- Great Lakes Laboratory for Fisheries and Aquatic Sciences, Fisheries and Oceans Canada, Burlington, ON, Canada
Global coastal aquatic ecosystems are negatively impacted by the introduction of harmful aquatic species through the discharge of ships’ ballast water. To reduce discharges of harmful aquatic organisms and pathogens, such as toxic phytoplankton species, ships are now transitioning to the use of ballast water management systems (BWMS) instead of ballast water exchange (BWE). This study examines the abundance and diversity of phytoplankton in ballast water managed by BWMS (or a combination of both BWE + BWMS) in comparison to those in ballast water managed by BWE alone (collected from ships arriving to Canada’s Pacific coast in 2017–2018 and 2008, respectively). The abundance and diversity of phytoplankton species were also examined in relation to key variables such as ballast water salinity and ballast water age. Total abundance of phytoplankton was significantly lower in preserved samples managed by either a BWMS or BWE + BWMS compared to BWE alone. Abundances in preserved samples were higher than observed in fresh (unpreserved) samples at the time of collection, with all samples managed by a BWMS meeting international limits for the number of viable organisms ≥10 and <50 μm in minimum dimension (based on six 1-mL live counts). While there was no apparent influence of factors such as treatment type [e.g., ultraviolet (UV) or chlorine], presence of filtration, ballast water salinity, ballast water age, nor location of last ballast water uptake on phytoplankton abundances in preserved samples, power to detect differences may be limited by sample size. Ballast water managed by BWMS also tended to have lower abundances of harmful phytoplankton species, although the difference was not statistically significant – additional research into the community composition of live cells in fresh samples could be valuable to discriminate the risk associated with phytoplankton surviving ballast water treatment.
Introduction
To serve the demand of globalization, trade by ships has increased steadily in recent decades and will continue to do so, with the number, sizes, and speed of ships also increasing (Calatayud et al., 2017; Carney et al., 2017). To control a ship’s trim, stability and maneuverability, large volumes of water are pumped into ballast tanks when the ship is not carrying a full cargo load; ballast water is subsequently discharged when cargo is loaded. Consequently, waters taken on in ports around the world, including associated living organisms, are thus frequently discharged far from the source ecosystem. Merchant shipping transports approximately 3.1 billion metric tonnes of ballast water around the world each year (David et al., 2015). Casas-Monroy et al. (2015) estimated that more than 116 million tonnes of ballast water (and associated living organisms) are discharged in Canadian coastal port environments annually.
Several phytoplankton species transported in ballast water are able to survive the voyage (Casas-Monroy et al., 2016), facilitated by life history characteristics such as mixotrophy and resting stage formation (Taylor et al., 2008), and may establish self-sustaining populations after release in new areas (Hallegraeff, 1998). Several phytoplankton species are harmful or toxic, and have been associated with ship-mediated introductions in different countries [e.g., Australia: Hallegraeff and Bolch (1992); Great Britain: Hamer et al. (2001)], sometimes with dramatic ecological and economic consequences, particularly for aquaculture farmers (Trottet et al., 2021). In North America, commercial ships have been implicated in the dispersal of the brown tide Aureococcus anophagefferens Hargraves et Sieburth, into the Great Lakes (Doblin et al., 2004). Thus the transport and introduction of non-indigenous species and harmful species of phytoplankton by ballast water is a pressing environmental problem worldwide (Bailey, 2015; Casas-Monroy et al., 2015; Bailey et al., 2020).
Over the past two decades, Canada and numerous other countries have implemented ballast water regulations to reduce the abundance of non-indigenous and harmful species being transported. The most commonly used ballast water management method to date has been offshore ballast water exchange (BWE) (Murphy et al., 2004). BWE was first recommended by Canada for use by ships arriving to the Laurentian Great Lakes in the late 1980s, and became mandatory under United States rules in 1993 (Canadian Coast Guard, 1989; United States Coast Guard [USCG], 1993). BWE involves replacing coastal source ballast water with offshore ocean water to purge high-risk harmful nearshore species from ballast water tanks; the incoming oceanic water can also be lethal to any residual organisms remaining in ballast tanks, particularly if they originated from freshwater or low-salinity environments (Locke et al., 1993; Bailey, 2015). Although BWE can be up to 99% effective based on volumetric exchange, studies have shown that biological efficacy can be much lower (29–40%), sometimes even increasing the abundance of microplankton such as protists (Molina and Drake, 2016).
In 2004, the International Maritime Organization (IMO) adopted the International Convention for the Control and Management of Ships’ Ballast Water and Sediments. Regulation D-2 restricts the concentration of viable organisms ≥50 μm in minimum dimension (to <10 per cubic meter) and ≥10 and <50 μm in minimum dimension (to <10 per milliliter) in ballast water discharge [IMO (International Maritime Organization), 2004]. With the Convention entering into force in 2017, most international ships are expected to install and use a ballast water management system (BWMS) by September 2024 to treat ballast water prior to release. A variety of BWMS have been developed which typically include two or three steps to disinfect ballast water. As an initial step, most BWMS use filtration to remove organisms larger than 50 μm. The secondary step involves a chemical or physical disinfection process such as electrochlorination, ozonation, or ultraviolet (UV) irradiation as main treatments. The third step can be a second application of UV treatment or, for chemical treatments, a neutralization step prior to discharge to the environment to meet maximum allowable discharge concentrations set by the IMO (typically 0.2–0.1 mg L–1 Total Residual Oxidants; GESAMP, 2016). Among those BWMS approved and commercially available, filtration devices are included in almost 80% of BWMS (Batista et al., 2017). Electrolytic disinfection devices are in almost 37%, UV irradiation devices in 31%, oxidation in 11.2%, ultrasound in 11%, disinfectant in 9%, and other technologies in 1% of BWMS, respectively (DESMI, 2020).
Depending on the treatment process, efficacy may be variable across taxonomic groups and different life stages may be more tolerant of treatment than others (de Lafontaine et al., 2009; Gregg et al., 2009; Bakalar, 2016; Casas-Monroy et al., 2018). Other factors may also influence the efficacy of ballast water treatment such as turbidity (Stehouwer et al., 2015) or source of ballast water (which in turn will determine the water quality, species composition and abundance). In recent years, numerous studies have shown that protection can be increased by combining BWE and BWMS, most notably when significant salinity changes are involved (e.g., Briski et al., 2015; Paolucci et al., 2015; Bradie et al., 2020). Furthermore, BWE may enhance BWMS efficacy since oceanic water may pose a lower challenge for treatment, generally being less turbid and having lower abundances of organisms in comparison to harbor water (Briski et al., 2013).
Technological advances in ballast water management have occurred in parallel with improvements in methods for sample collection and sample analysis. Historically, ballast water samples have been concentrated and preserved with Lugol’s acid to estimate the number of cells and to conduct taxonomic analysis of phytoplankton in ballast water (e.g., Burkholder et al., 2007). For preserved samples, typically, it has been assumed that organisms with intact cellular content were alive at the time of collection, although Lugol’s acid stains and shrinks cells resulting in difficult taxonomic identification. More recently (last 10–15 years), abundance and viability of cells have been assessed using fresh samples (unpreserved) in line with IMO guidelines for sample analysis associated with type approval testing of BWMS [IMO (International Maritime Organization), 2016; see also Veldhuis and Kraay, 2000; NSF International, 2010; Steinberg et al., 2011; Gollasch and David, 2021]; samples should not be concentrated for analysis unless the procedure has been validated to ensure that cells are not damaged by the process, however, validation is less feasible for shipboard ballast water sampling than at land-based type approval facilities. As cell abundances will be lower in unconcentrated samples, a sufficient volume must be analyzed to obtain a reliable estimate of the number of living cells (NSF International, 2010). In some cases, analysis of fresh samples also allows for observation of cell colors and movement, characteristics useful for taxonomic identification.
This study compares the abundance and diversity of phytoplankton in ballast water managed using a BWMS (or BWE + BWMS) to those in ballast water managed by BWE. We examine changes in community composition corresponding with different management strategies based on preserved samples and test the null hypothesis that there is no significant difference in the abundance of harmful species between ballast water management strategies. We also aimed to determine if ships using BWMS are consistently meeting the IMO Regulation D-2 standard for viable organisms ≥10 and <50 μm in minimum dimension (based on fresh samples).
