- 1Departamento de Biología, Facultad de Ciencias del Mar y Ambientales, Instituto Universitario de Investigaciones Marinas (INMAR), Universidad de Cádiz, Puerto Real, Spain
- 2Institute of Marine Sciences-National Research Council (ISMAR-CNR), La Spezia, Italy
- 3AZTI, Marine Research, Basque Research and Technology Alliance (BRTA), Pasaia, Spain
- 4ARGANS France, Sophia-Antipolis, France
- 5Research Institute for Applied Mechanics, Kyushu University, Fukuoka, Japan
- 6Department of Marine Sciences, University of the Aegean, Mytilene, Greece
Windrow is a long-established term for the aggregations of seafoam, seaweeds, plankton and natural debris that appear on the ocean surface. Here, we define a “litter windrow” as any aggregation of floating litter at the submesoscale domain (<10 km horizontally), regardless of the force inducing the surface convergence, be it wind or other forces such as tides or density-driven currents. The marine litter windrows observed to date usually form stripes from tens up to thousands of meters long, with litter densities often exceeding 10 small items (<2 cm) per m2 or 1 large item (>2 cm) per 10 m2. Litter windrows are generally overlooked in research due to their dispersion, small size and ephemeral nature. However, applied research on windrows offers unique possibilities to advance on the knowledge and management of marine litter pollution. Litter windrows are hot spots of interaction with marine life. In addition, since the formation of dense litter windrows requires especially high loads of floating litter in the environment, their detection from space-borne sensors, aerial surveys or other platforms might be used to flag areas and periods of severe pollution. Monitoring and assessing of management plans, identification of pollution sources, or impact prevention are identified as some of the most promising fields of application for the marine litter windrows. In the present Perspective, we develop a conceptual framework and point out the main obstacles, opportunities and methodological approaches to address the study of litter windrows.
Introduction
The spatial distribution of floating marine litter at macro scales of thousands of kilometers has been successfully addressed by oceanographic surveys and modeling (Law et al., 2010; Cózar et al., 2014; Eriksen et al., 2014; van Sebille et al., 2015). This has been possible because the macro-scale structures of convergence and accumulation of floating material (e.g., subtropical gyres) are highly persistent over time (Maximenko et al., 2012), and multi-annual samples can be gathered to determine consistent basin-scale distribution patterns. At the mesoscale, from tens to hundreds of kilometers horizontally, litter accumulations are more difficult to track since their timescales ranges from weeks to months (Cózar et al., 2015; Suaria et al., 2016; Macías et al., 2019). Relatively few studies have addressed the mesoscale patterns of litter accumulation on the ocean surface (e.g., Lebreton et al., 2018), but even fewer studies have examined the distribution of floating litter in the submesoscale domain, below 10 km. Instead, there are often photographs (e.g., Ryan, 2013) and sometimes video recordings (e.g., Law et al., 2014) showing the regular formation of small aggregations with striking litter densities.
The ephemeral and scattered occurrence of the small aggregations of floating litter, from a few meters to 10 km in length, make them particularly difficult to study from ship surveys. Indeed, ocean heterogeneity at the submesoscale represents a classical observational frontier for the operational oceanography (McWilliams, 2016). On the other hand, the ubiquity and effects of the small surface convergences are apparent since the beginnings of the ocean exploration. Records of conspicuous patterns of color and composition on the ocean surface date back to the scientific circumnavigations of the eighteenth and nineteenth centuries (Pineda, 1789; Darwin, 1839). The abundance of marine life associated to aggregations of buoyant material was also noted since the first observations (Thomson, 1862). In the twentieth century, the seminal work of Langmuir (1938) prompted the study of the physics underlying the formation of submesoescale structures of surface accumulation, although their full comprehension remains a grand challenge (McWilliams, 2016). Recently, field experiments demonstrated that while ocean currents may disperse floating items over increasingly large areas, these items tend to be concentrated into small clusters at the submesoscale (D’Asaro et al., 2018).
The present Perspective addresses the aggregations of floating litter in the submesoscale domain (<10 km), which we call “litter windrows,” in line with the long-established terminology used to refer to the rafts of seafoam, seaweeds, jellyfish and natural debris (Faller and Woodcock, 1964; Craik, 1970; Liebovich, 1983). We use the term “litter windrow” to embrace any small-sized aggregation of floating litter, regardless of its shape or the mechanism inducing its formation, including surface convergences driven by wind, tidal currents, river discharges, upwellings or any other force. The term focuses on the submesoscale patchiness of the floating litter in the broadest sense. The extraordinarily high density of litter observed in these patches might activate and trigger particular processes at the physical, chemical and biological levels, offering a hitherto overlooked view of the marine litter issue. Here, we aim to develop a conceptual framework for the marine litter windrows, identifying gaps and opportunities and proposing methodological approaches for future research.
Formation of Litter Windrows and Potential Concentration Factor
The formation of litter windrows requires, firstly, the activation of convergence zones on the ocean surface, and secondly, a sufficient load of floating litter in the surroundings. There is a wide array of forcing processes able to generate convergent structures within the submesoscale domain (van Sebille et al., 2020). In this section, we highlight internal waves (Figure 1A), Langmuir circulation (Figure 1B), estuarine fronts (Figure 1C), and density fronts (Figure 1D), although we are just beginning to understand the physics behind the litter windrows.
