- 1Department of Environmental and Earth Sciences (DISAT), University of Milano – Bicocca, Milan, Italy
- 2Marine Research and High Education Center (MaRHE Center), Magoodhoo, Maldives
- 3ENTROPIE, IRD, Université de la Réunion, CNRS, IFREMER, Université de Nouvelle-Calédonie, Nouméa, France
- 4Centre Scientifique de Monaco (CSM), Equipe Ecophysiologie Corallienne, Monaco, Monaco
- 5Department of Biotechnologies and Biosciences, University of Milano – Bicocca, Milan, Italy
The intensity and frequency of coral bleaching events have increased worldwide especially due to thermal stress and seawater pollution. Although it has been observed that metal concentration in seawater can affect the coral’s ability to adopt cellular defensive mechanisms to counteract bleaching, more investigations are needed to better understand the role of metals in coral physiology. In this study, we analyzed the individual and combined effects of prolonged heat stress and manganese (Mn) and iron (Fe) enrichments at the cellular level on the coral Stylophora pistillata. Thermal stress caused an up-regulation in the expression of the host Heat shock proteins (Hsps) 60 and 70, which showed a parallel pattern of modulation in all treatments, as well as an increase of lipid peroxidation (LPO) in the holobiont. Fe enrichment did not induce any change in Hsp expression or in the oxidative status of the corals both at the ambient temperature of 26°C or at increased temperature, suggesting that Fe didn’t seem to play a role in mitigating the cellular damages and the coral bleaching. Mn or MnFe enrichment at 26°C seemed to increase the oxidative stress in zooxanthellae, since high LPO and glutathione reductase (GR) levels were recorded, but it did not cause any effect on polyp Hsp expression, probably due to the antioxidant action of GR. With the temperature increase, Mn enrichments prevented any increase in Hsp levels and caused a significant decrease of LPO and GR activity, strengthening a previous hypothesis suggesting that Mn could mitigate the negative cellular effects produced by the thermal stress.
Introduction
Over the last decades, coral bleaching events have become more frequent and severe, representing a persistent threat to reef health (Oliver et al., 2018; Eakin et al., 2019). During these events, the symbiotic relationship between corals and their photosynthetic algae is disrupted, causing hosts to lose their color and ultimately to die (Brown, 1997). Although bleaching can be caused by multiple stressors (Lesser, 2011), rising seawater temperature unequivocally represents the main bleaching-inducing factor (Hughes et al., 2017; Lough et al., 2018).
Thermal stress may elicit different types of damage within coral and symbiont cells (Hoegh-Guldberg, 1999; Weis, 2008). It may also disrupt the inter-partner molecular and cell signaling, causing the impairment of the cellular and physiological mechanisms that maintain the partnership homeostasis (Davy et al., 2012; Cleves et al., 2020; Rosset et al., 2020; Suggett and Smith, 2020; Williams et al., 2021). In this context, the production of reactive oxygen species (ROS) by heat-stressed algal chloroplasts as well as by host cells increases the oxidative stress of the coral holobiont and has been proposed to be an initiating cause of a series of events leading to symbiont loss (Downs et al., 2002; Weis, 2008; Oakley and Davy, 2018; Cziesielski et al., 2019). However, recent studies have found that ROS production by the symbiont does not seem to be the direct cause of bleaching, highlighting the need for further investigations (Nielsen et al., 2018; Rädecker et al., 2021).
In addition, local increases in the level of seawater pollutants, in particular that of metals, are another significant stress source for corals (Goh, 1991; van Dam et al., 2011; Ranjbar Jafarabadi et al., 2018; Patterson et al., 2020). Metals like iron, copper, and manganese are required at trace concentrations for many cellular processes, such as antioxidant defenses, and for the physiological functions, especially in photosynthetic organisms (Merchant, 2009; DalCorso et al., 2014; Reich et al., 2020). However, when metal concentrations reach or exceed the threshold level of tolerance, which is species and site-specific, processes such as coral growth and reproduction, larval development, photosynthetic efficiency and calcification can be compromised (Bielmyer-Fraser et al., 2010; Negri and Hoogenboom, 2011; Biscéré et al., 2015; Reichelt-Brushett and Hudspith, 2016; Gissi et al., 2017). At cellular level, exposure to metals can lead to oxidative stress and subsequent damages of both host and symbiont cells (Mitchelmore et al., 2007; Vijayavel et al., 2012; Siddiqui and Bielmyer-Fraser, 2015; Marangoni et al., 2017). In addition, metal pollution acts in synergy with thermal stress and further compromises different coral physiological pathways (Nyström et al., 2001; Shick et al., 2011; Kwok and Ang, 2013; Ferrier-Pagès et al., 2018; Reich et al., 2020). In this regard, an increased bleaching susceptibility of scleractinian corals has been observed when subjected to the combined effects of thermal stress and copper or nickel enrichment (Biscéré et al., 2017; Fonseca et al., 2017; Banc-Prandi and Fine, 2019). Also, iron enrichment has been shown to impair calcification and induce significant bleaching (Wells et al., 2011; Biscéré et al., 2018). On the contrary, manganese enrichment may alleviate the deleterious effect of heat stress on corals, by enhancing chlorophyll concentrations, photosynthetic efficiency, and gross photosynthetic rates at ambient and elevated temperature (Biscéré et al., 2018). All together, these studies suggest that the effects of metals on coral physiology are metal and concentration-dependent, and that interactions between metals also exist. Therefore, more investigations are needed to better understand the role of metals in coral physiology. In this context, since changes at cellular level are the first detectable response of an organism to an environmental perturbation (Bierkens, 2000; Kültz, 2005), the analysis of cellular stress biomarkers may represent a useful diagnostic tool, able to reflect changes in cellular integrity and pathways due to trace metals before larger scale physiological processes are affected (Downs, 2005; Seveso et al., 2017).
Heat shock proteins (Hsps) are molecular chaperones and are part of a cytoprotective mechanism able to mitigate the deleterious effects of stressors, and have consequently been frequently adopted as cellular stress biomarkers in corals (Downs et al., 2000, 2005; Chow et al., 2012; Kenkel et al., 2014; Louis et al., 2017; Seveso et al., 2018). Hsps maintain protein homeostasis by facilitating proper protein folding and translocation, deterring and/or reducing the aggregation of other proteins, and assisting in refolding or degrading stress-damaged proteins (Balchin et al., 2016). Hsp expression is usually up-regulated when organisms face conditions that may affect their cellular protein structure. Hsps are involved in the coral heat stress-induced bleaching response, while acting as a protection from ROS toxicity (Downs et al., 2002; DeSalvo et al., 2008; Seneca et al., 2010; Mayfield et al., 2011; Olsen et al., 2013; Seveso et al., 2016, 2020). However, to date very few studies have analyzed their expression in corals subjected to metal pollution (Venn et al., 2009). In addition, corals produce antioxidant enzymes in order to neutralize the harmful effects of ROS caused by metals and other stressors, and they also represent useful oxidative stress biomarkers (Lesser, 2006; Dias et al., 2019b, 2020; Marangoni et al., 2019b). Among these, superoxide dismutase (SOD) works as the first line of defense in the detoxification process, reducing the damaging potential of superoxide anion radicals (O2•−) by catalyzing its dismutation to hydrogen peroxide (H2O2) (Lesser, 2006). Glutathione reductase (GR) catalyzes the NADPH-dependent reduction of glutathione disulfide (GSSG) to the sulfhydryl form glutathione (GSH), which is a critical molecule in counteracting oxidative stress and maintaining the reducing environment of the cell (Deponte, 2013). However, if ROS production is higher than the antioxidant system reductive potential, ROS may lead to cellular damage, including lipid peroxidation (LPO) (Gaschler and Stockwell, 2017).
A previous work dealing with the effect of manganese and/or iron enrichment in combination with thermal stress has shown interesting results on coral physiology, particularly with reference to the potential role of manganese in the reduction of heat stress impact, likely due to its involvement in the antioxidant system (Biscéré et al., 2018). Starting from these statements, we performed on the same coral samples an in-depth analysis of the cellular mechanisms underlying the response observed by Biscéré et al. (2018), in order to better understand the role of manganese and iron, which is poorly elucidated when compared to that of other metals (Schwarz et al., 2013; Biscéré et al., 2017; Bielmyer-Fraser et al., 2018; Zhou et al., 2018; Marangoni et al., 2019c). In particular, in this study we analyzed the individual and combined effects of prolonged heat stress and metal enrichment (Mn and Fe) at the cellular level on the coral Stylophora pistillata, by investigating changes in the host cellular protein homeostasis, and the holobiont oxidative status. In this regard, the expression of the cytoplasmic Hsp70 and the mitochondrial Hsp60 in the coral polyps, as well as the LPO levels and the SOD and GR enzymatic activity in the holobiont were measured. In the frame of the photophysiological parameters determined by Biscéré et al. (2018) on the same samples, the data presented here support a possible role of Mn in mitigating the physiological impact of heat stress causing bleaching.
Materials and Methods
Maintenance and Growth Condition of Corals
The experimental setup as well as the biological samples analyzed in this study corresponds to those reported in Biscéré et al. (2018). In particular, experiments were performed with small apexes (∼2 cm long) of the scleractinian species S. pistillata, obtained from several large mother colonies originally sampled in the Red Sea. Nubbins were kept in 25 l tanks for 3 weeks under controlled conditions in order to recover, as described in Biscéré et al. (2018). Briefly, tanks were supplied with seawater pumped from 40 m depth, with a 100% water turnover every 12 h. The photoperiod was generated by 400 W metal halide lamps (HPIT, Philips) at an irradiance of 200 ± 10 μmol photons m–2 s–1 switching from dark to light every 12 h. Light was controlled by a LI-COR data logger (LI-1000) connected to a spherical quantum sensor (LI-193) once a week. Temperature was set to an optimum of 26°C (±0.2°C) and was controlled along the experiment through heaters connected to the controllers (Elli-Well PC902/T). Corals were fed with nauplii of Artemia salina twice a week.
Experimental Design and Stress Exposure
After the recovery period, eight different experimental conditions were set up in duplicated aquaria by combining two seawater temperatures and two concentrations of Mn and Fe (Biscéré et al., 2018). Specifically, four different metal conditions were reproduced as follows: (1) Control, with Mn and Fe values of oligotrophic seawaters, respectively, 0.06 ± 0.05 μg/l Mn (Morley et al., 1997) and <0.22 μg/l Fe (Ferrier-Pagès et al., 2001); (2) Manganese enrichment (+Mn) to reach 4.1 ± 0.2 μg/l; (3) Iron enrichment (+Fe) to reach 3.0 ± 0.2 μg/l; (4) Manganese and iron enrichment (+MnFe) at the two respective concentrations indicated above. Mn and Fe enrichments were chosen based on the highest levels recorded for these metals in some fringing reefs along the coasts of New Caledonia (Moreton et al., 2009).