Materials and Methods
Sampling Ships With BWMS
Ballast water samples were collected from international ships arriving at various terminals within the port of Vancouver (BC, Canada) during two consecutive summers (2017–2018). Once on board, detailed information about the BWMS, the ballast water history (i.e., use of BWMS or BWE + BWMS; most recent ballast water source) were obtained from each ships’ Canadian Ballast Water Reporting Form and confirmed through interviews with ships’ Officers on board. Sampling was conducted in each ship’s engine room using the in-line ballast water sampling port associated with the BMWS. The in-line sampling port provides access to treated ballast water during de-ballasting operations. A representative sample of treated ballast water was collected during ballast discharge (from a single or paired ballast tanks) following procedures recommended by ICES/IOC/IMO Working Group on Ballast and Other Ship Vectors (2017) and the IMO (International Maritime Organization) (2019).
Approximately 30 L of ballast water were collected as a continuous subsample of the main ballast discharge at flow rates within the isokinetic sampling volume range. Flow rate was continually adjusted to maintain a sample flow rate between 1 and 2 Diso (isokinetic diameter) throughout sample collection. The specific volume collected and sampling duration were dependent on each ship’s ballast pump flow rate. In most cases sampling duration was approximately 50 min. The ballast water sample was then mixed well and split into a 10 L subsample (for preservation and later taxonomic assessment) and a 1 L subsample for live counts of organisms ≥10 and < 50 μm in minimum dimension using epifluorescence microscopy. The 10 L subsample was poured onto a 10 μm nitex mesh and the material retained on the mesh was rinsed, using 10 μm filtered ballast water, into a 250 mL graduated cylinder, stopping at 240 mL volume. The 240 mL were poured into a 250 mL brown plastic (HDPE) bottle using a funnel, and the graduated cylinder was rinsed twice using 5 mL of filtered ballast water each time to achieve a final volume of 250 mL. The brown bottle was pre-filled with 3.75 mL of Lugol’s acid to give a final concentration of 1.5% for preservation. Samples were kept at room temperature in the dark until microscopic examination of organisms ≥10 and <50 μm in minimum dimension within 12 months after sampling. The 1 L fresh subsample was kept in a dark, insulated container alongside insulated ice packs (samples kept near or below ambient ballast water temperature) for transport off ship for enumeration of live cells upon return to the laboratory (typically completed within 2.5–3 h of sample collection).
Ballast Water Exchange Data
For comparison purposes, BWE data were compiled from prior studies conducted in Canada during 2007–2009 (Klein et al., 2009; Roy et al., 2012; Casas-Monroy et al., 2016); only data for international ships arriving to the port of Vancouver (in 2008) were used since these were directly comparable to the 2017–2018 data. Detailed sample collection methods for these data, which were in line with recommendations of the IMO (International Maritime Organization) (2008) at that time, are provided in Klein et al. (2009) and Casas-Monroy et al. (2016). Briefly, samples of exchanged ballast water were collected with a 5 L Niskin bottle sampler through a single opened ballast tank “manhole” on each ship. For each ship, water was collected at four depths evenly distributed between the top and bottom of the accessible water column depth and mixed in a 20 L plastic bottle. A 3 L volume of raw (unprocessed) water was subsampled for analysis of diatoms (Bacillariophyceae). For dinoflagellates (Dinophyceae), at least 10 L were sieved through 73 μm, retaining the fraction collected on a secondary 20 μm mesh. Both subsamples (for analysis of diatoms and dinoflagellates) were then preserved with Lugol’s acid. Samples were kept at 4°C in the dark until microscopic examination of organisms ≥10 and <50 μm in minimum dimension within 12 months after sampling.
Ballast Water Sample Analysis
Preserved samples collected in 2017–2018 were processed to examine community composition (abundance and species diversity, considering both total and potentially harmful species) using the same methods as earlier BWE studies [Utermöhl (1958)] except that during the earlier study samples were split for separate examination of diatom and dinoflagellate taxa whereas phytoplankton (all taxa present) were assessed by a single analyst in 2017–2018. Only intact cells bigger than 10 μm (in minimum dimension), with clearly visible cell content were counted as being “potentially viable” organisms at the time of preservation. Taxonomic nomenclature used follows: Taylor and Waters (1982); Taylor et al. (2003); Klein et al. (2009, 2010); Roy et al. (2012); Casas-Monroy et al. (2016); and references therein. Samples were observed using a Zeiss Axio Vert.A1 inverted transmitted light/reflected light fluorescence microscope (with fluorescence excitation based on LED modules; Carl Zeiss Canada Ltd., Toronto, ON, Canada) at 250× to 1,000× magnification. Information about species distributions were obtained from the Ocean Biogeographic Information System (OBIS, 2020) and AlgaeBase (2020), and classified as freshwater, coastal and/or oceanic according to habitat types typically associated with each species based on literature review (Tomas, 1997; Bérard-Therriault et al., 1999; Villac et al., 2016). Species were considered potentially harmful algae as listed in the IOC–UNESCO Taxonomic Reference List of Harmful Microalgae.
Fresh samples (1 L subsamples without Lugol’s acid) collected in 2017–2018 were used to count live phytoplankton cells for evaluation against the relevant standard in Regulation D-2. Detailed information about the protocol can be found in Adams et al. (2014). Briefly, 417 μL of fluorescein diacetate (FDA) working solution was added to 5 mL of sample water in a 20 mL glass vial for “staining.” Samples were incubated in the dark for 10 min, after which 1 mL was transferred to a 1 mL gridded Sedgewick–Rafter cell and fluorescing cells were counted under a Zeiss Axiovert A1 inverted microscope FITC, equipped with fluorescence excitation based on LED modules. The number of living cells bigger than 10 μm (in minimum dimension), the number of colonies and the number of cells in each colony were recorded. Six 1 mL subsamples were analyzed and, as the abundances in all samples were low, the entire chamber was counted each time.
Location of Ballast Water Management
A map of most recent ballast water sources for sampled ships was created using the World Geodesic System (WGS84) Mercator Pacific Ocean projection within ArcGIS version 10.7.1 (Environmental Systems Research Institute, 2018) and partitioned based on FAO’s major fisheries oceanic regions (FAO, 2020). Ballast source locations were classified as coastal (defined as less than 50 nautical miles from shore) or oceanic (>50 nm from shore, in waters at least 2,000 m depth – as per Canadian BWE requirements), for corresponding analysis of the abundance of total and harmful species in ballast water samples (Figure 1).
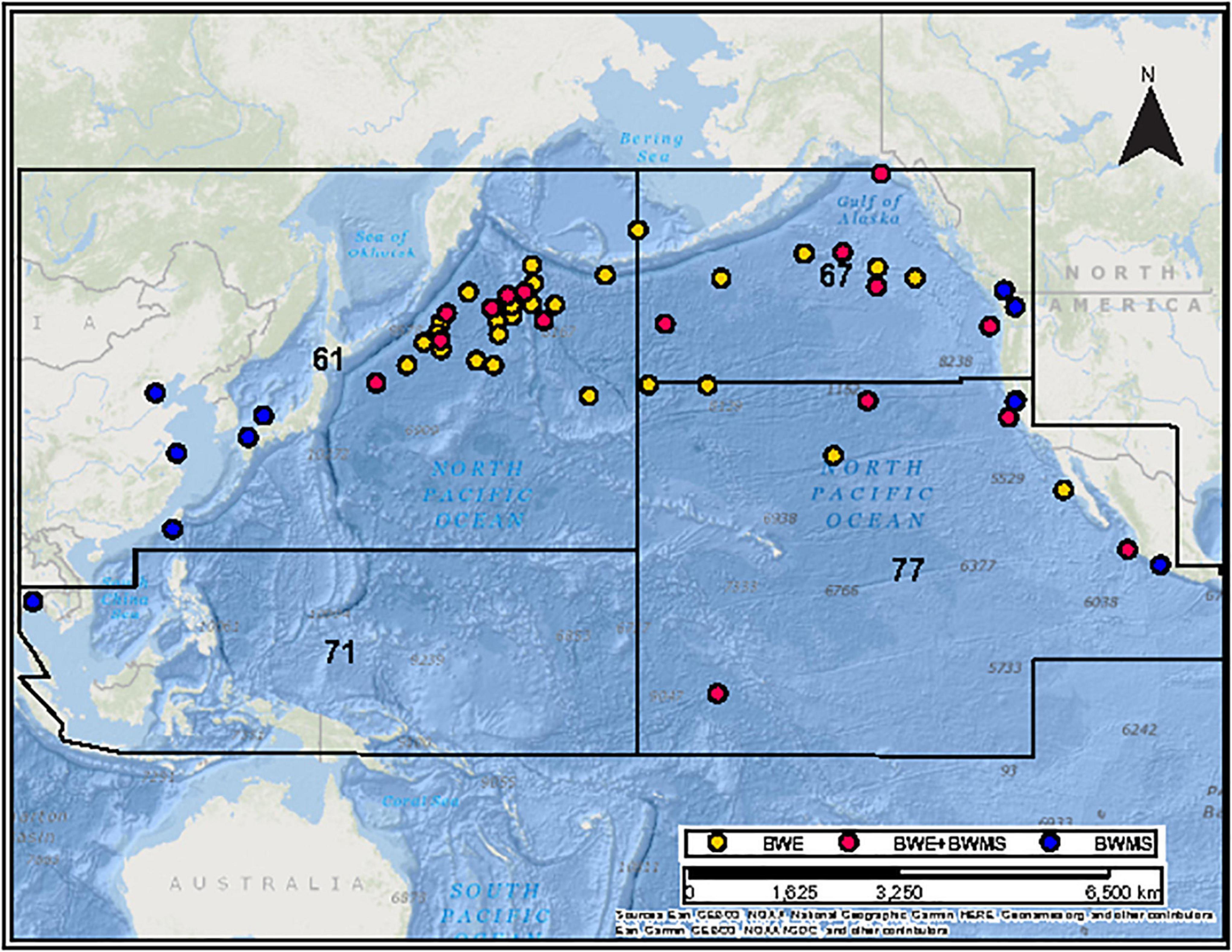
Figure 1. Map showing the location of most recent ballast uptake by ships using different ballast water management strategies: yellow dots denote ships performing ballast water exchange (BWE), red dots denote ships performing BWE + ballast water management system (BWMS) and blue dots denote ships using exclusively BWMS. Numbers denote major fisheries regions of the Food and Agriculture Organization of the United Nations: 61 – Pacific Northwest; 67 – Pacific Northeast; 71 – Pacific Western Central; 77 – Pacific Eastern Central.