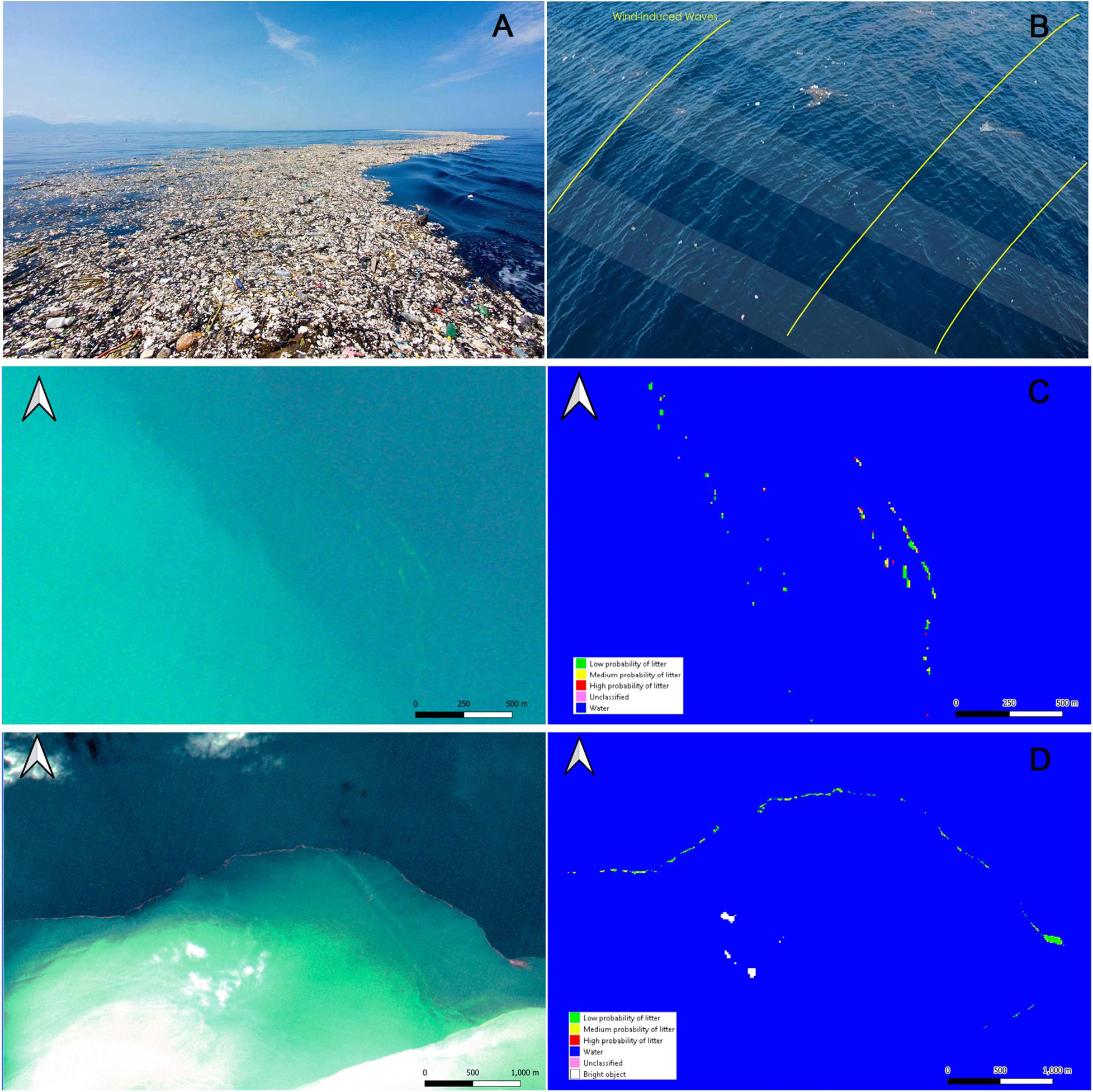
Figure 1. (A) Litter windrow likely associated to internal wave off Honduras (photo by C. Power). (B) Small patches and scattered litter arranged in bands by Langmuir circulation in southwestern Mediterranean (photo by A. Cózar). Convergence bands perpendicular to wave lines are highlighted. (C) Litter windrow associated to estuarine front in the plume of Po river (Italy). (D) Litter windrow associated to density front off Crete. Panels C and D show windrow-like structures of floating litter detected using Sentinel-2/MSI data according to Hennen et al. (2019). For each of the panels, the right image is a false color RGB composite, whilst the left panel is the result of the processed image, with red, yellow and green pixels showing high (>85%), medium (85–50%), and low confidence (<50%) of containing plastics, respectively.
Internal waves and estuarine fronts are submesoscale convergent structures typically associated with nearshore areas. Internal waves are one the few processes that have been confirmed to induce the formation of litter windrows (Gove et al., 2019). The effect of the continental shelf topography on tidal and other internal flows results in surface convergences and divergences above the troughs and crests of the internal waves, respectively (Shanks, 1987; Bruno et al., 2002). Estuarine fronts are also expected to be commonly associated with litter aggregations in nearshore waters, given that rivers are often point sources of litter (Acha et al., 2003). Langmuir circulation, frequently developed with steady moderate winds (>3 m s–1) in both nearshore and offshore areas, is the process commonly associated with the “windrow” term (Liebovich, 1983). Submesoscale density fronts and vortices seems to play a key role in offshore areas. These structures have been shown to lead to striking aggregations of Sargassum and oceanographic drifters in the open ocean (Zhong et al., 2012; D’Asaro et al., 2018).
The capacity of the convergence processes to form aggregations of floating litter can be quantified from concentration factors, estimated as ratios of litter concentrations (inside versus surrounding ambient concentrations, e.g., Shanks, 1983) or, alternatively, as ratios of linear distances or areas (ocean area swept by the convergence versus area of the accumulation zone; e.g., D’Asaro et al., 2018). Langmuir circulations typically generate convergence stripes of a few meters wide, which collect the floating material within tens of meters on either side of the stripe (van Sebille et al., 2020). From the first-order approximation of the width of the side bands affected by the convergence and that of the accumulation stripe, we derive a concentration factor for Langmuir circulation on the order of 10, which seem to be relatively low compared to the potential concentration capacity of other convergent processes (Table 1). However, the ubiquity and frequency of Langmuir circulations might make them overlap onto other accumulative processes.
Using the microplastic concentrations measured within and outside the convergence zones generated by internal waves in Hawai’i, Gove et al. (2019) estimated a concentration factor for these structures ranging from 10 to 102. Shanks (1987) obtained concentration factors in the same order of magnitude by measuring floating tar balls accumulated in internal waves in coastal waters of North Carolina. The internal waves propagated for kilometers to collect scattered litter in their convergence zones. It is likely that the capacity of the estuarine fronts to accumulate litter might be even higher than internal waves, since they are highly persistent structures able to trap the riverine inputs of floating material rolling onto the front (Acha et al., 2003).
Particularly relevant to show the significance of the open-ocean density fronts and vortices was the finding in D’Asaro et al. (2018). In their experiment in the Gulf of Mexico, a hundred drifters spread over 20 × 20 km2 were lined up into a single cluster less than 100 m long within a week, reaching a concentration factor on the order of 105. Surface density fronts that move in open waters can sweep enormous areas to capture buoyant material.