The metal enrichment was performed using two peristaltic pumps (ISMATEC), which continuously supplied the experimental tanks with 15 ml/h of a solution of stable Mn (MnCl2, Humeau, France) or stable Fe (FeCl2, Humeau, France). The divalent form was chosen for Fe and Mn, since Fe2+ and Mn2+ are considered the most soluble and bioavailable forms (Fairbrother et al., 2017). In addition, submersible pumps (Aquarium system, micro-jet MC 320, Mentor, OH, United States) were placed in each tank in order to avoid any metal concentration gradient and to ensure water circulation and oxygenation. To make sure that the metal concentrations in the tanks were the desired ones, seawater samples were collected and analyzed by sector field inductively coupled plasma-mass spectrometry (SF-ICP-MS) using a Thermo Element 2 instrument, as previously described (Biscéré et al., 2018).
Temperature was kept constant at 26°C in all tanks for the first 3 weeks of treatments, during which corals in the +Mn, +Fe, and +MnFe tanks were subject to metals enrichment as described above. After 3 weeks, temperature was gradually increased during 1 week to 32°C (1°C per day) in half of the tanks and maintained for an additional 2 week period without stopping metal enrichment (Biscéré et al., 2018). After 6 weeks from the beginning of the experiment, and thus after 3 weeks of thermal stress, five nubbins per condition were collected and immediately frozen to −80°C for further analyses.
Analysis of the Hsp Expression
Protein Extract Preparation and Western Analyses
To extract total proteins, frozen coral apexes were powdered with a pre-chilled mortar and pestle and homogenized in SDS-buffer (0.0625 M Tris–HCl, pH 6.8, 10% glycerol, 2.3% SDS, 5% 2-mercaptoethanol) containing 1 mM phenylmethylsulfonylfluoride (Sigma-Aldrich), and complete EDTA-free protease inhibitors cocktail (Roche Diagnostic). Samples were then processed following a previous method that allows removing, in addition to calcium carbonate debris, any Symbiodiniaceae contamination, obtaining extracts that contain only polyp proteins (Seveso et al., 2013, 2014). Extracts were stored at −80°C until further processing. Aliquots were used to determine total protein concentrations through Bio-Rad protein assay kit (Bio-Rad Laboratories). An equal amount of proteins for each sample was separated by SDS-PAGE on 8% polyacrylamide gels (Vai et al., 1986), then run in duplicates using a Mini-Protean Tetra Cell (Bio-Rad Laboratories). After the electrophoresis, a gel was stained with Coomassie Brilliant Blue to visualize total proteins, while the other was electroblotted onto nitrocellulose membrane (Amersham Protran 0,45 mm) for Western Blot analysis as previously described (Seveso et al., 2012). Filters were stained with Ponceau S Red (Sigma-Aldrich) to confirm correct protein transfer. The following primary antibodies were used: anti-Hsp70 monoclonal antibody (IgG2a mouse clone BB70, SPA-822, Enzo Life Sciences), anti-Hsp60 monoclonal antibody (IgGI1 mouse clone LK-2, SPA-807, Enzo Life Sciences) and anti-β-Actin monoclonal antibody (IgG1k mouse clone C4, MAB1501, Millipore). The primary antibodies were diluted 1:1,000 in TBS-0.1% Tween 20 and 5% skimmed milk, with the exception of β-Actin, diluted 1:3000. After being washed three times with fresh changes of TBS-0.1% Tween 20 (15 min each), filters were incubated with anti-mouse IgG polyclonal secondary antibodies conjugated with horseradish peroxidase (ADI-SAB-100, Enzo Life Sciences) diluted 1:1,000 for Hsp70 and 1:10,000 for Hsp60 and 1:15,000 for β–Actin in TBS-0,3% Tween 20 and 5% skimmed milk. Western blots were developed using Pierce ECL Western Blotting Substrate followed by exposition of filters to Amersham Hyperfilm ECL.
Densitometric Analysis
Densitometric analysis was performed as previously described (Seveso et al., 2013). Films were scanned on a Bio-Rad GS-800 calibrated imaging densitometer and the pixel density of the scanned bands were quantified with the ImageJ free software1 of the NIH Image software package (National Institutes of Health, Bethesda, Md.). For each blot, the scanned intensity of the bands of each Hsp was normalized against the intensity of the β-Actin ones, which was used as an internal loading control since in all our experiments the β-Actin level did not display significant changes (Supplementary Figure 1). The densitometric data were expressed as relative levels (arbitrary units).
Analysis of the Antioxidant Enzymatic Activities
Protein Extraction
Coral fragments reduced into powder with pre-chilled mortar and pestle were transferred into tubes and homogenized in 750 μl lysis buffer (Tris–HCl 50 mM, pH 7.4, NaCl 150 mM, glycerol 10%, NP40 detergent 1%, EDTA 5 mM) containing 1 mM phenylmethylsulfonylfluoride (Sigma-Aldrich). After a first centrifugation step (5 min, 3,000 rpm) to remove skeletal components, cells were broken by sonication (6 × 10 s pulse on ice, amplitude 10 μm, Soniprep 150, Sanyo). Samples were then subjected to a second centrifugation step (15 min, 14,000 rpm, 4°C) and the supernatant was sampled and frozen immediately (−80°C) until subsequent assays. Total protein content of each sample was determined through the Bradford method using bovine serum albumin (BSA) as a calibration curve.
Superoxide Dismutase Activity Assay
Superoxide dismutase activity was assessed according to Vance et al. (1972). As SOD competes with ferricytochrome c for oxygen radicals, its activity was detected as the ability to inhibit the reduction of ferricytochrome c by O2– generated from the xanthine/xanthine oxidase system. For the reaction mix, the following reagents (purchased from Sigma-Aldrich), ferricytochrome c 0.01 mM, EDTA 0.1 mM, xanthine 0.01 mM, and xanthine oxidase 0.0061 U were used in a final volume of 1 ml. Different volumes of each sample were tested and added to the reaction mix to determine the 50% inhibition of the reaction rate. The rate of reduction of ferricytochrome c was followed spectrophotometrically at 550 nm, 25°C, through a Varian Cary 50 Scan Spectrophotometer (Agilent Technologies). Under the above conditions, one unit of SOD was defined as the amount of enzyme inhibiting the reduction of ferricytochrome c by 50%. Results are expressed as units (U) of enzyme per mg of proteins.
Glutathione Reductase Activity Assay
The enzymatic assay of GR was performed according to Wang et al. (2001). The activity of GR was evaluated through the spectrophotometric detection of the absorbance at 340 nm (Varian Cary 50 Scan spectrophotometer, Agilent Technologies) of NADPH oxidation to NADP + reaction, which occurs in conjunction with the glutathione reduction, and is proportional to the decrease in absorbance over time. In particular, NADPH reaction was initially detected in the reaction mix (containing 0.1 M potassium phosphate buffer pH 7.6, 0.16 mM NADPH, 1 mg ml–1 BSA, and 4.6 mM oxidized glutathione), and then adding different volumes of sample. GR activity was obtained from the difference of the two absorbance values. One unit of GR activity was defined as the oxidation of 1 nmol NADPH/min at 25°C. Results are expressed as units (U) of enzyme per mg of proteins.
Lipid Peroxidation
Lipid peroxidation levels were assessed via malondialdehyde (MDA) contents determined using a commercially available MDA assay kit (Bioxytech LPO-586, Oxis International, United States). The method is based on the reaction of a chromogenic reagent, N-methyl-2-phenylindole, with MDA at 45°C. Specifically, frozen coral apexes (approximately 1 g each) were reduced into powder with pre-chilled mortar and pestle and homogenized in 1 ml of 20 mM phosphate buffer, pH 7.4. To prevent sample oxidation, 10 μl 0.5 M butylated hydroxytoluene in acetonitrile was added to 1 ml of tissue homogenate. Following sample centrifugation (3,000 × g at 4°C for 10 min), an aliquot of supernatant was used for protein determination using the Bradford method. The subsequent assay procedure (hydrochloric acid solvent procedure) was performed according to the manufacturer’s instructions. The blue product was quantified by measuring absorbance at 586 nm (Gérard-Monnier et al., 1998). Results are presented in μmol of MDA per μg of proteins.
Data Analysis
Data normality was verified using the Shapiro–Wilk test and where assumptions were violated, the data were corrected by transformations. To evaluate significant differences in Hsp expression, enzymatic activity and LPO in corals subjected to metal stress at both ambient temperature (26°C) and following heat stress (32°C), separated one-way ANOVAs followed by Tukey’s HSD post hoc tests were used. The same analysis was adopted in order to assess significant differences in the levels of each biomarker in coral colonies subjected to thermal stress within each metal treatment. All statistical analyses were performed using SPSS ver. 26 (IBM). Values were considered statistically significant at p < 0.05, and all data are presented as arithmetic means ± SE (n = 4, for each biomarker analyzed), unless stated otherwise.
A multivariate analysis was performed using the statistical package PRIMER-E v.7 (Clarke and Gorley, 2015) with the permutational multivariate analysis of variance (PERMANOVA) + add on (Anderson et al., 2008) to investigate together the modulation of all biomarkers in response to metal and thermal stresses. In particular, data related to the levels of all the biomarkers were normalized and square root transformed to calculate a matrix based on the Bray-Curtis similarity. To test for differences in biomarker levels among temperature and treatments and the combination of both, a non-parametric PERMANOVA was performed using 999 permutations with partial sum of squares and unrestricted permutation of raw data. Temperature and treatment were selected as fixed factors. Values were considered statistically significant at p < 0.05. Pairwise PERMANOVA tests were conducted to assess differences between treatments at 26 and 32°C and between temperatures within each metal treatment, as performed for the univariate analyses. Due to the restricted number of unique permutations in the pairwise tests, p-values were also obtained from Monte Carlo samplings (Anderson and Robinson, 2003). To visualize similarities among metal treatments and temperature responses, a non-metric multidimensional scaling plot (nMDS) was done using Bray-Curtis similarity.
Results
Hsp Expression
The expression of Hsp70 and Hsp60 in the coral S. pistillata was investigated in different temperature conditions and Mn-Fe concentrations, applied alone and in combinations. Each monoclonal antibody (anti-Hsp70 and anti-Hsp60) produced in all samples a single specific band (Supplementary Figure 1), whose molecular weight corresponds to that expected based on the amino acid sequences (Seveso et al., 2020). Moreover, both Hsps showed common and comparable patterns of expression in each treatment, and thus responded in a similar way to single and combined stressors (Figure 1), further confirming that they work in tandem.