Statistical Analysis
Abundance of total and harmful species in preserved samples (2008, 2017–2018) were analyzed fitting a negative binomial generalized linear model (GLM) to examine the relationships with independent variables such as management strategy (i.e., BWE alone, BWE + BWMS, or BWMS alone), ballast water salinity, presence or absence of filtration, ballast water age, and FAO region (i.e., location of ballast water management). Subsequently, a power analysis was conducted (using the R package “pwr”) to determine if sample size was sufficient to detect an effect of filtration. Where significant differences occurred between levels of a category and a given variable, pairwise comparisons were conducted using Tukey’s HSD test included in the estimated marginal means (or least squares means) R package (Lenth, 2019), and α = 0.05 was used to define statistical significance. All statistical analyses were performed using R software (version 3.6, R Development Core Team, 2020). For fresh samples, the mean abundance of live phytoplankton cells was calculated from the six 1-ml subsample counts and confidence intervals were calculated following a Poisson distribution as recommended by the Generic Protocol for Verification of Ballast Water Treatment Technology (NSF International, 2010). Results from fresh samples collected in 2017–2018 were used only for comparison against the D-2 standard.
Results
Sample Population
During the 2017–2018 study, treated ballast water was collected from twenty-seven ships fitted with eleven different BWMS models. Of these, 15 ships used a BWMS based on a physical treatment method (i.e., UV irradiation) while 12 used a chemical-based method such as electrochlorination (n = 7), ozonation (n = 2), chlorine injection (n = 2), or production of hydroxyl radicals (–OH) (n = 1) (Table 1). Filtration was a component (first step of treatment) included in all physical-based BWMS models (nominal mesh sizes ranging between 20 and 50 μm), while for chemical-based BWMS only three models used filtration (nominal mesh sizes ranging between 40 and 55 μm), one used large mesh strainers (3 mm), and the remaining two models did not use any filtration step (Table 1). All of the chemical-based BWMS had the capability to inject a neutralizer solution before discharge of treated ballast water to the environment, if needed (as indicated by measurements of Total Residual Oxidants by sensors installed as part of the BWMS). Eleven ships exclusively used their BWMS to manage coastal source ballast water (salinity 8–35) while the remaining sixteen ships performed BWE in addition to using a BWMS (BWE + BWMS), thereby applying treatment to oceanic source ballast water (salinity ≥ 30). The ballast water age at time of sampling ranged from 4 to 32 days (median 14 days).
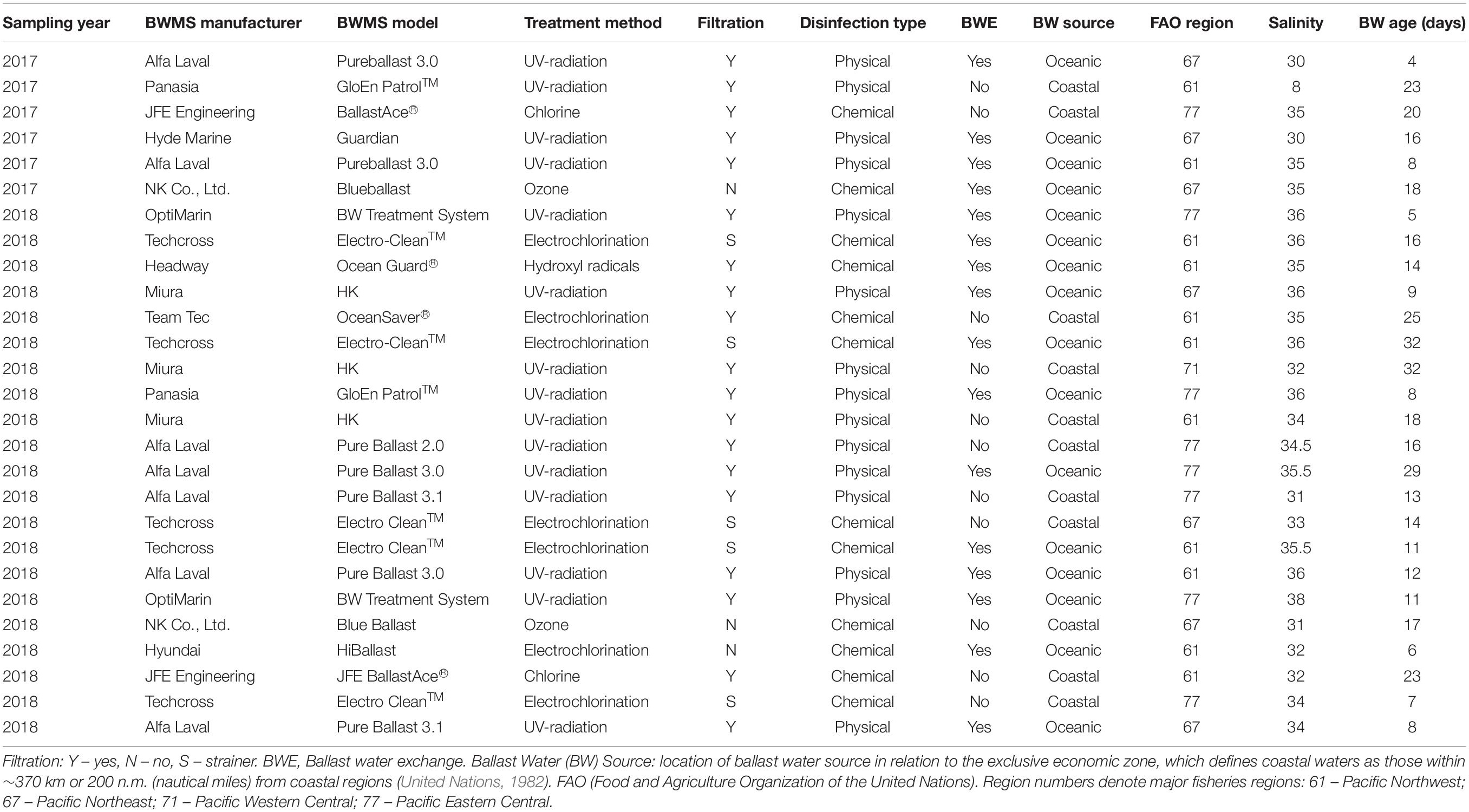
Table 1. Characteristics of the different ballast water management systems (BWMS) and ballast water histories for ships sampled in 2017–2018.