Apart from the concentration power, the convergence processes will induce the formation of litter windrows in those regions with a particularly high concentration of floating litter, and this, a priori, includes the macro-scale accumulation zone of the subtropical gyres (Law et al., 2010), the semienclosed seas with high human pressure (Cózar et al., 2015), or the waters close to litter sources such as rivers and cities (Pedrotti et al., 2016). It seems reasonable to hypothesize that the formation of litter windrows in coastal waters will be particularly frequent with the first rains after the dry season, after the snowmelt at high latitudes, or associated to events of heavy stormwater drainage (Ory et al., 2020).
Litter Concentrations in the Windrows
The purpose of this section is to provide a first reference for the range of litter concentrations hitherto measured in windrows compared to that derived from the traditional large-scale random sampling. Measurements of surface litter concentrations generally rely on visual counts for macro-litter (e.g., Eriksen et al., 2014; Galgani et al., 2015) and plankton-net trawling for micro-litter (e.g., Law et al., 2010; Goldstein et al., 2012), with both approaches developed over random line transects regardless of the small-scale heterogeneity. Visual-census transects are several kilometers long, while plankton-net transects are typically 1 km long. From these random samplings, the highest abundances of macro-litter (>2 cm) counted in visual transects are on the order of 1 macro-item per 1,000 m2 (Galgani et al., 2015), far below the concentration range measured in macro-litter windrows by Ruiz et al. (2020; Figure 2A). In the micro-litter size range (here considered < 2 cm), random net transects have occasionally reported concentrations up to 10 items per m2 (Figure 2B). So far, the measurements of micro-litter concentrations in windrows are limited to the study of Gove et al. (2019). These measurements overlap the range reported from random transects, but also reach concentrations an order of magnitude higher (Figure 2B).
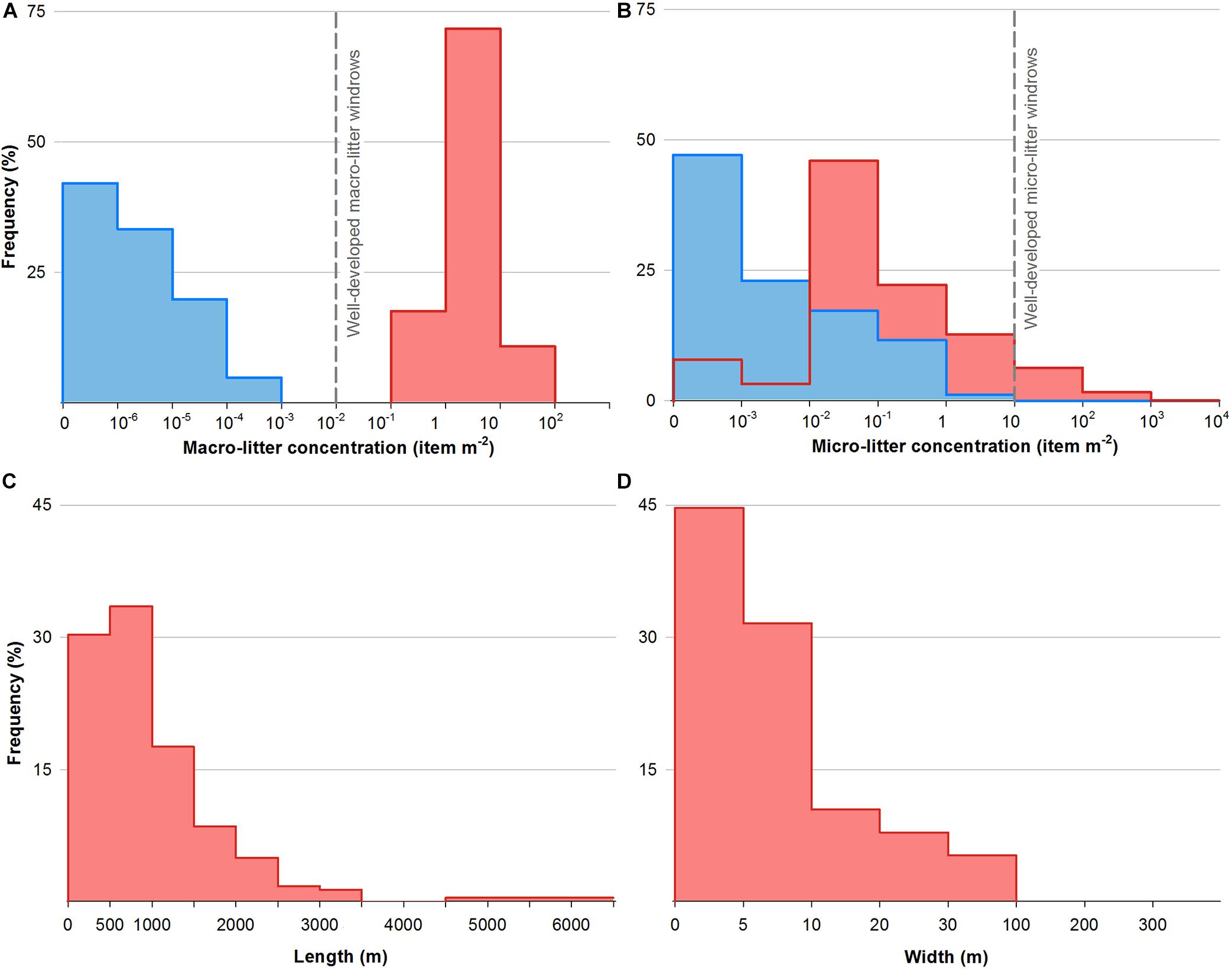
Figure 2. Concentrations of macro-litter (A) and micro-litter (B) measured in random (blue histograms) and windrow (red histograms) samplings. Minimum concentrations proposed for well-developed windrows are shown with vertical dashed lines. Frequency histograms of lengths (C) and widths (D) for litter windrows. For the analysis of litter concentrations (A,B), we used a total of 887 random visual transects for macro-litter concentrations (>2 cm, Eriksen et al., 2014), and 6,018 random plankton-net transects for micro-litter concentrations (Law et al., 2010, 2014; Goldstein et al., 2012; Reisser et al., 2013; Cózar et al., 2014, 2015, 2017; Ruiz-Orejón et al., 2016; Suaria et al., 2016; Martí et al., 2017; Lebreton et al., 2018). Litter concentrations in windrows were based on the macro-litter measurements by Ruiz et al. (2020) (n = 163) and micro-litter concentrations provided by Gove et al. (2019) (n = 63). Ruiz et al. (2020) used a 2 m wide macro-net with a 2 cm mesh size, while their counts included the items larger than 2.5 cm, which showed an average mass of 14 g per macro-item. For the analysis of lengths and widths of the litter windrows (C,D), data comprised well-developed litter windrows found in coastal waters of Hawai’i (n = 2, Gove et al., 2019), Bay of Biscay (n = 190, Ruiz et al., 2020), and Western Mediterranean (n = 32, unpublished data). Note that Gove et al. only provided widths for two windrows considered to be well developed.