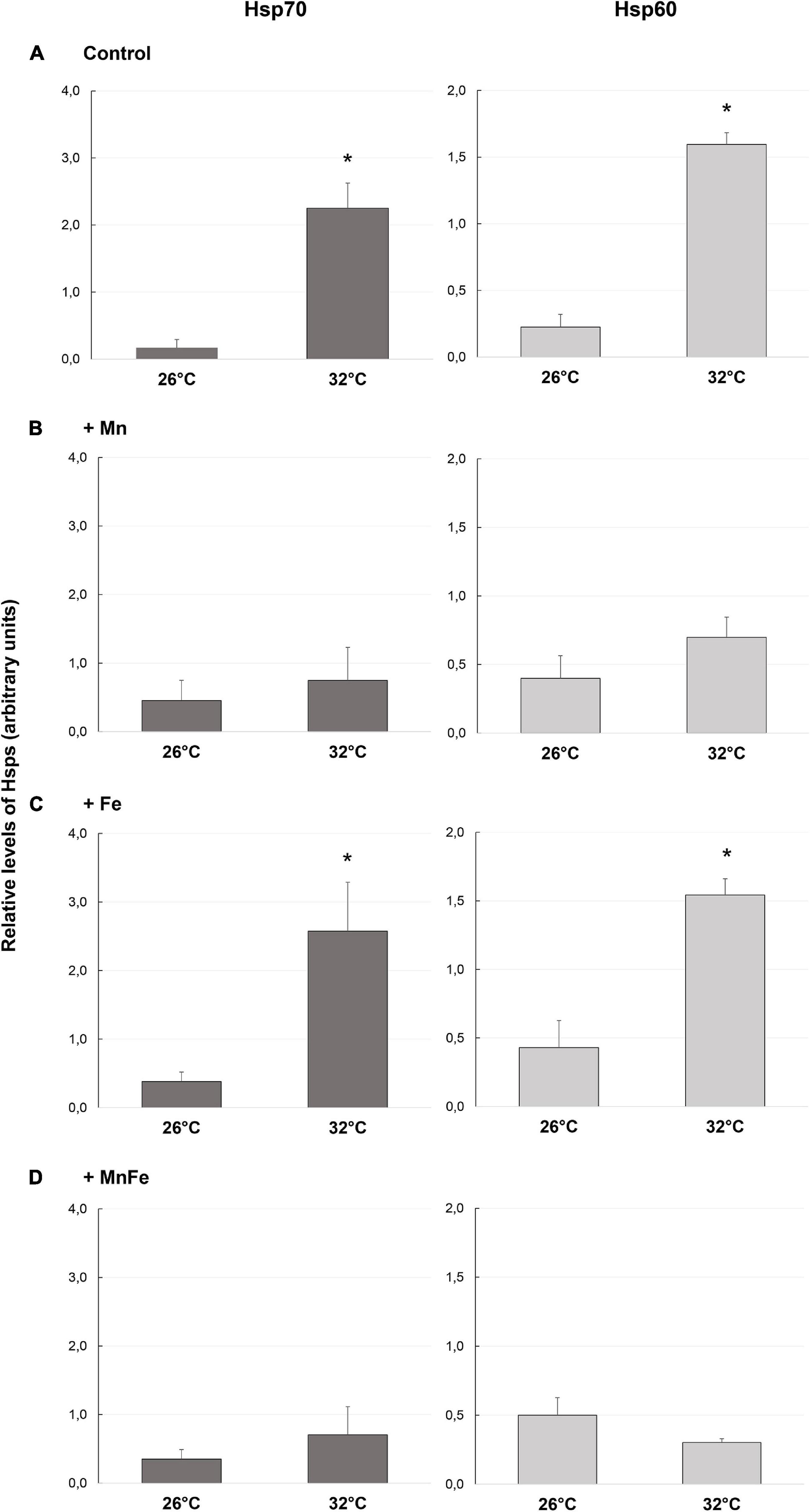
Figure 1. Levels of Hsp70 and Hsp60 detected in samples of S. pistillata exposed to the different metal treatments (A: Control; B: +Mn; C: +Fe; D: +MnFe) after 6 weeks at 26°C or 3 weeks at 32°C. The values were determined by densitometric analysis as described under section “Materials and Methods.” In the same section, the values related to the metal concentrations for each treatment are also shown. Data are expressed as arbitrary units and as mean ± SEM (n = 4, for both Hsp70 and Hsp60). For each biomarker, asterisks indicate significant differences between corals maintained at 26 and 32°C under different treatments and, within each treatment, between corals maintained at 26 and 32°C.
As expected, in the Control samples, starting from a low basal constitutive level, a significant up-regulation of the expression of both Hsp70 and Hsp60 was observed when the water temperature shifted from 26 to 32°C (Figure 1A and Table 1). On the contrary, no significant effect of metal addition was observed on Hsp expression at the ambient temperature of 26°C [one-way ANOVA, F(3,12) = 0.39, p > 0.05 for Hsp70; F(3,12) = 0.59, p > 0.05 for Hsp60; Figure 1].
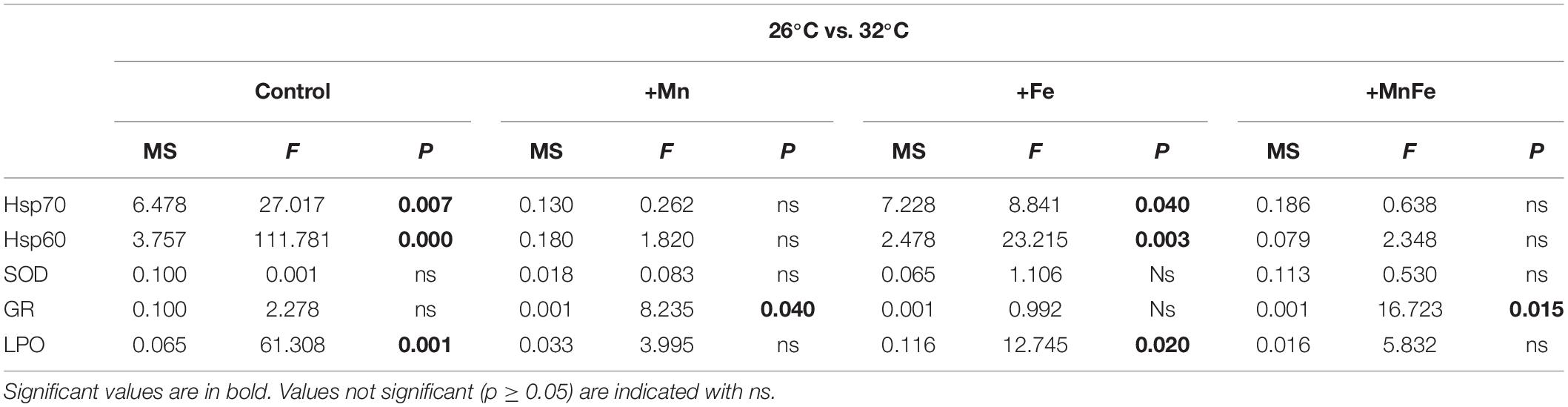
Table 1. Summary of the one-way analysis of variance (ANOVA) for values of Hsp70, Hsp60, SOD, GR, and LPO in colonies maintained at 26°C vs. those subjected to thermal stress (32°C) at each metal treatment.
At 32°C, the Hsp70 and Hsp60 levels detected in Mn and MnFe enriched samples were similar to those of corals maintained at 26°C and therefore no significant modulation of Hsps following heat stress was observed (Figures 1B,D and Table 1). In contrast, the combination of Fe enrichment and heat stress led to an expression of Hsp70 and Hsp60 similar to that observed in the Control tanks at 32°C (Figure 1C and Table 1). Consequently, significant differences in Hsp expression were observed among all conditions at 32°C [one-way ANOVA, F(3,12) = 13.56, p = 0.04 for Hsp70; F(3,12) = 36.56, p = 0.001 for Hsp60; Figure 1].
Antioxidant Activity
The antioxidant activity of two enzymes involved in ROS detoxification, namely SOD and GR, was assessed in the coral samples subjected to the different stressors.
No significant changes were observed in SOD antioxidant activity among different treatments both at 26°C or following the temperature shift at 32°C (p > 0.05; Figure 2).
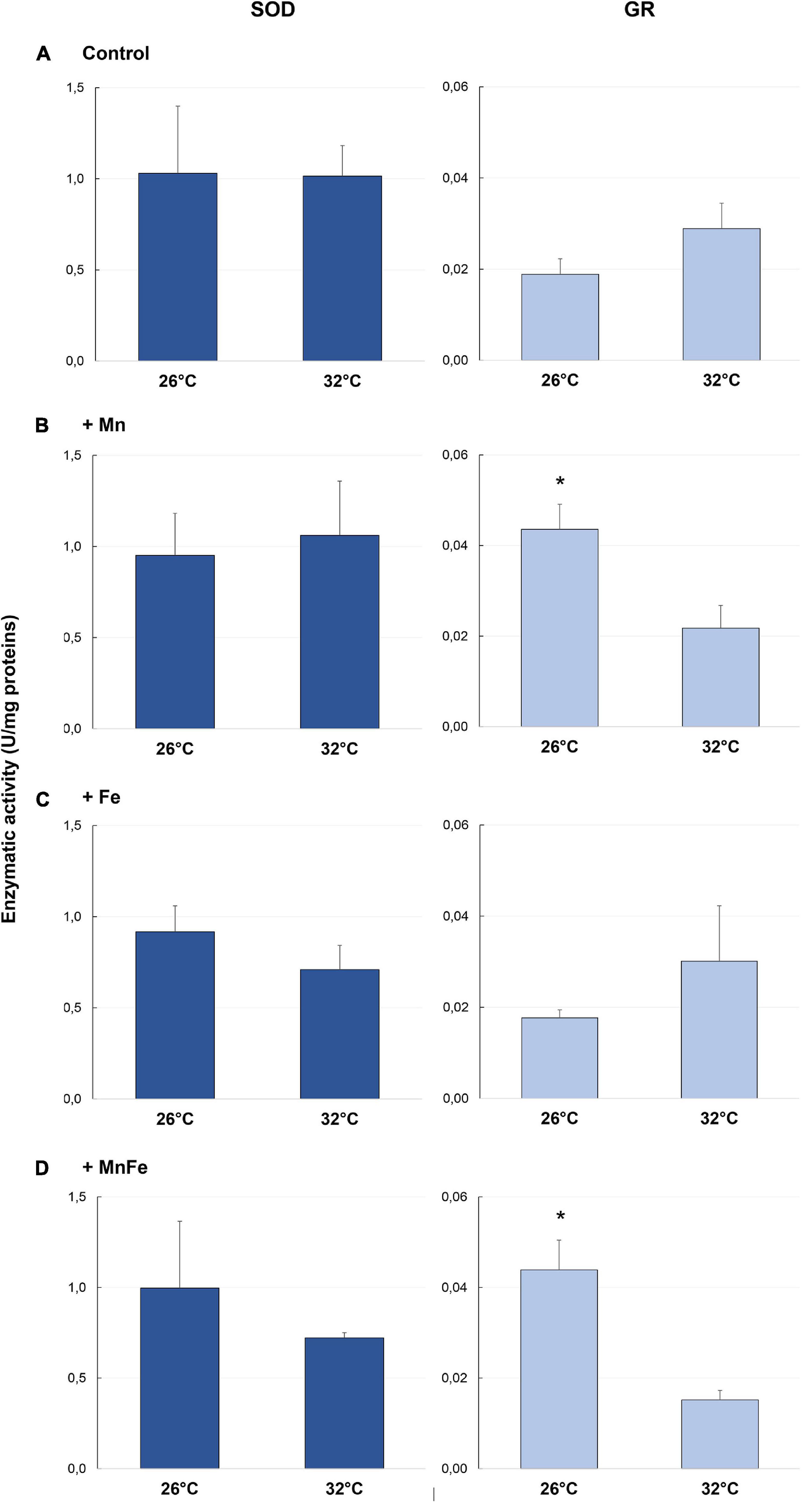
Figure 2. Enzymatic activity of SOD and GR detected in samples of S. pistillata exposed to the different metal treatments (A: Control; B: +Mn; C: +Fe; D: +MnFe) after 6 weeks at 26°C or 3 weeks at 32°C. The values related to the metal concentrations for each treatment are reported in section “Materials and Methods.” Data are expressed as units (U) of enzyme per mg of proteins and as mean ± SEM (n = 4, for both SOD and GR). For each biomarker, asterisks indicate significant differences between corals maintained at 26 and 32°C under different treatments and, within each treatment, between corals maintained at 26 and 32°C.