During the 2007–2009 study, exchanged ballast water was collected from a total of twenty seven ships arriving to different terminals at the port of Vancouver. All sampled ships had performed BWE in oceanic environments. As a result, the salinity of ballast water measured from sampled tanks ranged from 25 to 36. The ballast water age at time of sampling ranged from 0 to 25 days (median 4 days) (Klein et al., 2009; Roy et al., 2012; Casas-Monroy et al., 2016).
Community Composition in Treated Ballast Water Samples
Total abundances (all species) of phytoplankton in preserved treated ballast water samples ranged from 5 to 1,702 cells mL–1 (median 146 and 106 cells mL–1 for BWMS and BWE + BWMS samples, respectively) (Table 2). Total abundances were similar in treated samples, between chlorine-based (median 108 cells mL–1, across 10 ships) and UV treatment (median 86 cells mL–1, across 15 ships), while being higher for ozone treatment (range 349–338 cells mL–1, across 2 ships). Community composition was similar between samples subject to BWMS-only and BWE + BWMS, although the latter had higher relative abundance of Dinophyceae and greater frequency of occurrence for all taxonomic groups (Table 3). The diversity of Bacillariophyceae and Dinophyceae (116 and 78 species, respectively) was also higher in samples collected after BWE + BWMS. Most phytoplankton identified were considered marine species, although freshwater species were present in low abundance across ships (Supplementary Appendix 1).

Table 2. Average abundance (cells mL–1 ship-1) and cumulative number of total and harmful phytoplankton species (spp.) observed in ballast water samples from ships using different ballast water management strategies: ballast water exchange (BWE), BWMS alone, or combining BWE + BWMS.
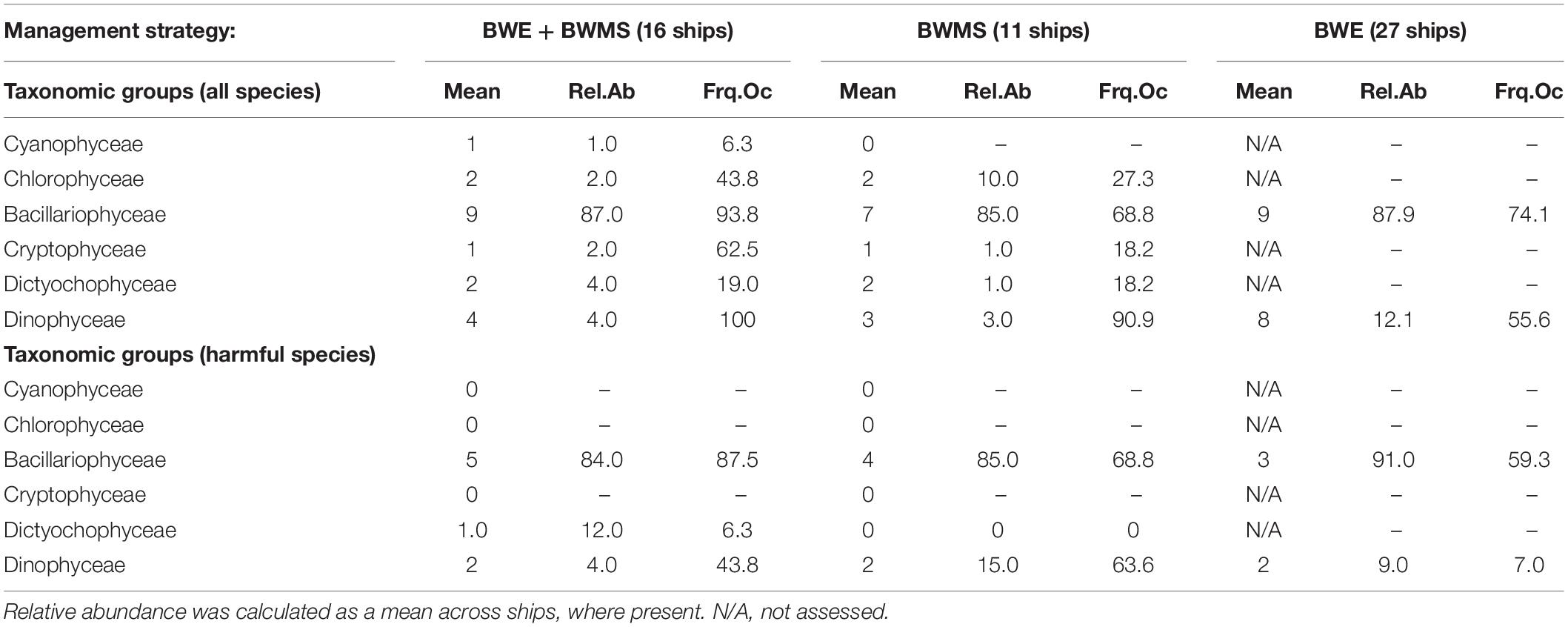
Table 3. Statistics for the mean number of species, relative abundance (%), and the frequency of occurrence (%) of total and harmful phytoplankton identified from ballast water samples, by taxonomic group and management strategy.
The abundance of harmful phytoplankton species ranged from 0 to 844 cells mL–1 in preserved treated ballast water samples. Harmful species of Bacillariophyceae and Dinophyceae were documented in samples from ships using both BWMS management strategies, with Bacillariophyceae dominating relative abundance (Table 2). Harmful Bacillariophyceae were documented more frequently from BWE + BWMS samples, while harmful Dinophyceae had higher frequency of occurrence in BWMS-only samples. Across all treated samples, nine Bacillariophyceae and six Dinophyceae harmful species were identified (Supplementary Appendix 1). The most frequently occurring harmful diatom species were Ditylum brightwellii (T.West) Grunow, Pseudo-nitzschia delicatissima complex, Rhizosolenia setigera Brightwell, and Thalassiosira rotula Meunier while the most frequently observed harmful dinoflagellate species were Dinophysis norvegica Claparède and Lachmann, Dinophysis acuta Ehrenberg (both associated with diarrhetic shellfish poisoning), and Tripos furca (Ehrenberg) F. Gómez. Overall, 66.7% of sampled ships had at least one harmful species in their treated ballast water. The abundance of harmful diatoms (cells mL–1) was typically greater than that of harmful dinoflagellates (Supplementary Appendix 1).
Community Composition in Exchanged Ballast Water Samples
Total abundances (all species) of phytoplankton in preserved exchanged samples ranged from 1 to 11,574 cells mL–1 (median 122 cells mL–1) (Table 3). Bacillariophyceae and Dinophyceae were the only taxa enumerated, with similar relative abundances to those observed in samples managed by BWMS (Table 2). The frequency of occurrence of Dinophyceae was lower in samples of exchanged ballast water compared to treated ballast water samples. A maximum of 70 and 50 Bacillariophyceae and Dinophyceae species were identified, respectively; there was an overlap of 42 species also reported in BWE + BWMS or BWMS samples (most being harmful species; Supplementary Appendix 1). Most phytoplankton in exchanged samples were considered marine species, although species able to live in freshwater ecosystems (e.g., Asterionella formosa Hassall) were observed in five samples.
The abundance of harmful phytoplankton species ranged from 0 to 1,460 cells mL–1 in preserved exchanged ballast water samples. The most frequently documented harmful diatom species were Cylindrotheca closterium (Ehrenberg) Reimann & J. C. Lewin, D. brightwellii, and Pseudo-nitzschia pungens (Grunow ex Cleve) Hasle, while the most frequently observed harmful dinoflagellates were Dinophysis acuminata Claparède & Lachmann and Phalacroma rotundatum (Claparède & Lachmann) Kofoid & Michener. Overall, 85.2% of sampled ships that performed BWE had at least one harmful species. The abundance of harmful diatoms (cells mL–1) was typically greater than that for harmful dinoflagellates (Supplementary Appendix 1).
On average, the three management strategies showed similar numbers of total species (11–16 spp.) and harmful species (4–6 spp.) per ship.
Live Counts
Total abundances measured from fresh (unpreserved) BWMS samples ranged from 0 to 5 cells mL–1. Mean abundance of live cells in samples managed by BWMS alone with filtration (0.5 cells mL–1) was lower compared to those managed by BWE + BWMS (1.5 cells mL–1) with filtration, however, a power analysis using the R package “pwr” showed sample size was too small to determine if the difference is statistically significant. All mean total abundance values from samples treated by BWMS were below the standard in Regulation D-2 (with or without BWE). Only one sample had live cell abundances close to the limit when taking into account the confidence intervals around the mean (mean = 4.15, C.I. lower = 1.4, and C.I. upper = 9.1 cells mL–1).