The wide overlapping between ranges of microplastic concentrations in random and windrow tows is firstly explained from the sampling method used by Gove et al. (2019). They identified and sampled convergent fronts on visual assessment, by locating smooth waters with clearly identifiable edges of rippled water. Their samples showed that many of these convergent fronts were only lightly populated with microplastic (Figure 2B). Secondly, it is unlikely that random visual-census transects fit into a macro-litter windrow, but some of the thousands of plankton-net transects randomly spread on the global ocean could have matched with micro-litter windrows, due to plankton-net transects being shorter and far more frequent than visual transects. Indeed, abnormally high concentrations of microplastics (102 items per m2) were found in random tows conducted in the Sea of Japan or the Mediterranean Sea (Isobe et al., 2015; van der Hal et al., 2017).
Despite the limitations derived from the compilation of data from different sources and methods, our order-of-magnitude analysis showed apparent differences between the upper ranges of litter concentrations measured in windrows and in random samplings (Figures 2A,B). Based on these two frequency distributions of litter concentrations, we set baseline values for well-developed windrows using thresholds rarely exceeded in random transects (<1% of the total) but often exceeded in windrows. Therefore, we used 1 macro-item per 100 m2 and 10 micro-items per m2 as minimum concentrations for well-developed macro- and micro-litter windrows, respectively. The baseline for well-developed macro-litter windrows may seem too low to refer to them as aggregations, but we must note that the distribution of macro-items often shows gaps along the convergence zone that considerably reduce average concentrations (see satellite images in Figure 1 for illustrative examples of gaps along the windrow).
The litter load in the windrow defines its physical structure, therefore we propose reference concentrations for the characterization of the development degree of the windrow. Nevertheless, we must note that the formation of a litter windrow is determined by the ratio of its litter concentration relative to the background concentration (concentration factor > 1) rather than by its absolute litter concentration.
Size and Shape of the Litter Windrows
The shape of the litter windrows reported to date is mainly elongated, ranging from few meters to several kilometers in length, and up to 100 m in width (Figures 2C,D). The formation of parallel bands of litter is also common as a result of the typical pattern of alternating convergences and divergences of internal waves and Langmuir cells (Figure 1B). Detailed categorizations of the windrow morphotypes have been developed for Sargassum rafts. Ody et al. (2019) used five morphotypes ordered by increasing surface coverage from scattered and small patches to bands, bands with patches and large quasi-circular clumps, shapes that are also observed for the litter windrows (Figure 1). The shape of the windrows evolves along with its growth. Swelling and merging of bands lead to compact clumps, while a change in wind or current conditions may turn into a rapid disaggregation in small patches. The life times of the windrows could be on the order of hours or days (e.g., Shanks, 1987; Bruno et al., 2002; Ody et al., 2019), from the short-lived Langmuir circulations and tidal waves to more persistent density fronts in open waters and estuaries.
The downwelling flow, typically associated with surface convergence zones, is expected to result in a vertical extension of the windrows (van Sebille et al., 2020), although there is no measurement of the depth to which litter aggregations may extend. The injection of litter deeper in the water column and its eventual trapping into rotating cells will depend on the balance between the downward flow and the buoyancy of each individual item (Kukulka et al., 2012). Micro-litter, and generically all those items with low buoyant rise velocity, are less susceptible to stay at surface. Nevertheless, available observations prove the existence of well-developed micro-litter windrows, accumulating high loads of microplastics on the surface (Law et al., 2014; Gove et al., 2019). Moreover, macro-litter windrows seem to be regularly accompanied by high surface microplastic loads. Interestingly, the capacity of the windrows to trap and retain microplastics at surface also increases as a patch accumulates more material (Feng et al., 2020).
Significance and Opportunities
Gove et al. (2019) studied 1,000 km2 of ocean surface off Hawaii for a targeted windrow research. While surface convergence zones occupied only 8% of the surface, these convergences accumulated 92% of all floating microplastics. The weight of the litter windrows in the total litter load on the ocean surface can be huge. Measuring litter over long random transects is an appropriate method for estimating loads at meso- and macro-scale if the number of samples is large enough to cover the patchiness of the floating litter in the submesoscale. However, there is a lack of guidelines on the minimum number of random transects needed to overcome the submesoscale heterogeneity. Goldstein et al. (2013) suggested a minimum of 50 transects based on a survey across the North Pacific Gyre, although further studies are certainly needed to better address the submesoscale variability on the ocean surface.
On the other hand, floating litter, mainly plastic, provides an unprecedented opportunity to track the submesoscale convergences as well as an added interest for its understanding. The spectrum of marine plastic sizes shows an extraordinary diversity of windages and buoyancies to track ocean circulation (Yoon et al., 2010; Isobe et al., 2014), and its abundance and ubiquity allow for tagging convergence zones on a scale that conventional oceanographic drifters (around 1,000 globally) cannot cover.
