon fixation in diatoms,” in The Structural Basis of Biological Energy Generation, ed M. F. Hohmann-Marriott (Heidelberg: Springer), 335–362. doi: 10.1007/978-94-017-8742-0_18
Mehrbach, C., Culberson, C. H., Hawley, J. E., and Pytkowicx, R. M. (1973). Measurement of the apparent dissociation constants of carbonic acid in seawater at atmospheric pressure. Limnol. Oceanogr. 18, 897–907. doi: 10.4319/lo.1973.18.6.0897
Millero, F. J. (2010). Carbonate constants for estuarine waters. Mar. Freshw. Res. 61, 139–142. doi: 10.1071/MF09254
Orr, J. C., Fabry, V. J., Aumont, O., Bopp, L., Doney, S. C., Feely, R. A., et al. (2005). Anthropogenic ocean acidification over the twenty-first century and its impact on calcifying organisms. Nature 437, 681–686. doi: 10.1038/nature04095
Parsons, T. R., Maita, Y., and Lalli, C. M. (1984). A Manual of Chemical and Biological Methods for Seawater Analysis. New York, NY: Pergamon Press, 173.
Pérez, C. A., DeGrandpre, M. D., Lagos, N. A., Sald i as, G. S., Cascales, E.-K., and Vargas, C. A. (2015). Influence of climate and land use in carbon biogeochemistry in lower reaches of rivers in central southern Chile: implications for the carbonate system in river-influenced rocky shore environments: Riverine contributions to coastal areas. J. Geophys. Res. Biogeosci. 120, 673–692. doi: 10.1002/2014JG002699
Pierrot, D., Lewis, E., and Wallace, D. (2006). CO2SYS Dos Program Developed for CO2 System Calculations. ORNL/CDIAC-105. Oak Ridge, TN: Carbon Dioxide Information Analysis Center; Oak Ridge National Laboratory; US Deparstment of Energy.
Pino, M., Perillo, G. M. E., and Santamarina, P. (1994). Residual fluxes in a cross-section of the Valdivia River estuary, Chile. Estuar. Coast. Mar. Sci. 38, 491–505. doi: 10.1006/ecss.1994.1034
Raymond, P. A., and Cole, J. J. (2003). Increase in the export of alkalinity from North America's largest river. Science 301, 88–91. doi: 10.1126/science.1083788
Reinfelder, J. R. (2011). Carbon concentrating mechanisms in eukaryotic marine phytoplankton. Annu. Rev. Mar. Sci. 3, 291–315. doi: 10.1146/annurev-marine-120709-142720
Riebesell, U., Fabry, V. J., Hansson, L., and Gattuso, J.-P. (2010). Guide to Best Practices for Ocean Acidification Research and Data Reporting. Luxembourg: Publications Office of the European Union.
Riebesell, U., Schulz, K. G., Bellerby, R. G. J., Botros, M., Fritsche, P., Meyerhöfer, M., et al. (2007). Enhanced biological carbon consumption in a high CO2 ocean. Nature 450, 545–549. doi: 10.1038/nature06267
Riebesell, U., Zondervan, I., Rost, B., Tortell, P. D., and Morel, F. M. M. (2000). Reduced calcification of marine plankton in response to increased atmospheric CO2. Nature 407, 364–367. doi: 10.1038/35030078
Rost, B., Riebesell, U., Burkhardt, S., and Sültemeyer, D. (2003). Carbon acquisition of bloom-forming marine phytoplankton. Limnol. Oceanogr. 48, 55–67. doi: 10.4319/lo.2003.48.1.0055
Rost, B., Zondervan, I., and Wolf-Gladrow, D. (2008). Sensitivity of phytoplankton to future changes in ocean carbonate chemistry: current knowledge, contradictions and research directions. Mar. Ecol. Prog. Ser. 373, 227–237. doi: 10.3354/meps07776
Sabine, C. L., Feely, R. A., Gruber, N., Key, R. M., Lee, K., Bullister, J. L., et al. (2004). The oceanic sink for anthropogenic CO2. Science 305, 367–371. doi: 10.1126/science.1097403
Saderne, V., Fietzek, P., and Herman, P. M. J. (2013). Extreme variations of pCO2 and pH in a macrophyte meadow of the Baltic sea in summer: evidence of the effect of photosynthesis and local upwelling. PLoS ONE 8:e62689. doi: 10.1371/journal.pone.0062689
Shamberger, K. E. F., Feely, R. A., Sabine, C. L., Atkinson, M. J., DeCarlo, E. H., Mackenzie, F. T., et al. (2011). Calcification and organic production on a Hawaiian coral reef. Mar. Chem. 127, 64–75. doi: 10.1016/j.marchem.2011.08.003
Sobarzo, M., Bravo, L., Donoso, D., Garcés-Vargas, J., and Schneider, W. (2007). Coastal upwelling and seasonal cycles that influence the water column over the continental shelf off central Chile. Prog. Oceanogr. 75, 363–382. doi: 10.1016/j.pocean.2007.08.022
Strickland, J., and Parsons, T. R. (1968). Practical Handbook of Seawater Analysis, 167th Edn. Fisheries Research Board of Canada Bulletin.