Effect of Ballast Water Source Location
The 11 ships using BWMS alone last loaded ballast water at ports in Asia or North America (coastal ballast water sources, FAO regions 61, 67, 71, and 77) (Figure 1); all preserved samples contained harmful species, most of which are typically associated with oceanic habitats. Ships using BWE + BWMS loaded oceanic ballast water in FAO regions 61, 67, and 77; all but one BWE + BWMS sample contained harmful species. The 27 ships using BWE alone loaded oceanic ballast water in FAO regions 61, 67, and 77 (Figure 1); from these preserved samples 67% contained harmful species. Samples with high abundance of harmful species were not associated with any single FAO region.
Model Results
The GLM analyses run as a function of ballast water salinity (df = 53; AIC = 728.7; θ = 0.38; and SE = 0.06) or ballast water age (df = 53; AIC = 758.5; θ = 0.262; and SE = 0.04) showed that the total abundance (all species) of phytoplankton was significantly different in samples from ships performing BWE than those using BWMS only (Z-value = −2.79; P-value < 0.001) and BWE + BWMS (Z-value = −2.81; P-value < 0.001). No difference in total abundance was observed between ships using only BWMS or BWE + BWMS (Figure 2A). There was no significant difference in the abundance of harmful phytoplankton species across the three management strategies (Figure 2B).
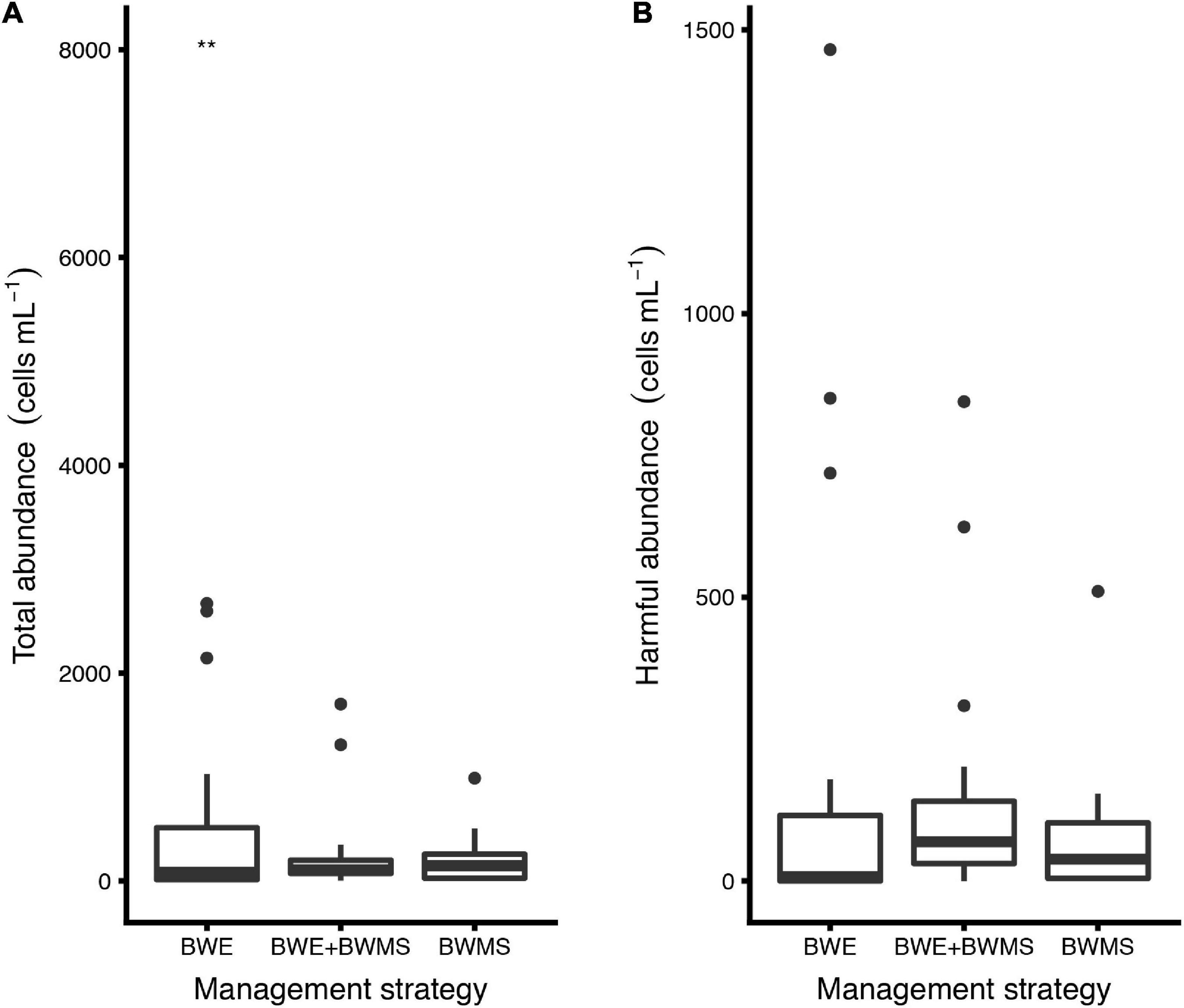
Figure 2. Estimated abundance of (A) total and (B) harmful phytoplankton species in preserved ballast water samples collected from ships using different ballast water management strategies (BWMS, ballast water management system; BWE, ballast water exchange). In the box plots, the boundary of the box closest to zero indicates the 25th percentile, the black line within the box marks the median, and the boundary of the box farthest from zero indicates the 75th percentile. Whiskers above and below the box indicate the 10th and 90th percentiles. Points above the whiskers indicate outliers outside the 90th percentile. Note difference in scale for y-axis. Asterisks denote statistically significant differences (p-value < 0.001).
The model did not support the presence of filtration as a main factor influencing abundance of phytoplankton in preserved ballast water samples (either total or harmful species; Figure 3), although there was insufficient power to detect differences (based on power analysis, results not shown). Samples managed by a BWMS having a filtration step tended to have higher total abundances of phytoplankton in preserved ballast water samples than those without a filtration step, though this is confounded by individual ballast histories (ballast water sources).
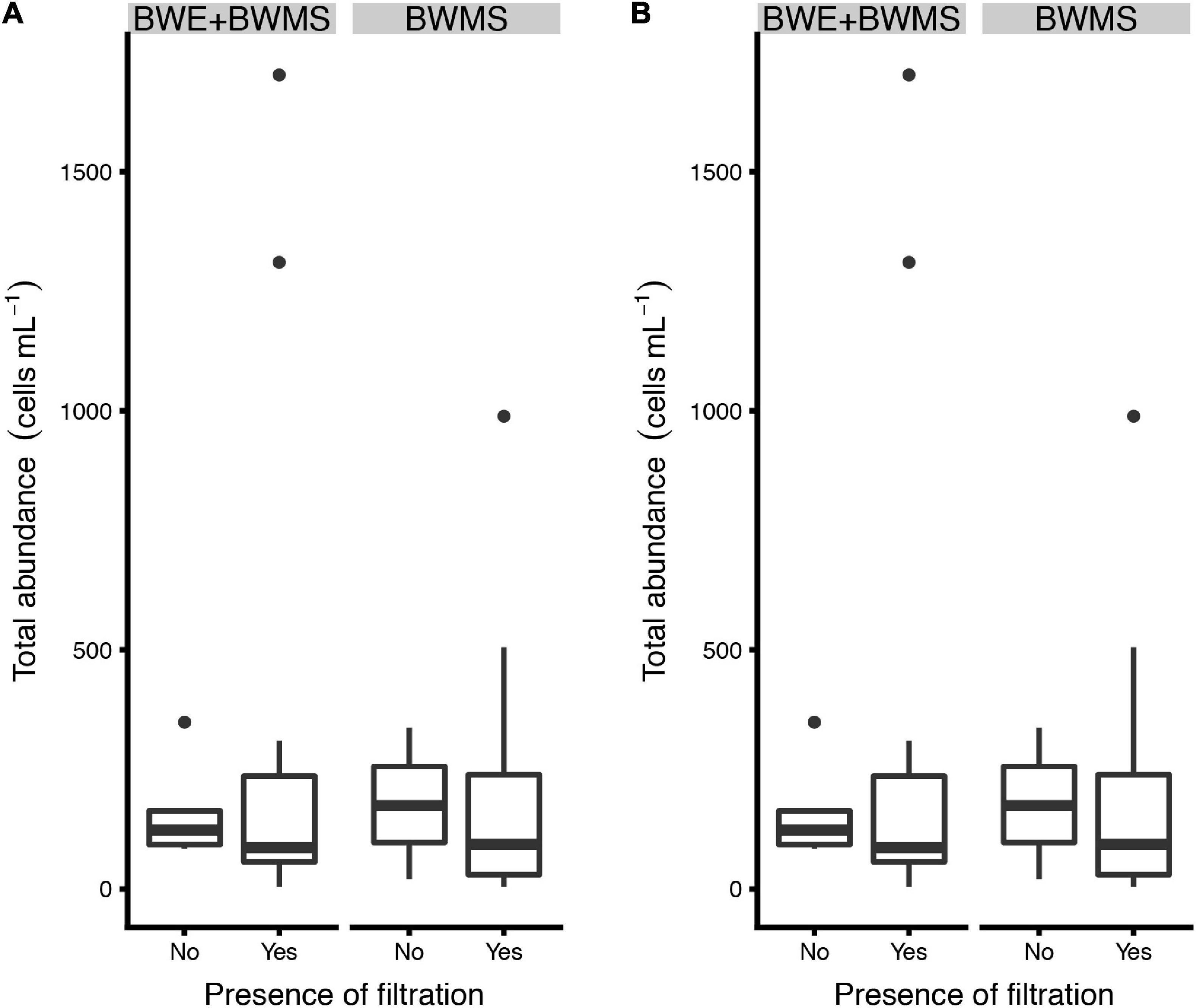
Figure 3. Estimated abundance of (A) total and (B) harmful phytoplankton species present in preserved ballast water samples collected from ships using BWMS with or without a filtration step; ships using a combined strategy of ballast water exchange and treatment (BWE + BWMS) are shown separately from those using a BWMS alone. In the box plots, the boundary of the box closest to zero indicates the 25th percentile, the black line within the box marks the median, and the boundary of the box farthest from zero indicates the 75th percentile. Whiskers above and below the box indicate the 10th and 90th percentiles. Points above the whiskers indicate outliers outside the 90th percentile.