As far as GR activity is concerned, significant differences were detected at 26°C [one-way ANOVA, F(3,12) = 9.45, p = 0.005]. In particular, a higher activity was recorded in +Mn and +MnFe treatments, compared to Control and +Fe samples (Figure 2). Moreover, at 32°C, GR activity significantly decreased in the treatment +Mn and +MnFe (Figures 2B,D and Table 1), while it did not change significantly in corals under Control conditions and in those subjected to Fe enrichment (Figures 2A,C and Table 1). As a result of these modulations, no significant differences were detected among all conditions at 32°C [one-way ANOVA, F(3,12) = 0.89, p > 0.05; Figure 2].
Lipid Peroxidation
Oxidative damage on S. pistillata was evaluated by analyzing the LPO levels through MDA production. Significant variations of the MDA levels were observed in corals maintained at 26°C and subjected to different metal enrichments [one-way ANOVA, F(3,12) = 17.81, p = 0.001]. Specifically, the enrichment of Mn and MnFe caused a significant increase of the MDA levels compared to Control and +Fe treatments (Figure 3).
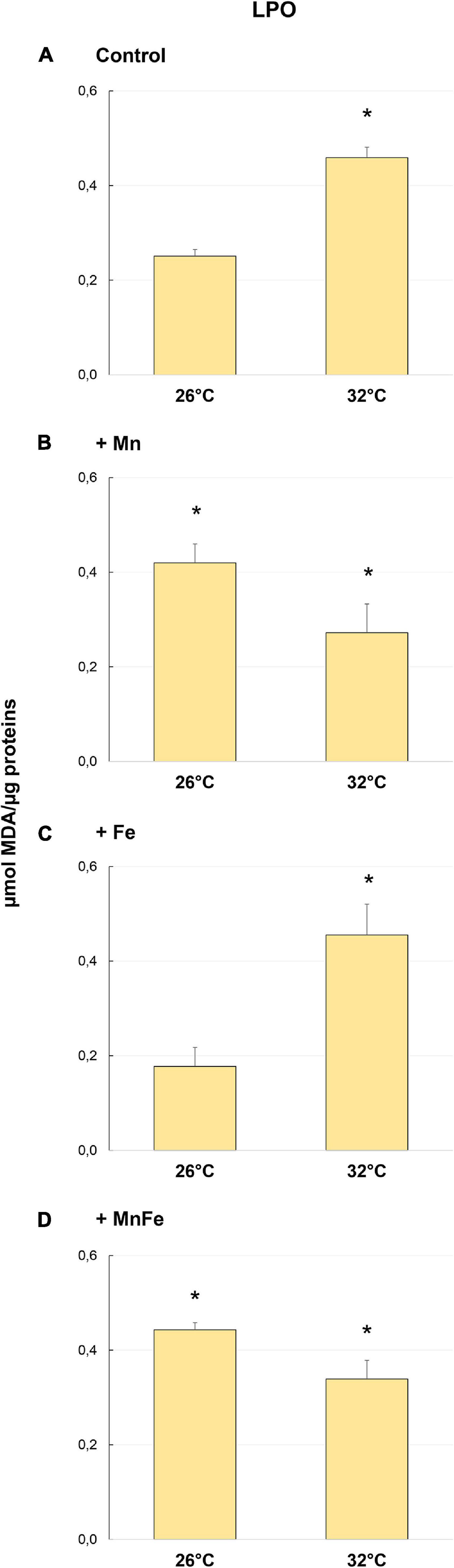
Figure 3. Levels of lipid peroxidation (LPO) detected in samples of S. pistillata exposed to the different metal treatments (A: Control; B: +Mn; C: +Fe; D: +MnFe) after 6 weeks at 26°C or 3 weeks at 32°C. The values related to the metal concentrations for each treatment are reported in section “Materials and Methods.” Data are expressed as μmol of MDA per μg of proteins and as mean ± SEM (n = 4). Asterisks indicate significant differences between corals maintained at 26 and 32°C under different treatments and, within each treatment, between corals maintained at 26 and 32°C.
However, heat stress produced a significant increase in MDA levels only in Control and in +Fe samples (Figures 3A,C and Table 1), while in coral subjected to Mn and MnFe enrichments a slight and not significant decrease of the MDA level was detected (Figures 3B,D). As a result, no significant differences were detected among all conditions at 32°C [one-way ANOVA, F(3,12) = 3.26, p > 0.05; Figure 2].
Multivariate Analysis
In order to investigate together the modulation of Hsp expression, enzymatic activity and LPO in response to metal and thermal stresses, multivariate analyses were performed. The PERMANOVA revealed significant differences in biomarker levels among temperatures and treatments and the combination of both (Table 2). In particular, the biomarker levels in Mn and MnFe enriched corals appeared significantly different from that recorded in Control and +Fe treatments, especially when corals were subjected to temperature increase at 32°C (Table 3). In line with this, the nMDS analysis showed that Mn and MnFe enriched corals maintained at both ambient and increased temperatures, displayed a cellular response similar to corals kept in Control condition at 26°C, but different from that of Control and Fe enriched corals subjected to thermal stress (Figure 4).

Table 2. Results of the PERMANOVA testing the effects of the temperature (26 and 32°C) and treatments (Control, +Mn, +Fe, and +MnFe) on the levels of Hsp70, Hsp60, SOD, GR, and LPO obtained by permutations (perm) for each group.
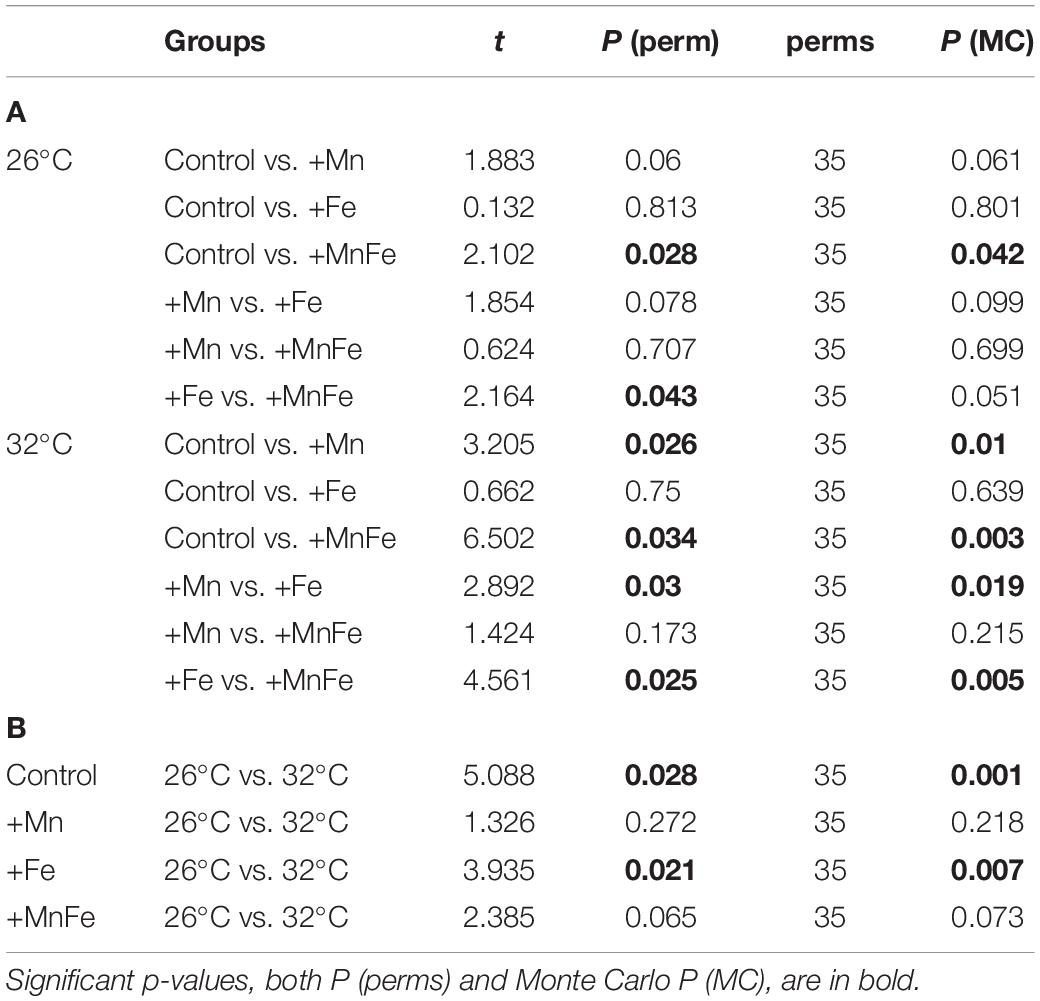
Table 3. Results of the PERMANOVA pairwise comparisons between treatments at 26°C and 32°C (A), and between temperatures within each metal treatment (B) obtained by permutations (perm) for each group.