Windrows have also the potential to boost the interactions between litter and marine life (Thiel and Gutow, 2005; Gove et al., 2019). Merging litter and organisms into small ocean parcels has strong implications for both. The likelihood of litter ingestion, entanglement or colonization of litter surfaces increases for the biota (e.g., Gove et al., 2019). In parallel, the escalation of these processes should accelerate the removal of litter from the surface (Cózar et al., 2014). Litter windrows may act as conduits for plastic transfer to the ocean interior and marine life. Not only the planktonic organisms trapped in the convergences, but also ocean-going predators are most likely attracted to the litter windrows. Simple buoys or rafts have been used for centuries by fishermen to increase their catches. Today’s so-called “fish aggregating devices” (FADs) attract both small fish and large predators around them (Josse et al., 2000). Fish shoals, flocks of seabirds, turtles and mammals also aggregate near Sargassum windrows, which are often used as feeding grounds (Haney, 1986; Coston-Clements et al., 1991; Acha et al., 2015). Indeed, many of the striking media reports on marine top predators with stomachs full of plastic might be explained from the massive ingestion of material in litter windrows. Furthermore, convergence zones increase opportunities for marine species to settle on plastic debris and to be transported long distances to non-native ranges (Thiel and Gutow, 2005).
If we are interested in observing marine debris from space, the chances of success do not seem high. Surface concentrations of floating litter measured from the random transects (≥ 1 km long) imply spectral signals hardly detectable by remote sensors, even in the most polluted regions. The percentage of ocean surface physically covered by plastic across the North Pacific Subtropical Gyre was estimated to range from 2⋅10–5 to 0.02% (Goldstein et al., 2013). However, plastic concentrations can be orders of magnitude higher in the litter windrows, particularly in the macro-litter windrows. With the technology currently available, macro-litter windrows are likely the best chance for the remotely sensed monitoring of floating litter (Figure 1).
While prevention is the priority in the marine litter issue, the gathering of macro-litter in floating patches offers a chance for cleanup actions at sea. In the southeast coast of the Bay of Biscay, the abundance of windrows and the macro-litter load per windrow (78 kg on average) enabled active fishing for floating macro-litter (Ruiz et al., 2020). A 17 m long fishing vessel recovered litter from windrows during 68 working days, with average catches of 240 kg per day and up to 1.2 tons in a single day. As concluded by the authors, the fishing for floating litter would not be workable if it was scattered.
Study Approaches
Past experience suggests that progress in understanding the windrow phenomenon will come most rapidly by the concertation of field observation, laboratory experimentation and computational simulation (Liebovich, 1983). However, the underdevelopment of the field component is apparent for the submesoscale domain (McWilliams, 2016).
Knowing where and when will litter windrows emerge is likely to be the main limitation for their study in situ. Some submesoscale convergences are somewhat predictable (e.g., Bruno et al., 2002), but anticipating the location and time for litter windrows is still illusive due to the uncertainty added by the dependence on the litter loading. Within this context, the artificial generation of litter windrows represents a particularly useful tool for field research. Topouzelis et al. (2019) successfully used artificial litter windrows as fake targets to assess the feasibility of the satellite-based observation. Our capacity for detecting real litter windrows from satellite is still in progress (Hennen et al., 2019), but we already have the technology and methods necessary to monitor litter from aircraft or similar platforms (Lebreton et al., 2018; Lambert et al., 2020). A single flight at low altitude (from 150 to 400 m) is able to detect litter items larger than 30–50 cm over hundreds of square kilometers, being a powerful tool for the wide-scale monitoring of macro-litter windrows. The collaboration with fishermen, seafarers and other stake-holders who spend long time at sea, is also a valuable option for increasing the field records of litter windrows, as well as expanding the size range of observable items (Ruiz et al., 2020). Micro-litter windrows are, however, difficult to spot over the ocean surface even from a ship’s deck. As a result, seafoam or surface roughness is used to delimit convergence zones and conduct targeted sampling of micro-litter (Gove et al., 2019). Shanks (1987) used small surface drifters to identify convergence zones and track their dynamics. Today, advances in the cost and size of GPS trackers can greatly contribute to the real-time monitoring of windrows with long-term high-frequency data (Duncan et al., 2020). Interestingly, Miyao and Isobe (2016) combined balloon photography and buoy-tracking for mapping convergence structures at high spatial resolution.
The sighting of litter windrows from oceanographic vessels is very occasional. As such, these encounters are special opportunities to gain empirical insight into this little-known phenomenon. Basic variables to characterize litter windrows include location and time; size, shape and orientation; and litter density and composition, both within and out the windrow. In this regard, drones allow for a rapid characterization of the litter windrows. Visible-light sensors are able to provide details of macro-litter surface coverage across the convergence zones (Kako et al., 2020). As for the supplementary environmental information to be recorded, it should ideally include sea state, wind speed and direction, and at least a qualitative description of the surface current field. More targeted approaches to study litter windrows should comprise of monitoring it over time or conducting underwater analyses of vertical fine structure. The range of litter sizes in the windrows should be selectively arranged in circulation cells according to the specific buoyancy of the item.
One of the most relevant research lines relates to the implications of the litter windrows as hubs of planktonic organisms and attractants of nektonic animals. Existing information (e.g., Josse et al., 2000; Acha et al., 2015; Gove et al., 2019) suggests that litter windrows likely play a significant role in foraging process of pelagic predators, increasing the risks of litter ingestion, entanglement and toxicity for the food web. We call for greater attention to the possible impacts of the litter windrows on the functioning of the pelagic ecosystem. The behavior of pelagic predators in relation to litter windrows is potentially assessable by optical and acoustic sensors with minimal disturbance (Josse et al., 2000); and floating ghost nets or artificial windrows (e.g., made with strung litter items) might be used as long-lasting observational platforms for the monitoring of the biological activity around them.
Overall Picture
Litter windrows are aggregations of floating litter generally visible to the naked eye. They are formed from the wide variety of submesoscale processes inducing convergence zones on the ocean surface, particularly when these convergences take place in regions and periods with severe litter pollution. The formation and disintegration of windrows could be on the order of hours or days. So far, the most commonly observed shape of litter windrow is elongated, within a typical range of 1 km in length and a few meters in width, although its shape may evolve from small scattered patches to large quasi-circular clumps. These convergent structures have showed the highest concentrations of floating litter found in the ocean. We consider well-developed litter windrows as those exceeding 10 micro-items per m2 or 1 macro-item per 10 × 10 m2.