Discussion
In this study, ballast water samples managed by a BWMS consistently met the D-2 Regulation limiting the number of viable organisms ≥10 and <50 μm in minimum dimension (based on six 1-mL live counts using FDA as an indicator of viable cells conducted immediately after sample collection). As of October 2019, nearly 10,000 BWMS have been installed on ships within the global fleet (DESMI, 2020). The use of new BWMS, alone or in combination with BWE, appears to significantly reduce the total abundance of phytoplankton being discharged in ballast water in comparison to the use of BWE alone (based on community composition in preserved samples). Ballast water managed by BWMS also tends to have lower abundances of harmful phytoplankton species than with BWE (although not statistically significant in this study). Interestingly, we found no influence of factors such as treatment type (e.g., UV or chlorine), presence of filtration, ballast water characteristics (i.e., salinity or ballast water age), nor location of last ballast water uptake on phytoplankton abundances (total or harmful species) in preserved samples, however, ability to detect differences may be limited by sample size.
While there has been very limited research on the use of BWMS on operational ships, the results of our study are consistent with general patterns observed during previous research. In an assessment of BWMS alone vs. BWE + BWMS, Briski et al. (2015) found an increasing effect of combining BWE with BWMS with greater abundance of organisms, but found no difference in the two management strategies when abundances were low. Briski et al. (2015) also found a larger effect of BWE + BWMS on community composition of treated ballast water samples, owing to their targeted research objective to examine voyages having freshwater ballast prior to management. Shipboard studies conducted by Paolucci et al. (2015, 2017) similarly found that treatment, alone or combined with exchange, lowered total abundance of viable microplankton (≥10 and <50 μm size class). While Paolucci et al. (2015, 2017) found only the combined management method met the D-2 standard, it should be noted that chlorine was applied directly to ballast tanks from the ship’s deck without a primary filtration step, so results are not directly comparable with those of BWMS.
As historical surveys of ballast water did not evaluate fresh samples for living cells as is currently recommended, this study conducted comparisons of phytoplankton abundance in preserved samples of treated ballast water to that in preserved samples of exchanged ballast water collected in 2008 (using the same analysis methods) (Klein et al., 2009; Roy et al., 2012; Casas-Monroy et al., 2016). This also enabled an assessment of the different sample handling and analysis methods. Regardless of the management strategy, this research found that preserved ballast water samples had significantly higher phytoplankton abundances compared to fresh samples (live counts), possibly explained by different laboratory analysis methods. While both fresh and preserved samples were taken from the same, well-mixed container of water at the time of collection on board the ship, different volumes were subsequently examined using different indicators of viability. Preserved samples were analyzed using the Utermöhl method (counting 50 mL of 40× concentrated sample in a sedimentation chamber), whereas six 1-mL aliquots of fresh unconcentrated sample were analyzed using a Sedgewick–Rafter plate. Counting a larger, concentrated volume is likely to improve sample representativeness, as well as detection and confidence limits (e.g., Costa et al., 2016); however, live cells could be damaged or lost (trapped in or passing through the filter mesh) during the concentration process. Further, one of the drawbacks of live samples is that they must be analyzed within a relatively short period of time (i.e., a few hours) following sample collection to avoid organism mortality, limiting the number of subsamples that can be examined to determine cell concentration.
It will be beneficial to conduct future research to better understand the underlying mechanism(s) for the observed differences between counts in fresh vs. preserved samples and to determine if concentrating fresh ballast water samples provides a more accurate or sensitive assessment of cell abundances. Three recent shipboard studies examining phytoplankton communities in ballast water samples have performed concentration for live sample analysis (Maranda et al., 2013; Briski et al., 2015; Bradie et al., 2018), although it is not clear if these methods were formally validated. Preliminary laboratory experiments conducted to assess any effect of sample concentration on Hamilton Harbor water (fresh water from Lake Ontario, Canada) show no statistical difference in the number of cells mL–1 in live samples examined with and without concentration for two of three trials (see Supplementary Appendix 2). It will be necessary to confirm the suitability of concentration methods when cells are stressed after transport in ballast water.
A second possible explanation for differences in cell counts between preserved and live samples is the methodology used during enumeration. In line with historical methods for assessment of preserved samples, only cells with intact cell structures and cell content were counted, as these are assumed to have been alive at the time of sample collection. In contrast, FDA was used as a vital marker for assessment of fresh samples, where cells that take up FDA and appear fluorescent during evaluation are counted as live. While both methods have been widely used to assess phytoplankton samples, the error rates (rates of false positives and false negatives) are known to vary by species, by environment and also by analyst (e.g., Roy et al., 2012; MacIntyre and Cullen, 2016; First et al., 2020). Additional research evaluating historical and more recently recommended methods against “known” concentrations of viable cells, and their utility for assessment of treated ballast water samples would also be valuable.
Conclusion
The use of new BWMS, alone or in combination with BWE, appears to significantly reduce the total abundance of phytoplankton species being discharged in ballast water in comparison to the use of BWE. In this study, all ballast water samples managed by a BWMS met the D-2 Regulation limiting the number of viable organisms ≥10 and <50 μm in minimum dimension (based on live counts). While there was no apparent influence of factors such as treatment type (e.g., UV or chlorine), presence of filtration, ballast water characteristics (i.e., salinity or ballast water age), nor location of last ballast water uptake on phytoplankton abundances in preserved samples, power to detect differences may be limited by sample size. Ballast water managed by BWMS tended to have lower abundances of harmful phytoplankton species, although the difference was not statistically significant – additional research into the community composition of live cells in fresh samples could be valuable to discriminate the risk associated with phytoplankton surviving ballast water treatment.
Data Availability Statement
The original contributions presented in the study are included in the article/Supplementary Material, further inquiries can be directed to the corresponding author.
Author Contributions
Both authors have made a substantial, direct and intellectual contribution to the work, and approved it for publication.
Funding
This study was funded by Transport Canada and Fisheries, and Oceans Canada.
Conflict of Interest
The authors declare that the research was conducted in the absence of any commercial or financial relationships that could be construed as a potential conflict of interest.
Publisher’s Note
All claims expressed in this article are solely those of the authors and do not necessarily represent those of their affiliated organizations, or those of the publisher, the editors and the reviewers. Any product that may be evaluated in this article, or claim that may be made by its manufacturer, is not guaranteed or endorsed by the publisher.