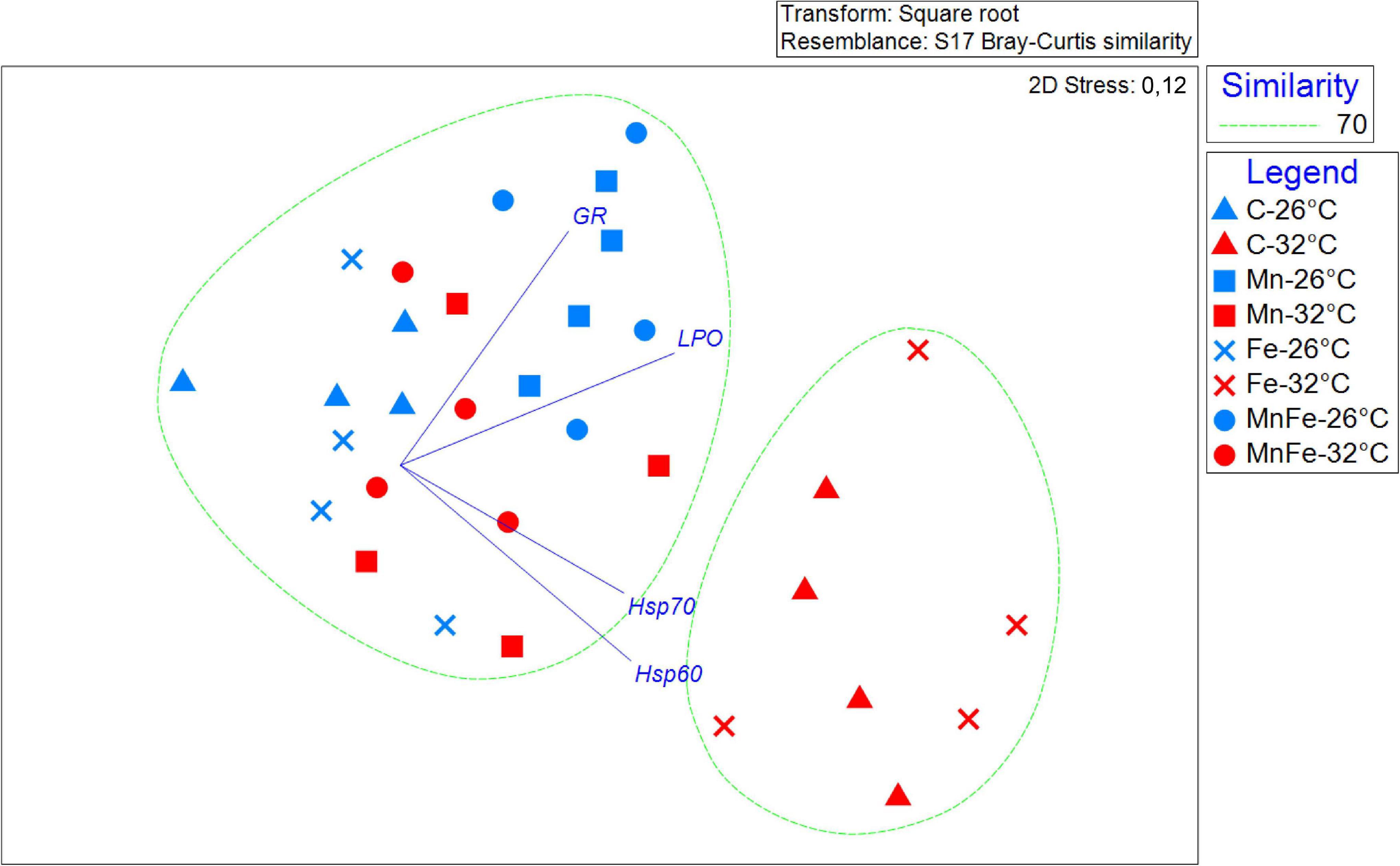
Figure 4. Non-metric multidimensional scaling plot (nMDS) showing the response pattern of all the S. pistillata biomarkers analyzed by metal treatment and different temperatures, with vectors (Pearson’s correlations ≥ 0.8) representing the variables (biomarkers) that drive significant similarities among experimental conditions.
Discussion
The tolerance of corals to heat stress-induced bleaching can be influenced by metal concentrations in seawater. In this context, manganese and iron enrichments have previously been shown to exhibit contrasting effects on S. pistillata physiological parameters, such as cellular chlorophyll concentrations, photosynthetic efficiency, gross photosynthetic rates, and calcification (Biscéré et al., 2018). Going on with the characterization of the same coral samples, the results here obtained contribute to clarify the impact of these metals on the coral cellular homeostasis and their possible role in thermal stress-induced bleaching, suggesting that both temperature, metal enrichment and their interaction significantly affect the coral cellular response.
Hsp70 and Hsp60 showed similar patterns of expression in response to metal enrichment, temperature stress, and the combination of both stressors. Hsp70, as cytosolic chaperonin, is engaged in assembly of newly synthesized proteins and in the refolding of misfolded or aggregated proteins, contributing to the transfer of proteins to different cellular compartments or to the proteolytic machinery (Balchin et al., 2016). Hsp60 is a mitochondrial chaperonin that plays a crucial role in the synthesis and transportation of mitochondrial proteins and in the folding of newly imported or stress-denatured proteins (Arya et al., 2007). A parallel pattern of modulation of Hsp70 and Hsp60 in all treatments confirms that, despite their different cellular localization, these chaperones work in tandem and plays a synergic role in assisting the folding process, under both physiological and stressful conditions (Zhou et al., 1996; Feder and Hofmann, 1999; Papp et al., 2003; Chen et al., 2018; Rosenzweig et al., 2019). Moreover, both the Hsps displayed a low basal constitutive level in corals maintained at 26°C, as commonly observed in healthy corals (Fitt et al., 2009; Chow et al., 2012; Ross, 2014; Seveso et al., 2016, 2017, 2020).
We observed significant changes in the cellular response of S. pistillata to thermal stress. Elevated temperatures indeed resulted in a significant up-regulation of Hsp70 and Hsp60, as previously reported (Downs et al., 2002; Baird et al., 2009; Seveso et al., 2018, 2020; Louis et al., 2020), as well as in the LPO levels. LPO reflects the structural integrity of the cell membranes and production of LPOs indicates that levels of ROS are overwhelming the antioxidant pathways, accumulating and damaging cellular membrane lipids, thus signaling an ongoing oxidative stress (Lesser, 2006; Weis, 2008). An increase of LPO in response to heat stress-inducing bleaching has been widely observed in corals (Downs et al., 2000; Flores-Ramírez and Liñán-Cabello, 2006; Dias et al., 2019a,b; Marangoni et al., 2019a). The increase of both Hsps and LPO in control samples following heat stress coincided with a significant decrease of symbiont density, chlorophyll concentration, and photosynthetic rate, measured previously on the same coral samples (Biscéré et al., 2018).
Iron enrichment did not induce any change in the cellular protein homeostasis and in the oxidative status of S. pistillata at the normal growth temperature of 26°C. This has to be linked to the lack of Fe effect on S. pistillata photophysiological parameters previously analyzed (Biscéré et al., 2018). However, when Fe was coupled to thermal stress, a strong rise in LPO and Hsp levels was observed, in conjunction with coral bleaching and reduced calcification reported by Biscéré et al. (2018). This indicates that Fe enrichment did not provide significant beneficial effects to the coral, although Fe is present in many molecules involved in photosynthesis such as chlorophyll, photosystem I and II, cytochrome b6f complex, and ferredoxin (Geider and La Roche, 1994; Shcolnick and Keren, 2006). This is surprising, as it has previously been reported that Fe limitation increased coral bleaching susceptibility (Shick et al., 2011), while a slight enrichment enhanced photosynthesis, but significantly decreased calcification (Ferrier-Pagès et al., 2001). Overall, these results suggest that Fe is essential to corals, as a complete lack of it impairs coral physiology, but it should remain in trace amounts to avoid becoming toxic. In addition, the high Fe level used in this study did not mitigate the thermal stress.
As far as Mn is concerned, it is a key element in photosynthetic organisms. It is directly involved in chlorophyll synthesis and is an essential component of the Oxygen Evolving Complex of photosystem II, one of the photosynthetic molecules of coral symbionts most affected by heat stress and responsible for ROS production (Hill and Ralph, 2008; Millaleo et al., 2010). In the same samples analyzed previously (Biscéré et al., 2018), Mn enrichment allowed corals to maintain high symbiont density and chlorophyll concentrations, maximal rates of photosynthesis, and to enhance host metabolism and calcification both at control temperature and during thermal stress, avoiding bleaching. While in this study there was no effect of Mn or MnFe on Hsps of S. pistillata at 26°C, we indeed observed beneficial effects of Mn or MnFe on the oxidative stress response of S. pistillata to thermal stress, as Mn is an essential metal involved in cell catalytic functions and antioxidant mechanisms (Papanikolaou and Pantopoulos, 2005; Santamaria, 2008). Mn and MnFe enrichments prevented any increase in Hsp levels following thermal stress, suggesting the absence of protein damage and strengthening the hypothesis that Mn could mitigate the negative cellular effects produced by the thermal stress (Biscéré et al., 2018). In addition to this, our data suggest that Mn could also be able to maintain the normal cellular protein homeostasis in the host facing heat shock, probably due to its key role in cellular adaptation to oxidative stress and ROS detoxification (Horsburgh et al., 2002; Aguirre and Culotta, 2012). There was also no change in LPO levels of Mn and MnFe-enriched corals between control and heat stress conditions. However, the lack of post-thermal change in LPO may be due to the pre-existing high LPO level detected in Mn-enriched corals kept at normal temperature, in which the Mn increase alone likely caused oxidative stress, as also indicated by the antioxidant activity of the GR. Indeed, our results showed that at 26°C the GR activity increased as well in corals under Mn enrichments (+Mn and +MnFe). In previous studies, an increased activity of the enzyme, together with LPO level, has been observed in larvae of Pocillopora damicornis after 7-days exposure to iron chloride [100 ppm] (Vijayavel et al., 2012), and after 96-h exposure to Cu (Bielmyer-Fraser et al., 2018). The enzyme GR is involved in maintaining and restoring the balance between oxidized and reduced forms of glutathione, a tripeptide able to react with different ROS forming a disulphide bond with another oxidized glutathione. This antioxidant action in coral bleaching response is well known (Lesser, 2006; Krueger et al., 2015). For this reason, it appears surprising that GR activity did not change with the temperature increase, although this trend has been already observed in corals (Liñán-Cabello et al., 2010; Nielsen et al., 2018). The same pattern was detected for corals exposed to Fe enrichment, while a significant decrease in the GR activity was observed after thermal stress in corals exposed to Mn enrichments.
The contrasting effect of Mn enrichments on the oxidative status of the holobiont and on the protein homeostasis of the host, revealed by LPO/GR and Hsps, respectively, needs to be explored. We speculate that Mn may initially be toxic to zooxanthellae, and the resulting oxidative damage could affect the polyp. Therefore, the high level of LPO and GR may be mostly associated with zooxanthellae, considering that Mn enhanced the coral’s photosynthetic rate even at ambient temperature as measured in the same coral samples (Biscéré et al., 2018) and this increase may result in an overproduction of ROS in zooxanthellae (Roth, 2014; Foyer, 2018). In this context, high levels of GR in Mn enrichment conditions could detoxify the generated ROS, protecting the host proteins. This could possibly explain the low levels of Hsps measured here and the similar levels of symbiont density and bleaching status observed by Biscéré et al. (2018) in +Mn and Control conditions. In addition, the increased symbiont photosynthetic activity promoted by Mn could support the coral host for longer during heat stress, in line with recent findings indicating that altered symbiotic nutrient cycling may be the direct cause for symbiont expulsion (Rädecker et al., 2021).
Unexpectedly, the results of the SOD did not follow the same trend as those of the GR and LPO, as SOD activity did not show any significant variation at different temperatures and in the various treatments. SOD propels the disproportion of O2– ion into H2O2 and molecular oxygen (O2) and can be found mainly in three forms, Cu/Zn SOD, MnSOD, and FeSOD, each one with a specific cellular location (Verma et al., 2019). Although our corals were treated with +Mn and +Fe, two fundamental cofactors for the enzyme functioning, SOD activity was not influenced by enhanced availability of these metals. In addition, the rise in temperature did not affect its activity, in line with previous reports (Yakovleva et al., 2004; Liñán-Cabello et al., 2010; Krueger et al., 2015).