Ship-based studies focused on litter windrows are rare, especially those addressing their attracting effect on marine life. The shortage of field information is explained by the lack of knowledge on how to find litter windrows, and the fact that usually they are not properly investigated when encountered. On the other hand, aerial surveys have already demonstrated their potential for the large-scale mapping of macro-litter windrows (Lambert et al., 2020), and space-borne sensors are starting to deliver encouraging results (Figure 1).
Well-developed macro-litter windrows are presented as the greatest hope for the remote sensing of ocean litter and thus for the development of a global monitoring system. Given that dense windrows can only be formed from particularly high ambient litter loads, the detections of litter windrows may represent severe-pollution flags, useful for tagging highly polluted zones and periods of time, or for assessing the effectiveness of waste management plans.
The first and foremost approach against marine litter pollution is to prevent more litter from entering the ocean, and secondly, its capture at sea. Notwithstanding, the gathering of high loads of floating litter in patches raises expectations for mitigation actions at sea. Active fishing for windrows has proved capable of removing large amounts of litter from certain nearshore areas even in the absence of search guides (Ruiz et al., 2020). Ocean cleanups might be of interest when they prevent contamination of sensitive zones of high ecological and economic value, such as tourist beaches, marine protected areas, aquaculture facilities or shipping routes. However, it is imperative to conduct proper environmental impact studies before these actions are recommended. While sufficiently large mesh openings and slow tow speeds may be useful in reducing the catch of fish, turtles and mammals, any mechanical cleanup on ocean surface might also remove drifting biomass (e.g., seaweed, coastal vegetation), as well as biota attached to floating items, including eggs of fishes or others. Alas, we have dumped huge amounts of waste into the ocean when we still know little about the ecological role of the aggregations of natural debris.
This Perspective advocates targeted windrow research to advance the understanding of marine litter pollution. We have appropriate tools for the physical-chemical and biological characterization of windrows, the main challenge for the field surveys remains the uncertainty about the areas and periods of litter-windrow formation. This gap could be addressed through a combination of modeling, aerial studies and remote sensing. Either way, any progress in the prediction and detection on a large scale would open up great prospects for research and management of marine litter.
Data Availability Statement
Publicly available datasets were analyzed in this study. This data can be found here: https://www.pnas.org/content/116/48/24143/tab-figures-data; https://www.frontiersin.org/articles/10.3389/fmars.2020.00308/full.
Author Contributions
AC drafted the manuscript. All authors contributed to delineate the research and wrote the manuscript.
Funding
This study is an outcome of the research project entitled “Mapping Windrows as Proxy for Marine Litter Monitoring from Space” (WASP), funded by the European Space Agency (ESA) Contract No. 4000130627/20/NL/GLC, within the Discovery Campaign in Marine Litter. AC had additional support from MIDaS (CTM2016- 77106-R, AEI/FEDER/UE), and SA from PRIN 2017-2017WERYZP-EMME project. AI was supported by the Environmental Research and Technology Development Fund (JPMEERF18S20201) of the Ministry of the Environment, Japan, and by SATREPS of Japan International Cooperation Agency and Japan Science and Technology Agency. OB and AR contribution was funded through the EU’s LIFE Program (LIFE LEMA project, grant agreement no. LIFE15 ENV/ES/000252). This is contribution number 1016 of AZTI, Marine Research, Basque Research and Technology Alliance (BRTA).
Conflict of Interest
The authors declare that the research was conducted in the absence of any commercial or financial relationships that could be construed as a potential conflict of interest.
References
Acha, E. M., Mianzan, H. W., Iribarne, O., Gagliardini, D. A., Lasta, C., and Daleo, P. (2003). The role of the Río de la Plata bottom salinity front in accumulating debris. Mar. Pollut. Bull. 46, 197–202. doi: 10.1016/s0025-326x(02)00356-9
Acha, E. M., Piola, A., Iribarne, O., and Mianzan, H. (2015). Ecological Processes at Marine Fronts. Oases in the Ocean. Cham: Springer International Publishing, 68.
Bruno, M., Alonso, J. J., Cózar, A., Vidal, J., Ruiz-Cañavate, A., Echevarrıa, F., et al. (2002). The boiling-water phenomena at Camarinal Sill, the Strait of Gibraltar. Deep Sea Res. Part II 49, 4097–4113. doi: 10.1016/s0967-0645(02)00144-3
Coston-Clements, L., Settle, L. R., Hoss, D. E., and Cross, F. A. (1991). Utilization of the Sargassum habitat by marine invertebrates and vertebrates. A review. NOAA Tech. Mem. NMFS SEFSC 296, 32.
Cózar, A., Echevarría, F., González-Gordillo, J. I., Irigoien, X., Úbeda, B., Hernández-León, S., et al. (2014). Plastic debris in the open ocean. Proc. Natl. Acad. Sci. U.S.A. 111, 10239–10244. doi: 10.1073/pnas.1314705111
Cózar, A., Martí, E., Duarte, C. M., García-de-Lomas, J., van Sebille, E., Ballatore, T. J., et al. (2017). The Arctic Ocean as a dead end for floating plastics in the North Atlantic branch of the Thermohaline circulation. Sci. Adv. 3:e1600582. doi: 10.1126/sciadv.1600582
Cózar, A., Sanz-Martín, M., Martí, E., González-Gordillo, J. I., Ubeda, B., Gálvez, J. A., et al. (2015). Plastic accumulation in the Mediterranean Sea. PLoS One 10:e0121762. doi: 10.1371/journal.pone.0121762
Craik, A. (1970). A wave-interaction model for the generation of windrows. J. Fluid Mech. 41, 801–821. doi: 10.1017/s0022112070000939
Darwin, C. R. (1839). Narrative of the surveying voyages of His Majesty’s Ships adventure and Beagle between the years 1826 and 1836, describing their examination of the southern shores of South America, and the Beagle’s circumnavigation of the globe. J. Remarks 3, 1832–1836.