Strickland, J. D. H. (1960). Measuring the production of marine phytoplankton. Fish. Res. Bd. Canada Bull. 122:172.
Taucher, J., Jones, J., James, A., Brzezinski, M. A., Carlson, C. A., Riebesell, U., et al. (2015). Combined effects of CO2 and temperature on carbon uptake and partitioning by the marine diatoms Thalassiosira weissflogii and Dactyliosolen fragilissimus. Limnol. Oceanogr. 60, 901–919. doi: 10.1002/lno.10063
Thornton, D. C. O. (2002). Diatom aggregation in the sea: mechanisms and ecological implications. Eur. J. Phycol. 37, 149–161. doi: 10.1017/S0967026202003657
Torres, R., Pantoja, S., Harada, N., González, H. E., Daneri, G., Frangopulos, M., et al. (2011). Air-sea CO2 fluxes along the coast of Chile: from CO2 outgassing in central northern upwelling waters to CO2 uptake in southern Patagonian fjords. J. Geophys. Res. Oceans 116, 1–17. doi: 10.1029/2010JC006344
Tortell, P. D., DiTullio, G. R., Sigman, D. M., and Morel, F. M. M. (2002). CO2 effects on taxonomic composition and nutrient utilization in an Equatorial Pacific phytoplankton assemblage. Mar. Ecol. Prog. Ser. 236, 37–43. doi: 10.3354/meps236037
Tortell, P. D., Payne, C. D., Li, Y. Y., Trimborn, S., Rost, B., Smith, W. O., et al. (2008). CO2 sensitivity of Southern Ocean phytoplankton. Geophys. Res. Lett. 35:L04605. doi: 10.1029/2007GL032583
Trimborn, S., Brenneis, T., Sweet, E., and Rost, B. (2013). Sensitivity of Antarctic phytoplankton species to ocean acidification: growth, carbon acquisition, and species interaction. Limnol. Oceanogr. 58, 997–1007. doi: 10.4319/lo.2013.58.3.0997
Trimborn, S., Lundholm, N., Thoms, S., Richter, K.-U., Krock, P., Hansen, P. J., et al. (2008). Inorganic carbon acquisition in potentially toxic and non-toxic diatoms: the effect of pH-induced changes in seawater carbonate chemistry. Physiol. Plant. 133, 92–105. doi: 10.1111/j.1399-3054.2007.01038.x
Utermöhl, H. (1958). Zur Vervollkomnung der quantita- tiven Phytoplankton-Methodik. Mitteilungen. Int. Vereiningung Theoret. Angew. Limnol. 9, 1–38.