Overall, our results, together with those obtained previously (Biscéré et al., 2018), provide a clear indication of how Mn enrichment causes a cellular and physiological response in S. pistillata samples significantly different from the one recorded in Control and +Fe treatments. At the concentrations and exposure times considered here, Mn could increase the levels of oxidative stress by enhancing photosynthetic rates at 26°C (Biscéré et al., 2018), although this did not affect the cellular homeostasis of corals. In addition, Mn seems able to mitigate the negative cellular effects produced by thermal stress, increasing the coral’s resistance to heat stress-induced bleaching, as previously hypothesized (Biscéré et al., 2018). On the contrary, the Fe enrichment does not appear to play any role, since it showed neither a mitigating effect of thermal stress nor a damaging effect at 26°C (Biscéré et al., 2018). Therefore, it appears evident that the underlying molecular mechanisms and pathways of host and symbiont response to metal and thermal stresses are more complex than expected. In this context, it becomes essential to better clarify the role of Mn, and in general that of metals, in preventing catastrophic outputs for coral reefs, such as coral bleaching.
Data Availability Statement
The raw data supporting the conclusions of this article will be made available by the authors, without undue reservation.
Author Contributions
EM and DS wrote the manuscript and analyzed the results. DS, TB, CF-P, and FH conceived and designed the research and the experiments. MV and CF-P provided significant contributions and revisions. PG secured funding for this research and for all reagents and materials. TB performed aquaria experiments. EM, IO, and MF performed and supervised the laboratory activities. All authors contributed to the article and approved the submitted version.
Funding
TB was beneficiary of a Ph.D. grant (CIFRE No. 2015/0301, France) supported by the Koniambo Nickel SAS and Ginger Soproner companies in New Caledonia. These funders were not involved in the study design, collection, analysis, interpretation of data, the writing of this article or the decision to submit it for publication. Part of this work was also funded by the CNRT Nickel (DYNAMINE project) to TB and FH.
Conflict of Interest
The authors declare that the research was conducted in the absence of any commercial or financial relationships that could be construed as a potential conflict of interest.
Acknowledgments
The authors would like to thank Simone Montano and Roberto Giacchini for the logistic support and technical assistance. The authors would also like to thank Elena Cotugno and Hayden Weiler for English revision.
Supplementary Material
The Supplementary Material for this article can be found online at: https://www.frontiersin.org/articles/10.3389/fmars.2021.681119/full#supplementary-material
Footnotes
References
Aguirre, J. D., and Culotta, V. C. (2012). Battles with iron: manganese in oxidative stress protection. J. Biol. Chem. 287, 13541–13548. doi: 10.1074/jbc.R111.312181
Anderson, M., Gorley, R., and Clarke, K. (2008). PERMANOVA+ for PRIMER: Guide to Software and Statistical Methods. Plymouth: PRIMER-E.
Anderson, M. J., and Robinson, J. (2003). Generalized discriminant analysis based on distances. Aust. N. Z. J. Stat. 45, 301–318. doi: 10.1111/1467-842X.00285
Arya, R., Mallik, M., and Lakhotia, S. C. (2007). Heat shock genes - integrating cell survival and death. J. Biosci. 32, 595–610. doi: 10.1007/s12038-007-0059-3
Baird, A. H., Bhagooli, R., Ralph, P. J., and Takahashi, S. (2009). Coral bleaching: the role of the host. Trends Ecol. Evol. 24, 16–20. doi: 10.1016/j.tree.2008.09.005
Balchin, D., Hayer-Hartl, M., and Hartl, F. U. (2016). In vivo aspects of protein folding and quality control. Science 353:aac4354.
Banc-Prandi, G., and Fine, M. (2019). Copper enrichment reduces thermal tolerance of the highly resistant Red Sea coral Stylophora pistillata. Coral Reefs 38, 285–296. doi: 10.1007/s00338-019-01774-z
Bielmyer-Fraser, G. K., Grosell, M., Bhagooli, R., Baker, A. C., Langdon, C., Gillette, P., et al. (2010). Differential effects of copper on three species of scleractinian corals and their algal symbionts (Symbiodinium spp.). Aquat. Toxicol. 97, 125–133. doi: 10.1016/j.aquatox.2009.12.021
Bielmyer-Fraser, G. K., Patel, P., Capo, T., and Grosell, M. (2018). Physiological responses of corals to ocean acidification and copper exposure. Mar. Pollut. Bull. 133, 781–790.
Bierkens, J. (2000). Applications and pitfalls of stress-proteins in biomonitoring. Toxicology 153, 61–72.
Biscéré, T., Ferrier-Pagès, C., Gilbert, A., Pichler, T., and Houlbrèque, F. (2018). Evidence for mitigation of coral bleaching by manganese. Sci. Rep. 8:16789. doi: 10.1038/s41598-018-34994-4
Biscéré, T., Lorrain, A., Rodolfo-Metalpa, R., Gilbert, A., Wright, A., Devissi, C., et al. (2017). Nickel and ocean warming affect scleractinian coral growth. Mar. Pollut. Bull. 120, 250–258. doi: 10.1016/j.marpolbul.2017.05.025
Biscéré, T., Rodolfo-Metalpa, R., Lorrain, A., Chauvaud, L., Thébault, J., Clavier, J., et al. (2015). Responses of two scleractinian corals to cobalt pollution and ocean acidification. PLoS One 10:e0122898. doi: 10.1371/journal.pone.0122898
Chen, B., Feder, M. E., and Kang, L. (2018). Evolution of heat-shock protein expression underlying adaptive responses to environmental stress. Mol. Ecol. 27, 3040–3054.
Chow, A. M., Beraud, E., Tang, D. W., Ferrier-Pagès, C., and Brown, I. R. (2012). Hsp60 protein pattern in coral is altered by environmental changes in light and temperature. Comp. Biochem. Physiol. A Mol. Integr. Physiol. 161, 349–353. doi: 10.1016/j.cbpa.2011.12.004
Cleves, P. A., Krediet, C. J., Lehnert, E. M., Onishi, M., and Pringle, J. R. (2020). Insights into coral bleaching under heat stress from analysis of gene expression in a sea anemone model system. Proc. Natl. Acad. Sci. U.S.A. 117, 28906–28917. doi: 10.1073/pnas.2015737117
Cziesielski, M. J., Schmidt-Roach, S., and Aranda, M. (2019). The past, present, and future of coral heat stress studies. Ecol. Evol. 9, 10055–10066. doi: 10.1002/ece3.5576
DalCorso, G., Manara, A., Piasentin, S., and Furini, A. (2014). Nutrient metal elements in plants. Metallomics 6, 1770–1788. doi: 10.1039/c4mt00173g
Davy, S. K., Allemand, D., and Weis, V. M. (2012). Cell Biology of Cnidarian-Dinoflagellate Symbiosis. Microbiol. Mol. Biol. Rev. 76, 229–261. doi: 10.1128/MMBR.05014-11
Deponte, M. (2013). Glutathione catalysis and the reaction mechanisms of glutathione-dependent enzymes. Biochim. Biophys. Acta 830, 3217–3266. doi: 10.1016/j.bbagen.2012.09.018
DeSalvo, M. K., Voolstra, C. R., Sunagawa, S., Schwarz, J. A., Stillman, J. H., Coffroth, M. A., et al. (2008). Differential gene expression during thermal stress and bleaching in the Caribbean coral Montastraea faveolata. Mol. Ecol. 17, 3952–3971. doi: 10.1111/j.1365-294X.2008.03879.x
Dias, M., Ferreira, A., Gouveia, R., Madeira, C., Jogee, N., Cabral, H., et al. (2019a). Long-term exposure to increasing temperatures on scleractinian coral fragments reveals oxidative stress. Mar. Environ. Res. 150, 104758. doi: 10.1016/j.marenvres.2019.104758
Dias, M., Ferreira, A., Gouveia, R., and Vinagre, C. (2019b). Synergistic effects of warming and lower salinity on the asexual reproduction of reef-forming corals. Ecol. Indic. 98, 334–348. doi: 10.1016/j.ecolind.2018.11.011
Dias, M., Madeira, C., Jogee, N., Ferreira, A., Gouveia, R., Cabral, H., et al. (2020). Integrative indices for health assessment in reef corals under thermal stress. Ecol. Indic. 113:106230. doi: 10.1016/j.ecolind.2020.106230
Downs, C., Mueller, E., Phillips, S., Fauth, J. E., and Woodley, C. M. (2000). A molecular biomarker system for assessing the health of coral (Montastraea faveolata) during heat stress. Mar. Biotechnol. 2, 533–544. doi: 10.1007/s101260000038
Downs, C. A. (2005). “Cellular diagnostics and its application to aquatic and marine toxicology,” in Techniques in Aquatic Toxicology, Vol. 2, ed. G. K. Ostrander (Boca Raton, FL: CRC Press), 181–208.
Downs, C. A., Fauth, J. E., Halas, J. C., Dustan, P., Bemiss, J., and Woodley, C. M. (2002). Oxidative stress and seasonal coral bleaching. Free Radic. Biol. Med. 33, 533–543. doi: 10.1016/S0891-5849(02)00907-3
Downs, C. A., Fauth, J. E., Robinson, C. E., Curry, R., Lanzendorf, B., Halas, J. C., et al. (2005). Cellular diagnostics and coral health: declining coral health in the Florida Keys. Mar. Pollut. Bull. 51, 558–569.
Eakin, C. M., Sweatman, H. P. A., and Brainard, R. E. (2019). The 2014–2017 global-scale coral bleaching event: insights and impacts. Coral Reefs 38, 539–545. doi: 10.1007/s00338-019-01844-2
Fairbrother, A., Wenstel, R., Sappington, K., and Wood, W. (2017). Framework for metals risk assessment. Ecotoxicol. Environ. Saf. 68, 145–227. doi: 10.1016/j.ecoenv.2007.03.015
Feder, M. E., and Hofmann, G. E. (1999). Heat-shock proteins, molecular chaperones, and the stress response: evolutionary and ecological physiology. Annu. Rev. Physiol. 61, 243–282.
Ferrier-Pagès, C., Sauzéat, L., and Balter, V. (2018). Coral bleaching is linked to the capacity of the animal host to supply essential metals to the symbionts. Glob. Change Biol. 24, 3145–3157. doi: 10.1111/gcb.14141
Ferrier-Pagès, C., Schoelzke, V., Jaubert, J., Muscatine, L., and Hoegh-Guldberg, O. (2001). Response of a scleractinian coral, Stylophora pistillata, to iron and nitrate enrichment. J. Exp. Mar. Biol. Ecol. 259, 249–261. doi: 10.1016/s0022-0981(01)00241-6
Fitt, W. K., Gates, R. D., Hoegh-Guldberg, O., Bythell, J. C., Jatkar, A., Grottoli, A. G., et al. (2009). Response of two species of Indo-Pacific corals, Porites cylindrica and Stylophora pistillata, to short-term thermal stress: the host does matter in determining the tolerance of corals to bleaching. J. Exp. Mar. Biol. Ecol. 373, 102–110.