D’Asaro, E. A., Shcherbina, A. Y., Klymak, J. M., Molemaker, J., Novelli, G., Guigand, C. M., et al. (2018). Ocean convergence and the dispersion of flotsam. Proc. Natl. Acad. Sci. U.S.A. 115, 1162–1167. doi: 10.1073/pnas.1718453115
Duncan, E. M., Davies, A., Brooks, A., Chowdhury, G. W., Godley, B. J., Jambeck, J., et al. (2020). Message in a bottle: open source technology to track the movement of plastic pollution. PLoS ONE 15:e0242459. doi: 10.1371/journal.pone.0242459
Eriksen, M., Lebreton, L., Carson, H. S., Thiel, M., Moore, C. J., Borerro, J. C., et al. (2014). Plastic pollution in the world’s oceans: more than 5 trillion plastic pieces weighing over 250,000 tons afloat at sea. PLoS One 9:e111913. doi: 10.1371/journal.pone.0111913
Faller, A. J., and Woodcock, A. H. (1964). The spacing of windrows of Sargassum in the ocean. J. Mar. Res 22, 22–29.
Feng, Z., Zhang, T., Shi, H., Gao, K., Huang, W., Xu, J., et al. (2020). Microplastics in bloom-forming macroalgae: distribution, characteristics and impacts. J. Hazard. Mater. 397:e122752.
Galgani, F., Hanke, G., and Maes, T. (2015). “Global distribution, composition and abundance of marine litter,” in Marine Anthropogenic Litter, eds M. Bergmann, L. Gutow, and M. Klages, (Cham: Springer), 29–56. doi: 10.1007/978-3-319-16510-3_2
Goldstein, M. C., Rosenberg, M., and Cheng, L. (2012). Increased oceanic microplastic debris enhances oviposition in an endemic pelagic insect. Biol. Lett. 8, 817–820. doi: 10.1098/rsbl.2012.0298
Goldstein, M. C., Titmus, A. J., and Ford, M. (2013). Scales of spatial heterogeneity of plastic marine debris in the Northeast Pacific Ocean. PLoS One 8:e80020. doi: 10.1371/journal.pone.0080020
Gove, J. M., Whitney, J. L., McManus, M. A., Lecky, J., Carvalho, F. C., Lynch, J. M., et al. (2019). Prey-size plastics are invading larval fish nurseries. Proc. Natl. Acad. Sci. U.S.A. 116, 24143–24149. doi: 10.1073/pnas.1907496116
Haney, J. C. (1986). Seabird patchiness in tropical oceanic waters: the influence of Sargassum “reefs”. Auk 103, 141–151. doi: 10.1093/auk/103.1.141
Hennen, M., Arias, M., Aliani, S., Cózar, A., Jacobs, C., Topouzelis, K., et al. (2019). EO Tracking of Marine Debris in the Mediterranean Sea from Public Satellites. Final Report. ESA Contract No. 4000124861/18/I-NB. Paris: European Space Agency, 31.
Isobe, A., Kubo, K., Tamura, Y., Kako, S., Nakashima, E., and Fujii, N. (2014). Selective transport of microplastics and mesoplastics by drifting in coastal waters. Mar. Pollut. Bull. 89, 324–330. doi: 10.1016/j.marpolbul.2014.09.041
Isobe, A., Uchida, K., Tokai, T., and Iwasaki, S. (2015). East Asian seas: a hot spot of pelagic microplastics. Mar. Pollut. Bull. 101, 618–623. doi: 10.1016/j.marpolbul.2015.10.042
Josse, E., Dagorn, L., and Bertrand, A. (2000). Typology and behaviour of tuna aggregations around fish aggregating devices from acoustic surveys in French Polynesia. Aquat. Living Resour. 13, 181–190.
Kako, S., Morita, S., and Taneda, T. (2020). Estimation of plastic marine debris volumes on beaches using unmanned aerial vehicles and image processing based on deep learning. Mar. Pollut. Bull. 155, 111127. doi: 10.1016/j.marpolbul.2020.111127
Kukulka, T., Proskurowski, G., Morét-Ferguson, S., Meyer, D. W., and Law, K. L. (2012). The effect of wind mixing on the vertical distribution of buoyant plastic debris. Geophys. Res. Lett. 39:L07601.
Lambert, C., Authier, M., Doremus, G., Laran, S., Panigada, S., Spitz, J., et al. (2020). Setting the scene for Mediterranean litterscape management: the first basin-scale quantification and mapping of floating marine debris. Environ. Pollut. 263:e114430.
Langmuir, I. (1938). Surface motion of water induced by wind. Science 87, 119–123. doi: 10.1126/science.87.2250.119
Law, K. L., Morét-Ferguson, S. E., Goodwin, D. S., Zettler, E. R., DeForce, E., Kukulka, T., et al. (2014). Distribution of surface plastic debris in the eastern Pacific ocean from an 11-year data set. Environ. Sci. Technol. 48, 4732–4738. doi: 10.1021/es4053076
Law, K. L., Morét-Ferguson, S. E., Maximenko, N., Proskurowski, G., Peacock, E. E., Hafner, J., et al. (2010). Plastic accumulation in the North Atlantic Subtropical Gyre. Science 329, 1185–1188. doi: 10.1126/science.1192321
Lebreton, L., Slat, B., Ferrari, F., Sainte-Rose, B., Aitken, J., Marthouse, R., et al. (2018). Evidence that the Great Pacific Garbage Patch is rapidly accumulating plastic. Sci. Rep. 8:e4666.
Liebovich, S. (1983). The form and dynamics of Langmuir circulations. Ann. Rev. Fluid Mech. 15, 391–427. doi: 10.1146/annurev.fl.15.010183.002135
Macías, D., Cózar, A., Garcia-Gorriza, E., González-Fernández, D., and Stips, A. (2019). Surface water circulation develops seasonally changing patterns of floating litter accumulation in the Mediterranean Sea. A modelling approach. Mar. Pollut. Bull. 149, e110619.