Valle-Levinson, A., Atkinson, L. P., Figueroa, D., and Castro, L. (2003). Flow induced by upwelling winds in an equatorward facing bay: Gulf of Arauco, Chile. J. Geophys. Res. C Oceans 108:3054. doi: 10.1029/2001JC001272
Vargas, C. A., Araneda, S. E., and Valenzuela, G. (2003). Influence of tidal phase and circulation on larval fish distribution in a partially mixed estuary, Corral Bay, Chile. J. Mar. Biol. Assoc. U. K. 83, 217–222. doi: 10.1017/S0025315403006994h
Vargas, C. A., Arriagada, L., Sobarzo, M., Contreras, P. Y., and Saldías, G. (2013). Bacterial production along a river-to-ocean continuum in central Chile: implications for organic matter cycling. Aquat. Microb. Ecol. 68, 195–213. doi: 10.3354/ame01608
Vargas, C. A., Contreras, P. Y., Pérez, C. A., Sobarzo, M., Sald i as, G. S., and Salisbury, J. (2016). Influences of riverine and upwelling waters on the coastal carbonate system off Central Chile, and their ocean acidification implications: carbonate system in the coastal ocean. J. Geophys. Res. Biogeosci. 121, 1468–1483. doi: 10.1002/2015JG003213
Vargas, C. A., Cuevas, L. A., Silva, N., González, H. E., De Pol-Holz, R., and Narváez, D. A. (2018). Influence of glacier melting and river discharges on the nutrient distribution and DIC recycling in the Southern Chilean Patagonia. J. Geophys. Res. Biogeosci. 123, 256–270. doi: 10.1002/2017JG003907
Vargas, C. A., Lagos, N. A., Lardies, M. A., Duarte, C., Manr i quez, P. H., Aguilera, V. M., et al. (2017). Species-specific responses to ocean acidification should account for local adaptation and adaptive plasticity. Nat. Ecol. Evol. 1:84. doi: 10.1038/s41559-017-0084
Vargas, C. A., Martínez, R. A., Cuevas, L. A., Pavez, M. A., Cartes, C., González, H. E., et al. (2007). The relative importance of microbial and classical food webs in a highly productive coastal upwelling area. Limnol. Oceangr. 52, 1495–1510. doi: 10.4319/lo.2007.52.4.1495
Waldbusser, G. G., and Salisbury, J. E. (2014). Ocean acidification in the coastal zone from an organism's perspective: multiple system parameters, frequency domains, and habitats. Annu. Rev. Mar. Sci. 6, 221–247. doi: 10.1146/annurev-marine-121211-172238
Wallace, R. B., Baumann, H., Grear, J. S., Aller, R. C., and Gobler, C. J. (2014). Coastal ocean acidification: the other eutrophication problem. Estuar. Coast. Shelf Sci. 148, 1–13. doi: 10.1016/j.ecss.2014.05.027
Wu, Y., and Gao, K. (2010). Combined effects of solar UV radiation and CO2-induced seawater acidification on photosynthetic carbon fixation of phytoplankton assemblages in the South China Sea. Chin. Sci. Bull. 55, 3680–3686. doi: 10.1007/s11434-010-4119-y
Keywords: climate change, environmental variability, carbonate chemistry, community structure, coastal upwelling, estuary
Citation: Osma N, Latorre-Melín L, Jacob B, Contreras PY, von Dassow P and Vargas CA (2020) Response of Phytoplankton Assemblages From Naturally Acidic Coastal Ecosystems to Elevated pCO2. Front. Mar. Sci. 7:323. doi: 10.3389/fmars.2020.00323
Received: 13 November 2019; Accepted: 21 April 2020;
Published: 25 May 2020.
Edited by:
Nina Bednarsek, Southern California Coastal Water Research Project, United StatesReviewed by:
José Pinho, University of Minho, PortugalJun Sun, Tianjin University of Science and Technology, China
Copyright © 2020 Osma, Latorre-Melín, Jacob, Contreras, von Dassow and Vargas. This is an open-access article distributed under the terms of the Creative Commons Attribution License (CC BY). The use, distribution or reproduction in other forums is permitted, provided the original author(s) and the copyright owner(s) are credited and that the original publication in this journal is cited, in accordance with accepted academic practice. No use, distribution or reproduction is permitted which does not comply with these terms.
*Correspondence: Natalia Osma, bmF0YWxpYS5vc21hQGltby1jaGlsZS5jbA==