Flores-Ramírez, L. A., and Liñán-Cabello, M. A. (2006). Relationships among thermal stress, bleaching and oxidative damage in the hermatypic coral, Pocillopora capitata. Comp. Biochem. Physiol. C Toxicol. Pharmacol. 146, 194–202. doi: 10.1016/j.cbpc.2006.09.008
Fonseca, J. S., Marangoni, L. F. B., Marques, J. A., and Bianchini, A. (2017). Effects of increasing temperature alone and combined with copper exposure on biochemical and physiological parameters in the zooxanthellate scleractinian coral, Mussismilia harttii. Aquat. Toxicol. 190, 121–132.
Foyer, C. H. (2018). Reactive oxygen species, oxidative signaling and the regulation of photosynthesis. Environ. Exp. Bot. 154, 134–142. doi: 10.1016/j.envexpbot.2018.05.003
Gaschler, M. M., and Stockwell, B. R. (2017). Lipid peroxidation in cell death. Biochem. Biophys. Res. Commun. 482, 419–425. doi: 10.1016/j.bbrc.2016.10.086
Geider, R. J., and La Roche, J. (1994). The role of iron in phytoplankton photosynthesis, and the potential for iron-limitation of primary productivity in the sea. Photosynth. Res. 39, 275–301. doi: 10.1007/BF00014588
Gérard-Monnier, D., Erdelmeier, I., Régnard, K., Moze-Henry, N., Yadan, J. C., and Chaudiere, J. (1998). Reactions of 1-methyl-2-phenylindole with malondialdehyde and 4-hydroxyalkenals. Analytical applications to a colorimetric assay of lipid peroxidation. Chem. Res. Toxicol. 11, 1176–1183.
Gissi, F., Stauber, J., Reichelt-Brushett, A., Harrison, P. L., and Jolley, D. F. (2017). Inhibition in fertilisation of coral gametes following exposure to nickel and copper. Ecotoxicol. Environ. Saf. 145, 32–41. doi: 10.1016/j.ecoenv.2017.07.009
Goh, B. P. (1991). Mortality and settlement success of Pocillopora damicornis planula larvae during recovery from low levels of nickel. Pac. Sci. 45, 276–286.
Hill, R., and Ralph, J. R. (2008). Dark-induced reduction of the plastoquinone pool in zooxanthellae of scleractinian corals and implications for measurements of chlorophyll a fluorescence. Symbiosis 46, 45–56.
Hoegh-Guldberg, O. (1999). Climate change, coral bleaching and the future of the world’s coral reefs. Mar. Freshw. Res. 50, 839–866.
Horsburgh, M. J., Wharton, S. J., Karavolos, M., and Foster, S. J. (2002). Manganese: elemental defence for a life with oxygen. Trends Microbiol. 10, 496–501. doi: 10.1016/s0966-842x(02)02462-9
Hughes, T. P., Kerry, J., Álvarez-Noriega, M., Álvarez-Romero, J. G., Anderson, K. D., Baird, A. H., et al. (2017). Global warming and recurrent mass bleaching of corals. Nature 543, 373–377. doi: 10.1038/nature21707
Kenkel, C. D., Sheridan, C., Leal, M. C., Bhagooli, R., Castillo, K. D., Kurata, N., et al. (2014). Diagnostic gene expression biomarkers of coral thermal stress. Mol. Ecol. Resour. 14, 667–678. doi: 10.1111/1755-0998.12218
Krueger, T., Hawkins, T. D., Becker, S., Pontasch, S., Dove, S., Hoegh-Guldberg, O., et al. (2015). Differential coral bleaching contrasting the activity and response of enzymatic antioxidants in symbiotic partners under thermal stress. Comp. Biochem. Physiol. A Mol. Integr. Physiol. 190, 15–25. doi: 10.1016/j.cbpa.2015.08.012
Kültz, D. (2005). Molecular and evolutionary basis of the cellular stress response. Annu. Rev. Physiol. 67, 225–257. doi: 10.1146/annurev.physiol.67.040403.103635
Kwok, C. K., and Ang, P. O. Jr. (2013). Inhibition of larval swimming activity of the coral (Platygyra acuta) by interactive thermal and chemical stresses. Mar. Pollut. Bull. 74, 264–273. doi: 10.1016/j.marpolbul.2013.06.048
Lesser, M. P. (2006). Oxidative stress in marine environments: biochemistry and physiological ecology. Annu. Rev. Physiol. 68, 253–278. doi: 10.1146/annurev.physiol.68.040104.110001
Lesser, M. P. (2011). “Coral bleaching: causes and mechanisms,” in Coral Reefs: An Ecosystem in Transition, eds Z. Dubinsky and N. Stambler (Dordrecht: Springer). doi: 10.1007/978-94-007-0114-4_23
Liñán-Cabello, M. A., Flores-Ramírez, L. A., Zenteno-Savin, T., Olguín-Monroy, N. O., Sosa-Avalos, R., Patiño-Barragan, M., et al. (2010). Seasonal changes of antioxidant and oxidative parameters in the coral Pocillopora capitata on the Pacific coast of Mexico. Mar. Ecol. 31, 407–417. doi: 10.1111/j.1439-0485.2009.00349.x
Lough, J. M., Anderson, K. D., and Hughes, T. P. (2018). Increasing thermal stress for tropical coral reefs: 1871–2017. Sci. Rep. 8:6079. doi: 10.1038/s41598-018-24530-9
Louis, Y. D., Bhagooli, R., Kenkel, C. D., Baker, A. C., and Dyall, S. D. (2017). Gene expression biomarkers of heat stress in scleractinian corals: promises and limitations. Comp. Biochem. Physiol. C Toxicol. Pharmacol. 191, 63–77. doi: 10.1016/j.cbpc.2016.08.007
Louis, Y. D., Bhagooli, R., Seveso, D., Maggioni, D., Galli, P., Vai, M., et al. (2020). Local acclimatisation-driven differential gene and protein expression patterns of Hsp70 in Acropora muricata: implications for coral tolerance to bleaching. Mol. Ecol. 29, 4382–4394. doi: 10.1111/mec.15642
Marangoni, L. F. B., Dalmolin, C., Marques, J. A., Klein, R. D., Abrantes, D. P., Pereira, C. M., et al. (2019a). Oxidative stress biomarkers as potential tools in reef degradation monitoring: a study case in a South Atlantic reef under influence of the 2015–2016 El Niño/Southern Oscillation (ENSO). Ecol. Indic. 106:105533. doi: 10.1016/j.ecolind.2019.105533
Marangoni, L. F. B., Marques, J. A., Duarte, G. A. S., Pereira, C. M., Calderon, E. N., Barreira, C., et al. (2017). Copper effects on biomarkers associated with photosynthesis, oxidative status and calcification in the Brazilian coral Mussismilia harttii (Scleractinia, Mussidae). Mar. Environ. Res. 130, 248–257. doi: 10.1016/j.marenvres.2017.08.002
Marangoni, L. F. B., Mies, M., Güth, A. Z., Banha, T. N. S., Inague, A., Fonseca, J. D. S., et al. (2019b). Peroxynitrite generation and increased heterotrophic capacity are linked to the disruption of the coral–dinoflagellate symbiosis in a scleractinian and hydrocoral species. Microorganisms 7:426.
Marangoni, L. F. B., Pinto, M. M. A. N., Marques, J. A., and Bianchini, A. (2019c). Copper exposure and seawater acidification interaction: antagonistic effects on biomarkers in the zooxanthellate scleractinian coral Mussismilia harttii. Aquat. Toxicol. 206, 123–133. doi: 10.1016/j.aquatox.2018.11.005
Mayfield, A. B., Wang, L. H., Tang, P. C., Fan, T. Y., Hsiao, Y. Y., Tsai, C. L., et al. (2011). Assessing the impacts of experimentally elevated temperature on the biological composition and molecular chaperone gene expression of a reef coral. PLoS One 6:e26529. doi: 10.1371/journal.pone.0026529
Merchant, S. S. (2009). His protects heme as it crosses the membrane. Proc. Natl. Acad. Sci. U.S.A. 106, 10069–10070. doi: 10.1073/pnas.0905189106
Millaleo, R., Reyes-Diaz, M., Ivanov, A. G., Mora, M. L., and Alberdi, M. (2010). Manganese as essential and toxic element for plants: transport, accumulation and resistance mechanisms. J. Soil Sci. Plant Nutr. 10, 470–481.
Mitchelmore, C. L., Alan Verde, E., and Weis, V. M. (2007). Uptake and partitioning of copper and cadmium in the coral Pocillopora damicornis. Aquat. Toxicol. 85, 48–56. doi: 10.1016/j.aquatox.2007.07.015
Moreton, B. M., Fernandez, J. M., and Dolbecq, M. B. (2009). Development of a field preconcentration/elution unit for routine determination of dissolved metal concentrations by ICP-OES in marine waters: application for monitoring of the New Caledonia lagoon. Geostand. Geoanal. Res. 33, 205–218. doi: 10.1111/j.1751-908X.2009.00899.x
Morley, N. H., Burton, J. D., Tankere, S. P. C., and Martin, J. M. (1997). Distribution and behaviour of some dissolved trace metals in the western Mediterranean Sea. Deep Sea Res. Part II Top. Stud. Oceanogr. 44, 675–691.
Negri, A. P., and Hoogenboom, M. O. (2011). Water contamination reduces the tolerance of coral larvae to thermal stress. PLoS One 6:e19703. doi: 10.1371/journal.pone.0019703
Nielsen, D. A., Petrou, K., and Gates, R. D. (2018). Coral bleaching from a single cell perspective. ISME J. 12, 1558–1567. doi: 10.1038/s41396-018-0080-6
Nyström, M., Nordemar, I., and Tedengren, M. (2001). Simultaneous and sequential stress from increased temperature and copper on the metabolism of the hermatypic coral Porites cylindrica. Mar. Biol. 138, 1225–1231. doi: 10.1007/s002270100549
Oakley, C. A., and Davy, S. K. (2018). “Cell biology of coral bleaching,” in Coral Bleaching. Ecological Studies (Analysis and Synthesis), Vol. 233, eds M. van Oppen and J. Lough (Cham: Springer). doi: 10.1007/978-3-319-75393-5_8
Oliver, J. K., Berkelmans, R., and Eakin, C. M. (2018). “Coral bleaching in space and time,” in Coral Bleaching. Ecological Studies (Analysis and Synthesis, Vol. 233, eds M. van Oppen and J. Lough (Cham: Springer). doi: 10.1007/978-3-319-75393-5_3
Olsen, K., Ritson-Williams, R., Ochrietor, J. D., Paul, V. J., and Ross, C. (2013). Detecting hyperthermal stress in larvae of the hermatypic coral Porites astreoides: the suitability of using biomarkers of oxidative stress versus heat-shock protein transcriptional expression. Mar. Biol. 160, 2609–2618. doi: 10.1007/s00227-013-2255-z
Papanikolaou, G., and Pantopoulos, K. (2005). Iron metabolism and toxicity. Toxic. Appl. Pharmacol. 202, 199–211. doi: 10.1016/j.taap.2004.06.021
Papp, E., Nardai, G., Söti, C., and Csermely, P. (2003). Molecular chaperones, stress proteins and redox homeostasis. Biofactors 17, 249–257. doi: 10.1002/biof.5520170124
Patterson, J., Jeyasanta, K. I., Sathish, N., Edward, J. K. P., and Booth, A. M. (2020). Microplastic and heavy metal distributions in an Indian coral reef ecosystem. Sci. Total Environ. 744:140706. doi: 10.1016/j.scitotenv.2020.140706
Rädecker, N., Pogoreutz, C., Gegner, H. M., Cárdenas, A., Roth, F., Bougoure, J., et al. (2021). Heat stress destabilizes symbiotic nutrient cycling in corals. Proc. Natl. Acad. Sci. U.S.A. 118:e2022653118. doi: 10.1073/pnas.2022653118
Ranjbar Jafarabadi, A., Bakhtiari, A. R., Maisano, M., Pereira, P., and Cappello, T. (2018). First record of bioaccumulation and bioconcentration of metals in Scleractinian corals and their algal symbionts from Kharg and Lark coral reefs (Persian Gulf, Iran). Sci. Total Environ. 640-641, 1500–1511. doi: 10.1016/j.scitotenv.2018.06.029
Reich, H. G., Rodriguez, I. B., LaJeunesse, T. C., and Ho, T. Y. (2020). Endosymbiotic dinoflagellates pump iron: differences in iron and other trace metal needs among the Symbiodiniaceae. Coral Reefs 39, 915–927. doi: 10.1007/s00338-020-01911-z
Reichelt-Brushett, A., and Hudspith, M. (2016). The effects of metals of emerging concern on the fertilization success of gametes of the tropical scleractinian coral Platygyra daedalea. Chemosphere 150, 398–406. doi: 10.1016/j.chemosphere.2016.02.048
Rosenzweig, R., Nillegoda, N. B., Mayer, M. P., and Bukau, B. (2019). The Hsp70 chaperone network. Nat. Rev. Mol. Cell Biol. 20, 665–680.