Martí, E., Martin, C., Cózar, A., and Duarte, C. M. (2017). Low abundance of plastic fragments in the surface waters of the Red Sea. Front. Mar. Sci. 4:e00333. doi: 10.3389/fmars.2017.00333
Maximenko, N., Hafner, J., and Niiler, P. (2012). Pathways of marine debris derived from trajectories of Lagrangian drifters. Mar. Pollut. Bull. 65, 51–62. doi: 10.1016/j.marpolbul.2011.04.016
Miyao, Y., and Isobe, A. (2016). Combined balloon photography and buoy-tracking experiment for mapping surface currents in coastal waters. J. Atmos. Ocean. Technol. 33, 1237–1250. doi: 10.1175/jtech-d-15-0113.1
Ody, A., Thibaut, T., Berline, L., Changeux, T., André, J.-M., Chevalier, C., et al. (2019). From In Situ to satellite observations of pelagic Sargassum distribution and aggregation in the Tropical North Atlantic Ocean. PLoS One 14:e0222584. doi: 10.1371/journal.pone.0222584
Ory, N. C., Lehmann, A., Javidpour, J., Stöhr, R., Walls, G. L., and Clemmesen, C. (2020). Factors influencing the spatial and temporal distribution of microplastics at the sea surface. A year-long monitoring case study from the urban Kiel Fjord, southwest Baltic Sea. Sci. Total Env. 736:139493. doi: 10.1016/j.scitotenv.2020.139493
Pedrotti, M. L., Petit, S., Elineau, A., Bruzaud, S., Crebassa, J. C., Dumontet, B., et al. (2016). Changes in the floating plastic pollution of the Mediterranean Sea in relation to the distance to land. PLoS One 11:e0161581. doi: 10.1371/journal.pone.0161581
Pineda, A. (1789). Narrative of the voyage around the world by D. A. P. R. aboard His Majesty’s corvette La Descubierta, extracted from his diary, notes and accounts (in Spanish). Arch. Museo Naval, Madrid, doc. Expedición Malaspina, ms. 122, 236–293.
Reisser, J., Shaw, J., Wilcox, C., Hardesty, B. D., Proietti, M., Thums, M., et al. (2013). Marine plastic pollution in waters around Australia: characteristics, concentrations, and pathways. PLoS One 8:e80466. doi: 10.1371/journal.pone.0080466
Ruiz, I., Basurko, O. C., Rubio, A., Delpey, M., Granado, I., Declerck, A., et al. (2020). Litter windrows in the south-east coast of the Bay of Biscay: an ocean process enabling effective active fishing for litter. Front. Mar. Sci. 7:308. doi: 10.3389/fmars.2020.00308
Ruiz-Orejón, L. F., Sardá, R., and Ramis-Pujol, J. (2016). Floating plastic debris in the Central and Western Mediterranean Sea. Mar. Environ. Res. 120, 136–144. doi: 10.1016/j.marenvres.2016.08.001
Ryan, P. G. (2013). A simple technique for counting marine debris at sea reveals steep litter gradients between the Straits of Malacca and the Bay of Bengal. Mar. Pollut. Bull. 69, 128–136. doi: 10.1016/j.marpolbul.2013.01.016
Shanks, A. L. (1983). Surface slicks associated with tidally forced internal waves may transport pelagic larvae of benthic invertebrates and fishes shoreward. Mar. Ecol. Prog. Ser. 13, 311–315. doi: 10.3354/meps013311
Shanks, A. L. (1987). The onshore transport of an oil spill by internal waves. Science 235, 1198–1200. doi: 10.1126/science.235.4793.1198
Suaria, G., Avio, C., Mineo, A., Lattin, G. L., Magaldi, M. G., Belmonte, G., et al. (2016). The Mediterranean plastic soup: synthetic polymers in Mediterranean surface waters. Sci. Rep. 6:e3755.
Thiel, M., and Gutow, L. (2005). The ecology of rafting in marine environment. II. The rafting organisms and community. Oceanogr. Mar. Biol. Annu. Rev. 43, 279–418. doi: 10.1201/9781420037449.ch7
Thomson, J. (1862). On the calm lines often seen on a rippled sea. Philos. Mag. Ser. 4, 247–248. doi: 10.1080/14786446208643350
Topouzelis, K., Papakonstantinou, A., and Garaba, S. P. (2019). Detection of floating plastics from satellite and unmanned aerial systems (Plastic Litter Project 2018). Int. J. Appl. Earth Obs. Geoinf. 79, 175–183. doi: 10.1016/j.jag.2019.03.011
van der Hal, N., Ariel, A., and Angel, D. L. (2017). Exceptionally high abundances of microplastics in the oligotrophic Israeli Mediterranean coastal waters. Mar. Pollut. Bull. 116, 151–155. doi: 10.1016/j.marpolbul.2016.12.052
van Sebille, E., Aliani, S., Law, K. L., Maximenko, N., Alsina, J. M., Bagaev, A., et al. (2020). The physical oceanography of the transport of floating marine debris. Environ. Res. Lett. 15:e023003.
van Sebille, E., Wilcox, C., Lebreton, L., Maximenko, N., Hardesty, B. D., van Franeker, J. A., et al. (2015). A global inventory of small floating plastic debris. Environ. Res. Lett. 10:e124006.
Yoon, J. H., Kawano, S., and Igawa, S. (2010). Modeling of marine litter drift and beaching in the Japan Sea. Mar Poll Bull. 60, 448–463. doi: 10.1016/j.marpolbul.2009.09.033
Keywords: marine litter, floating plastic, ocean surface, submesoscale, litter impacts, remote sensing, management
Citation: Cózar A, Aliani S, Basurko OC, Arias M, Isobe A, Topouzelis K, Rubio A and Morales-Caselles C (2021) Marine Litter Windrows: A Strategic Target to Understand and Manage the Ocean Plastic Pollution. Front. Mar. Sci. 8:571796. doi: 10.3389/fmars.2021.571796
Received: 11 June 2020; Accepted: 26 January 2021;
Published: 24 February 2021.
Edited by:
Christopher Edward Cornwall, Victoria University of Wellington, New ZealandReviewed by:
Martin Thiel, Catholic University of the North, ChileTamay Ozgokmen, University of Miami, United States
Annalisa Bracco, Georgia Institute of Technology, United States
Tomaso Fortibuoni, Higher Institute for Environmental Protection and Research (ISPRA), Italy
Copyright © 2021 Cózar, Aliani, Basurko, Arias, Isobe, Topouzelis, Rubio and Morales-Caselles. This is an open-access article distributed under the terms of the Creative Commons Attribution License (CC BY). The use, distribution or reproduction in other forums is permitted, provided the original author(s) and the copyright owner(s) are credited and that the original publication in this journal is cited, in accordance with accepted academic practice. No use, distribution or reproduction is permitted which does not comply with these terms.
*Correspondence: Andrés Cózar, YW5kcmVzLmNvemFyQHVjYS5lcw==