Acknowledgments
The authors gratefully acknowledge all ship crews, agents, operators, and owners, for their participation in this research. The authors thank former and current members of the ballast water field sampling team (R. Dallas Linley, Alexis Burt, Stuart Campbell, Torben Brydges, Jocelyn Kydd, and Robin Rozon) for their dedication to collect samples, and Dawson Ogilvie for constructive comments on an earlier draft. The authors also thank the joint Working Group on Ballast and Other Ship Vectors of the International Council for the Exploration of the Sea, Intergovernmental Oceanographic Commission of UNESCO, and International Maritime Organization, as discussions during annual meetings enriched this research.
Supplementary Material
The Supplementary Material for this article can be found online at: https://www.frontiersin.org/articles/10.3389/fmars.2021.691723/full#supplementary-material
References
Adams, J. K., Briski, E., Ram, J. L., and Bailey, S. A. (2014). Evaluating the response of freshwater organisms to vital staining. Manage. Biol. Invasions 5, 197–208. doi: 10.3391/mbi.2014.5.3.02
AlgaeBase (2020). World-Wide Electronic Publication, National University of Ireland, Galway. Available online at: https://www.algaebase.org (accessed October 19, 2020).
Bailey, S. A. (2015). An overview of thirty years of research on ballast water as vector for aquatic invasive species to freshwater and marine environments. Aqua. Ecosyst. Health Manage. 18, 261–268. doi: 10.1080/14634988.2015.1027129
Bailey, S. A., Brown, L., Campbell, M. L., Cannin-Clode, J., Carlton, J. T., Castro, N., et al. (2020). Trends in the detection of aquatic non-indigenous species across global marine, estuarine and freshwater ecosystems: a 50-year perspective. Divers. Distrib. 26, 1780–1797. doi: 10.1111/ddi.13167
Bakalar, G. (2016). Comparisons of interdisciplinary ballast water treatment systems and operational experiences from ships. SpringerPlus 5, 1–12.
Batista, W. R., Fernandes, F. C., Lopes, C. C., Lopes, R. S. C., Miller, W., and Ruiz, G. (2017). Which ballast water management system will you put aboard? Remnant anxieties: a mini review. Environnements 4, 54–66. doi: 10.3390/environments4030054
Bérard-Therriault, L., Poulin, M., and Bossé, L. (1999). Guide d’identification du phytoplancton marin de l’estuaire et du golfe du Saint-Laurent. Publ. Spéc. Can. Sci. Halieut. Aquat. 128.
Bradie, J., Broeg, K., Gianoli, C., He, J., Heitmüller, S., Lo Curto, A., et al. (2018). A shipboard comparison of analytic methods for ballast water compliance monitoring. J. Sea Res. 133:11. doi: 10.1016/j.seares.2017.01.006
Bradie, J. N., Drake, D. A. R., Ogilvie, D., Casas-Monroy, O., and Bailey, S. (2020). Ballast water exchange plus treatment lowers species invasion rate in freshwater ecosystems. Environ. Sci. Technol. 55, 82–89. doi: 10.1021/acs.est.0c05238
Briski, E., Allinger, L. E., Balcer, M., Cangelosi, A., Fanberg, L., Markee, T. P., et al. (2013). Multi-dimensional approach to invasive species prevention. Environ. Sci. Technol. 47, 1216–1221.
Briski, E., Gollasch, S., David, M., Linley, R. D., Casas-Monroy, O., Rajakaruna, H., et al. (2015). Combining ballast water exchange and treatment to maximize prevention of species introductions to freshwater ecosystems. Environ. Sci. Technol. 49, 9566–9573.
Burkholder, J. M., Hallegraeff, G. M., Melia, G., Cohen, A., Bowers, H. A., Oldach, D. W., et al. (2007). Phytoplankton and bacterial assemblages in ballast water of U.S. military ships as a function of port of origin, voyage time, and ocean exchange practices. Harmful Algae 6, 486–518. doi: 10.1016/j.hal.2006
Calatayud, A., Mangan, J., and Palacin, R. (2017). Vulnerability of international freight flows to shipping network disruption: a multiplex network perspective. Transport Res. Part E Logistics Transport. Rev. 108, 195–208. doi: 10.1016/j.tre.2017.10.015
Canadian Coast Guard (1989). Voluntary Guidelines for the Control of Ballast Water Discharges From Ships Proceeding to the St. Lawrence River and Great Lakes. Ottawa, ON: Government of Canada.
Carney, K. J., Minton, M. S., Holzer, K. K., Miller, A. W., McCann, L. D., and Ruiz, G. M. (2017). Evaluating the combined effects of ballast water management and trade dynamis on transfers of marine organisms by ships. PLoS One 12:e0172468. doi: 10.1371/journal.pone.0172468
Casas-Monroy, O., Linley, D. R., Adams, J. K., Chan, F., Drake, D. A. R., and Bailey, S. A. (2015). Relative invasion risk for phytoplankton across marine and freshwater systems: examining efficacy of proposed international ballast water discharge standards. PLoS One 10:e0118267. doi: 10.1371/journal.pone.0118267
Casas-Monroy, O., Parenteau, M., Drake, D. A. R., Roy, S., and Rochon, A. (2016). Absolute estimates of the propagule pressure across Canadian coasts: the variable influence of ballast water exchange. Mar. Biol. 163:174. doi: 10.1007/s00227-016-2946-3
Casas-Monroy, O., Vanden Byllaardt, J., Bradie, J. N., Sneekes, A., Kaag, K., and Bailey, S. A. (2018). Effect of temperature on chlorine treatment for elimination of freshwater phytoplankton in ballast water: bench scale test. Can. J. Fish. Aqua. Sci. 76, 1768–1780. doi: 10.1139/cjfas-2018-0179
Costa, E. G., Lopes, R. M., and Singer, J. M. (2016). Sample size for estimating the mean concentration of organisms in ballast water. J. Environ. Manage. 180, 433–438. doi: 10.1016/j.jenvman.2016.05.043
David, M., Gollasch, S., Leppäkoski, E., and Hewitt, C. (2015). Risk Assessment in Ballast Water Management. Global Maritime Transport and Ballast Water Management. New York, NY: Springer Science & Business Media.
de Lafontaine, Y., Despatie, S.-P., Veilleux, E., and Wiley, C. (2009). Onboard ship evaluation of the effectiveness and the potential environmental effects of PERACLEAN Ocean for ballast water treatment in very cold temperatures. Environ. Toxicol. 24, 49–65. doi: 10.1002/tox.20394
DESMI (2020). Ballast Water Management Technologies: A Comparison Between UV Based Systems and Electochlorination Systems. Denmark: DESMI Ocean Guard A/S.
Doblin, M., Popels, L. C., Coyne, K. J., Hutchins, D. A., Cary, S. C., and Dobbs, F. C. (2004). Transport of the Harmful Bloom Alga Aureococcus anophagefferens by oceangoing ships and coastal boats. Appl. Environ. Microbiol. 70, 6495–6500. doi: 10.1128/aem.70.11.6495-6500.2004
Environmental Systems Research Institute (ESRI) (2018). ArcGISTM 10.7.1 [Computer Software]. Redlands, CA: Environmental Systems Research Institute (ESRI).
FAO (2020). “Major Fishing Areas. Pacific, Northwest (Major Fishing Area 61), Pacific, Northeast (Major Fishing Area 67), Pacific Western Central (Major Fishing Area 71), Pacific Eastern Central (Major Fishing Area 77), CWP data collection,” in FAO Fisheries and Aquaculture Department [fao.or], (Rome: FAO).
First, M. R., Riley, S. C., Robbins-Wamsley, S. H., Parson, E. W. J., Grant, J. F., and Drake, L. A. (2020). Measurement uncertainty in determining concentrations of living organisms. Manage. Biol. Invasions 11, 493–511. doi: 10.3391/mbi.2020.11.3.10
GESAMP (2016). Group of Experts on Scientific Aspects of Marine Environmental Protection. 43rd session 43/4/1 45p. London: Publisher UN Environment.