Ross, C. (2014). Nitric oxide and heat shock protein 90 co-regulate temperature-induced bleaching in the soft coral Eunicea fusca. Coral Reefs 33, 513–522.
Rosset, S. L., Oakley, C. A., Ferrier-Pagès, C., Suggett, D. J., Weis, V. M., and Davy, S. K. (2020). The molecular language of the Cnidarian–Dinoflagellate symbiosis. Trends Microbiol. 29, 320–333. doi: 10.1016/j.tim.2020.08.005
Roth, M. S. (2014). The engine of the reef: photobiology of the coral-algal symbiosis. Front. Microbiol. 5:422. doi: 10.3389/fmicb.2014.00422
Santamaria, A. B. (2008). Manganese exposure, essentiality & toxicity. Indian J. Med. Res. 128, 484–500.
Schwarz, J. A., Mitchelmore, C. L., Jones, R., O’Dea, A., and Seymour, S. (2013). Exposure to copper induces oxidative and stress responses and DNA damage in the coral Montastraea franksi. Comp. Biochem. Physiol. C Toxicol. Pharmacol. 157, 272–279. doi: 10.1016/j.cbpc.2012.12.003
Seneca, F. O., Forêt, S., Ball, E. E., Smith-Keune, C., Miller, D. J., and van Oppen, M. J. (2010). Patterns of gene expression in a scleractinian coral undergoing natural bleaching. Mar. Biotechnol. 12, 594–604. doi: 10.1007/s10126-009-9247-5
Seveso, D., Arrigoni, R., Montano, S., Maggioni, D., Orlandi, I., Berumen, M. L., et al. (2020). Investigating the heat shock protein response involved in coral bleaching across scleractinian species in the central Red Sea. Coral Reefs 39, 85–98. doi: 10.1007/s00338-019-01878-6
Seveso, D., Montano, S., Maggioni, D., Pedretti, F., Orlandi, I., Galli, P., et al. (2018). Diel modulation of Hsp70 and Hsp60 in corals living in a shallow reef. Coral Reefs 37, 801–806. doi: 10.1007/s00338-018-1703-0
Seveso, D., Montano, S., Reggente, M. A. L., Maggioni, D., Orlandi, I., Galli, P., et al. (2017). The cellular stress response of the scleractinian coral Goniopora columna during the progression of the black band disease. Cell Stress Chaperones 22, 225–236. doi: 10.1007/s12192-016-0756
Seveso, D., Montano, S., Strona, G., Orlandi, I., Galli, P., and Vai, M. (2013). Exploring the effect of salinity changes on the levels of Hsp60 in the tropical coral Seriatopora caliendrum. Mar. Environ. Res. 90, 96–103. doi: 10.1016/j.marenvres.2013.06.002
Seveso, D., Montano, S., Strona, G., Orlandi, I., Galli, P., and Vai, M. (2014). The susceptibility of corals to thermal stress by analyzing Hsp60 expression. Mar. Environ. Res. 99, 69–75. doi: 10.1016/j.marenvres.2014.06.008
Seveso, D., Montano, S., Strona, G., Orlandi, I., Galli, P., and Vai, M. (2016). Hsp60 expression profiles in the reef-building coral Seriatopora caliendrum subjected to heat and cold shock regimes. Mar. Environ. Res. 119, 1–11.
Seveso, D., Montano, S., Strona, G., Orlandi, I., Vai, M., and Galli, P. (2012). Up-regulation of Hsp60 in response to skeleton eroding band disease but not by algal overgrowth in the scleractinian coral Acropora muricata. Mar. Environ. Res. 78, 34–39. doi: 10.1016/j.marenvres.2012.03.008
Shcolnick, S., and Keren, N. (2006). Metal homeostasis in cyanobacteria and chloroplasts. Balancing benefits and risks to the photosynthetic apparatus. Plant Physiol. 141, 805–810. doi: 10.1104/pp.106.079251
Shick, M. J., Iglic, K., Wells, M. L., Trick, C. G., Doyle, J., and Dunlap, W. C. (2011). Responses to iron limitation in two colonies of Stylophora pistillata exposed to high temperature: implications for coral bleaching. Limnol. Oceanogr. 56, 813–828. doi: 10.4319/lo.2011.56.3.0813
Siddiqui, S., and Bielmyer-Fraser, G. K. (2015). Responses of the sea anemone, Exaiptasia pallida, to ocean acidification conditions and copper exposure. Aquat. Toxicol. 167, 228–239. doi: 10.1016/j.aquatox.2015.08.012
Suggett, D. J., and Smith, D. J. (2020). Coral bleaching patterns are the outcome of complex biological and environmental networking. Glob. Change Biol. 26, 68–79. doi: 10.1111/gcb.14871
Vai, M., Popolo, L., and Alberghina, L. (1986). Immunological cross-reactivity of fungal and yeast plasma membrane H+-ATPase. FEBS Lett. 206, 135–141. doi: 10.1016/0014-5793(86)81355-2
van Dam, J. W., Negri, A. P., Uthicke, S., and Mueller, J. F. (2011). “Chemical pollution on Coral Reefs: exposure and ecological effects,” in Ecological Impact of Toxic Chemicals, eds F. Sanchez-Bayo, P. J. van den Brink, and R. M. Mann (Sharjah: Bentham Science Publishers Ltd).
Vance, P. G., Keele, B. B. Jr., and Rajagopalan, K. V. (1972). Superoxide dismutase from Streptococcus mutans. Isolation and characterization of two forms of the enzyme. J. Biol. Chem. 247, 4782–4786.
Venn, A. A., Quinn, J., Jones, R., and Bodnar, A. (2009). P-glycoprotein (multi-xenobiotic resistance) and heat shock protein gene expression in the reef coral Montastraea franksi in response to environmental toxicants. Aquat. Toxicol. 93, 188–195.
Verma, M. K., Jaiswal, A., Sharma, P., Kumar, P., and Singh, A. N. (2019). Oxidative stress and biomarker of TNF-α, MDA and FRAP in hypertension. J. Med. Life 12, 253–259. doi: 10.25122/jml-2019-0031
Vijayavel, K., Downs, C., Ostrander, G., and Richmond, R. (2012). Oxidative DNA damage induced by iron chloride in the larvae of the lace coral Pocillopora damicornis. Comp. Biochem. Physiol. C Toxicol. Pharmacol. 155, 275–280. doi: 10.1016/j.cbpc.2011.09.007
Wang, Y., Oberley, L. W., and Murhammer, D. W. (2001). Antioxidant defense systems of two lipidopteran insect cell lines. Free Radic. Biol. Med. 30, 1254–1262. doi: 10.1016/s0891-5849(01)00520-2
Weis, V. M. (2008). Cellular mechanisms of Cnidarian bleaching: stress causes the collapse of symbiosis. J. Exp. Biol. 211, 3059–3066. doi: 10.1242/jeb.009597
Wells, M., Shick, J. M., and Long, P. (2011). Effects of Trace Metal Limitation on Oxidative Stress in Zooxanthellae and Its Role in Coral Bleaching. University of Maine Office of Research and Sponsored Programs: Grant Reports Paper. (Orono, ME: University of Maine), 291.
Williams, A., Chiles, E. N., Conetta, D., Pathmanathan, J. S., Cleves, P. A., Putnam, H. M., et al. (2021). Metabolomic shifts associated with heat stress in coral holobionts. Sci. Adv. 7:eabd4210.
Yakovleva, I., Bhagooli, R., Takemura, A., and Hidaka, M. (2004). Differential susceptibility to oxidative stress of two scleractinian corals: antioxidant functioning of mycosporine-glycine. Comp. Biochem. Physiol. B Biochem. Mol. Biol. 139, 721–730. doi: 10.1016/j.cbpc.2004.08.016
Zhou, J., Bruns, M. A., and Tiedje, J. M. (1996). DNA recovery from soils of diverse composition. Appl. Environ. Microbiol. 62, 316–322. doi: 10.1128/AEM.62.2.316-322.1996
Keywords: metal enrichment, manganese, iron, thermal stress, Stylophora pistillata, coral bleaching, bleaching mitigation
Citation: Montalbetti E, Biscéré T, Ferrier-Pagès C, Houlbrèque F, Orlandi I, Forcella M, Galli P, Vai M and Seveso D (2021) Manganese Benefits Heat-Stressed Corals at the Cellular Level. Front. Mar. Sci. 8:681119. doi: 10.3389/fmars.2021.681119
Received: 15 March 2021; Accepted: 03 June 2021;
Published: 29 June 2021.
Edited by:
Valerio Matozzo, University of Padua, ItalyReviewed by:
Daniel Aagren Nielsen, University of Technology Sydney, AustraliaAdília Pires, University of Aveiro, Portugal
Copyright © 2021 Montalbetti, Biscéré, Ferrier-Pagès, Houlbrèque, Orlandi, Forcella, Galli, Vai and Seveso. This is an open-access article distributed under the terms of the Creative Commons Attribution License (CC BY). The use, distribution or reproduction in other forums is permitted, provided the original author(s) and the copyright owner(s) are credited and that the original publication in this journal is cited, in accordance with accepted academic practice. No use, distribution or reproduction is permitted which does not comply with these terms.
*Correspondence: Marina Vai, bWFyaW5hLnZhaUB1bmltaWIuaXQ=; Davide Seveso, ZGF2aWRlLnNldmVzb0B1bmltaWIuaXQ=