Gollasch, S., and David, M. (2021). Abiotic and biological differences in ballast water uptake and discharge samples. Mar. Pollut. Bull. 62, 1589–1591. doi: 10.1016/j.marpolbul.2021.112046
Gregg, M., Rigby, G., and Hallegraeff, G. M. (2009). Review of two decades of progress in the development of management options for reducing or eradicating phytoplankton, zooplankton and bacteria in ship’s ballast water. Aquatic Invasions 4, 521–565. doi: 10.3391/ai.2009.4.3.14
Hallegraeff, G. M. (1998). Transport of toxic dinoflagellates via ships ballast water: bioeconomic risk assessment and efficacy of possible ballast water management strategies. Mar. Ecol. Prog. Ser. 168, 297–309. doi: 10.3354/meps168297
Hallegraeff, G. M., and Bolch, C. J. (1992). Transport of diatom and dinoflagellates resting spores in ship’s ballast water: implications for plankton biogeography and aquaculture. J. Plankton Res. 14, 1067–1084. doi: 10.1093/plankt/14.8.1067
Hamer, J. P., Lucas, I., and McCollin, T. A. (2001). Harmful dinoflagellate resting cysts in ships’ ballast tank sediments: potential for introduction into English and Welsh waters. Phycologia 40, 246–255. doi: 10.2216/i0031-8884-40-3-246.1
ICES/IOC/IMO Working Group on Ballast and Other Ship Vectors (WGBOSV) (2017). Standard Operating Procedures Collection of Treated Ballast Water Samples Using an Inline Sample Port. International Council for the Exploration of the Sea. Copenhagen: 24.
IMO (International Maritime Organization) (2004). International Convention for the Control and Management of Ships Ballast Water and Sediments. London: International Maritime Organization.
IMO (International Maritime Organization) (2008). Guidelines for Ballast Water Sampling (G2). Resolution MEPC.173(58). London: International Maritime Organization.
IMO (International Maritime Organization) (2016). Guidelines for Approval of Ballast Water Management Systems. Resolution MEPC.279(70). London: International Maritime Organization.
IMO (International Maritime Organization) (2019). Data gathering and analysis plan for the Experience-Building Phase associated with the BWM Convention. BWM.2/Circ.67/Rev.1. London: International Maritime Organization.
Klein, G., Kaczmarska, K., and Ehrman, J. M. (2009). The diatom Chaetoceros in ships’ ballast waters survivorship of stowaways. Acta Bot. Croatica 68, 325–338.
Klein, G., MacIntosh, K., Kaczmarska, I., and Ehrman, J. M. (2010). Diatom survivorship in ballast water during trans-pacific crossings. Biol. Invasions 12, 1031–1044. doi: 10.1007/s10530-009-9520-6
Lenth, R. (2019). emmeans: Estimated Marginal Means, Aka Least-Squares Means. R Package Version 1.3.5.1.
Locke, A., Reid, D. M., Vanleeuwen, H. C., Sprules, W. G., and Carlton, J. T. (1993). Ballast water exchange as a means controlling dispersal of freshwater organisms by ships. Can. J. Fish. Aquatic Sci. 50, 2086–2093. doi: 10.1139/f93-232
MacIntyre, H. L., and Cullen, J. J. (2016). Classification of phytoplankton cells as live or dead using the vital stains fluorescein diacetate and 5-chloromethylfluorescein diacetate. J. Phycol. 52, 572–589. doi: 10.1111/jpy.12415
Maranda, L., Cox, A. M., Campbell, R. G., and Smith, D. C. (2013). Chlorine dioxide as a treatment water to control invasive species: shipboard testing. Mar. Pollut. Bull. 75, 76–89. doi: 10.1016/j.marpolbul.2013.08.002
Molina, V., and Drake, L. A. (2016). Efficacy of open-ocean ballast water exchange: a review. Manage. Biol. Invasions 7, 375–388. doi: 10.3391/mbi.2016.7.4.07
Murphy, K., Boehme, J., Coble, P. G., Cullen, J., Field, P., Moore, W., et al. (2004). Verification of mid-ocean ballast water exchange using naturally occurring coastal tracers. Mar. Pollut. Bull. 48, 711–730. doi: 10.1016/j.marpolbul.2003.10.015
NSF International (2010). Generic Protocol for the Verification of Ballast Water Treatment Technology. Produced for the U.S. Environmental Protection Agency Environmental Technology Verification Program as EPA/600/R-10/146, Ann Arbor (156 pp.).
OBIS (2020). Ocean Biodiversity Information System. Intergovernmental Oceanographic Commission of UNESCO. Available online at: www.iobis.org (accessed October 22, 2020).
Paolucci, E., Hernandez, M., Potapov, A., Lewis, M. A., and MacIsaac, H. J. (2015). Hybrid system increase efficiency of ballast water treatment. J. Appl. Ecol. 52, 348–357. doi: 10.1111/1365-2664.12397
Paolucci, E., Ron, L., and MacIsaac, H. J. (2017). Combining ballast water treatment and ballast water exchange: reducing colonization pressure and propagule of phytoplankton organisms. Aquatic Ecosyst. Health Manage. 20, 369–377. doi: 10.1080/14634988.2017.1404419
R Development Core Team (2020). R: A Language and Environment for Statistical Computing. Vienna: R Foundation for statistical Computing.
Roy, S., Parenteau, M., Casas-Monroy, O., and Rochon, A. (2012). Coastal ship traffic: a significant introduction vector for potentially harmful dinoflagellates in eastern Canada. Can. J. Fish. Aquatic Sci. 69, 627–644. doi: 10.1139/f2012-008
Stehouwer, P. P., Buma, A., and Peperzak, L. (2015). A comparison of six different ballast water treatment systems based on UV radiation, electrochlorination and chlorine dioxide. Environ. Technol. 36, 2094–2104. doi: 10.1080/09593330.2015.1021858
Steinberg, M. K., Lemieux, E. J., and Drake, L. A. (2011). Determining the viability of marine protists using a combination of vital, fluorescent stains. Mar. Biol. 158, 1431–1437.
Taylor, F. J. R., Fukuyo, Y., Larsen, J., and Hallegraeff, G. M. (2003). “Taxonomy of harmful dinoflagellates,” in Manual on Harmful Marine Microalgae. Monographs on Oceanographic Methodology 11, eds G. M. Hallegraeff, D. M. Anderson, and A. D. Cembella (Paris: UNESCO Publishing), 389–432.
Taylor, F. J. R., Hoppenrath, M., and Saldarriaga, J. F. (2008). Dinoflagellates diversity and distribution. Biodivers. Conserv. 17, 407–418.
Taylor, F. J. R., and Waters, R. E. (1982). Spring phytoplankton in the subarctic North Pacific Ocean. Mar. Biol. 67, 323–335. doi: 10.1007/bf00397673
Trottet, A., George, Ch, Drillet, G., and Lauro, F. M. (2021). Aquaculture in coastal urbanized areas: a comparative review of the challenges posed by Harmful Alagal Blooms. Crit. Rev. Environ. Sci. Technol. 1–42. doi: 10.1080/10643389.2021.1897372
United Nations (1982). Third United Nations Convention of the Law of the Sea, Part V, Article 55. New York, NY: United Nations.
United States Coast Guard [USCG] (1993). Ballast Water Management for Ships Entering the Great Lakes. Code of Federal Regulations, 33-CFR Part 151.1510. Washington, DC: USCG.
Utermöhl, H. (1958). Zur Vervollkommnung der Quantitativen Phytoplankton-Methodik. Mitt. Int. Ver. Theor. Angew. Limnol. 9, 1–38. doi: 10.1080/05384680.1958.11904091
Veldhuis, M. J. W., and Kraay, G. W. (2000). Application of flow cytometry in marine phytoplankton research: current applications and future perspectives. Sci. Mar. 64, 121–134. doi: 10.3989/scimar.2000.64n2121
Keywords: aquatic invasive species, ballast water management, ballast water treatment, microalgae, phytoplankton, Pacific coast
Citation: Casas-Monroy O and Bailey SA (2021) Do Ballast Water Management Systems Reduce Phytoplankton Introductions to Canadian Waters? Front. Mar. Sci. 8:691723. doi: 10.3389/fmars.2021.691723
Received: 06 April 2021; Accepted: 23 July 2021;
Published: 19 August 2021.
Edited by:
Pedro Morais, University of Algarve, PortugalReviewed by:
Matej David, Dr. Matej DAVID Consult, Izola, SloveniaJasmine Ferrario, University of Pavia, Italy
Copyright © 2021 Casas-Monroy and Bailey. This is an open-access article distributed under the terms of the Creative Commons Attribution License (CC BY). The use, distribution or reproduction in other forums is permitted, provided the original author(s) and the copyright owner(s) are credited and that the original publication in this journal is cited, in accordance with accepted academic practice. No use, distribution or reproduction is permitted which does not comply with these terms.
*Correspondence: Oscar Casas-Monroy, T3NjYXIuY2FzYXMtbW9ucm95QGRmby1tcG8uZ2MuY2E=
†These authors have contributed equally to this work and share first authorship